- Department of Biomedical Sciences, University of Illinois, Rockford, IL, United States
Lymphatic filariasis (LF) is a tropical parasitic infection of human transmitted by mosquitoes. Chronic infection results in severe physical disability in the infected patients. Although several potential vaccine antigens were identified by several groups, there are no licensed prophylactic vaccine to date against this infection in the human. Previous attempts from our laboratory to develop a trivalent prophylactic vaccine against LF showed that >90% protection could be achieved in rodent models. However, this trivalent vaccine gave only 35% protection in non-human primates. The major focus of this study was to develop a tetravalent prophylactic vaccine (rBmHAXT) and test the vaccine potential in a mouse model. We evaluated three different adjuvant formulations; alum, glucopyranosyl lipid adjuvant in stable emulsion (GLA/SE) alum (AL019), and mannosylated chitosan (MCA) to determine the optimum adjuvant formulation for rBmHAXT. Results presented in this study show that rBmHAXT + AL019 gave the highest rate of protection (>88%) against challenge infection, compared to rBmHAXT + AL007 (79%), rBmHAXT + MCA (79%) and controls. Analysis of the immune correlates of protection showed that all three adjuvants elicited high titer of antigen-specific IgG1, IgG2a, and IgG2b antibodies. High number of IFN-γ-producing antigen-specific memory cells were generated in the vaccinated animals irrespective of the adjuvants used. Similarly, spleen cells from rBmHAXT-vaccinated animals secreted IL-4, IL-10, and IFN-γ in response to rBmHAXT suggesting the generation of a balanced Th1/Th2 response. There was also an increase in IL-17-secreting cells in rBmHAXT-vaccinated animals. These findings thus suggest that rBmHAXT + AL019 is a better prophylactic formulation for LF.
Introduction
Lymphatic filariasis (LF) is a neglected tropical parasitic disease caused by three filarial parasites, Wuchereria bancrofti, Brugia malayi, and Brugia timori and is transmitted by mosquitoes. According to the World Health Organization, currently 856 million people residing in 52 countries require preventive chemotherapy to stop the spread of infection (1–4). As a preventive strategy, The Global Program to Eliminate LF was launched in 2000 to eradicate LF by 2020 using Mass Drug Administration (MDA) with diethylcarbamazine (6 mg/kg) and albendazole (400 mg) (3). So far, preventive chemotherapy is the only option to stop the spread of LF infection in endemic regions. Ten countries (Egypt, Cambodia, Cook Islands, Maldives, Marshall Islands, Niue, Sri Lanka, Thailand, Togo, and Vanuatu) have to date declared achieving elimination of LF (4). However, there have been few reports of reemergence of LF infection in Sri Lanka (5). Preventive chemotherapy is still needed in all other countries endemic for this disease and has not achieved the desired levels of coverage as of 2017 (6, 7). As in Sri Lanka, there is fear of reemergence of the infection in areas where MDA was given, mainly due to subject noncompliance (8–11). Even one infected individual left untreated in a community can be disastrous because drugs can only treat current infection and will not prevent future infections, especially once the drug effect wanes from the system. Therefore, there is a need for a more sustainable approach to control and eliminate the infection. Prophylactic vaccination with MDA will be a more sustainable and long lasting approach to eliminate infection from a community by eliciting herd immunity (2).
Several potential vaccine candidates were identified from our laboratory and others (12–21) were shown to confer varying degrees of protection against challenge infection with B. malayi in experimental animals. Out of the several candidate vaccine antigens reported to date, we selected three best candidate antigens; heat shock protein 12.6 (HSP-12.6) (22), abundant larval transcript-2 (ALT-2) (21), and tetraspanin large extracellular loop (TSPLEL) (23) that repeatedly showed maximum protection in rodent models in our laboratory and other. Two of the selected antigens were then combined with a bivalent vaccine antigen and tested the vaccine potential in rodent models (24–28). Subsequently, we combined three of the antigens with a trivalent fusion protein vaccine and tested in rodents (29–33) and in non-human primates (34). The trivalent vaccine gave excellent protection against challenge infections in the rodent models (~95% protection). However, the trivalent vaccine (rBmHAT) when given along with alum gave only ~35% protection in non-human primates (34). The immune responses generated following the vaccination in non-human primates was predominantly Th2 biased and this was attributed to the poor protective responses. Protection against LF in the human and rhesus macaques is correlated with a balanced Th1/Th2 response (29, 35, 36). Therefore, the major focus of this study was to construct a tetravalent vaccine (rBmHAXT) by adding another antigen, thioredoxin peroxide (BmTPX-2) that is known to promote a Th1 response, to the trivalent vaccine and evaluate its vaccine potential in a mouse model. Thioredoxin peroxidase (TPX-2) is a highly immunogenic protein within the total secretome of B. malayi (37–39). Several groups including ours have reported the vaccine potential of TPX-2 previously (24, 27, 28, 40–42). In fact, one of our studies showed that a trivalent fusion protein rBmHAX (HSP12.6 + ALT-2 + TPX-2) vaccine conferred 67.5% protection in the mouse model (30). Immunization with TPX-2 skewed the protective immune response to a Th1 bias (30, 41, 43). Therefore, we hypothesized that a tetravalent fusion protein could generate a more balanced Th1/Th2 response and will be more effective as a prophylactic vaccine against LF infection. In this study, we also evaluated the potential of three different adjuvant formulations [alum (AL007), glucopyranosyl lipid adjuvant in stable emulsion (GLA-SE)/alum (a synthetic toll-like receptor 4 agonist on alhydrogel, AL019), and mannosylated chitosan (MCA)] for their ability to promote a balanced Th1/Th2 response when given along with the tetravalent rBmHAXT vaccine. One of our previous studies showed that AL019 and MCA are excellent adjuvants for the trivalent rBmHAT vaccine antigen and promoted significant vaccine-induced protection in the mouse model (30).
