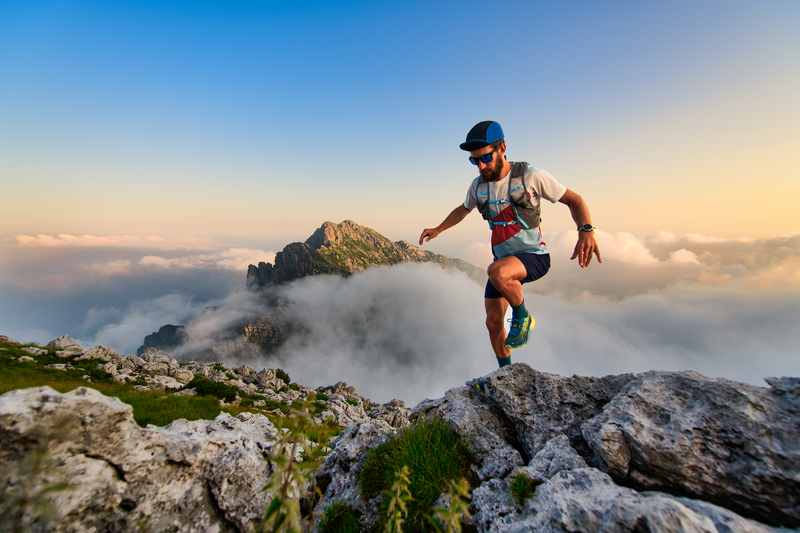
95% of researchers rate our articles as excellent or good
Learn more about the work of our research integrity team to safeguard the quality of each article we publish.
Find out more
REVIEW article
Front. Immunol. , 04 December 2018
Sec. Alloimmunity and Transplantation
Volume 9 - 2018 | https://doi.org/10.3389/fimmu.2018.02837
This article is part of the Research Topic Safety, Efficacy and Mechanisms of Action of Mesenchymal Stem Cell Therapies View all 22 articles
Mesenchymal stromal cells (MSCs) are self-renewing, culture-expandable adult stem cells that have been isolated from a variety of tissues, and possess multipotent differentiation capacity, immunomodulatory properties, and are relatively non-immunogenic. Due to this unique set of characteristics, these cells have attracted great interest in the field of regenerative medicine and have been shown to possess pronounced therapeutic potential in many different pathologies. MSCs' mode of action involves a strong paracrine component resulting from the high levels of bioactive molecules they secrete in response to the local microenvironment. For this reason, MSCs' secretome is currently being explored in several clinical contexts, either using MSC-conditioned media (CM) or purified MSC-derived extracellular vesicles (EVs) to modulate tissue response to a wide array of injuries. Rather than being a constant mixture of molecular factors, MSCs' secretome is known to be dependent on the diverse stimuli present in the microenvironment that MSCs encounter. As such, the composition of the MSCs' secretome can be modulated by preconditioning the MSCs during in vitro culture. This manuscript reviews the existent literature on how preconditioning of MSCs affects the therapeutic potential of their secretome, focusing on MSCs' immunomodulatory and regenerative features, thereby providing new insights for the therapeutic use of MSCs' secretome.
Mesenchymal stromal cells (MSCs), defined by the International Society for Stem Cell Research (ISSCR) as fibroblast-like non-hematopoietic cells, have been explored in recent years due to the clinical promise they hold for tissue repair in regenerative medicine (1, 2). They present a capacity to differentiate into multiple lineages, which was on the basis of the high number of clinical trials using MSCs. By 2015, 493 MSC-based clinical trials were reported (2), a number that greatly increased in the next 2 years, reaching a total of 861 trials in 2018 according to the official database of the US National Institutes of Health. In an effort to address this fast-increasing knowledge base, several reviews have been published to provide a thorough analysis of the evolution of MSC-based clinical trials (3, 4). Perhaps one of the best documented properties of these cells is their ability to promote regeneration in a variety of tissues and to be a major contributor to the positive results achieved in many published papers (5, 6). Indeed, up to 2015 most of the studies with MSCs had focused on their use to treat disorders of the musculoskeletal system, namely in their application to repair bone or cartilage (2). Looking beyond their potential in tissue repair and regeneration, MSCs have also been used extensively for their immunomodulatory properties, for example to treat graft-vs.-host disease (GVHD) (7) and auto-immune diseases such as lupus (8, 9), or Crohn's disease (10). Furthermore, MSCs' clinical potential has been extended to treat myocardial infarction (11, 12), stroke (13), multiple sclerosis (14, 15), liver cirrhosis (16, 17), diabetes (18, 19), lung injuries (20), among others. MSCs are known as relatively immune-inert cells (21), but depending on the context can have immunosuppressive (22–24), or immune-stimulating capacity (25, 26) (see Figure 1).
Figure 1. MSCs phenotype, differentiation potential, and immunological properties. Schematic representation of MSCs phenotype and immunological profile. (A) MSCs capacity of differentiation into osteogenic, chondrogenic and adipogenic lineages. (B) MSCs phenotype accordingly with the International Society for Stem Cell Research (ISSCR). (C) MSCs immunological profile. (D) Soluble factors families produced by MSCs and profile of interaction with immune cells.
Despite this great promise, however, their therapeutic benefits are not limited solely to their regenerative abilities. MSCs have also been referred to as trophic “factories” due to the large number of bioactive molecules they secrete in response to the local environment, which then exert paracrine effects upon neighboring cells and tissues (27). Indeed, an increasing number of authors have come to consider these paracrine or trophic properties to be the primary means by which MSCs conduct many of their therapeutic effects (28–30). This conclusion has been furthered by the observation that, in many cases, the number of differentiated cells is far too small to explain the observed response (27). Nevertheless, this paracrine action is known to be influenced by the microenvironment surrounding the cells (31). Therefore, there's a need to understand how in vitro culture conditions affect the regenerative and immunomodulatory potential of MSCs' secretome, with the ultimate goal of defining an optimal “cocktail” to precondition MSCs for a given therapeutic application. While the fast pace of research in this field is providing a large amount of data related to MSCs' therapeutic potential, an integrated investigation into how preconditioning can specifically influence the MSC secretome is lacking. To address this deficiency, we performed a comprehensive literature search on the following databases: clinicaltrials.gov, Google Scholar, Scopus, and PubMed, using either direct word-correspondence search or MESH integrated search, with several combinations of the following words: mesenchymal stem cells, hypoxia, inflammatory, pretreatment, preconditioning, stimulation, stimulus, priming, regeneration, immunomodulation, secretome, conditioned medium (CM), paracrine, therapeutic, brain, nervous system, bone, cartilage, kidney, liver, lung, pancreas, cancer, tumor, diabetes, skin, heart, cardiovascular, and intervertebral disc. The compilation of database outputs (~20,000 papers) was analyzed according to the focus of the study and relevance of the results obtained. From these results, articles found within reference lists were also screened and included when relevant to this article, considering the focus on MSCs preconditioning.
The MSCs-derived cell-free secretome appears to be able to recapitulate many of the properties/effects that have been described for the MSCs themselves. MSCs secretome is enriched in several soluble factors including cytokines, chemokines, immunomodulatory molecules, and growth factors (32). Additionally, paracrine factors produced by cells can be found encapsulated in cell-secreted vesicles. These Extracellular Vesicles (EV) are usually divided according to their size and origin in the cell into exosomes, microvesicles and apoptotic bodies. The smaller nanosized vesicle populations have deserved the most attention. Microvesicles (100–1,000 nm) originate on the plasma membrane, and exosomes (30–120 nm) that are formed in the multivesicular endosomes, have overlapping size ranges and when their separation cannot be completely ascertained are collectively designated EV (33, 34). EV content is thought to mimic that of the cells (35). The exact composition of MSCs' secretome has been investigated to identify the key molecules responsible for MSCs therapeutic potential, with the final goal being the substitution of a cell-free product to achieve the desired therapeutic effect (see Table 1) (32, 36–38, 40–43). Pro-regenerative effects of MSCs secretome have been observed in many different systems, acting by modulating the immune system (44), inhibiting cell death and fibrosis (45, 46), stimulating vascularization (44), promoting tissue remodeling, and recruiting other cells (47).
Preclinical evidence of the regenerative potential of MSCs secretome will be briefly described. ASC (adipose tissue-derived MSCs)-CM was able to regenerate/repair mandible lesions in rabbits. In the ASC-CM obtained from 24 h culture in serum-free medium under hypoxic conditions, the authors detected 43 angiogenic factors, 11 of which also appeared to be involved in bone regeneration: IGF-1, TGF-β1, VEGF, Angiogenin, IL-6, PDGF-BB, basic FGF (bFGF), EGF, RANTES, MCP-1, and MCP3 (38). This repertoire of secreted factors seemed to be in accordance with the BM-derived MSCs-CM composition reported by other authors (36, 41, 43, 48), with the remarkable exception that the BM-derived MSCs-CM also contained HGF (41) and BMP-1 (36). HGF in particular seems to be a key factor in MSCs-mediated reversal of hepatic fibrosis (49). Other studies exploring the effect of locally administered MSCs to degenerated tissues found evidence to support the notion that the soluble factors produced in response to the injury played a decisive role in the observed benefits of MSCs administration (50–52).
In the context of intervertebral disc (IVD) injury, MSCs also seem to act via a paracrine role through crosstalk with IVD cells (53–55). In an ex-vivo bovine model of pro-inflammatory/degenerated IVDs, MSCs in co-culture were able to immunomodulate the inflammatory reaction mediated by the nucleus pulposus (NP), even though few cells were found to have actually migrated to the disc (56). Zheng et al. further analyzed MSCs-CM effect on the gene expression of NP-like cells, and found an upregulation of KRT19 and downregulation of MMP12 and MGP (57). As MMP12, KRT19, and MGP have been associated with IVD degeneration, the authors suggested that a healthy NP-like phenotype could be restored by MSCs-CM. In fact, it was further proposed that the MSCs' secretome was stimulating IVD progenitor cells activity (54) and the communication mechanism between MSCs and NP cells was at least partially via secretion of microvesicles (58).
Evidence for the pivotal role of MSCs paracrine activity in injured tissues continues to arise in many different systems and pathologic conditions. In 2007, Dai et al. observed that, in myocardial infarction, using MSCs-CM had a similar, albeit less intense, effect to what had been reported earlier for MSCs per se, indicating that at least part of the effect that had been observed following MSCs injection could be attributed to soluble factors (59). In the context of neuronal damage, a local injection of MSCs to the lesion site in a stroke model improved coordinated function, inhibited scar tissue formation and cell apoptosis, and stimulated angiogenesis (60). Despite these marked improvements, no neural differentiation of the transplanted MSCs was observed, reinforcing the key role of their paracrine mode of action. Moreover, it has been established that the presence of BDNF, Glial Cell Line-derived Neurotrophic Factor (GDNF), Nerve Growth Factor (NGF), and IGF in the MSCs secretome is necessary to observe the MSCs-induced neuronal survival and differentiation both in vivo and in vitro (61). Other models in which MSCs-CM has demonstrated therapeutic efficacy include chronic kidney disease, in which administration of MSCs-CM partially rescued kidney function, mainly by attracting endothelial cells, which led to neo-angiogenesis and stimulated wound closure (62). In this study, the authors concluded that the renal-protective paracrine factors present within the MSCs-CM were likely to be VEGF, HGF, and IGF.
