- 1MalarVx, Inc., Seattle, WA, United States
- 2Seattle Children's Research Institute, Seattle, WA, United States
Each year malaria kills hundreds of thousands of people and infects hundreds of millions of people despite current control measures. An effective malaria vaccine will likely be necessary to aid in malaria eradication. Vaccination using whole sporozoites provides an increased repertoire of immunogens compared to subunit vaccines across at least two life cycle stages of the parasite, the extracellular sporozoite, and intracellular liver stage. Three potential whole sporozoite vaccine approaches are under development and include genetically attenuated parasites, radiation attenuated sporozoites, and wild-type sporozoites administered in combination with chemoprophylaxis. Pre-clinical and clinical studies have demonstrated whole sporozoite vaccine immunogenicity, including humoral and cellular immunity and a range of vaccine efficacy that depends on the pre-exposure of vaccinated individuals. While whole sporozoite vaccines can provide protection against malaria in some cases, more recent studies in malaria-endemic regions demonstrate the need for improvements. Moreover, challenges remain in manufacturing large quantities of sporozoites for vaccine commercialization. A promising solution to the whole sporozoite manufacturing challenge is in vitro culturing methodology, which has been described for several Plasmodium species, including the major disease-causing human malaria parasite, Plasmodium falciparum. Here, we review whole sporozoite vaccine immunogenicity and in vitro culturing platforms for sporozoite production.
Introduction
According to the 2016 World Malaria Report by the World Health Organization (WHO), nearly half of the world's population live in areas at risk of malaria transmission (1). The people most impacted by malaria are children under the age of five and pregnant women living in sub-Saharan Africa. In 2016, Plasmodium falciparum (Pf) caused the majority of the 445 thousand deaths and more than 200 million clinical cases, despite recent progress in malaria control efforts, which include vector control with insecticidal bed-nets and insecticide spray and chemoprevention with anti-malarial drugs (1). While these efforts have reduced morbidity and mortality caused by the disease, their impact has declined since the number of deaths and clinical cases have remained constant in the recent years highlighting the need for an effective vaccine. Vaccines represent the most effective form of control for certain infectious disease as demonstrated by the eradication of smallpox and near eradication of polio. However, vaccine development against Plasmodium has proved more challenging than vaccine development against simpler bacteria or viruses. Plasmodia are complex eukaryotic parasites with large genomes (5,300 genes) and transform through multiple life cycle stages with stage-specific gene (antigen) expression (2). Thus, malaria vaccine development has been more challenging. For example, RTS, S/AS-01 (RTS, S) the most developed malaria vaccine candidate, which elicits antibodies against the major sporozoite (SPZ) surface protein, circumsporozoite protein (CSP), only provides partial efficacy (3, 4).
Whole Sporozoite Malaria Vaccines in Development
Whole sporozoite vaccines (WSV) have demonstrated a range of vaccine efficacy that depends on prior exposure to malaria and ranges from ~35 to 100% efficacy, in which malaria naïve volunteers are better protected. WSV in development include radiation attenuated sporozoites (RAS), genetically attenuated parasites (GAP), and WT sporozoites administered under drug cover (known as CPS–chemical prophylaxis with SPZ, ITV–infection treatment vaccination, or CVac–using Sanaria's injectable SPZ; hereafter referred to as CPS) (Figure 1). These live SPZ infect hepatocytes but do not lead to an established blood stage infection either by arresting in liver stage development (RAS and GAP) or by being eliminated during the initial stages of red blood cell (RBC) infection (CPS). WSV elicit immunity during two stages of the parasite life cycle, extracellular SPZ and intracellular liver stage for GAP and RAS and up to the initial stages of RBC infection for CPS. Thus, WSV can elicit immunity without causing clinical malaria symptoms. RAS delivered by bites of irradiated mosquitoes were first tested in humans in 1973 and protected humans from Pf malaria challenge (5). Since then, Hoffman and colleagues have made significant progress to produce vialed SPZ from aseptic mosquitoes for clinical testing of RAS (PfSPZ Vaccine) and CVac [Reviewed in (6)]. A recent PfSPZ study involving malaria-experienced individuals in Mali, in which 66% of the Malian vaccine group developed malaria infection, demonstrated reduced efficacy compared to trials involving malaria-naïve individuals (7). Thus, the PfSPZ vaccine failed to provide adequate protection in pre-exposed individuals. This study highlights the need for an improved dosing strategy, immunogenicity enhancement, and/or alternative vaccine approach for populations in malarious regions.