Thus, the major focus of this study was to evaluate the vaccine potential of a tetravalent fusion protein antigen, rBmHAXT against B. malayi challenge infections in a mouse model.
Materials and Methods
Construction of Tetravalent Coding Sequence
Multivalent gene sequences of bmhaxt consisting of bmhsp12.6 (accession #AY692227.1), bmalt-2 (accession #JF795950.1), bmtpx-2 (accession #AF319997.1), and bmtsp (accession #JF795955.1) was constructed at Genscript (Piscataway, NJ, USA).
Cloning, Expression, and Purification of rBmHAXT Recombinant Protein
GenScript supplied the sequences in the pUC57 vector. The genes were amplified using forward CGGGATCCATGGAAGAAAAGGTAGTG and reverse CCCGAATTCTTAATGTTTCTCAAAATATGCTTT primers with restriction sites for BamHI and EcoRI. The PCR-amplified products were cloned into the pRSETA expression vector, transformed into competent BL21 (DE3) Escherichia coli cells for expression of the recombinant proteins with 6× histidine tag. Recombinant fusion proteins were purified using immobilized metal affinity Ni+-charged Sepharose column (GE Healthcare Life Sciences, Pittsburg, PA) and eluted with 300 mM imidazole. Endotoxin in the final purified protein preparation was removed using an endotoxin removal column (Thermo Fisher Scientific, Rockford, IL, USA). The expression and purity of recombinant proteins was confirmed in 12% SDS-PAGE gel and Western blot using anti-His antibodies (Qiagen, Valencia, CA, USA). Protein concentration was determined using a Bradford reagent (Thermo Fisher Scientific).
Adjuvants
We used three different adjuvant formulations with recombinant BmHAXT. Alum (AL007) and Alum plus GLA, a synthetic TLR4 agonist (AL019) was purchased from the Infectious Disease Research Institute, Seattle, WA and MCA was a gift from Pacific GeneTech, Hong Kong.
Animals and Parasite
Six- to eight-week-old BALB/c mice purchased from Taconic biosciences (Hudson, NY, USA) were used in these experiments. Use of animal in this study was approved by the animal care committee of the University of Illinois, Rockford following the National Institutes of Health guidelines for the care and use of laboratory animals. The infective larval stage (L3) of B. malayi was obtained from the NIAID/NIH Filariasis Research Reagent Resource Center (University of Georgia, Athens, GA, USA).
Immunization of BALB/c Mice
For the immunization, mice were randomly divided into seven groups of five mice each per group as described below: (1) rBmHAXT + AL007 given s/c, (2) rBmHAXT + AL019 given s/c, (3) rBmHAXT + MCA (first dose s/c and booster doses given orally), (4) AL007 control given s/c, (5) AL019 control given s/c, (6) MCA control (first dose s/c and booster doses given orally), and (7) rBmHAXT + MCA control (all doses were given orally). Each mouse received three doses of 15 µg of rBmHAXT and 15 µg of respective adjuvant formulation at 15 days interval.
Collection of Serum, Peritoneal Fluid, and Spleen
Blood samples were collected from the submandibular vein of each mouse on day 0 (pre-immune), and then 2 weeks after each immunization and kept at room temperature for 1 h to clot. Serum was separated, and aliquots were kept frozen at −80°C for further use. Peritoneal cavity was washed with 500 µl of sterile saline solution and the fluid was collected from each mouse and processed. Spleen was then collected from each animal, washed three times with complete RPMI-1640 medium supplemented with 10% FBS and 1 × antibiotic/mycotic solution (Sigma, St. Louis, MO, USA).
Determining the Antibody Titer by ELISA
Titer of IgG Antibodies in the Serum and Peritoneal Fluids
The titer of rBmHAXT-specific IgG antibodies in the sera samples and in the peritoneal fluids were evaluated using an indirect ELISA as described previously. Wells were coated with 1 µg/ml of rBmHAXT overnight at 4°C. After washing and blocking the plates, diluted (1:100, 1:1,000, 1:5,000, 1:10,000, 1:20,000, and 1:40,000) sera or peritoneal fluid samples were added and incubated for 1 h at room temperature. HRP-conjugated chicken anti-mouse IgG antibodies (Thermo Fisher scientific) were used as the secondary antibodies and color was developed using the 1-step Ultra TMB-ELISA substrate (Thermo Fisher Scientific). The reaction was stopped using 0.16 M H2SO4, and optical density was determined at 450 nm in a BioTek Synergy 2 ELISA reader.
Levels of Antigen-Specific Antibody Isotypes in the Serum and Peritoneal Fluids
Levels of rBmHAXT-specific antibody isotypes (IgG1, IgG2a, IgG2b, IgG3, IgE, IgM and IgA) were determined in the sera and peritoneal fluid samples using an indirect ELISA as described above. Respective isotype-specific biotinylated goat anti-mouse antibodies (Sigma) and streptavidin-HRP (1:20,000) were used as the secondary antibodies. Color was developed with 1-step Ultra-TMB. The reaction was stopped using 0.16 M H2SO4 and optical density was determined at 450 nm in a BioTek Synergy 2 ELISA reader.