MSCs-derived EV, particularly exosomes, have been increasingly shown to contribute to or even completely replicate the therapeutic effects observed with the use of the entire secretome (63). They were shown to improve cardiac function after a porcine myocardial infarction, reducing infarct size and maintaining the systolic and diastolic performance, as a result of inducing neo-revascularization and modulating the inflammatory response (64). Similarly, hBM-MSCs-derived exosomes injected locally 24 h after an induced focal cerebral ischemia were able to reduce the resulting functional impairments through an increase of angioneurogenesis and the modulation of the peripheral immune response (65). Additionally, the treatment seemed to also induce long-term neuroprotection. Other studies reported that MSCs-derived exosomes could mediate the transfer of the micro RNA (miRNA)-133b to neuronal cells, which induced neurite outgrowth and functional recovery after stroke (66), hinting to the importance of this mechanism in the neuronal protective capacity exhibited by MSCs. These effects were also observed by others in different models of ischemic injury (67, 68), even though their ability to modulate the local inflammatory reaction has not been observed by all (67). In another study, a single administration of MSCs-derived microvesicles inhibited apoptosis and stimulated tubular epithelial cell proliferation, thus protecting animals from acute kidney injury (69). Bruno et al. has demonstrated as well that the treatment of acute kidney injury with MSC-EVs leads to functional improvements and reduced mortality through an inhibition of the apoptotic cascade (70). Moreover, treatment with multiple administrations was shown to be significantly more effective than a single administration of the EVs. In a similar fashion, MSCs-derived exosomes were shown to protect hepatocytes and reduce both hepatic inflammation and collagen deposition (45). Indeed, MSCs-derived vesicles have consistently been reported to play a key role in the paracrine activity of these cells.
While the preclinical evidence showing the regenerative and immunomodulatory potential of the MSCs secretome continues to expand rapidly, the clinical studies revolving around this hypothesis are still scarce. Even so, the few clinical trials performed using the product of the MSCs paracrine activity seem to have already established the safety and feasibility of this method, as none of them reported related adverse effects (71–75). Furthermore, the use of the secretome seemed to be effective in improving the clinical outcomes of the involved patients. In the case of alveolar bone regeneration, conditioned media from commercially available BM-MSCs was administered to 8 patients suffering from severe alveolar bone atrophy and needing bone augmentation (75). These patients received either porous pure beta-tricalcium phosphate (β-TCP) or shell-shaped atelocollagen sponge (ACS) scaffold grafts soaked in the CM. After the surgery, minor inflammation of the local tissues was observed with less infiltration of inflammatory cells recorded. The scaffold was gradually replaced by newly formed bone, with no records of bone resorption in any of the cases and early mineralization observed in the augmented bone. IGF-1, VEGF, TGF-β, and HGF were present in the CM, even though molecules typically involved in bone homeostasis, like BMP-2, were not detected by the methodology used.
Clinical trials addressing alopecia (73) and Female Pattern Hair Loss (74) were able to increase hair density after injecting patients not with the MSCs secretome but with a commercially available product containing its protein components. Furthermore, the treatment of one treatment-refractory GVHD patient with MSCs-derived exossomes yielded a pronounced clinical improvement shortly after the administration with a decrease in more than 50% of the IL-1β/INF-γ/TNF-α-producing peripheral blood mononuclear cells (PBMCs) (72). After 4 months, the clinical condition of the patient was still stable, indicating a long-lasting therapeutic effect of the exossomes. Currently, allogeneic MSC-derived exosomes, enriched for miR-124, are reported in a registered clinical trial, directed to stroke patients (http://clinicaltrials.gov).
Although MSCs have an innate potential to induce and/or contribute to regeneration, this potential is now known to be greatly influenced by diverse extrinsic factors such as the tissue source of the MSCs, the health status and age of the MSCs donor, the batch/lot of serum used for the in vitro culture of the MSCs, passage number, oxygen concentration, and the presence/absence of a pro-inflammatory environment when the MSCs are infused (76–82). Thus, in vitro preconditioning of MSCs with a variety of different factors has been explored to enhance the therapeutic capacity/potential of MSCs, which included: 3D culture (83–85), pharmacological compounds (86–88), inflammatory cytokines (89, 90), and hypoxia (91, 92) (Table 2). Considering MSCs main mechanism of action upon transplantation might be via paracrine signaling, it is somewhat surprising that only a few groups have studied how preconditioning of MSCs affects their secretory profile. This is particularly relevant when the MSCs' secretome may ultimately prove to be an extremely valuable therapeutic tool. The influence of these factors on MSCs' secretome will be reviewed in the following section.
Normoxic oxygen tension, as used for standard cell culture, is the atmospheric pressure (21% O2). The term hypoxia, when employed in the context of cell culture is routinely used to refer to oxygen tensions ranging from 0 to 10% (108). The physiological oxygen tension in tissues can vary from 1% in cartilage and bone marrow to 12% in peripheral blood (109). Thus, the 21% O2 routinely used for MSCs culture is far higher than the oxygen found physiologically.
In general, hypoxic preconditioning enhances MSCs' regenerative and cytoprotective effects (82, 91–98). Moreover, culturing MSCs in hypoxic conditions has been shown to maintain MSCs' multipotency (110), enhance MSCs proliferation (111), and increase their levels of cytoprotective molecules (98) (Table 3), thereby improving the ability of MSCs to survive in the harsh environment found within injury sites upon transplantation. The beneficial effects of hypoxic culture preconditioning can likely be explained by the fact that MSCs exist in vivo in hypoxic environments (131) and hence have the ability to respond to a hypoxic microenvironment through the upregulation of the transcription factor HIF-1α (132). When stabilized due to the lack of oxygen, and dependent upon the increase of phosphorylated Akt and p38 mitogen-activated protein kinase (p38MAPK), this factor binds to the promoter regions of genes responsive to hypoxia, leading to an increase in available glucose (109). As MSCs are capable of switching from aerobic to anaerobic metabolic pathways, they are then able to endure very low oxygen tension values in their microenvironment (133). Therefore, using these culture conditions to precondition MSCs enhances their capacity to survival for longer periods, increases their proliferation rate, and maintains them in an undifferentiated state (109, 131). Small differences, however, in the oxygen tension used to culture MSCs, and in the culture protocol itself, can influence both their ability to differentiate into each of the different mesenchymal lineages (134) and their paracrine production (109). This extreme sensitivity to oxygen tension is an important factor to bear in mind when analyzing results from studies using different preconditioning protocols. The various studies to-date that have used hypoxia as a means of preconditioning MSCs used a concentration up to 2% O2, for a time period of 4–72 h (Table 4). Unfortunately, a high degree of variability exists between the protocols that have been employed, and this must be considered when assessing the MSCs' therapeutic function.
HIF-1α activation due to preconditioning MSCs with hypoxia leads to the induction of factors such as VEGF and Angiotensin, promoters of vascularization (136, 137). As neovascularization is a key factor in the regenerative process of damaged tissues, this may account, in itself, for the better therapeutic capacity that has been seen with MSCs pretreated with hypoxia. This hypothesis is supported by a growing number of publications identifying VEGF as a crucial molecule for the observed pro-regenerative effects of MSCs (47, 121, 138, 139). Liu et al. described a direct impact of the hypoxia-preconditioned MSCs treatment on endothelial cell proliferation with a simultaneous reduction in apoptosis (139). In addition, infusion of hypoxia-preconditioned BM-MSCs into the portal vein of rats subject to hepatectomy promoted hepatocyte proliferation and survival and improved serum albumin levels after surgery through a TGF-β dependent mechanism (95). Again, increased production of VEGF was observed. Hypoxia-preconditioning induced MSCs to express higher levels of HIF-1α, and the growth factors GDNF, BDNF, VEGF, Ang-1, and SDF-1, as well as its receptor CXCR4, all of which have been linked to neovascularization, as well as EPO and its receptor EPOR, a neuroprotective and pro-angiogenic molecule (120). Also, when using specifically hypoxia-preconditioned MSCs-derived EVs to treat acute myocardial infarction, authors reported the importance of the increased vascularization in the therapeutic effects. Bian et al. observed that EVs derived from BM-MSCs preconditioned with hypoxia for 72 h were able to significantly improve cardiac function after acute myocardial infarction, mainly through the promotion of angiogenesis (140). Indeed, a comprehensive proteomic analysis of exosomes derived from hypoxia-exposed MSCs showed that these exosomes induce angiogenesis in endothelial cells via the activation of the NFκB pathway (141). However, in another study exosomes derived from hypoxia-preconditioned MSCs contributed to the attenuation of the injury resulting from an ischemia/reperfusion episode via the Wnt signaling pathway (142). Beyond that, hypoxia seems to increase exosome secretion in general (141). Also, in a fat graft model, co-transplantation of exosomes from hypoxia pre-conditioned adipose-derived MSC improved vascularization and graft survival (143) (see Table 5).
Nevertheless, other growth factors are also upregulated in response to this stimulus (43, 46, 147) (Table 3), and these factors likely contribute to the specificity of tissue regeneration in a variety of scenarios. An analysis of the hypoxia-preconditioned MSCs' CM used to treat wounds in diabetic rats, revealed higher levels of VEGF, IGF-1, and bFGF (94), while another study reported increased production of VEGF-1α and Bcl-2, with upregulation of HIF-1α, HGF, bFGF, MMP9, and PDGF in MSCs pretreated with hypoxia (101). In agreement with these aforementioned studies, Zhang and colleagues observed that pre-treatment with hypoxia led to increased levels of VEGF, bFGF, and Akt that were implicated in the enhancement of MSCs' anti-oxidative, anti-apoptotic, and pro-angiogenic effects in a rat model of acute kidney injury (96). Additionally, other studies showed that both hypoxia and, to an even greater degree, forced overexpression of Akt, upregulated expression of VEGF, bFGF, HGF, IGF, and TB4, molecules associated with tissue repair and regeneration (113). The Akt signaling pathway was also reported to play a role in the enhanced wound healing observed in mice treated with the secretome from hypoxia-preconditioned MSCs (121). The effect of this secretome was related to increased levels of fibronectin, AKT, PI3K, and SMAD2 in the injured tissue; molecules that are all involved in cell proliferation and migration. The hypoxia-preconditioned MSCs secretome was also shown to contain higher levels of VEGF and TGF-β, which led to increased cell proliferation and migration of dermal fibroblasts, via the TGF-β/SMAD2 and PI3K/AKT signaling pathways (121). These results were further validated by Chen et al. who demonstrated that the hypoxia-preconditioned MSCs secretome significantly increased proliferation and migration of keratinocytes, fibroblasts, endothelial cells, and monocytes in vitro, and that skin wound contraction was accelerated in an in vivo mouse model (47). The secretome produced by hypoxia-preconditioned placenta-derived MSCs was also shown to reduce scar formation and inhibit proliferation and migration of skin fibroblasts in vitro (126). In this case, IL-10 was identified as the key player in the process. In agreement with all these results, Lan and colleagues reported increased expression of anti-apoptotic (HGF, Bcl-2), anti-oxidative (catalase, HO-1), and pro-angiogenic (VEGF) factors in hypoxia-treated BM-MSCs infused with the goal of improving the respiratory function of mice suffering from pulmonary fibrosis (98). Chen et al. observed an increase in the MSCs production levels of not only VEGF-A and bFGF, but also IL-6 and IL-8 (molecules involved in the inflammatory response) under hypoxic conditions (47).
The cytoprotective effect of the hypoxia-pretreatment of MSCs, along with changes in metabolism and maintenance of their differentiation potential, have now been repeatedly demonstrated by a variety of authors, despite differences in the hypoxic conditions used (138, 148). From these studies, it has been concluded that hypoxia-preconditioning increases MSCs' survival in harsh environments (148) and enhances their angiogenic capacity, which together boost MSCs' regenerative and immunomodulatory abilities, contributing to the regulation of excessive fibrosis and cell death due to uncontrolled inflammation (96, 98, 101).