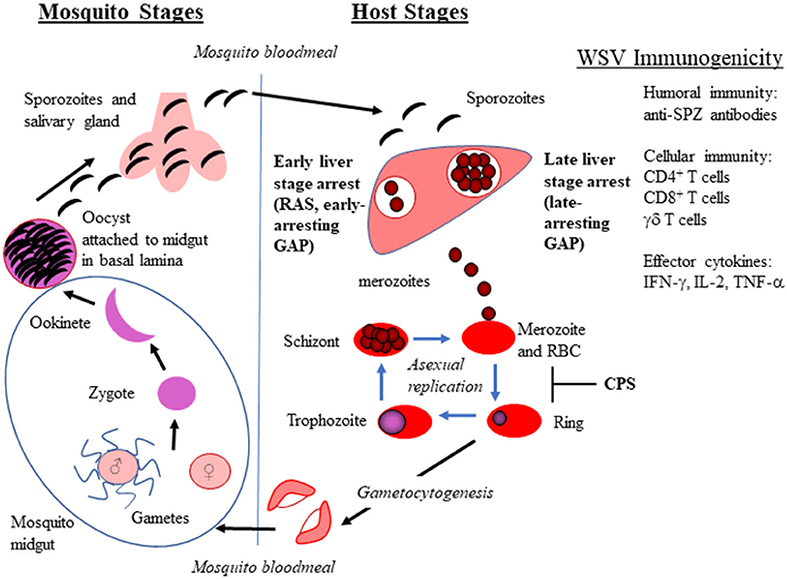
Figure 1. Plasmodium life cycle in mosquitoes and vertebrate hosts. The whole sporozite vaccine (WSV) strategy for radiation attenuated sporozoites (RAS), genetically attenuated parasites (GAP), and chemoprophylaxis with WT sporozoites (CPS) is shown in bold. The WSV immunogenicity provided by WSV administration is also listed.
Alternative approaches include other WSV types described here and immunogenicity enhancement of WSV and subunit vaccines described in the following section (Whole Sporozoite Vaccine Immunogenicity). Current GAP in clinical development include early-arresting liver stage parasites that arrest at a similar developmental stage as RAS (< 24 h) (8, 9). More recent P. yoelii pre-clinical GAP studies have engineered late-arresting liver stage GAP (2–3 days) (10–12). Whole parasites that arrest later in liver stage development (late-arresting GAP) or progress to the blood stage (CPS), provide a larger biomass and broader repertoire of immunogens, permitting lower SPZ doses for protection. For example, clinical CPS studies showed volunteers required 10- to 100-fold fewer parasites than RAS to achieve complete protection against malaria challenge, and pre-clinical late-arresting GAP studies showed that at least 10-fold fewer parasites could provide sterilizing protection and also protect against blood stage challenge (12, 13). Broad protection of late-arresting GAP was demonstrated by immunization with late-arresting P. yoelii GAP, which protected against cross-species challenge using P. berghei SPZ (11); however, heterologous challenge after CPS vaccination resulted in only modest protection after challenge (14). Pf GAP3KO safety has been demonstrated in a human clinical trial in which parasites arrested during liver stage development as all ten volunteers remained blood stage negative (8). However, in a previous safety trial using the first developed GAP, GAP2KO (p36− and p52−) one in six volunteers showed patent blood stage infection (15). GAP2KO arrest later in liver stage development compared to GAP3KO (16), thus highlighting the challenge in developing a late-arresting GAP that is also completely liver-stage attenuated. Nevertheless, several groups are working on the development of late-arresting GAP due to their potential benefits.
Whole Sporozoite Vaccine Immunogenicity
Pre-clinical and clinical studies using GAP, RAS, and CPS have demonstrated safety of vaccination with WSV, immunogenicity, and vaccine efficacy [(17); Reviewed in (6, 8, 18). WSV immunogenicity data supports CD8+ T cells as playing a critical role in protection. CD4+ T cells, γδ T cells, and antibodies are also elicited after immunization, but their roles in the protective immune response are less clear. Complete reviews focused on WSV immunogenicity and potential clinical correlates of protection are discussed elsewhere (19, 20).
Cellular Immunity: CD8+, and γδ T Cells
Numerous studies support roles for cellular immunity in WSV protection, including CD8+, γδ, and CD4+ T cells. The strongest immunological data supports roles for cytokine-producing CD8+ T cells, liver resident CD8+ T cells, and γδ T cells as possible WSV immune correlates during liver stage infection. For example, in rodents, depletion of interferon-gamma (IFN-γ) or CD8+ T cells blocked RAS mediated sterile immunity (21). Subsequent studies were performed to analyze cellular responses and cytokine production after immunization by obtaining peripheral blood mononuclear cells (PBMC) and stimulating with Pf specific antigens, whole SPZ, or infected RBC. For Pf GAP2KO studies, interferon-γ (IFN-γ) producing CD8+ T cell responses increased after immunization (15). For PfSPZ Vaccine immunization, levels of CD8+ T cells that produced effector cytokine molecules [IFN-γ, interleuken-2 (IL-2), or transcription factor-alpha (TNF-α)] increased compared to pre-vaccination (22, 23). However, cytokine-producing T cell responses did not correlate with protection (22, 23).
Non-recirculating liver resident CD8+ T cells represent an immunological marker that cannot be sampled in peripherally circulating cell populations (e.g., PBMC) from human clinical trials. Several studies support liver-resident and IFN-γ-producing CD8+ T cells in providing protection after WSV administration. First, a PfSPZ immunization study was performed and involved different vaccine administration routes in mice, non-human primates (NHP), and humans (24). The greatest protection after CHMI was provided by IV vaccination compared to intradermal or intramuscular. Also, animal studies in mice and NHP identified IFN-γ-producing CD8+ T cells in the liver, which correlated with protection by IV administration (24). Subsequent parabiosis experiments in mice confirmed the existence of liver-resident CD8+ T cells (25), which provided RAS-induced malaria immunity (26). Further PfSPZ vaccination studies of NHP support liver resident IFN-γ-producing CD8+ T cells in providing immunity as these cell levels were ~100-fold higher in liver than in blood (27). Finally, several pre-clinical and clinical PfSPZ and CVac studies have also identified γδ T cells as possible correlates of protection that increase after subsequent vaccinations (22, 23, 27, 28).