Challenge Studies
To determine the vaccine-induced protection, we used a micropore chamber challenge method as described previously (29–33). Briefly, 20 L3s of B. malayi were placed in a micropore chamber and surgically implanted into the peritoneum of each mouse. Following, 72 h implantation, the micropore chambers were recovered. Contents of each chamber were emptied and larvae were counted and examined microscopically for adherence of cells and for larval death. Larvae that were transparent, straight, and with no movement were counted as dead. Larvae that were active, coiled, and translucent were counted as live. The percentage of protection was expressed as the number of dead parasites/number of total parasites recovered × 100.
Levels of Secreted Cytokines in Culture Supernatant of Splenocytes
Single cell suspension of spleen cells was prepared and stimulated with 1 µg/ml of rBmHAXT or ConA. Unstimulated spleen cells were kept as negative control for the assay. After 72 h incubation, culture supernatants were collected and levels of IL-2, IL-4, IL-6, IFNγ, TNFα, IL-10, and IL-17A were determined using a cytokine bead array kit (BD Bio Sciences, San Jose, CA, USA).
Analysis of T Cell Subsets by Flow Cytometer
Spleen cells from the above cultures were then washed and labeled with fluorescent-labeled anti-mouse CD3 (APC), CD4 (PE), and CD8 (PE/cya7) and the percent population of each cell type was determined in a flow cytometer. Briefly, cells were incubated with FcγII receptor blocker in staining buffer (2% FBS + 0.1% sodium azide) for 30 min at 4°C with subsequent wash in staining buffer. All the three fluorescent-labeled antibodies were added to the cells and incubated for 1 h at 4°C in the dark. After washing with staining buffer, cells were fixed in 4% paraformaldehyde and analyzed in a BD Facs Calibur (BD Biosciences) flow cytometer.
Another set of cells from the above experiment was stained with CD3 (APC) and within the CD3 gated population, the CD62L (PE/Cya7) and CCR7 (PE) positive T cells were identified as T-central memory cells. We also stained these cell populations for intracellular IFN-γ (FITC) to determine the percent of IFN-γ positive T-central memory cells.
Statistical Analysis
Data presented are mean ± SD. Statistical significance of mean differences among different sample groups was analyzed using non-parametric Kruskal–Wallis test followed by Bonferroni correction for multiple tests using SPSS software (v24.0, IBM, NY). The significance level was defined as p < 0.05.
Results
rBmHAXT Was Cloned and Purified
We cloned and prepared purified recombinant BmHAXT protein. On the SDS-PAGE gel, the molecular mass of rBmHAXT was around 60 kDa and appeared as a single band (Figure 1). Endotoxin levels in the final purified preparations were <3 EU/0.1 mg of protein.
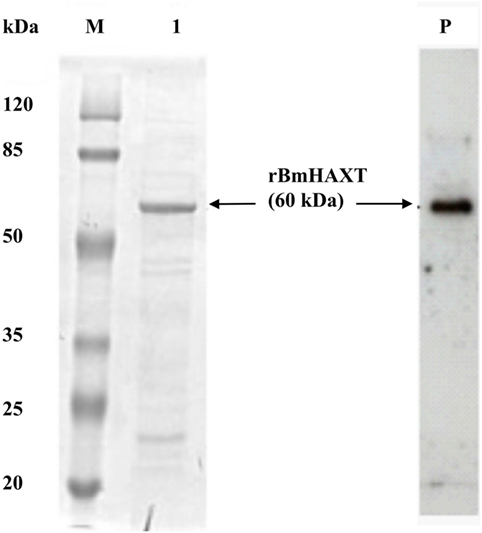
Figure 1. Expression of recombinant proteins. Analysis of expressed and purified rBmHAXT protein was carried out using 12% SDS-PAGE gel. Lanes: M, protein molecular weight marker; 1, rBmHAXT purified. Lane P: Western blot analysis of purified rBmHAXT showing 6× his-tagged protein.
Titer of rBmHAXT-Specific IgG Antibody Was High in the Serum and Peritoneal Fluid of All Immunized Mice
The titer of rBmHAXT-specific IgG antibody was high (1:20,000) in rBmHAXT + AL007 group and in rBmHAXT + AL019 group (p < 0.05) (Figure 2A). However, the titer was less (1:10,000) in rBmHAXT + MCA group. In rBmHAXT + MCA group where all the doses were given orally, we found there was very little titer of antigen-specific antibodies nearly same as AL007, AL019, and MCA adjuvant control groups (Figure 2A). Similarly, when we analyzed the peritoneal fluids, we observed high titer of antigen-specific IgG antibody in rBmHAXT + AL019 and rBmHAXT + AL007-vaccinated animals when compared to rBmHAXT + MCA group (Figure 2B). The titer of IgG antibodies was less in the peritoneal fluids when compared to the respective sera samples from the same animals.