When considering a significant amount of experimental data regarding MSCs preconditioning with inflammatory cytokines, it is readily apparent that such a stimulus seems to predominantly promote an increase in the production of factors involved in the regulation of the immune response (see Table 3). This includes chemoattraction of most immune cells, modulation of inflammation, and even enhancing migration and homing of transplanted MSCs to sites with higher concentrations of such inflammatory molecules. Their immunoregulatory abilities encompass the inhibition of the complement system activation, the inhibition of NK cells, the guidance of monocyte differentiation toward anti-inflammatory macrophages (M2 phenotype), the suppression of cytotoxic T cell proliferation, and the increase in the numbers of regulatory T cells (149). Many of these outcomes are explained by the large number of chemokines produced by the MSCs that effectively attract numerous immune cells to resolve an inflammatory response (150). Specifically, IL-6, PGE2, and IDO all seem to be major effector molecules in the immunoregulatory effects MSCs mediate (123, 151, 152). The production of this potent triad of immunomodulatory molecules is stimulated by the presence of pro-inflammatory factors such as IL-1β, TNF-α, IFN-γ, and LPS (22, 42, 123, 153, 154), that induce MSCs to adopt an immunomodulatory phenotype and to trigger the production of a cocktail of growth factors. These studies thus collectively indicate the close relationship that exists between inflammation and regeneration. In agreement with this supposition, the therapeutic effects that were observed with TNF-α treated MSCs in a wound closure model, mainly mediated by increased angiogenesis and immune cells infiltration, were observed to be dependent on increased levels of IL-6 and IL-8 (116). Indeed, a recent publication featuring an extensive proteomic analysis of the secretome from BM-MSCs preconditioned with pro-inflammatory factors (IL-1β, IL-6, and TNF-α) clearly demonstrates how a pro-inflammatory stimulus mainly increases MSCs production of proteins involved in inflammation and angiogenesis (155). Moreover, the authors also explore the idea that MSCs role in regulating the proteolytic activity in tissues is key for the regulation of these processes.
Still, the mechanism by which these factors seem to influence MSCs is still largely undefined. There is evidence that MSCs immunomodulatory abilities are mediated by both cell-to-cell contact-derived mechanisms (156–158) and paracrine communication (159–161). Also, some authors believe that MSCs are not naturally immunosuppressive and thus, need licensing at the site of inflammation to become so (162–165). This theory is supported by results demonstrating that molecules such as IFN-γ, TNF-α, or IL-1β are necessary to activate the MSCs immunomodulatory activity (166, 167). One study exploring the effect of the preconditioning with TNF-α on MSCs-derived exosomes demonstrated that the effect the stimulatory effects these vesicles had on human osteoblasts was potentiated through increase of Wnt-3a content in ASC-exosomes (168). Conversely, IFN-γ priming of MSC before EV isolation was reported not to influence the immunomodulatory capacity of exosomes or microparticles, which displayed dose-dependent immunomodulatory effects in inflammatory animal models (169). Additionally, TLRs (Toll-Like Receptor) have also been implicated as important mediators of this activation. Optiz et al. reported that activation of TLR3 and TLR4 lead to the induction of IDO which, in turn, mediated the immunosuppressive actions of the MSCs (170). Activation of TLR-2 was shown to cause an increase in the production of galectin-3 by MSCs and, thus, potentiate their capacity to suppress T-cell activation (171). Nonetheless, contradictory reports have also been published. Liotta et al. demonstrated that TLR3 and TLR4 activation not only increased the production of pro-inflammatory molecules but also reduced their inhibitory effect on the proliferation of T-cells (172). Furthermore, they observed that the activation of these TLRs didn't have any effect on levels of IDO. More recently, along with the demonstration that priming with IFN-γ enhanced MSCs immunosuppressive abilities, mainly through the induction of IDO, it was also shown that TLR3 activation did not affect IDO levels and did not influence the cells immunosuppressive activity (165).
Preconditioning with a myriad of other soluble factors, such as growth factors or hormones, seems to also potentiate MSCs regenerative capacity, mainly by stimulating angiogenesis and inhibiting fibrosis. For example, intracardiac transplantation of SDF-1-preconditioned MSCs increased angiogenesis and reduced fibrosis in the ischemic area of a post-infarct heart (89). The effects observed were attributed to the activation of the Akt signaling pathway, similarly to what was described for hypoxia-preconditioned MSCs. TGF-α-preconditioned MSCs enhanced cardiac function mainly through increased VEGF production via a p38 MAPK-dependent mechanism (90). TNF-α or hypoxia were then combined with the TGF-α during prestimulation, and this led to a further improvement in cardiac function. Once more, VEGF seemed to play a key role in MSCs' mode of action. Preconditioning MSCs with a cocktail of growth-factors (FGF-2, IGF-1, and BMP-2) was also attempted, and this was found to yield protective effects on cardiomyocytes and to improve left ventricular systolic function in a rat myocardial infarction model (173). Another soluble molecule that has been used to precondition MSCs is melatonin, which activates the ERK 1/2 signaling pathway, and consequently enhances cell survival under oxidative stress (100). Thus, melatonin-preconditioned MSCs increased angiogenesis and neurogenesis, reduced infarct size, and improved neurobehavioral outcome in a rat cerebral ischemia model, and once more this seems to have been related to increased VEGF levels (100). Melatonin-preconditioned MSCs also exhibited significantly higher survival rates after intraparenchymal injection in a rat kidney ischemia model (99). This effect was attributed to an upregulation of the enzymes catalase and superoxide dismutase-1 that imbued MSCs with greater antioxidant capacity. Lastly, H2O2 has been used to precondition MSCs whose exosomes were used to treat and ischemia/reperfusion injury in a rat model (174). The treatment lead to increased vascularization, which led to higher survival rates, and a reduced inflammatory reaction.
MSCs culture in a 3-dimensional (3D) environment is another type of preconditioning that aims to more closely mimic the physiological conditions which the cells would see in vivo. 3D culture of MSCs, namely as spheroids, induces an increase in the production of factors associated with cell survival and proliferation and vascularization (129, 175) (Table 3). This, in turn, has been shown to increase these cells' immunomodulatory, angiogenic, anti-fibrotic, and anti-apoptotic activities (83, 85, 104, 176). MSCs spheroids decreased neutrophil activity, the levels of the pro-inflammatory molecules TNF-α, IL-1β, CXCL2/MIP-2, PGE2, and plasmin activity in a mouse peritonitis model (83). Other studies demonstrated that 3D culture of MSCs in spheroids seems to stimulate the cells' pro-angiogenic ability, as demonstrated by the implantation of AT-MSCs aggregates leading to an improvement in renal function in a rat model of acute renal ischemia/reperfusion (85). As previously mentioned, the 3D culture of MSCs has also been demonstrated to increase their anti-fibrotic potential. MSCs spheroids were shown to decrease tissue fibrosis in a mouse model of hepatic fibrosis (104). However, in another model system, MSCs' anti-fibrotic activity was shown to be dependent on the dose of MSCs aggregates, with higher density aggregates being unable to regenerate the cartilage in rabbits suffering from full-thickness osteochondral defects (84). Administration of 3D cultures of MSCs aggregates (with more than 125,000 cells) during 24 h increased wound healing rate in mice with full-thickness diabetic wounds. Lower cell doses yielded results similar to those observed in the vehicle-treated mice (76), supporting the notion that the dose used is key.
The spheroid 3D culture creates a microenvironment where inner layers are exposed to much lower levels of oxygen and nutrients, originating an hypoxic environment (177). Although, as a consequence, MSCs express higher levels of molecules associated with apoptosis (32), their immunomodulatory capacity seems secured by the diverse and abundant production of factors involved in inflammation and immune response (83, 114, 177) (Table 3). Accordingly, 3D MSCs-preconditioning was shown to upregulate TSG-6 expression, as well as SCT-1 (anti-inflammatory/anti-apoptotic protein), LIF, IL-24, TRAIL, and CXCR4, a chemokine involved in spheroid-derived-duction of angiogenic factors such as AngiMSCs adhesion to endothelial cells. Wnt signaling cascade seemed to be involved in this effect as the expression of its inhibitor DKK1 was decreased (175). In fact, 3D pre-conditioning of MSCs demonstrated an influence in the production of, not only inflammatory cytokines, but also matrix constituents and degrading enzymes (76, 84, 85), which contributes to their anti-fibrotic capacity. Culturing MSCs as spheroids seems to further enhance their innate pro-angiogenic ability, presumably by increasing their production of angiogenic factors such as Angiogenin, bFGF, VEGF, and HGFa (85, 129). In addition, culturing MSCs in this more physiologically relevant 3D architecture was found to increase their expression of E-cadherin which, by activating the ERK/AKT signaling pathway, was responsible for the higher levels of VEGF production observed (178).
Pre-conditioning of MSCs with pharmacological agents may be considered as an alternative option in specific cases. For example, MSCs were pre-conditioned with atorvastatin (a statin associated with the prevention of cardiovascular disease events) (107), oxytocin (hormone) (179), Curcumin (strong anti-oxidant with anti-inflammatory properties) (180), lipopolysaccharide (LPS—endotoxin) (105), and diazoxide (used as a vasodilator in acute hypertension) (86), and their ability to treat myocardial infarction tested. All of the preconditioning protocols enhanced the survival of the transplanted MSCs in vivo and led to improved functional recovery and reduced infarct size (86, 105, 107, 179, 180). In general, these effects were due to increased neovascularization and reduced tissue fibrosis. All the studies, except for those using atorvastatin and oxytocin (86, 105, 180) reported a link between the observed effect and increased levels of VEGF, FGF-2, or HGF and activation of the Akt signaling pathway. In concordance, MSCs-derived EVs obtained after preconditioning with Buyang Huanwu Decoction (BYHWD), a drug that has been used for centuries for the treatment of paralysis and stroke, was shown to attenuate brain injury in a rat local cerebral ischemia model by increasing local VEGF levels (146). Atorvastatin seemed to potentiate MSCs' immunomodulatory capacity, decreasing the infiltration of inflammatory cells and the levels of TNF-α and IL-6, via a CXCR4-dependent mechanism, which the authors concluded was the primary mediator of the improvement of functional recovery and reduction of infarct size in a rat stroke model upon MSCs injection (106). Thus, it is becoming clear that MSCs' paracrine response is as dynamic as the microenvironment that surrounds them. Even minor changes in culture conditions or in the microenvironment of the injured tissue can induce dramatically different results (32).
Extensive evidence now exists to support the benefits of preconditioning of MSCs with respect to improving their capacity to induce regeneration/repair across the wide array of tissues and pathologic conditions in which these cells have been explored. Depending on the preconditioning factors used in the MSCs' culture, different signaling pathways are activated. Understanding how each different stimulus affects MSCs behavior is crucial to validate MSCs preconditioning as a tool to enhance both the safety and the disease-specific therapeutic potential of MSCs for clinical use.
Although a great deal of work to comprehend the full mechanisms of MSCs paracrine signaling upon pre-conditioning is needed, some patterns can be recognized (see Figure 2). In summary, pre-conditioning of MSCs with hypoxia, 3D structural organization or soluble factors as SDF-1, or TGF-β seem to activate Akt, ERK, and p38MAPK signaling pathways, that seem to increase the production of cytoprotective molecules (Catalase, HO-1, etc.), pro-regenerative (bFGF, HGF, IGF, etc.) and pro-angiogenic (VEGF) soluble factors and immunomodulatory cytokines (IDO, PGE2, IL6, etc.). On the other hand, priming of MSCs with inflammatory cytokines such as IFN-γ, IL-1β activate TLRs (namely, TLR-2/3/4) on MSCs surface which then increases the production of similar cytoprotective, pro-regenerative, pro-angiogenic and immunomodulatory molecules and further promotes chemokines secretion. Nevertheless, to-date, it has not been possible to identify one single mechanism responsible for this effect.
Figure 2. The effect of different preconditioning stimuli in the MSCs response. Schematic representation of known effects of highly studied preconditioning factors—hypoxia (in blue), 3D culture (in blue), specific soluble factors (green), and inflammatory cytokines (red)—in the MSCs response. Blue pathway presents the effect of a hypoxic environment on the cells, which is mediated by specific signaling pathaways (Akt, ERK, p38MAPK) and culminates in the stimulation of the above signaled effects. Tridimensional culture is also represented in blue. MSCs preconditioning with specific soluble factors (SDF-1, TGF-α, and melatonin) seems to stimulate the same signaling pathways as a hypoxic environment and, thus, elicit the same general response from these cells. The use of inflammatory cytokines to influence the MSC response, as represented in red, besides promoting the specific above shown effects, also stimulates the production of factors that seem to be common to all the other preconditioning factors. The pathways that mediate this activity are still to be determined.