While WSV have reduced immunogenicity and efficacy in some pre-exposed individuals (7, 29), vaccination strategies are underway to improve WSV immunogenicity. This work was motivated by numerous studies that support liver-resident CD8+ T cells as primary mediators of long-term pre-erythrocytic immunity. Several groups are developing either “prime and target” or “prime and trap” approaches in which peripheral CD8+ T cells are first primed and then targeting to the liver by high expression of Plasmodium subunit antigens or RAS infection (30, 31). These methods lead to higher levels of liver-resident CD8+ T cells and improved RAS or subunit vaccine efficacy compared to RAS or subunit alone (30, 31). Further clinical studies will determine whether these pre-clinical results translate to protect diverse populations of humans, especially in malarious regions.
Additional WSV immunogenicity studies must be performed to identify strong immune correlates for the purpose of guiding clinical trials, including dose and regimen strategies. These studies may be focused on identifying antigen specificity of important T cell populations (γδ and liver resident CD8+ T cells). For example, sequencing studies of T-cell receptors that bind malaria-specific antigens may identify strong correlates of protection. Moreover, since liver resident T-cells cannot be studied using PBMC from human clinical trials, future studies using NHP will prove valuable in identifying strong immune correlates of protection provided by WSV.
In vitro Sporozoites for Pf Malaria Vaccines
While WSV are the most effective vaccines against Pf malaria, major hurdles prevent the manufacturing of sufficient quantities at a commercial scale. Current methods of vaccination with SPZ include delivery by mosquito bite or by injection after technically challenging and laborious mosquito dissections. Neither approach is practical for large-scale or cost-effective vaccination, which is required for commercialization. Culturing systems that produce infective SPZ independent of mosquitoes would enable scalable production of Pf SPZ for WSV.
Plasmodium Stage Development in Mosquitoes
Continuous culture of blood stage malaria parasites (asexual and sexual) has been established for decades (32–35). However, the in vitro culturing of mosquito stages has proven extremely challenging. To culture SPZ, one must understand Pf development within the mosquito and recapitulate this in culture. Pf develops through five sequential stages in mosquitoes: gamete, zygote, ookinete, oocyst, and SPZ (Figure 1). Prior to mosquito stages, gametocytes are ingested by mosquitoes during a blood meal. After ingestion, gametocytes within the mosquito midgut emerge and transform into gametes that fuse to form a zygote. The zygote undergoes DNA replication and maturation into a motile and invasive ookinete that traverses the mosquito peritrophic membrane and midgut epithelium, and finally embeds into the basal lamina on the midgut periphery. The interaction between the ookinete and the mosquito midgut basal lamina is thought to trigger the transformation into the early oocyst (36–38). The sessile oocyst is the site of SPZ development (up to several thousand SPZ per mature oocyst). Once released, SPZ travel to the salivary glands through the hemocoel where they can be inoculated into a host during a mosquito blood meal (39).
As Plasmodium develops through multiple life cycle stages, studies indicate that transitions are driven by coordinated changes in stage-specific gene expression controlled by the apicomplexan Apetala2 (AP2) family of transcription factors (TFs) (40, 41). The 26 AP2 TFs represent the only known family of Plasmodia TFs and contain at least one AP2 DNA-binding domain (42). TFs controlling stage transitions include AP2-O, AP2-O2, AP2-O3, and AP2-O4 for ookinetes and oocysts (41, 43, 44), AP2-Sp, AP2-Sp2, and AP2-Sp3 for mature oocysts and/or SPZ (41, 45), AP2-G, and AP2-G2 for gametocytes (41, 46, 47), and AP2-L for liver stages (48). These TFs co-regulate expression of genes with common cis promoter elements enabling control of the Plasmodium life cycle (41). Understanding stage transition through gene regulation could improve SPZ culturing systems. Other culturing system improvements could include applying knowledge of the parasite's environment while developing in mosquitoes.