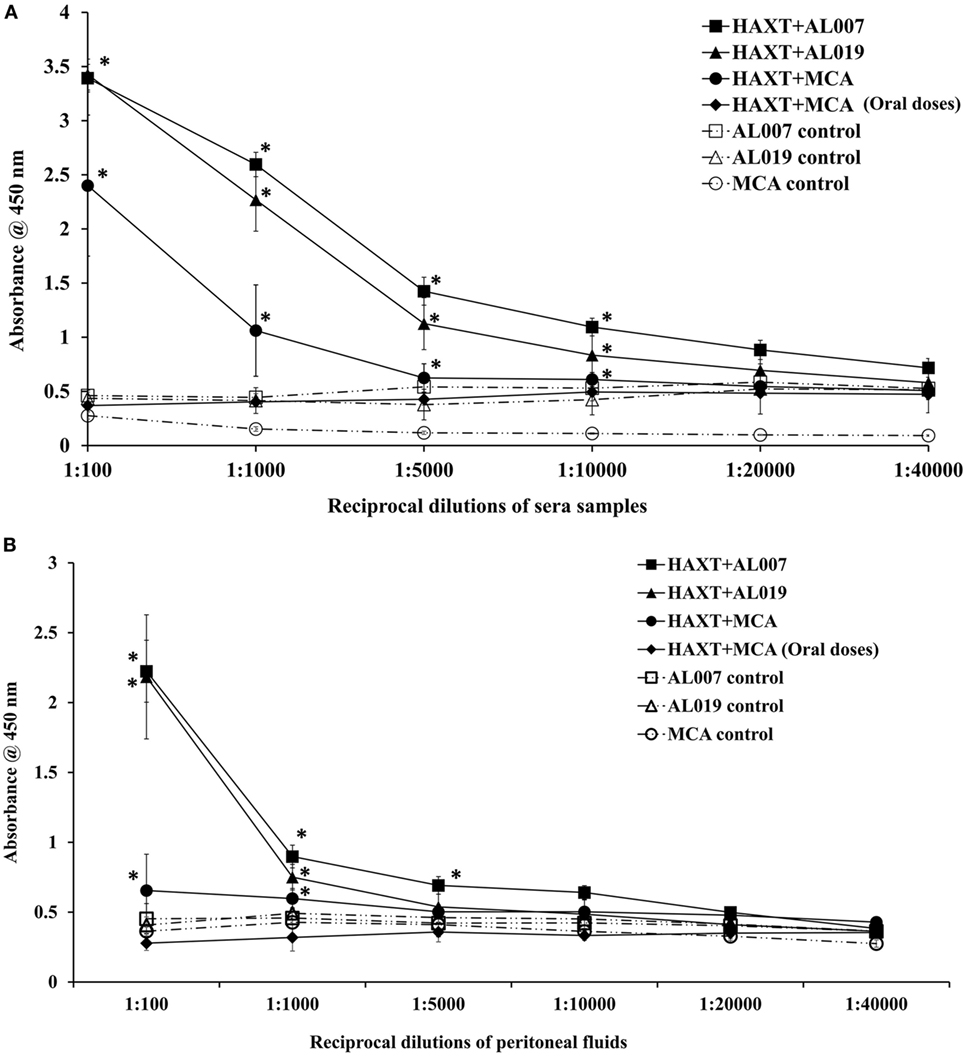
Figure 2. Titer of rBmHAXT-specific IgG antibodies in immunized animals. (A) rBmHAXT-specific IgG titer in sera samples showed that both AL007 and AL019 adjuvants-based formulations are better in generating the IgG titer (1:20,000) when compared to mannosylated chitosan (MCA) formulations (1:10,000). Pre-immune sera samples were used as baseline controls in these assays. (B) In peritoneal fluid, titer of IgG is comparatively low (1:10,000) in AL007 and AL019-based formulations when compared to sera samples. In contrast, IgG titer is significantly reduced in rBmHAXT + MCA-treated animal’s peritoneal fluid than sera samples. n = 5 per group, *p < 0.05 compared to respective group as analyzed by Kruskal–Wallis test followed by Bonferroni correction for multiple analysis.
Antibody Isotypes in Serum and Peritoneal Fluid
To determine the type of humoral immune response generated against rBmHAXT, we determined the antibody isotypes IgG1, IgG2a, IgG2b, IgG3, IgE, IgM, and IgA in serum and peritoneal fluid samples. Our results showed that, IgG1 was the predominant isotype of antibody in all vaccinated groups (p = 0.0001) except rBmHAXT + MCA (all oral dose) group which was similar to the controls (Figure 3A). Titer of IgG2a and IgG2b antibodies were also significantly high in all vaccinated animals (p < 0.05) compared to controls except in rBmHAXT + oral MCA-vaccinated group (Figure 3A). Titer of IgG3, IgE, IgM, and IgA antibodies in the vaccinated animals did not show any significant changes compared to the controls. Significantly, high titers of IgG1 antibodies were present in the peritoneal fluids of rBmHAXT + AL007 and rBmHAXT + AL019-vaccinated animals (p = 0.0001) compared to rBmHAXT + MCA subcutaneous group and controls (Figure 3B). Titer of IgG2a and IgG2b antibodies in the peritoneal fluid of all vaccinated animals were not significantly different from the controls (Figure 3B).