Moreover, data on the effects that preconditioning of MSCs exerts on the composition and therapeutic potential of their secretome is still lacking. Most studies have focused solely on the effects of hypoxia on the MSCs' secretome content and its therapeutic potential or, in alternative, on the effect that other preconditioning factors could exert on its composition. In most of the studies, there is a lack of evidence on the influence of preconditioning on both the therapeutic effect of the secretome and its composition. Perhaps most importantly, the great majority of studies exploring the clinical utility of the MSCs' secretome have tended to utilize a fairly myopic approach to study its composition, focusing on specific factors of interest in the unique pathological setting being explored, which has contributed to the difficulty in gathering a widely applicable understanding of how the secretome can be fine-tuned for maximal effect in each specific pathology.
Furthermore, due to the wide heterogeneity in MSCs employed in the research community, with respect to their tissue of origin, the health and age of the donor, protocols of cell isolation, culture and preconditioning, as well as the animal model used for testing, it becomes rather difficult to dissect the mechanistic action behind the observed effects on preconditioning of MSCs. Advances in high-throughput techniques and bioinformatic tools, in combination with a database in the area, would help to create a more comprehensive and complete understanding of the way preconditioning can be fine-tuned to increase MSCs' therapeutic utility in the future. This aspect of big data is particularly relevant when the number of studies using MSCs secretome is increasing, accomplished by an expansion of the EVs/exossomes field, in which their secretion by MSCs is being largely explored (181, 182).
Cell therapies as established so far, notwithstanding its great promise, present several obstacles concerning safety, process standardization, and practicality of the procedures needed to deliver viable cells to the hostile microenvironment often present within injured tissues (49, 183, 184). A consensus in the shifting of MSCs' therapeutic potential to their paracrine mechanism of action is being formed within the scientific community, which points to the development of guidelines to refine the experimental settings of the production of MSCs secretome, to establish more standardized protocols among the scientific community and to promote future collaborative work to close the wide gap that has existed for decades between MSCs experimental research and their clinical use.
JF and RG conceived the main conceptual ideas and outlined the proof. JF gathered, analyzed, and sorted the revised literature and wrote the manuscript. RG, GT, SS, GA-P, and MB revised and provided critical feedback on the manuscript. RG provided funding.
The authors declare that the research was conducted in the absence of any commercial or financial relationships that could be construed as a potential conflict of interest.
We would like to acknowledge Norte Portugal Regional Operational Programme (NORTE 2020) in the framework of the project “Bioengineered Therapies for Infectious Diseases and Tissue Regeneration” (NORTE-01-0145-FEDER-000012). We also acknowledge Fundação para a Ciência e a Tecnologia (FCT) and Fundo Europeu de Desenvolvimento Regional (FEDER) funds through the COMPETE 2020-Operacional Programme for Competitiveness and Internationalization (POCI), Portugal 2020-in the framework of the project “Institute for Research and Innovation in Health Sciences” (POCI-01-0145-FEDER-007274). We also acknowledge EUROSPINE TRF for the funded project “Disc Regeneration, Immuno, and Neuro Modulation”, ref. 2017_05 . In addition, JF and RG also acknowledge FCT for funding the BiotechHealth Ph.D. fellowship (PD/BD/135486/2018) and the FCT Investigator Grant (IF/00638/2014), respectively.
1. Ullah I, Subbarao RB, Rho GJ. Human mesenchymal stem cells - current trends and future prospective. Biosci Rep. (2015) 35:2. doi: 10.1042/BSR20150025
2. Squillaro T, Peluso G, Galderisi U. Clinical trials with mesenchymal stem cells: an update. Cell Trans. (2016) 25:829–48. doi: 10.3727/096368915X689622
3. Wang J, Liao L, Tan J. Mesenchymal-stem-cell-based experimental and clinical trials: current status and open questions. Expert Opin Biol Ther. (2011) 11:893–909. doi: 10.1517/14712598.2011.574119
4. Kim N, Cho SG. Clinical applications of mesenchymal stem cells. Korean J Intern Med. (2013) 28:387–402. doi: 10.3904/kjim.2013.28.4.387
5. Richardson SM, Hoyland JA, Mobasheri R, Csaki C, Shakibaei M, Mobasheri A. Mesenchymal stem cells in regenerative medicine: opportunities and challenges for articular cartilage and intervertebral disc tissue engineering. J Cell Physiol. (2010) 222:23–32. doi: 10.1002/jcp.21915
6. Rohban R, Pieber TR. Mesenchymal stem and progenitor cells in regeneration: tissue specificity and regenerative potential. Stem Cells Int. (2017) 2017:1–16. doi: 10.1155/2017/5173732
7. Christensen ME, Turner BE, Sinfield L, Kollar JK, Cullup H, Waterhouse NJ, et al. Mesenchymal stromal cells transiently alter the inflammatory milieu post-transplant to delay graft-versus-host disease. Haematologica (2010) 95:2102–10. doi: 10.3324/haematol.2010.028910
8. Sun L, Akiyama K, Zhang H, Yamaza T, Hou Y, Zhao S, et al. Mesenchymal stem cell transplantation reverses multi-organ dysfunction in systemic lupus erythematosus mice and humans. Stem Cells (2009) 27:1421–32. doi: 10.1002/stem.68
9. Liang J, Zhang H, Hua B, Wang H, Lu L, Shi S, et al. Allogenic mesenchymal stem cells transplantation in refractory systemic lupus erythematosus: a pilot clinical study. Ann Rheum Dis. (2010) 69:1423–9. doi: 10.1136/ard.2009.123463
10. Ciccocioppo R, Bernardo ME, Sgarella A, Maccario R, Avanzini MA, Ubezio C, et al. Autologous bone marrow-derived mesenchymal stromal cells in the treatment of fistulising Crohn's disease. Gut (2011) 60:788–98. doi: 10.1136/gut.2010.214841
11. Amado LC, Saliaris AP, Schuleri KH, St John M, Xie JS, Cattaneo S, et al. Cardiac repair with intramyocardial injection of allogeneic mesenchymal stem cells after myocardial infarction Proc Natl Acad Sci USA. (2005) 102:11474–9. doi: 10.1073/pnas.0504388102
12. Cai M, Shen R, Song L, Lu M, Wang J, Zhao S, et al. Bone marrow mesenchymal stem cells (BM-MSCs) improve heart function in swine myocardial infarction model through paracrine effects. Sci Rep. (2016) 6:28250. doi: 10.1038/srep28250
13. Bang OY, Lee JS, Lee PH, Lee G. Autologous mesenchymal stem cell transplantation in stroke patients. Ann Neurol. 57:874–82. doi: 10.1002/ana.20501
14. Mohyeddin Bonab M, Yazdanbakhsh S, Lotfi J, Alimoghaddom K, Talebian F, Hooshmand F, et al. Does mesenchymal stem cell therapy help multiple sclerosis patients? Rep Pilot Study Iran J Immunol. (2007) 4:50–7.
15. Karussis D, Karageorgiou C, Vaknin-Dembinsky A, Gowda-Kurkalli B, Gomori J, Kassis IM, et al. Safety and immunological effects of mesenchymal stem cell transplantation in patients with multiple sclerosis and amyotrophic lateral sclerosis. Arch Neurol. (2010) 67:1187–94. doi: 10.1001/archneurol.2010.248
16. Kharaziha P, Hellstrom PM, Noorinayer B, Farzaneh F, Aghajani K, Jafari F, et al. Improvement of liver function in liver cirrhosis patients after autologous mesenchymal stem cell injection: a phase I-II clinical trial. Eur J Gastroenterol Hepatol. (2009) 21:1199–205. doi: 10.1097/MEG.0b013e32832a1f6c
17. Shi M, Zhang Z, Xu R, Lin H, Fu J, Zou Z, et al. Human mesenchymal stem cell transfusion is safe and improves liver function in acute-on-chronic liver failure patients. Stem Cells Transl Med. (2012) 1:725–31. doi: 10.5966/sctm.2012-0034
18. Chang C, Wang X, Niu D, Zhang Z, Zhao H, Gong F. Mesenchymal stem cells adopt beta-cell fate upon diabetic pancreatic microenvironment. Pancreas (2009) 38:275–81. doi: 10.1097/MPA.0b013e318191521c
19. Holmes D. Diabetes: MSC transplant prevents [beta]-cell dysfunction. Nat Rev Endocrinol. (2014) 10:701. doi: 10.1038/nrendo.2014.172
20. Kursova LV, Konoplyannikov AG, Pasov VV, Ivanova IN, Poluektova MV, Konoplyannikova OA. Possibilities for the use of autologous mesenchymal stem cells in the therapy of radiation-induced lung injuries. Bull Exp Biol Med. (2009) 147:542–6. doi: 10.1007/s10517-009-0538-7
21. Chamberlain G, Fox J, Ashton B, Middleton J. Concise review: mesenchymal stem cells: their phenotype, differentiation capacity, immunological features, and potential for homing. Stem Cells (2007) 25:2739–49. doi: 10.1634/stemcells.2007-0197
22. Aggarwal S, Pittenger MF. Human mesenchymal stem cells modulate allogeneic immune cell responses. Blood (2005) 105:1815–22. doi: 10.1182/blood-2004-04-1559
23. Casiraghi F, Azzollini N, Cassis P, Imberti B, Morigi M, Cugini D, et al. Pretransplant infusion of mesenchymal stem cells prolongs the survival of a semiallogeneic heart transplant through the generation of regulatory T cells. J Immunol. (2008) 181:3933–46. doi: 10.4049/jimmunol.181.6.3933
24. Jarvinen L, Badri L, Wettlaufer S, Ohtsuka T, Standiford T, Toews GB, et al. Lung resident mesenchymal stem cells isolated from human lung allografts inhibit T cell proliferation via a soluble mediator. J Immunol. (2008) 181:4389–96. doi: 10.4049/jimmunol.181.6.4389
25. Chan JL, Tang KC, Patel AP, Bonilla LM, Pierobon N, Ponzio NM, et al. Antigen-presenting property of mesenchymal stem cells occurs during a narrow window at low levels of interferon-gamma. Blood (2006) 107:4817–24. doi: 10.1182/blood-2006-01-0057
26. Stagg J, Pommey S, Eliopoulos N, Galipeau J. Interferon-gamma-stimulated marrow stromal cells: a new type of nonhematopoietic antigen-presenting cell. Blood (2006) 107:2570–7. doi: 10.1182/blood-2005-07-2793
27. Caplan AI, Dennis JE. Mesenchymal stem cells as trophic mediators. J Cell Biochem. 98: 1076–84. doi: 10.1002/jcb.20886
28. Picinich SC, Mishra PJ, Mishra PJ, Glod J, Banerjee D. The therapeutic potential of mesenchymal stem cells. Cell- & tissue-based therapy. Expert Opin Biol Ther. (2007) 7:965–73. doi: 10.1517/14712598.7.7.965
30. Vizoso FJ, Eiro N, Cid S, Schneider J, Perez-Fernandez R. Mesenchymal stem cell secretome: toward cell-free therapeutic strategies in regenerative medicine. Int J Mol Sci. (2017) 18:1852. doi: 10.3390/ijms18091852