Plasmodium in vitro Sporozoite Culturing Systems
Although several publications already exist that describe in vitro culturing of Plasmodium SPZ for the mouse (P. berghei, P. yoelii), avian (P. gallinaceum), and human (Pf) species (49–52), there is only a single publication outlining culturing methods for each species and SPZ characterization. Specific details on the different Plasmodium culturing systems are provided in Table 1. Culturing system factors that likely support production of SPZ include co-culturing with Drosophila support cells (L2/S2), an optimal medium (Roswell Park Memorial Institute [RPMI] or Schneider's), and culturing on a gel-like surrogate for the mosquito basal lamina. All culturing systems required Drosophila co-culturing or using conditioned media (spent media) from L2/S2 cells. Drosophila cells may provide nutrients for the developing oocyst and/or oocyst capsule, including soluble nutrients, growth/signaling factors and/or extracellular matrix molecules that are normally present in the mosquito midgut basal lamina or hemolymph. For culturing media, the avian and human culturing systems utilized RPMI, which is also used for Pf blood stage culture. Subsequent studies on culturing the murine-specific Plasmodia determined that Schneider's media optimally supported Drosophila cell growth (49). Finally, for the basal lamina, all culturing systems except the P. yoelii system found the necessity of Matrigel, which is a basal lamina-like gel derived from murine Engleberth-Holm-Swarm tumor cells and consists of laminin, collagen type IV, heparan sulfate proteoglycan, entactin, and growth factors. Laminin and collagen IV are known components of the mosquito basal lamina and are considered important for ookinete binding and oocyst transformation for mosquito and in vitro development (36–38, 56). In sum, there are shared characteristics of the Plasmodium in vitro sporozoite (IVS) culturing systems that reveal important factors supporting axenic SPZ development, including Drosophila cells, Schneider's media, and Matrigel or a similar basal lamina.
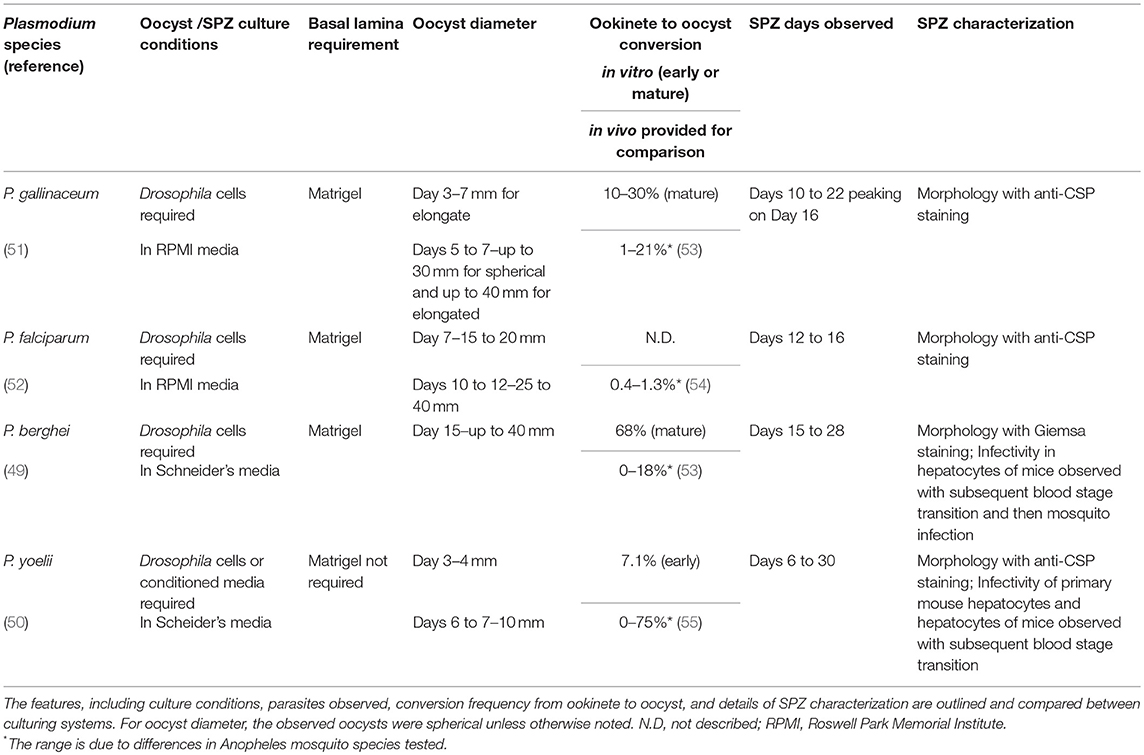
Table 1. Characteristics of in vitro sporozoite culturing systems for Plasmodium gallinaceum, falciparum, berghei and yoelli.
Characterization of Cultured Oocysts and Sporozoites
For the different Plasmodium culturing systems, oocyst, and SPZ development have been characterized to varying degrees (Table 1). The study that describes Pf culturing demonstrated that IVS morphology was similar to mosquito-derived SPZ and that IVS expressed the major SPZ surface protein, CSP (52). However, IVS functionality in terms of motility or hepatocyte traversal/invasion/infection was not analyzed. Similarly, for P. gallinaceum, only morphology and CSP expression were described (51). Separate studies on the rodent malaria parasites, P. berghei (49), and P. yoelii (50) analyzed morphology and sporozoite invasion of hepatocytes in mice. For P. berghei, IVS were produced with similar morphology and mouse infectivity compared to mosquito-derived SPZ, and blood stage infection was also observed (49). For P. yoelii, IVS infected primary hepatocytes in culture at a low rate (1/10,000) and hepatocytes in mice but infectivity was reduced compared to mosquito-derived SPZ. Furthermore, blood stage parasites were only seen in 1/15 infected mice (50).