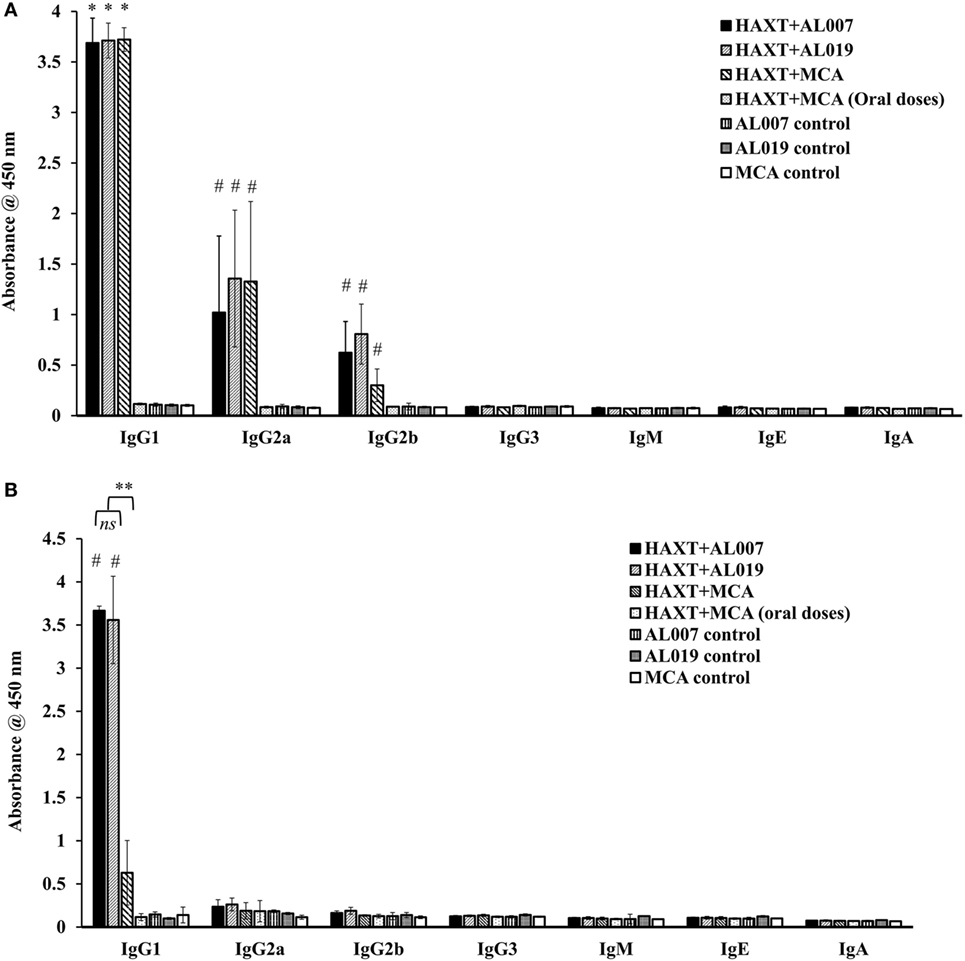
Figure 3. Level of antigen-specific antibody isotypes was determined by indirect ELISA in sera samples as well as in peritoneal fluid. (A) IgG1 was found predominant in all the immunized animals when compared to their respective adjuvant control groups. Specifically, IgG2a was found to be increased in rBmHAXT + AL019 and rBmHAXT + mannosylated chitosan (MCA) groups compared to the rBmHAXT + AL007 group. IgG2b antibody level was significantly increased in rBmHAXT + AL019 immunized group (B) IgG1 is much lesser in the peritoneal fluid of rBmHAXT + MCA (s/c) group when compared to rBmHAXT + AL007 and rBmHAXT + AL019 groups. Moreover, IgG2a and IgG2b antibodies were not detected in peritoneal fluid of all the group of animals as observed in the sera of vaccinated animals. n = 5 per group, *p < 0.05, #p < 0.001 compared to respective control group. **p < 0.001 compared to rBmHAXT + MCA group. ns = not significant, as analyzed by Kruskal-Wallis test followed by Bonferroni correction for multiple analysis.
Vaccination With rBmHAXT + AL019 Conferred Maximum Protection
Vaccine-induced protection was determined using a micropore chamber challenge method as described previously (28–33). Our results showed that maximum protection was observed in animals vaccinated with rBmHAXT + AL019 (88.05 ± 3.9%; p = 0.0001) followed by rBmHAXT + AL007 (79.47 ± 2.6%; p = 0.0001) and rBmHAXT + MCA (78.67 ± 5.47%; p = 0.0001) (Figure 4). Larvae collected from micropore chamber were observed under the light microscope and showed several cells were found attached to the surface of the dead larvae. These results suggest that AL019 may be a better adjuvant for rBmHAXT compared to AL007 (p = 0.0037) and MCA (p = 0.02). Vaccination with rBmHAXT + oral MCA conferred only 17.97 ± 5.75%, which was similar to the adjuvant control groups (Figure 4).