31. Baraniak PR, McDevitt TC. Stem cell paracrine actions and tissue regeneration. Regenerat Med. (2010) 5:121–43. doi: 10.2217/rme.09.74
32. Madrigal M, Rao KS, Riordan NH. A review of therapeutic effects of mesenchymal stem cell secretions and induction of secretory modification by different culture methods. J Transl Med. (2014) 12:1–14. doi: 10.1186/s12967-014-0260-8
33. Lötvall J, Hill AF, Hochberg F, Buzás EI, Di Vizio D, Gardiner C, et al. Minimal experimental requirements for definition of extracellular vesicles and their functions: a position statement from the International Society for Extracellular Vesicles. J Extracellu Vesicles (2014) 3, 1–6. doi: 10.3402/jev.v3403.26913
34. Stahl PD, Raposo G. Exosomes and extracellular vesicles: the path forward. Essays Biochem. (2018) 62:119–24. doi: 10.1042/EBC20170088
35. Camussi G, Deregibus MC, Bruno S, Cantaluppi V, Biancone L. Exosomes/microvesicles as a mechanism of cell-to-cell communication. Kidney Int. (2010) 78:838–48. doi: 10.1038/ki.2010.278
36. Polacek M, Bruun JA, Elvenes J, Figenschau Y, Martinez I. The secretory profiles of cultured human articular chondrocytes and mesenchymal stem cells: implications for autologous cell transplantation strategies. Cell Trans. (2011) 20:1381–93. doi: 10.3727/096368910X550215
37. Kyurkchiev D, Bochev I, Ivanova-Todorova E, Mourdjeva M, Oreshkova T, Belemezova K, et al. Secretion of immunoregulatory cytokines by mesenchymal stem cells. World J Stem Cells (2014) 6:552–70. doi: 10.4252/wjsc.v6.i5.552
38. Linero I, Chaparro O. Paracrine effect of mesenchymal stem cells derived from human adipose tissue in bone regeneration. PLoS ONE (2014) 9:e107001. doi: 10.1371/journal.pone.0107001
39. Pereira T, Ivanova G, Caseiro AR, Barbosa P, Bártolo PJ, Santos JD, et al. MSCs conditioned media and umbilical cord blood plasma metabolomics and composition. PLoS ONE (2014) 9:e113769. doi: 10.1371/journal.pone.0113769
40. Wang M, Crisostomo PR, Herring C, Meldrum KK, Meldrum DR. Human progenitor cells from bone marrow or adipose tissue produce VEGF, HGF, and IGF-I in response to TNF by a p38 MAPK-dependent mechanism. Am J Physiol Regul Integr Comp Physiol. (2006) 291:R880–4. doi: 10.1152/ajpregu.00280.2006
41. Inukai T, Katagiri W, Yoshimi R, Osugi M, Kawai T, Hibi H, et al. Novel application of stem cell-derived factors for periodontal regeneration. Biochem Biophys Res Commun. (2013) 430:763–8. doi: 10.1016/j.bbrc.2012.11.074
42. Liu CH, Hwang S-M. Cytokine interactions in mesenchymal stem cells from cord blood. Cytokine (2005) 32:270–9. doi: 10.1016/j.cyto.2005.11.003
43. Osugi M, Katagiri W, Yoshimi R, Inukai T, Hibi H, Ueda M. Conditioned media from mesenchymal stem cells enhanced bone regeneration in rat calvarial bone defects. Tissue Eng Part A (2012) 18:1479–89. doi: 10.1089/ten.tea.2011.0325
44. Teng X, Chen L, Chen W, Yang J, Yang Z, Shen Z. Mesenchymal stem cell-derived exosomes improve the microenvironment of infarcted myocardium contributing to angiogenesis and anti-inflammation. Cell Physiol Biochem. (2015) 37:2415–24. doi: 10.1159/000438594
45. Li T, Yan Y, Wang B, Qian H, Zhang X, Shen L, et al. Exosomes derived from human umbilical cord mesenchymal stem cells alleviate liver fibrosis. Stem Cells Dev. (2012) 22:845–54. doi: 10.1089/scd.2012.0395
46. Teixeira FG, Carvalho MM, Neves-Carvalho A, Panchalingam KM, Behie LA, Pinto L, et al. Secretome of mesenchymal progenitors from the umbilical cord acts as modulator of neural/glial proliferation and differentiation. Stem Cell Rev. (2015) 11:288–97. doi: 10.1007/s12015-014-9576-2
47. Chen L, Xu Y, Zhao J, Zhang Z, Yang R, Xie J. Conditioned medium from hypoxic bone marrow-derived mesenchymal stem cells enhances wound healing in mice. PLoS ONE (2014) 9:e96161. doi: 10.1371/journal.pone.0096161
48. Ando Y, Matsubara K, Ishikawa J, Fujio M, Shohara R, Hibi H, et al. Stem cell-conditioned medium accelerates distraction osteogenesis through multiple regenerative mechanisms. Bone (2014) 61:82–90. doi: 10.1016/j.bone.2013.12.029
49. Parekkadan B, van Poll D, Suganuma K, Carter EA, Berthiaume F, Tilles AW, et al. Mesenchymal stem cell-derived molecules reverse fulminant hepatic failure. PLoS ONE (2007) 2:e941. doi: 10.1371/journal.pone.0000941
50. Imberti B, Morigi M, Tomasoni S, Rota C, Corna D, Longaretti L, et al. Insulin-like growth factor-1 sustains stem cell mediated renal repair. J Am Soc Nephrol. (2007) 18:2921–8. doi: 10.1681/ASN.2006121318
51. Iso Y, Spees JL, Serrano C, Bakondi B, Pochampally R, Song YH, et al. Multipotent human stromal cells improve cardiac function after myocardial infarction in mice without long-term engraftment. Biochem Biophys Res Commun. (2007) 354:700–6. doi: 10.1016/j.bbrc.2007.01.045
52. Javazon EH, Keswani SG, Badillo AT, Crombleholme TM, Zoltick PW, Radu AP, et al. Enhanced epithelial gap closure and increased angiogenesis in wounds of diabetic mice treated with adult murine bone marrow stromal progenitor cells Wound Repair Regen. (2007) 15:350–9. doi: 10.1111/j.1524-475X.2007.00237.x
53. Yang SH, Wu CC, Shih TT, Sun YH, Lin FH. In Vitro study on interaction between human nucleus pulposus cells and mesenchymal stem cells through paracrine stimulation. Spine (2008) 33:1951–7. doi: 10.1097/BRS.0b013e31817e6974
54. Brisby H, Papadimitriou N, Brantsing C, Bergh P, Lindahl A, Barreto Henriksson H. The presence of local mesenchymal progenitor cells in human degenerated intervertebral discs and possibilities to influence these in vitro: a descriptive study in humans. Stem Cells Dev. (2013) 22:804–14. doi: 10.1089/scd.2012.0179
55. Pereira CL, Teixeira GQ, Ribeiro-Machado C, Caldeira J, Costa M, Figueiredo F, et al. Mesenchymal stem/stromal cells seeded on cartilaginous endplates promote intervertebral disc regeneration through extracellular matrix remodeling. Sci Rep. (2016) 6:33836. doi: 10.1038/srep33836
56. Teixeira GQ, Pereira CL, Ferreira JR, Maia AF, Gomez-Lazaro M, Barbosa MA, et al. Immunomodulation of human mesenchymal stem/stromal cells in intervertebral disc degeneration: insights from a proinflammatory/degenerative ex vivo model. Spine (2017) 43:E673–82. doi: 10.1097/BRS.0000000000002494
57. Zheng ZM, Lu MM, Zhou L, Sun Y, Lv F, Leung VYL, et al. The potential of umbilical cord derived mesenchymal stem cells in intervertebral disc repair. Global Spine J. Germany, Georg Thieme Verlag (2014) 4:S81. doi: 10.1055/s-0034-1376649
58. Strassburg S, Hodson NW, Hill PI, Richardson SM, Hoyland JA. Bi-directional exchange of membrane components occurs during co-culture of mesenchymal stem cells and nucleus pulposus cells. PLoS ONE (2012) 7:e33739. doi: 10.1371/journal.pone.0033739
59. Dai W, Hale SL, Kloner RA. Role of a paracrine action of mesenchymal stem cells in the improvement of left ventricular function after coronary artery occlusion in rats. Regenet Med. (2007) 2:63–8. doi: 10.2217/17460751.2.1.63
60. Li Y, Chen J, Zhang CL, Wang L, Lu D, Katakowski M, et al. Gliosis and brain remodeling after treatment of stroke in rats with marrow stromal cells. Glia (2005) 49:407–17. doi: 10.1002/glia.20126
61. Salgado AJ, Reis RL, Sousa NJ, Gimble JM. Adipose tissue derived stem cells secretome: soluble factors and their roles in regenerative medicine. Curr Stem Cell Res Ther. (2010) 5:103–10. doi: 10.2174/157488810791268564
62. van Koppen A, Joles JA, van Balkom BWM, Lim SK, de Kleijn D, Giles RH, et al. Human embryonic mesenchymal stem cell-derived conditioned medium rescues kidney function in rats with established chronic kidney disease. PLoS ONE (2012) 7:e38746. doi: 10.1371/journal.pone.0038746
63. Silva AM, Teixeira JH, Almeida MI, Goncalves RM, Barbosa MA, Santos SG. Extracellular Vesicles: Immunomodulatory messengers in the context of tissue repair/regeneration. Eur J Pharm Sci. (2017) 98:86–95. doi: 10.1016/j.ejps.2016.09.017
64. Lai RC, Arslan F, Lee MM, Sze NS, Choo KA, Chen TS, et al. Exosome secreted by MSC reduces myocardial ischemia/reperfusion injury. Stem Cell Res. (2010) 4:214–22. doi: 10.1016/j.scr.2009.12.003
65. Doeppner TR, Herz J, Gorgens A, Schlechter J, Ludwig A, Radtke KS, et al. Extracellular vesicles improve post-stroke neuroregeneration and prevent postischemic immunosuppression. Stem Cells Transl Med. (2015) 4:1131–43. doi: 10.5966/sctm.2015-0078
66. Xin H, Li Y, Liu Z, Wang X, Shang X, Cui Y, et al. MiR-133b promotes neural plasticity and functional recovery after treatment of stroke with multipotent mesenchymal stromal cells in rats via transfer of exosome-enriched extracellular particles. Stem Cells (2013) 31:2737–46. doi: 10.1002/stem.1409
67. Ophelders DR, Wolfs TG, Jellema RK, Zwanenburg A, Andriessen P, Delhaas T, et al. Mesenchymal stromal cell-derived extracellular vesicles protect the fetal brain after hypoxia-ischemia. Stem Cells Transl Med. (2016) 5:754–63. doi: 10.5966/sctm.2015-0197
68. Gangadaran P, Rajendran RL, Lee HW, Kalimuthu S, Hong CM, Jeong SY, et al. Extracellular vesicles from mesenchymal stem cells activates VEGF receptors and accelerates recovery of hindlimb ischemia J Control Rel. (2017) 264:112–26. doi: 10.1016/j.jconrel.2017.08.022
69. Gatti S, Bruno S, Deregibus MC, Sordi A, Cantaluppi V, Tetta C, et al. Microvesicles derived from human adult mesenchymal stem cells protect against ischaemia–reperfusion-induced acute and chronic kidney injury. Nephrol Dial Trans. (2011) 26:1474–83. doi: 10.1093/ndt/gfr015
70. Bruno S, Grange C, Collino F, Deregibus MC, Cantaluppi V, Biancone L, et al. Microvesicles derived from mesenchymal stem cells enhance survival in a lethal model of acute kidney injury. PLoS ONE (2012) 7:e33115. doi: 10.1371/journal.pone.0033115
71. Zhou BR, Xu Y, Guo SL, Xu Y, Wang Y, Zhu F, et al. The effect of conditioned media of adipose-derived stem cells on wound healing after ablative fractional carbon dioxide laser resurfacing. Biomed Res Int. (2013) 2013:519126. doi: 10.1155/2013/519126
72. Kordelas L, Rebmann V, Ludwig AK, Radtke S, Ruesing J, Doeppner TR, et al. MSC-derived exosomes: a novel tool to treat therapy-refractory graft-versus-host disease Leukemia (2014) 28:970–3. doi: 10.1038/leu.2014.41
73. Fukuoka H, Suga H. Hair regeneration treatment using adipose-derived stem cell conditioned medium: follow-up with trichograms. Eplasty (2015) 15:e10.