While these studies demonstrate the feasibility of producing IVS that are capable of hepatocyte infection, many researchers have failed to replicate mosquito-stage culturing of Pf and other Plasmodium species due to the complexity of Plasmodium mosquito stage development and the extreme scientific and technical challenges of establishing culturing systems. We and others, including Sanaria, have made further improvements on developing Pf IVS culturing systems to ultimately produce SPZ for use in WSV development. For example, our Pf culturing system uses the unique nutrient, insect (silk-worm) hemolymph (56). Oocysts and maturing SPZ may receive soluble nutrients, growth factors, and/or signaling factors from the insect hemolymph as from the mosquito hemolymph. Successful in vitro Pf conversion frequencies compared to mosquito development have been achieved for life cycle stages up to the mature oocyst stage (56). Current developing approaches are focused on improving conversion to the mature oocyst and SPZ stages by using 3-dimensional culturing on non-Matrigel basal lamina-like substrates. Matrigel presents challenges for cGMP vaccine production as it is tumor-derived and may vary between batches.
Summary and Future Considerations
Future hurdles for the production of IVS to use in WSV include demonstrating equivalent functionality compared to mosquito-derived SPZ in terms of hepatocyte invasion/infection using cell lines and human-liver chimeric mouse models (57). While P. berghei IVS exhibit infectivity comparable to mosquito-derived SPZ, P. yoelii IVS have decreased infectivity, and hepatocyte infectivity was not assessed for Pf or P. gallinaceum IVS. IVS may resemble immature mosquito midgut SPZ more so than fully infectious mosquito salivary gland SPZ, which further develop in the mosquito hemocoel and salivary gland (58). Additional cues may be necessary for the IVS culturing systems to produce fully infectious, mature SPZ for use as a WSV. Moreover, GAP or RAS IVS will also need to satisfy safety requirements in terms of liver stage arrest and demonstrate absence of toxins, pathogens, or other contaminants. Thus, hurdles must be overcome in the purification of IVS from the 3D basal lamina and from Drosophila cells while maintaining IVS functionality. If these issues can be solved, culturing of SPZ would provide scalable production of WSV for further development as Pf malaria vaccines.
Author Contributions
LSI, YZ, JD, AMG, AMV, and AKG developed the manuscript. LSI and AKG drafted the manuscript. All authors read and approved the final manuscript.
Funding
The manuscript was supported by private funds from MalarVx, Inc.
Disclaimer
The opinions expressed in this article are the author's own and do not reflect the view of the National Institutes of Health, the Department of Health and Human Services, or the United States government.
Conflict of Interest Statement
YZ, JD, AMG, and AKG are employed by a malaria vaccine company, MalarVx, Inc. LSI was employed at MalarVx, Inc. at the time of the study.
The remaining author declares that the research was conducted in the absence of any commercial or financial relationships that could be construed as a potential conflict of interest.
Acknowledgments
The authors acknowledge Mr. James W. Davie for supporting this manuscript.
Abbreviations
CHMI, controlled human malaria infection; CPS, chemoprophylaxis with Pf sporozoites; CSP, circumsporozoite protein; CVac, CPS using Sanaria's injectable sporozoites; GAP, genetically attenuated parasite; IFN-γ, interferon-γ; IL-2, interleuken-2; ITV, infection treatment vaccination; IVS, in vitro sporozoite; NHP, non-human primate; Pf, Plasmodium falciparum; PMBC, peripheral blood mononuclear cells; RAS, radiation attenuated sporozoite; RBC, red blood cell; SPZ, sporozoite; RPMI, Roswell Park Memorial Institute; RTS, S, RTS, S/AS-01; SPZ, sporozoite; TNF-α, transcription factor α; WHO, World Health Organization; WSV, whole sporozoite vaccine.
References
2. Gardner MJ, Hall N, Fung E, White O, Berriman M, Hyman RW, et al. Genome sequence of the human malaria parasite Plasmodium falciparum. Nature (2002) 419:498–511. doi: 10.1038/nature01097
3. White MT, Verity R, Griffin JT, Asante KP, Owusu-Agyei S, Greenwood B, et al. Immunogenicity of the RTS,S/AS01 malaria vaccine and implications for duration of vaccine efficacy: secondary analysis of data from a phase 3 randomised controlled trial. Lancet Infect Dis. (2015) 15:1450–8. doi: 10.1016/S1473-3099(15)00239-X