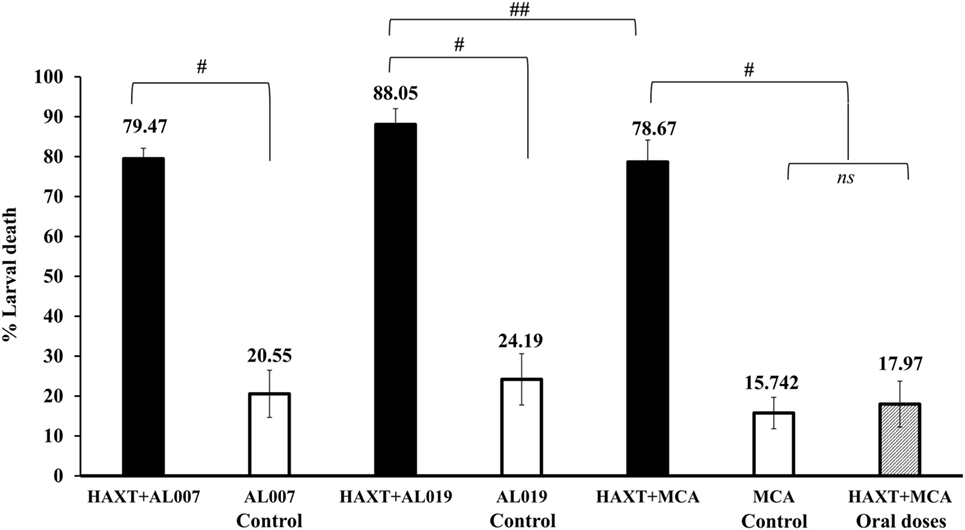
Figure 4. Vaccine-induced protection was calculated by percent larval death in immunized animals. Approximately, 20 live L3s were sealed in micropore chambers and surgically implanted in peritoneal cavity of mice. After 72 h, micropore chambers were taken out and L3s larvae were recovered and counted as live and dead worms to determine the percent larval death. Compared to adjuvant control groups, there was significant death of larva in vaccinated groups. Results showed that rBmHAXT + AL019 immunized group showed maximum protection (88%), followed by 79.47 and 78.67% in rBmHAXT + AL007 and rBmHAXT + mannosylated chitosan, respectively. n = 5 mice per group, #p < 0.0001 and ##p < 0.05, ns = not significant compared to respective group as analyzed by Kruskal–Wallis test followed by Bonferroni correction for multiple analysis.
Spleen Cells From rBmHAXT-Vaccinated Animals Secreted Both Th1 and Th2 Cytokines
Cytokines level in the culture supernatants of spleen cells was determined using a cytokine bead array. Our results showed that secreted levels of Th1 (IFN-γ, IL-2, IL-6, and IL-17A) and Th2 (IL-4 and IL-10) cytokines were significantly (p < 0.05) increased in the culture supernatants of spleen cells from rBmHAXT + AL019 and rBmHAXT + AL007-vaccinated animals compared to respective adjuvant control animals (Figure 5). Spleen culture supernatants from rBmHAXT + MCA-vaccinated animals had significantly high levels of IFN-γ (p = 0.01) and IL-6 (p = 0.0001) (Figure 5) compared to the respective adjuvant control. However, there was no significant difference in the levels of the other cytokines measured. Cytokine levels in the culture supernatants from rBmHAXT + oral MCA group were similar to the adjuvant controls (Figure 5).
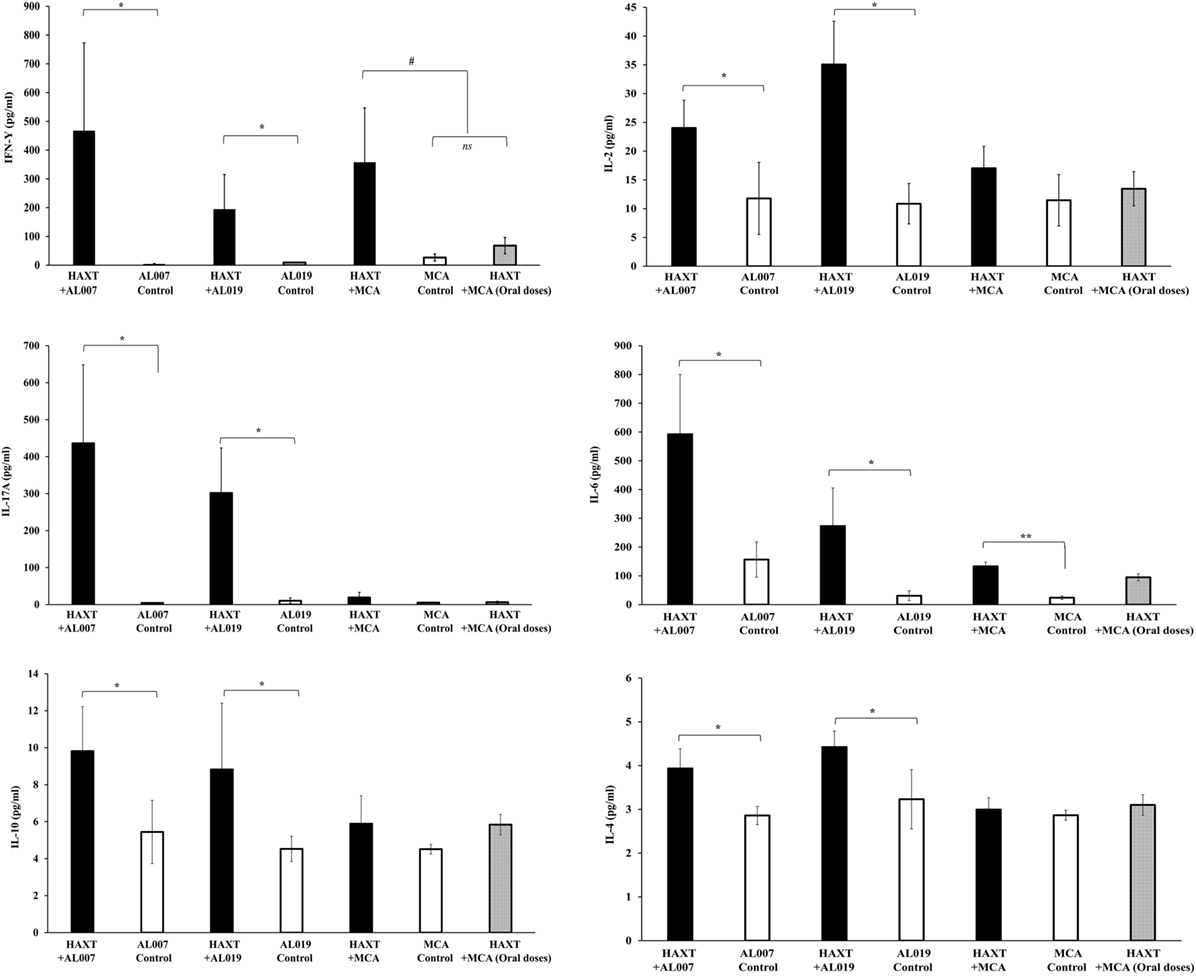
Figure 5. Level of cytokines (IFN-γ, IL-2, IL-6, IL-17A, IL-4, and IL-10) in culture supernatant of spleen cells were determined by cytokine bead array. After 72 h incubation, culture supernatant was collected and stored in −80°C until use. 50 µl of supernatant was used in the assay as per the manufacturer recommendation. Results showed that rBmHAXT + AL007 and rBmHAXT + AL019 were better in the expression of both Th1/Th2 cytokines compared to rBmHAXT + mannosylated chitosan group. This showed that AL007 and AL019 can produce balanced Th1/Th2 cytokines response. *p < 0.05, #p < 0.01, **p < 0.001 compared to respective group, ns = not significant as analyzed by Kruskal-Wallis test followed by Bonferroni correction for multiple analysis.
TCM Cells Were Generated in the Spleen of rBmHAXT-Vaccinated Animals