74. Shin H, Ryu HH, Kwon O, Park BS, Jo SJ. Clinical use of conditioned media of adipose tissue-derived stem cells in female pattern hair loss: a retrospective case series study. Int J Dermatol. (2015) 54:730–5. doi: 10.1111/ijd.12650
75. Katagiri W, Osugi M, Kawai T, Hibi H. First-in-human study and clinical case reports of the alveolar bone regeneration with the secretome from human mesenchymal stem cells. Head Face Med. (2016) 12:5. doi: 10.1186/s13005-016-0101-5
76. Amos PJ, Kapur SK, Stapor PC, Shang H, Bekiranov S, Khurgel M, et al. Human adipose-derived stromal cells accelerate diabetic wound healing: impact of cell formulation and delivery. Tissue Eng Part A (2010) 16:1595–606. doi: 10.1089/ten.tea.2009.0616
77. Hemeda H, Jakob M, Ludwig AK, Giebel B, Lang S, Brandau S. Interferon-gamma and tumor necrosis factor-alpha differentially affect cytokine expression and migration properties of mesenchymal stem cells. Stem Cells Dev. (2010) 19:693–706. doi: 10.1089/scd.2009.0365
78. Hagmann S, Moradi B, Frank S, Dreher T, Kämmerer P, Richter WW, et al. Different culture media affect growth characteristics, surface marker distribution and chondrogenic differentiation of human bone marrow-derived mesenchymal stromal cells. BMC Musculoskeletal Disord. (2013) 14:223. doi: 10.1186/1471-2474-14-223
79. Lee KS, Cha SH, Kang HW, Song JY, Lee KW, Ko KB, et al. Effects of serial passage on the characteristics and chondrogenic differentiation of canine umbilical cord matrix derived mesenchymal stem cells asian-australasian J Anim Sci. (2013) 26:588–95. doi: 10.5713/ajas.2012.12488
80. Elahi KC, Klein G, Avci-Adali M, Sievert KD, MacNeil S, Aicher WK. Human mesenchymal stromal cells from different sources diverge in their expression of cell surface proteins and display distinct differentiation patterns. Stem Cells Int. (2016) 2016:5646384. doi: 10.1155/2016/5646384
81. Heathman TRJ, Rafiq QA, Chan AKC, Coopman K, Nienow AW, Kara B, et al. Characterization of human mesenchymal stem cells from multiple donors and the implications for large scale bioprocess development. Biochem Eng J. (2016) 108:14–23. doi: 10.1016/j.bej.2015.06.018
82. Hu X, Xu Y, Zhong Z, Wu Y, Zhao J, Wang Y, et al. A large-scale investigation of hypoxia-preconditioned allogeneic mesenchymal stem cells for myocardial repair in nonhuman primates: paracrine activity without remuscularization. Circ Res. (2016) 118:970–83. doi: 10.1161/CIRCRESAHA.115.307516
83. Bartosh TJ, Ylöstalo JH, Mohammadipoor A, Bazhanov N, Coble K, Claypool K, et al. Aggregation of human mesenchymal stromal cells (MSCs) into 3D spheroids enhances their antiinflammatory properties. Proc Natl Acad Sci USA. (2010) 107:13724–9. doi: 10.1073/pnas.1008117107
84. Suzuki S, Muneta T, Tsuji K, Ichinose S, Makino H, Umezawa A, et al. Properties and usefulness of aggregates of synovial mesenchymal stem cells as a source for cartilage regeneration. Arthritis Res Ther. (2012) 14:R136. doi: 10.1186/ar3869
85. Xu Y, Shi T, Xu A, Zhang L. 3D spheroid culture enhances survival and therapeutic capacities of MSCs injected into ischemic kidney. J Cell Mol Med. (2016) 20:203–13. doi: 10.1111/jcmm.12651
86. Cui X, Wang H, Guo H, Wang C, Ao H, Liu X, et al. Transplantation of mesenchymal stem cells preconditioned with diazoxide, a mitochondrial ATP-sensitive potassium channel opener, promotes repair of myocardial infarction in rats. Tohoku J Exp Med. (2010) 220:139–47. doi: 10.1620/tjem.220.139
87. Pessina A, Cocce V, Pascucci L, Bonomi A, Cavicchini L, Sisto F, et al. Mesenchymal stromal cells primed with paclitaxel attract and kill leukaemia cells, inhibit angiogenesis and improve survival of leukaemia-bearing mice. Br J Haematol. (2013) 160:766–78. doi: 10.1111/bjh.12196
88. Kang H, Kim KH, Lim J, Kim YS, Heo J, Choi J, et al. The therapeutic effects of human mesenchymal stem cells primed with sphingosine-1 phosphate on pulmonary artery hypertension. Stem Cells Dev. (2015) 24:1658–71. doi: 10.1089/scd.2014.0496
89. Pasha Z, Wang Y, Sheikh R, Zhang D, Zhao T, Ashraf M. Preconditioning enhances cell survival and differentiation of stem cells during transplantation in infarcted myocardium. Cardiovasc Res. (2008) 77:134–42. doi: 10.1093/cvr/cvm025
90. Herrmann JL, Wang Y, Abarbanell AM, Weil BR, Tan J, Meldrum DR. Preconditioning mesenchymal stem cells with transforming growth factor-alpha improves mesenchymal stem cell-mediated cardioprotection. Shock (2010) 33:24–30. doi: 10.1097/SHK.0b013e3181b7d137
91. Uemura R, Xu M, Ahmad N, Ashraf M. Bone marrow stem cells prevent left ventricular remodeling of ischemic heart through paracrine signaling. Circ Res. (2006) 98:1414–21. doi: 10.1161/01.RES.0000225952.61196.39
92. Leroux L, Descamps B, Tojais NF, Seguy B, Oses P, Moreau C, et al. Hypoxia preconditioned mesenchymal stem cells improve vascular and skeletal muscle fiber regeneration after ischemia through a wnt4-dependent pathway. Mol Ther. (2010) 18:1545–52. doi: 10.1038/mt.2010.108
93. Wei L, Fraser JL, Lu ZY, Hu X, Yu SP. Transplantation of hypoxia preconditioned bone marrow mesenchymal stem cells enhances angiogenesis and neurogenesis after cerebral ischemia in rats. Neurobiol Dis. (2012) 46:635–45. doi: 10.1016/j.nbd.2012.03.002
94. Kong P, Xie X, Li F, Liu Y, Lu Y. Placenta mesenchymal stem cell accelerates wound healing by enhancing angiogenesis in diabetic Goto-Kakizaki (GK) rats. Biochem Biophys Res Commun. (2013) 438:410–9. doi: 10.1016/j.bbrc.2013.07.088
95. Yu J, Yin S, Zhang W, Gao F, Liu Y, Chen Z. Hypoxia preconditioned bone marrow mesenchymal stem cells promote liver regeneration in a rat massive hepatectomy model. Stem Cell Res Ther. (2013) 4:83. doi: 10.1186/scrt234
96. Zhang W, Liu L, Huo Y, Yang Y, Wang Y. Hypoxia-pretreated human MSCs attenuate acute kidney injury through enhanced angiogenic and antioxidative capacities. Biomed Res Int. (2014) 2014:462472. doi: 10.1155/2014/462472
97. Jiang CM, Liu J, Zhao JY, Xiao L, An S, Gou YC, et al. Effects of hypoxia on the immunomodulatory properties of human gingiva-derived mesenchymal stem cells J Dent Res. (2015) 94:69–77. doi: 10.1177/0022034514557671
98. Lan YW, Choo KB, Chen CM, Hung TH, Chen YB, Hsieh CH, et al. Hypoxia-preconditioned mesenchymal stem cells attenuate bleomycin-induced pulmonary fibrosis. Stem Cell Res Ther. (2015) 6:97. doi: 10.1186/s13287-015-0081-6
99. Mias C, Trouche E, Seguelas MH, Calcagno F, Dignat-George F, Sabatier F, et al. Ex vivo pretreatment with melatonin improves survival, proangiogenic/mitogenic activity, and efficiency of mesenchymal stem cells injected into ischemic kidney. Stem Cells (2008) 26:1749–57. doi: 10.1634/stemcells.2007-1000
100. Tang Y, Cai B, Yuan F, He X, Lin X, Wang J, et al. Melatonin pretreatment improves the survival and function of transplanted mesenchymal stem cells after focal cerebral ischemia. Cell Trans. (2014) 23:1279–91. doi: 10.3727/096368913X667510
101. Liu C, Fan Y, Zhou L, Zhu HY, Song YC, Hu L, et al. Pretreatment of mesenchymal stem cells with angiotensin II enhances paracrine effects, angiogenesis, gap junction formation and therapeutic efficacy for myocardial infarction. Int J Cardiol. (2015) 188:22–32. doi: 10.1016/j.ijcard.2015.03.425
102. Wang CC, Chen CH, Hwang SM, Lin WW, Huang CH, Lee WY, et al. Spherically symmetric mesenchymal stromal cell bodies inherent with endogenous extracellular matrices for cellular cardiomyoplasty. Stem Cells (2009) 27:724–32. doi: 10.1634/stemcells.2008-0944
103. Suenaga H, Furukawa KS, Suzuki Y, Takato T, Ushida T. Bone regeneration in calvarial defects in a rat model by implantation of human bone marrow-derived mesenchymal stromal cell spheroids. J Mater Sci Mater Med. (2015) 26:254. doi: 10.1007/s10856-015-5591-3
104. Zhang X, Hu M-G, Pan K, Li C-H, Liu R. 3D spheroid culture enhances the expression of antifibrotic factors in human adipose-derived mscs and improves their therapeutic effects on hepatic fibrosis. Stem Cells Int. (2016) 2016:4626073. doi: 10.1155/2016/4626073
105. Yao Y, Zhang F, Wang L, Zhang G, Wang Z, Chen J, et al. Lipopolysaccharide preconditioning enhances the efficacy of mesenchymal stem cells transplantation in a rat model of acute myocardial infarction. J Biomed Sci. (2009) 16:74. doi: 10.1186/1423-0127-16-74
106. Tsai LK, Wang Z, Munasinghe J, Leng Y, Leeds P, Chuang DM. Mesenchymal stem cells primed with valproate and lithium robustly migrate to infarcted regions and facilitate recovery in a stroke model. Stroke (2011) 42:2932–9. doi: 10.1161/STROKEAHA.110.612788
107. Li N, Yang YJ, Qian HY, Li Q, Zhang Q, Li XD, et al. Intravenous administration of atorvastatin-pretreated mesenchymal stem cells improves cardiac performance after acute myocardial infarction: role of CXCR(2013) 4. Am J Trans Res. (2015) 7:1058–70.