4. RTSS Clinical Trials Partnership. Efficacy and safety of RTS,S/AS01 malaria vaccine with or without a booster dose in infants and children in Africa: final results of a phase 3, individually randomised, controlled trial. Lancet (2015) 386:31–45. doi: 10.1016/S0140-6736(15)60721-8
5. Clyde DF, Most H, McCarthy VC, Vanderberg JP. Immunization of man against sporozoite-induced falciparum malaria. Am J Med Sci. (1973) 266:169–77. doi: 10.1097/00000441-197309000-00002
6. Hoffman SL, Vekemans J, Richie TL, Duffy PE. The march toward malaria vaccines. Vaccine (2015) 33:13–23. doi: 10.1016/j.vaccine.2015.07.091
7. Sissoko MS, Healy SA, Katile A, Omaswa F, Zaidi I, Gabriel EE, et al. Safety and efficacy of PfSPZ vaccine against Plasmodium falciparum via direct venous inoculation in healthy malaria-exposed adults in Mali: a randomised, double-blind phase 1 trial. Lancet Infect Dis. (2017) 17:498–509. doi: 10.1016/S1473-3099(17)30104-4
8. Kublin JG, Sack BK, Fishbaugher ME, Seilie A, Shelton L, VonGoedert T, et al. Complete attenuation of genetically engineered Plasmodium falciparum sporozoites in human subjects. Sci Transl Med. (2017) 9:1–11. doi: 10.1126/scitranslmed.aad9099
9. van Schaijk BC, Ploemen IH, Annoura T, Vos MW, Foquet L, van Gemert GJ, et al. A genetically attenuated malaria vaccine candidate based on P. falciparum b9/slarp gene-deficient sporozoites. Elife (2014) 3:e03582. doi: 10.7554/eLife.03582
10. Vaughan AM, O'Neill MT, Tarun AS, Camargo N, Phuong TM, Aly ASI, et al. Type II fatty acid synthesis is essential only for malaria parasite late liver stage development. Cell Microbiol. (2009) 11:506–20. doi: 10.1111/j.1462-5822.2008.01270.x
11. Butler NS, Schmidt NW, Vaughan AM, Aly AS, Kappe SH, Harty JT. Superior antimalarial immunity after vaccination with late liver stage-arresting genetically attenuated parasites. Cell Host Microbe (2011) 9:451–62. doi: 10.1016/j.chom.2011.05.008
12. Vaughan AM, Sack BK, Dankwa D, Minkah N, Nguyen T, Cardamone H, et al. A plasmodium parasite with complete late liver stage arrest protects against preerythrocytic and erythrocytic stage infection in mice. Infect Immun. (2018) 86:e00088–18. doi: 10.1128/IAI.00088-18
13. Bijker EM, Bastiaens GJ, Teirlinck AC, van Gemert GJ, Graumans W, van de Vegte-Bolmer M, et al. Protection against malaria after immunization by chloroquine prophylaxis and sporozoites is mediated by preerythrocytic immunity. Proc Natl Acad Sci USA. (2013) 110:7862–7. doi: 10.1073/pnas.1220360110
14. Walk J, Reuling IJ, Behet MC, Meerstein-Kessel L, Graumans W, van Gemert GJ, et al. Modest heterologous protection after Plasmodium falciparum sporozoite immunization: a double-blind randomized controlled clinical trial. BMC Med. (2017) 15:168. doi: 10.1186/s12916-017-0923-4
15. Spring M, Murphy J, Nielsen R, Dowler M, Bennett JW, Zarling S, et al. First-in human evaluation of genetically attenuated Plasmodium falciparum sporozoites administered by bite of Anopheles Mosquitoes to adult volunteers. Vaccine (2013) 31:4975–83. doi: 10.1016/j.vaccine.2013.08.007
16. VanBuskirk KM, O'Neill MT, De La Vega P, Maier AG, Krzych U, Williams J, et al. Preerythrocytic, live-attenuated Plasmodium falciparum vaccine candidates by design. Proc Natl Acad Sci USA. (2009) 106:13004–9. doi: 10.1073/pnas.0906387106
17. Mikolajczak SA, Lakshmanan V, Fishbaugher M, Camargo N, Harupa A, Kaushansky A, et al. A next-generation genetically attenuated Plasmodium falciparum parasite created by triple gene deletion. Mol Ther. (2014) 22:1707–15. doi: 10.1038/mt.2014.85
18. Mordmüller B, Surat G, Lagler H, Chakravarty S, Ishizuka AS, Lalremruata A, et al. Sterile protection against human malaria by chemoattenuated PfSPZ vaccine. Nature (2017) 542:445–9. doi: 10.1038/nature21060
19. Doll KL, Harty JT. Correlates of protective immunity following whole sporozoite vaccination against malaria. Immunol Res. (2014) 59:166–76. doi: 10.1007/s12026-014-8525-0
20. Draper SJ, Sack BK, King CR, Nielsen CM, Rayner JC, Higgins MK, et al. Malaria vaccines: recent advances and new horizons. Cell Host Microbe (2018) 24:43–56. doi: 10.1016/j.chom.2018.06.008
21. Schofield L, Villaquiran J, Ferreira A, Schellekens H, Nussenzweig R, Nussenzweig V. Gamma interferon, CD8+ T cells and antibodies required for immunity to malaria sporozoites. Nature (1987) 330:664–6.