Spleen cells were cultured at 37°C for 72 h, stimulated with 1 µg/ml of rBmHAXT protein. After 72 h, cells were harvested and stained with CD3/CD4/CD8 antibodies and evaluated in flow cytometer. There was a slight but significant increase in the CD8+ cell population in rBmHAXT + AL019-treated group (p ≤ 0.05) compared to the other groups (data not shown). To determine the percent of TCM cells in the spleen, splenocytes were stained with CD62L/CCR7 antibodies and analyzed in a flow cytometer. Cells that were dual positive for CD62L/CCR7 were considered as TCM cells. Our results showed that rBmHAXT-treated animals showed high percentage of TCM cells irrespective of the adjuvant used (p ≤ 0.001) (Figure 6A).
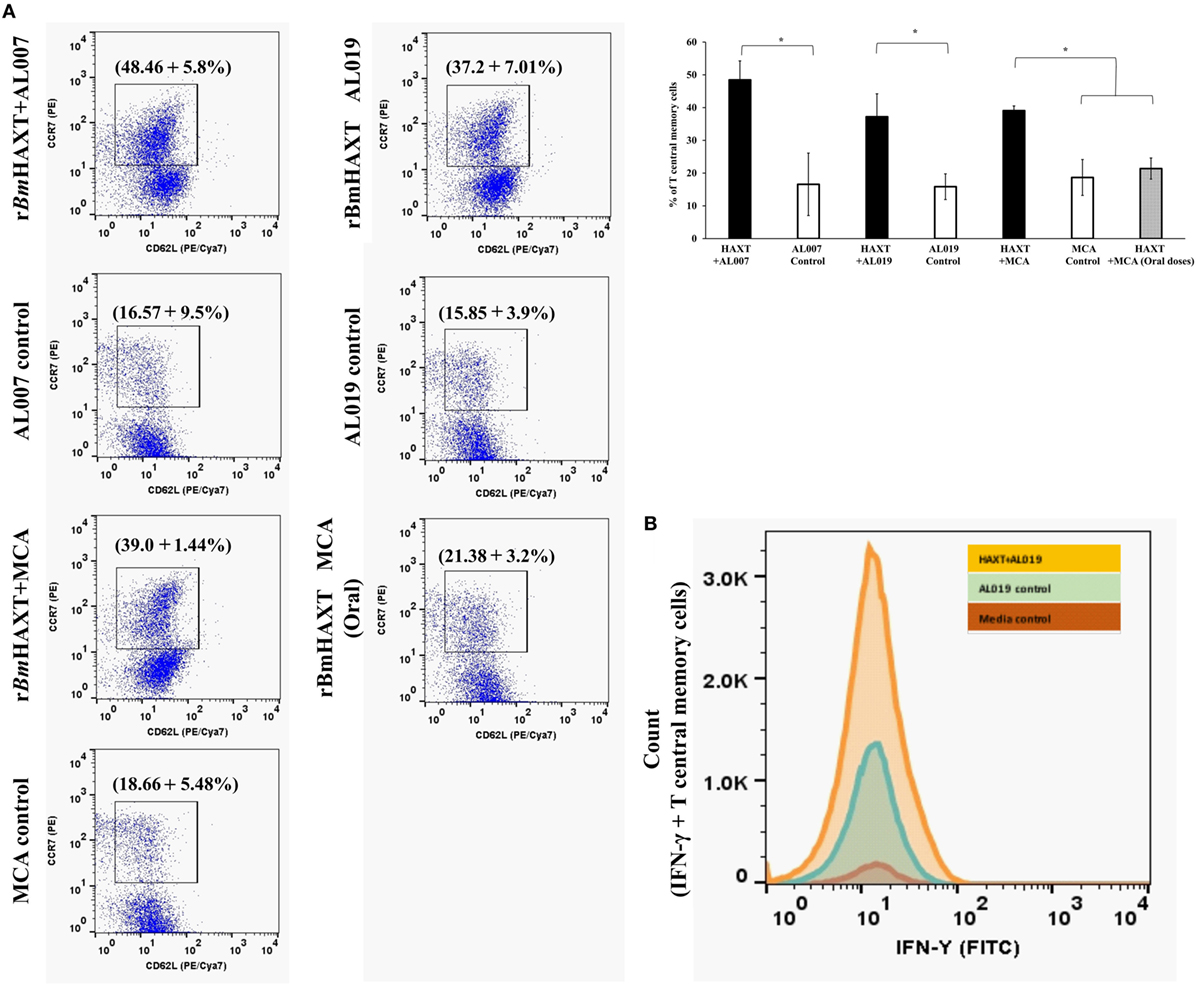
Figure 6. T-central memory cells and their IFN-γ secretion in the spleen of immunized mice. (A) Cultured spleen cells were stained with anti-mouse CCR7 (PE) and CD62L (PE/Cya7) antibodies. Cells which were dual positive for both the markers were selected as T central memory cells. Results showed that, T central memory cells were significantly high in rBmHAXT + AL007 compared to all the groups. Similarly, rBmHAXT + mannosylated chitosan (MCA) and rBmHAXT + AL019-treated mice also showed significant increase in T central memory cells. (B) Histogram showed that rBmHAXT + AL019 formulation-treated animals showed significantly increased expression of IFN-γ in T central memory cells. However, no significant difference was found in rBmHAXT plus Al007/MCA-treated groups with respect to their respective control group. *p < 0.05 compared to respective group as analyzed by Kruskal-Wallis test followed by Bonferroni correction for multiple analysis.
TCM Cells Were Predominantly IFNγ+
IFN-γ secreting TCM cells are believed to play a major role in vaccine-induced protection in parasitic infections (44). Therefore, we measured the percentage of CD62L+ CCR7+ TCM cells that expressed intracellular IFN-γ. Our results show that cells from rBmHAXT + AL019-vaccinated animals had the significantly (p < 0.01) high percentage of IFN-γ+ TCM cells compared to rBmHAXT + AL007 and rBmHAXT + MCA-vaccinated groups (Figure 6B).
Discussion
Results presented in this study show that both AL007 (alum) and AL019 (GLA + alum) are excellent adjuvants for rBmHAXT immunization in mice. Both the adjuvants elicited significant immunogenicity in vaccinated mice. However, the vaccine-induced protection was higher in animals immunized with rBmHAXT + AL019 suggesting that AL019 is probably a better adjuvant for rBmHAXT immunizations than alum or MCA.