108. Ejtehadifar M, Shamsasenjan K, Movassaghpour A, Akbarzadehlaleh P, Dehdilani N, Abbasi P, et al. The effect of hypoxia on mesenchymal stem cell biology. Adv Pharmaceut Bull. (2015) 5:141–9. doi: 10.15171/apb.2015.021
109. Das R, Jahr H, van Osch GJ, Farrell E. The role of hypoxia in bone marrow-derived mesenchymal stem cells: considerations for regenerative medicine approaches. Tissue Eng Part B Rev. (2010) 16:159–68. doi: 10.1089/ten.teb.2009.0296
110. Hawkins KE, Sharp TV, McKay TR. The role of hypoxia in stem cell potency and differentiation. Regenet Med. (2013) 8:771–82. doi: 10.2217/rme.13.71
111. Berniakovich I, Giorgio M. Low oxygen tension maintains multipotency, whereas normoxia increases differentiation of mouse bone marrow stromal cells. Int J Mol Sci. (2013) 14:2119–34. doi: 10.3390/ijms14012119
112. Carrero R, Cerrada I, Lledo E, Dopazo J, Garcia-Garcia F, Rubio MP, et al. IL1beta induces mesenchymal stem cells migration and leucocyte chemotaxis through NF-kappaB. Stem Cell Rev. (2012) 8:905–16. doi: 10.1007/s12015-012-9364-9
113. Gnecchi M, He H, Noiseux N, Liang OD, Zhang L, Morello F, et al. Evidence supporting paracrine hypothesis for Akt-modified mesenchymal stem cell-mediated cardiac protection and functional improvement. FASEB J. (2006) 20:661–9. doi: 10.1096/fj.05-5211com
114. Xie L, Mao M, Zhou L, Zhang L, Jiang B. Signal factors secreted by 2D and spheroid mesenchymal stem cells and by cocultures of mesenchymal stem cells derived microvesicles and retinal photoreceptor neurons. Stem Cells Int. (2017) 2017:2730472. doi: 10.1155/2017/2730472
115. Lee MJ, Kim J, Kim MY, Bae YS, Ryu SH, Lee TG, et al. Proteomic analysis of tumor necrosis factor-alpha-induced secretome of human adipose tissue-derived mesenchymal stem cells. J Proteome Res. (2010) 9:1754–62. doi: 10.1021/pr900898n
116. Heo SC, Jeon ES, Lee IH, Kim HS, Kim MB, Kim JH. Tumor necrosis factor-alpha-activated human adipose tissue-derived mesenchymal stem cells accelerate cutaneous wound healing through paracrine mechanisms. J Invest Dermatol. (2011) 131:1559–67. doi: 10.1038/jid.2011.64
117. Jin P, Zhao Y, Liu H, Chen J, Ren J, Jin J, et al. Interferon-γ and tumor necrosis factor-α polarize bone marrow stromal cells uniformly to a Th1 phenotype. Sci Rep. (2016) 6:26345. doi: 10.1038/srep26345
118. Liu H, Xue W, Ge G, Luo X, Li Y, Xiang H, et al. Hypoxic preconditioning advances CXCR4 and CXCR7 expression by activating HIF-1α in MSCs. Biochem Biophys Res Commun. (2010) 401:509–15. doi: 10.1016/j.bbrc.2010.09.076
119. Fan H, Zhao G, Liu L, Liu F, Gong W, Liu X, et al. Pre-treatment with IL-1β enhances the efficacy of MSC transplantation in DSS-induced colitis. Cell Mol Immunol. (2012) 9:473–81. doi: 10.1038/cmi.2012.40
120. Kim YS, Noh MY, Cho KA, Kim H, Kwon MS, Kim KS, et al. Hypoxia/reoxygenation-preconditioned human bone marrow-derived mesenchymal stromal cells rescue ischemic rat cortical neurons by enhancing trophic factor release Mol Neurobiol. (2015) 52:792–803. doi: 10.1007/s12035-014-8912-5
121. Jun EK, Zhang Q, Yoon BS, Moon J, Lee G, Park G. Hypoxic conditioned medium from human amniotic fluid-derived mesenchymal stem cells accelerates skin wound healing through TGF-β/SMAD2 and PI3K/Akt pathways. Int J Mol Sci. (2014) 15:605–28. doi: 10.3390/ijms15010605
122. Francois M, Romieu-Mourez R, Li M, Galipeau J. Human MSC suppression correlates with cytokine induction of indoleamine 2,3-dioxygenase and bystander M2 macrophage differentiation. Mol Ther. (2012) 20:187–95. doi: 10.1038/mt.2011.189
123. Liang C, Chen SL, Wang M, Zhai WJ, Zhou Z, Pang AM, et al. Synergistic immunomodulatory effects of interferon-gamma and bone marrow mesenchymal stem cell]. Zhonghua xueyexue zazhi. (2013) 34:213–6. doi: 10.3760/cma.j.issn.0253-2727.2013.03.007
124. Noone C, Kihm A, English K, O'Dea S, Mahon BP. IFN-γ stimulated human umbilical-tissue derived cells potently suppress NK activation and resist NK mediated cytotoxicity in vitro. Stem Cells Dev. (2013) 15, 3003–3014. doi: 10.1089/scd.2013.0028
125. Tu Z, Li Q, Bu H, Lin F. Mesenchymal stem cells inhibit complement activation by secreting factor H. Stem Cells Dev. (2010) 19:1803–9. doi: 10.1089/scd.2009.0418
126. Du L, Lv R, Yang X, Cheng S, Ma T, Xu J. Hypoxic conditioned medium of placenta-derived mesenchymal stem cells protects against scar formation. Life Sci. (2016) 149:51–7. doi: 10.1016/j.lfs.2016.02.050
127. Lotfinia M, Lak S, Mohammadi Ghahhari N, Johari B, Maghsood F, Parsania S, et al. Hypoxia pre-conditioned embryonic mesenchymal stem cell secretome reduces il-10 production by peripheral blood mononuclear cells. Iran Biomed J. (2016) 21:24–31. doi: 10.6091/.21.1.24
128. Ivanova-Todorova E, Bochev I, Dimitrov R, Belemezova K, Mourdjeva M, Kyurkchiev S, et al. Conditioned medium from adipose tissue-derived mesenchymal stem cells induces CD4+FOXP3+ cells and increases IL-10 secretion. J Biomed Biotechnol. (2012) 2012:295167. doi: 10.1155/2012/295167
129. Potapova IA, Gaudette GR, Brink PR, Robinson RB, Rosen MR, Cohen IS, et al. Mesenchymal stem cells support migration, extracellular matrix invasion, proliferation, and survival of endothelial cells in vitro Stem Cells (2007) 25:1761–8. doi: 10.1634/stemcells.2007-0022
130. Overath JM, Gauer S, Obermuller N, Schubert R, Schafer R, Geiger H, et al. Short-term preconditioning enhances the therapeutic potential of adipose-derived stromal/stem cell-conditioned medium in cisplatin-induced acute kidney injury. Exp Cell Res. (2016) 342:175–83. doi: 10.1016/j.yexcr.2016.03.002
131. Ma T, Grayson WL, Frohlich M, Vunjak-Novakovic G. Hypoxia and stem cell-based engineering of mesenchymal tissues. Biotechnol Prog. (2009) 25:32–42. doi: 10.1002/btpr.128
132. Kiani AA, Kazemi A, Halabian R, Mohammadipour M, Jahanian-Najafabadi A, Roudkenar MH. HIF-1α confers resistance to induced stress in bone marrow-derived mesenchymal stem cells. Arch Med Res. (2013) 44:185–93. doi: 10.1016/j.arcmed.2013.03.006
133. Deschepper M, Oudina K, David B, Myrtil V, Collet C, Bensidhoum M, et al. Survival and function of mesenchymal stem cells (MSCs) depend on glucose to overcome exposure to long-term, severe and continuous hypoxia. J Cellu Mol Med. (2011) 15:1505–14. doi: 10.1111/j.1582-4934.2010.01138.x
134. Cicione C, Muinos-Lopez E, Hermida-Gomez T, Fuentes-Boquete I, Diaz-Prado S, Blanco FJ. Effects of severe hypoxia on bone marrow mesenchymal stem cells differentiation potential. Stem Cells Int. (2013) 2013:232896. doi: 10.1155/2013/232896
135. Gnecchi M, He H, Liang OD, Melo LG, Morello F, Mu H, et al. Paracrine action accounts for marked protection of ischemic heart by Akt-modified mesenchymal stem cells Nat Med. (2005) 11:367–8. doi: 10.1038/nm0405-367
136. Imtiyaz HZ, Simon MC. Hypoxia-inducible factors as essential regulators of inflammation. Curr Top Microbiol Immunol. (2010) 345:105–20. doi: 10.1007/82_2010_74
137. Ahluwalia A, Tarnawski AS. Critical role of hypoxia sensor - HIF-1α in VEGF gene activation. Implications for angiogenesis and tissue injury healing. Curr Med Chem. (2005) 19:90–7. doi: 10.2174/092986712803413944
138. Bader AM, Klose K, Bieback K, Korinth D, Schneider M, Seifert M, et al. Hypoxic preconditioning increases survival and pro-angiogenic capacity of human cord blood mesenchymal stromal cells in vitro. PLoS ONE (2015) 10:e0138477. doi: 10.1371/journal.pone.0138477
139. Liu J, Hao H, Xia L, Ti D, Huang H, Dong L, et al. Hypoxia pretreatment of bone marrow mesenchymal stem cells facilitates angiogenesis by improving the function of endothelial cells in diabetic rats with lower ischemia. PLoS ONE (2015) 10:e0126715. doi: 10.1371/journal.pone.0126715
140. Bian S, Zhang L, Duan L, Wang X, Min Y, Yu H. Extracellular vesicles derived from human bone marrow mesenchymal stem cells promote angiogenesis in a rat myocardial infarction model. J Mol Med. (2014) 92:387–97. doi: 10.1007/s00109-013-1110-5
141. Anderson JD, Johansson HJ, Graham CS, Vesterlund M, Pham MT, Bramlett CS, et al. Comprehensive proteomic analysis of mesenchymal stem cell exosomes reveals modulation of angiogenesis via nuclear factor-kappab signaling. Stem Cells (2016) 34:601–13. doi: 10.1002/stem.2298
142. Park H, Park H, Mun D, Kang J, Kim H, Kim M, et al. Extracellular vesicles derived from hypoxic human mesenchymal stem cells attenuate GSK3beta expression via mirna-26a in an ischemia-reperfusion injury model. Yonsei Med J. (2018) 59:736–45. doi: 10.3349/ymj.2018.59.6.736
143. Han YD, Bai Y, Yan XL, Ren J, Zeng Q, Li XD, et al. Co-transplantation of exosomes derived from hypoxia-preconditioned adipose mesenchymal stem cells promotes neovascularization and graft survival in fat grafting Biochem Biophys Res Commun. (2018) 497:305–12. doi: 10.1016/j.bbrc.2018.02.076
144. Feng Y, Huang W, Wani M, Yu X, Ashraf M. Ischemic preconditioning potentiates the protective effect of stem cells through secretion of exosomes by targeting Mecp2 via miR-22. PLoS ONE (2014) 9:e88685. doi: 10.1371/journal.pone.0088685
145. Kilpinen L, Impola U, Sankkila L, Ritamo I, Aatonen M, Kilpinen S, et al. Extracellular membrane vesicles from umbilical cord blood-derived MSC protect against ischemic acute kidney injury, a feature that is lost after inflammatory conditioning. J Extracell Vesicles (2013) 2, 1–15. doi: 10.3402/jev.v2i0.21927
146. Yang J, Gao F, Zhang Y, Liu Y, Zhang D. Buyang huanwu decoction (BYHWD) enhances angiogenic effect of mesenchymal stem cell by upregulating vegf expression after focal cerebral ischemia. J Mol Neurosci. (2015) 56:898–906. doi: 10.1007/s12031-015-0539-0
147. Crisostomo PR, Wang Y, Markel TA, Wang M, Lahm T, Meldrum DR. Human mesenchymal stem cells stimulated by TNF-α, LPS, or hypoxia produce growth factors by an NFκB- but not JNK-dependent mechanism. Am J Physiol Cell Physiol. (2008) 294:C675–82. doi: 10.1152/ajpcell.00437.2007
148. Beegle J, Lakatos K, Kalomoiris S, Stewart H, Isseroff R, Nolta RJA, et al. Hypoxic preconditioning of mesenchymal stromal cells induces metabolic changes, enhances survival, and promotes cell retention in vivo. Stem Cells (2015) 33:1818–28. doi: 10.1002/stem.1976
149. Saparov A, Ogay V, Nurgozhin T, Jumabay M, Chen WCW. Preconditioning of human mesenchymal stem cells to enhance their regulation of the immune response. Stem Cells Int. (2016) 2016:3924858. doi: 10.1155/2016/3924858
150. Wang Y, Chen X, Cao W, Shi Y. Plasticity of mesenchymal stem cells in immunomodulation: pathological and therapeutic implications. Nat Immunol. (2014) 15:1009–16. doi: 10.1038/ni.3002
151. Park CW, Kim KS, Bae S, Son HK, Myung PK, Hong HJ, et al. Cytokine secretion profiling of human mesenchymal stem cells by antibody array international J Stem Cells (2009) 2:59–68.