22. Seder RA, Chang LJ, Enama ME, Zephir KL, Sarwar UN, Gordon IJ, et al. Protection against malaria by intravenous immunization with a nonreplicating sporozoite vaccine. Science (2013) 341:1359–65. doi: 10.1126/science.1241800
23. Lyke KE, Ishizuka AS, Berry AA, Chakravarty S, DeZure A, Enama ME, et al. Attenuated PfSPZ vaccine induces strain- transcending T cells and durable protection against heterologous controlled human malaria infection. Proc Natl Acad Sci USA. (2017) 114:2711–6. doi: 10.1073/pnas.1615324114
24. Epstein JE, Tewari K, Lyke KE, Sim BK, Billingsley PF, Laurens MB, et al. Live attenuated malaria vaccine designed to protect through hepatic CD8+ T cell immunity. Science (2011) 334:475–80. doi: 10.1126/science.1211548
25. Steinert EM, Schenkel JM, Fraser KA, Beura LK, Manlove LS, Igyártó BZ, et al. Quantifying memory CD8 T cells reveals regionalization of immunosurveillance. Cell (2015) 161:737–49. doi: 10.1016/j.cell.2015.03.031
26. Fernandez-Ruiz D, Ng WY, Holz LE, Ma JZ, Zaid A, Wong YC, et al. Liver-resident memory CD8+ T cells form a front-line defense against malaria liver-stage infection. Immunity (2016) 45:889–902. doi: 10.1016/j.immuni.2016.08.011
27. Ishizuka AS, Lyke KE, DeZure A, Berry AA, Richie TL, Mendoza FH, et al. Protection against malaria at 1 year and immune correlates following PfSPZ vaccination. Nat Med. (2016) 22:614–23. doi: 10.1038/nm.4110
28. Zaidi I, Diallo H, Conteh S, Robbins Y, Kolasny J, Orr-Gonzalez S, et al. γδ T Cells Are required for the induction of sterile immunity during irradiated sporozoite vaccinations. J Immunol. (2017) 199:3781–8. doi: 10.4049/jimmunol.1700314
29. Olotu A, Urbano V, Hamad A, Eka M, Chemba M, Nyakarungu E, et al. Advancing global health through development and clinical trials partnerships: a randomized, placebo-controlled, double-blind assessment of safety, tolerability, and immunogenicity of PfSPZ vaccine for malaria in healthy Equatoguinean men. Am J Trop Med Hyg. (2018) 98:308–18. doi: 10.4269/ajtmh.17-0449
30. Gola A, Silman D, Walters AA, Sridhar S, Uderhardt S, Salman AM, et al. Prime and target immunization protects against liver-stage malaria in mice. Sci Transl Med. (2018) 10:eaap9128. doi: 10.1126/scitranslmed.aap9128
31. Olsen TM, Stone BC, Chuenchob V, Murphy SC. Prime-and-trap malaria vaccination to generate protective CD8+ liver-resident memory T cells. J Immunol. (2018) 201:1984–93. doi: 10.4049/jimmunol.1800740
32. Haynes DJ, Diggs CL, Hines FA, Desjardins RE. Culture of human malaria parasites Plasmodium falciparum. Nature (1976) 263:767–9. doi: 10.1038/263767a0
33. Ifediba T, Vanderberg JP. Complete in vitro maturation of Plasmodium falciparum gametocytes. Nature (1981) 294:364–6. doi: 10.1038/294364a0
34. Ponnudurai T, Meuwissen JH, Leeuwenberg AD, Verhave JP, Lensen AH. The production of mature gametocytes of Plasmodium falciparum in continuous cultures of different isolates infective to mosquitoes. Trans R Soc Trop Med Hyg. (1982) 76:242–50. doi: 10.1016/0035-9203(82)90289-9
36. Arrighi RB, Hurd H. The role of Plasmodium berghei ookinete proteins in binding to basal lamina components and transformation into oocysts. Int J Parasitol. (2002) 32:91–8. doi: 10.1016/S0020-7519(01)00298-3
37. Adini A, Warburg A. Interaction of Plasmodium gallinaceum ookinetes and oocysts with extracellular matrix proteins. Parasitology (1999) 119:331–6. doi: 10.1017/S0031182099004874
38. Vlachou D, Lycett G, Siden-Kiamos I, Blass C, Sinden RE, Louis C. Anopheles gambiae laminin interacts with the P25 surface protein of Plasmodium berghei ookinetes. Mol Biochem Parasitol. (2001) 112:229–37. doi: 10.1016/S0166-6851(00)00371-6
39. Sinden RE. The biology of Plasmodium in the mosquito. Experientia (1984) 40:1330–43. doi: 10.1007/BF01951886
40. Zhou Y, Ramachandran V, Kumar KA, Westenberger S, Refour P, Zhou B, et al. Evidence-based annotation of the malaria parasite's genome using comparative expression profiling. PLoS ONE (2008) 3:e1570.
41. Modrzynska K, Pfander C, Chappell L, Yu L, Suarez C, Dundas K, et al. A knockout screen of ApiAP2 genes reveals networks of interacting transcriptional regulators controlling the plasmodium life cycle. Cell Host Microbe (2017) 21:11–22. doi: 10.1016/j.chom.2016.12.003
42. Balaji S, Babu MM, Iyer LM, Aravind L. Discovery of the principal specific transcription factors of Apicomplexa and their implication for the evolution of the AP2-integrase DNA binding domains. Nucleic Acids Res. (2005) 33:3994–4006. doi: 10.1093/nar/gki709
43. Yuda M, Iwanaga S, Shigenobu S, Mair GR, Janse CJ, Waters AP, et al. Identification of a transcription factor in the mosquito-invasive stage of malaria parasites. Mol Microbiol. (2009) 71:1402–14. doi: 10.1111/j.1365-2958.2009.06609.x
44. Kaneko I, Iwanaga S, Kato T, Kobayashi I, Yuda M. Genome-wide identification of the target genes of AP2-O, a plasmodium AP2-family transcription factor. PLoS Pathog. (2015) 11:e1004905. doi: 10.1371/journal.ppat.1004905
45. Yuda M, Iwanaga S, Shigenobu S, Kato T, Kaneko I. Transcription factor AP2-Sp and its target genes in malarial sporozoites. Mol Microbiol. (2010) 75:854–63. doi: 10.1111/j.1365-2958.2009.07005.x
46. Kafsack BFC, Rovira-Graells N, Clark TG, Bancells C, Crowley VM, Campino SG, et al. A transcriptional switch underlies commitment to sexual development in malaria parasites. Nature (2014) 507:248–52. doi: 10.1038/nature12920
47. Sinha A, Hughes KR, Modrzynska KK, Otto TD, Pfander C, Dickens NJ, et al. A cascade of DNA-binding proteins for sexual commitment and development in Plasmodium. Nature (2014) 507:253–7. doi: 10.1038/nature12970
48. Iwanaga S, Kaneko I, Kato T, Yuda M. Identification of an AP2-family protein that is critical for malaria liver stage development. PLoS ONE (2012) 7:e47557. doi: 10.1371/journal.pone.0047557
49. Al-Olayan EM, Beetsma AL, Butcher GA, Sinden RE, Hurd H. Complete development of mosquito phases of the malaria parasite in vitro. Science (2002) 295:677–9. doi: 10.1126/science.1067159
50. Porter-Kelley JM, Dinglasan RR, Alam U, Ndeta GA, Sacci JB Jr, Azad AF. Plasmodium yoelii: axenic development of the parasite mosquito stages. Exp Parasitol. (2006) 112:99–108. doi: 10.1016/j.exppara.2005.09.011
51. Warburg A, Miller LH. Sporogonic Development of a malaria parasite in vitro. Science (1992) 255:448–50. doi: 10.1126/science.1734521
52. Warburg A, Schneider I. In vitro culture of the mosquito stages of Plasmodium falciparum. Exp Parasitol. (1993) 76:121–6. doi: 10.1006/expr.1993.1014
53. Alavi Y, Arai M, Mendoza J, Tufet-Bayona M, Sinha R, Fowler K, et al. The dynamics of interactions between Plasmodium and the mosquito: a study of the infectivity of Plasmodium berghei and Plasmodium gallinaceum and their transmission by Anopheles stephensi, Anopheles gambiae and Aedes aegypti. Int J Parasitol. (2003) 33:933–43. doi: 10.1016/s0020-7519(03)00112-7
54. Vaughan JA, Noden BH, Beier JC. Sporogonic development of cultured Plasmodium falciparum in six species of laboratory-reared Anopheles mosquitoes. Am J Trop Med Hyg. (1994) 51:233–43. doi: 10.4269/ajtmh.1994.51.233
55. Vaughan JA, Hensley L, Beier JC. Sporogonic development of Plasmodium yoelii in five anopheline species. J Parasitol. (1994) 80:674–81. doi: 10.2307/3283245
56. Itsara LS, Zhou Y, Do J, Dungel S, Fishbaugher ME, Betz WW, et al. PfCap380 as a marker for Plasmodium falciparum oocyst development in vivo and in vitro. Malar J. (2018). 17:135. doi: 10.1186/s12936-018-2277-6
57. Vaughan AM, Mikolajczak SA, Camargo N, Lakshmanan V, Kennedy M, Lindner SE, et al. A transgenic Plasmodium falciparum NF54 strain that expresses GFP-luciferase throughout the parasite life cycle. Mol Biochem Parasitol. (2012) 186:143–7. doi: 10.1016/j.molbiopara.2012.10.004
Keywords: Plasmodium falciparum, malaria, whole sporozoite vaccines, genetically attenuated parasite, radiation attenuated sporozoite, in vitro culturing
Citation: Itsara LS, Zhou Y, Do J, Grieser AM, Vaughan AM and Ghosh AK (2018) The Development of Whole Sporozoite Vaccines for Plasmodium falciparum Malaria. Front. Immunol. 9:2748. doi: 10.3389/fimmu.2018.02748
Received: 22 August 2018; Accepted: 08 November 2018;
Published: 11 December 2018.
Edited by:
Ashraful Haque, QIMR Berghofer Medical Research Institute, AustraliaReviewed by:
Ian Andrew Cockburn, Australian National University, AustraliaDaniel Fernandez-Ruiz, Peter Doherty Institute for Infection and Immunity, Australia
Copyright © 2018 Itsara, Zhou, Do, Grieser, Vaughan and Ghosh. This is an open-access article distributed under the terms of the Creative Commons Attribution License (CC BY). The use, distribution or reproduction in other forums is permitted, provided the original author(s) and the copyright owner(s) are credited and that the original publication in this journal is cited, in accordance with accepted academic practice. No use, distribution or reproduction is permitted which does not comply with these terms.
*Correspondence: Anil K. Ghosh, QWdob3NoQG1hbGFydnguY29t
†Present Address: Leslie S. Itsara, National Institutes of Health (NIH), Bethesda, MD, United States