152. Hsu WT, Lin CH, Chiang BL, Jui HY, Wu KK, Lee CM. Prostaglandin E2 potentiates mesenchymal stem cell-induced IL-10+IFN-γ+CD4+ regulatory T cells to control transplant arteriosclerosis. J Immunol. (2013) 190:2372–80. doi: 10.4049/jimmunol.1202996
153. Németh K, Leelahavanichkul A, Yuen PST, Mayer B, Parmelee A, Doi K, et al. Bone marrow stromal cells attenuate sepsis via prostaglandin E(2)-dependent reprogramming of host macrophages to increase their interleukin-10 production. Nat Med. (2009) 15:42–9. doi: 10.1038/nm.1905
154. Chen K, Wang D, Du WT, Han ZB, Ren H, Chi Y, et al. Human umbilical cord mesenchymal stem cells hUC-MSCs exert immunosuppressive activities through a PGE2-dependent mechanism. Clin Immunol. (2010) 135:448–58. doi: 10.1016/j.clim.2010.01.015
155. Maffioli E, Nonnis S, Angioni R, Santagata F, Cali B, Zanotti L, et al. Proteomic analysis of the secretome of human bone marrow-derived mesenchymal stem cells primed by pro-inflammatory cytokines. J Proteomics (2017) 166:115–26. doi: 10.1016/j.jprot.2017.07.012
156. Augello A, Tasso R, Negrini SM, Amateis A, Indiveri F, Cancedda R, et al. Bone marrow mesenchymal progenitor cells inhibit lymphocyte proliferation by activation of the programmed death 1 pathway. Eur J Immunol. (2005) 35:1482–90. doi: 10.1002/eji.200425405
157. Ren G, Zhang L, Zhao X, Xu G, Zhang Y, Roberts AI, et al. Mesenchymal stem cell-mediated immunosuppression occurs via concerted action of chemokines and nitric oxide. Cell Stem Cell (2008) 2:141–50. doi: 10.1016/j.stem.2007.11.014
158. Akiyama K, Chen C, Wang D, Xu X, Qu C, Yamaza T, et al. Mesenchymal-stem-cell-induced immunoregulation involves FAS-ligand-/FAS-mediated T cell apoptosis. Cell Stem Cell (2012) 10:544–55. doi: 10.1016/j.stem.2012.03.007
159. Di Nicola M, Carlo-Stella C, Magni M, Milanesi M, Longoni P, Matteucci DP, et al. Human bone marrow stromal cells suppress T-lymphocyte proliferation induced by cellular or nonspecific mitogenic stimuli. Blood (2002) 99:3838–43. doi: 10.1182/blood.V99.10.3838
160. Lee RH, Pulin AA, Seo MJ, Kota DJ, Ylostalo J, Larson BL, et al. Intravenous hMSCs improve myocardial infarction in mice because cells embolized in lung are activated to secrete the anti-inflammatory protein TSG-6. Cell Stem Cell. (2009) 5:54–63. doi: 10.1016/j.stem.2009.05.003
161. Roddy GW, Oh JY, Lee RH, Bartosh TJ, Ylostalo J, Coble K, et al. Action at a distance: systemically administered adult stem/progenitor cells (MSCs) reduce inflammatory damage to the cornea without engraftment and primarily by secretion of TNF-α stimulated gene/protein 6. Stem Cells (2011) 29:1572–9. doi: 10.1002/stem.708
162. Ryan JM, Barry F, Murphy JM, Mahon BP. Interferon-γ does not break, but promotes the immunosuppressive capacity of adult human mesenchymal stem cells. Clin Exp Immunol. (2007) 149:353–63. doi: 10.1111/j.1365-2249.2007.03422.x
163. Polchert D, Sobinsky J, Douglas GW, Kidd M, Moadsiri A, Reina E, et al. IFN-γ activation of mesenchymal stem cells for treatment and prevention of graft versus host disease Eur J Immunol. (2008) 38:1745–55.
164. English K. Mechanisms of mesenchymal stromal cell immunomodulation. Immunol Cell Biol. (2013) 91:19–26. doi: 10.1038/icb.2012.56
165. Kim DS, Jang IK, Lee MW, Ko YJ, Lee D-H, Lee JW, et al. Enhanced immunosuppressive properties of human mesenchymal stem cells primed by interferon-γ. EBio Med. (2018) 28:261–73. doi: 10.1016/j.ebiom.2018.01.002
166. Krampera M, Cosmi L, Angeli R, Pasini A, Liotta F, Andreini A, et al. Role for interferon-gamma in the immunomodulatory activity of human bone marrow mesenchymal stem cells. Stem Cells (2006) 24:386–98. doi: 10.1634/stemcells.2005-0008
167. Ren G, Su J, Zhang L, Zhao X, Ling W, L'Huillie A, et al. Species variation in the mechanisms of mesenchymal stem cell-mediated immunosuppression. Stem Cells (2009) 27:1954–62. doi: 10.1002/stem.118
168. Lu Z, Chen Y, Dunstan C, Roohani-Esfahani S, Zreiqat H. Priming adipose stem cells with tumor necrosis factor-α preconditioning potentiates their exosome efficacy for bone regeneration. Tissue Eng Part A (2017) 23:1212–20. doi: 10.1089/ten.tea.2016.0548
169. Cosenza S, Toupet K, Maumus M, Luz-Crawford P, Blanc-Brude O, Jorgensen C, et al. Mesenchymal stem cells-derived exosomes are more immunosuppressive than microparticles in inflammatory arthritis. Theranostics (2018) 8:1399–410. doi: 10.7150/thno.21072
170. Opitz CA, Litzenburger UM, Lutz C, Lanz TV, Tritschler I, Koppel A, et al. Toll-like receptor engagement enhances the immunosuppressive properties of human bone marrow-derived mesenchymal stem cells by inducing indoleamine-2,3-dioxygenase-1 via interferon-beta and protein kinase R. Stem Cells (2009) 27:909–19. doi: 10.1002/stem.7
171. Sioud M, Mobergslien A, Boudabous A, Floisand Y. Evidence for the involvement of galectin-3 in mesenchymal stem cell suppression of allogeneic T-cell proliferation. Scand J Immunol. (2010) 71:267–74. doi: 10.1111/j.1365-3083.2010.02378.x
172. Liotta F, Angeli R, Cosmi L, Fili L, Manuelli C, Frosali F, et al. Toll-like receptors 3 and 4 are expressed by human bone marrow-derived mesenchymal stem cells and can inhibit their T-cell modulatory activity by impairing Notch signaling. Stem Cells (2008) 26:279–89. doi: 10.1634/stemcells.2007-0454
173. Hahn JY, Cho HJ, Kang HJ, Kim TS, Kim MH, Chung JH, et al. Pre-treatment of mesenchymal stem cells with a combination of growth factors enhances gap junction formation, cytoprotective effect on cardiomyocytes, and therapeutic efficacy for myocardial infarction. J Am Coll Cardiol. (2008) 51:933–43. doi: 10.1016/j.jacc.2007.11.040
174. Bai Y, Han YD, Yan XL, Ren J, Zeng Q, Li XD, et al. Adipose mesenchymal stem cell-derived exosomes stimulated by hydrogen peroxide enhanced skin flap recovery in ischemia-reperfusion injury. Biochem Biophys Res Commun. (2018) 500:310–7. doi: 10.1016/j.bbrc.2018.04.065
175. Potapova IA, Brink PR, Cohen IS, Doronin SV. Culturing of human mesenchymal stem cells as three-dimensional aggregates induces functional expression of CXCR4 that regulates adhesion to endothelial cells. J Biol Chem. (2008) 283:13100–7. doi: 10.1074/jbc.M800184200
176. Petrenko Y, Syková E, Kubinová Š. The therapeutic potential of three-dimensional multipotent mesenchymal stromal cell spheroids. Stem Cell Res Ther. (2017) 8:94. doi: 10.1186/s13287-017-0558-6
177. Cesarz Z, Tamama K. Spheroid culture of mesenchymal stem cells. Stem Cells Int. (2016) 2016:9176357. doi: 10.1155/2016/9176357
178. Lee EJ, Park SJ, Kang SK, Kim GH, Kang HJ, Lee SW, et al. Spherical bullet formation via E-cadherin promotes therapeutic potency of mesenchymal stem cells derived from human umbilical cord blood for myocardial infarction Mol Ther. (2012) 20:1424–33. doi: 10.1038/mt.2012.58
179. Kim YS, Ahn Y, Kwon JS, Cho YK, Jeong MH, Cho JG, et al. Priming of mesenchymal stem cells with oxytocin enhances the cardiac repair in ischemia/reperfusion injury. Cells Tissues Organs (2012) 195:428–42. doi: 10.1159/000329234
180. Liu J, Zhu P, Song P, Xiong W, Chen H, Peng W, et al. Pretreatment of adipose derived stem cells with curcumin facilitates myocardial recovery via antiapoptosis and angiogenesis. Stem Cells Int. (2015) 2015:638153. doi: 10.1155/2015/638153
181. Phan J, Kumar P, Hao D, Gao K, Farmer D, Wang A. Engineering mesenchymal stem cells to improve their exosome efficacy and yield for cell-free therapy. J Extracell Vesicles (2018) 7:1522236. doi: 10.1080/20013078.2018.1522236
182. Phelps J, Sanati-Nezhad A, Ungrin M, Duncan NA, Sen A. bioprocessing of mesenchymal stem cells and their derivatives: toward cell-free therapeutics. Stem Cells Int. (2018) 2018:9415367. doi: 10.1155/2018/9415367
183. Tolar J, Le Blanc K, Keating A, Blazar BR. Hitting the right spot with mesenchymal stromal cells (MSCs). Stem Cells (2010) 28:1446–55. doi: 10.1002/stem.459
Keywords: MSCs (Mesenchymal Stromal Cells), pre-conditioning, regeneration, immunomodulation, therapeutic potential, secretome
Citation: Ferreira JR, Teixeira GQ, Santos SG, Barbosa MA, Almeida-Porada G and Gonçalves RM (2018) Mesenchymal Stromal Cell Secretome: Influencing Therapeutic Potential by Cellular Pre-conditioning. Front. Immunol. 9:2837. doi: 10.3389/fimmu.2018.02837
Received: 19 September 2018; Accepted: 16 November 2018;
Published: 04 December 2018.
Edited by:
Guido Moll, Charité Universitätsmedizin Berlin, GermanyReviewed by:
Federica Casiraghi, Istituto Di Ricerche Farmacologiche Mario Negri, ItalyCopyright © 2018 Ferreira, Teixeira, Santos, Barbosa, Almeida-Porada and Gonçalves. This is an open-access article distributed under the terms of the Creative Commons Attribution License (CC BY). The use, distribution or reproduction in other forums is permitted, provided the original author(s) and the copyright owner(s) are credited and that the original publication in this journal is cited, in accordance with accepted academic practice. No use, distribution or reproduction is permitted which does not comply with these terms.
*Correspondence: Raquel M. Gonçalves, cmFxdWVsZ0BpbmViLnVwLnB0
†Present Address: Graciosa Q. Teixeira, Institute of Orthopaedic Research and Biomechanics, University of Ulm, Ulm, Germany
Disclaimer: All claims expressed in this article are solely those of the authors and do not necessarily represent those of their affiliated organizations, or those of the publisher, the editors and the reviewers. Any product that may be evaluated in this article or claim that may be made by its manufacturer is not guaranteed or endorsed by the publisher.
Research integrity at Frontiers
Learn more about the work of our research integrity team to safeguard the quality of each article we publish.