Our previous studies with a trivalent vaccine (rBmHAT) given along with alum (AL007) as the adjuvant gave close to 95% protection in the mouse model (29–33), however, when we tested the formulation in macaques only modest protection (~35%) was achieved against B. malayi L3 challenge infections (34). This prompted us to search for an improved vaccine formulation that could be eventually used in macaques. Therefore, in this study we used the mouse model to initially evaluate and dissect out the immune responses. In an effort to improve the current trivalent vaccine, the first modification we made was to add another vaccine candidate thioredoxin peroxide (rBmTPX-2) to the trivalent formulation.
Thioredoxin peroxidase is a member of the peroxidoxin superfamily of proteins that play a key role in a variety of cellular processes, such as DNA synthesis, defense against oxidative stress, detoxification, protein folding, and repair and operate through redox cascades that involve transfer of reducing equivalents from NADPH to targets through reversible dithiol-disulfide reactions (45). TPX is expressed in several organisms, including helminths (46–52) and protozoan (53) parasites. One of the major functions of TPX-2 in parasites is to protect the parasites from damaging effects of host-generated oxidative stress by producing H2O2-detoxifying activity (50, 54). TPX-2 protein is predominantly localized to the surface of the helminth parasites mainly hypodermis and cuticle (50, 54). In Onchocerca volvulus the Ov-tpx-2 cDNA represents roughly 2.5% of the total cDNAs from the L3 cDNA library suggesting that these are important proteins for the parasite helping them to evade host defense mechanisms (51). Given its critical role for survival of the parasite in the host, TPX-2 is a potential target for vaccine development. Our group and others (24, 27, 28, 40–42) have previously reported the vaccine potential of TPX. Studies showed that rBmTRX could confer 62% protection against B. malayi challenge in Mastomys model (43) and 43–69.5% protection in mouse and jird models (27, 28).
We inserted the gene sequence of Bmtpx2 in between ALT-2 and TSP. This allowed us to use the same primers as the trivalent gene to amplify the tetravalent gene. Subsequent expression of the tetravalent vaccine protein (rBmHAXT) showed that we could get substantially higher and better expression of the tetravalent protein compared to our previous trivalent protein preparations. As demonstrated in Figure 1, we could get >98% pure final rBmHAXT vaccine protein in our studies.
Analysis of the type of immune responses generated following TPX-2 immunization suggested that predominantly an IFN-γ-mediated response is generated in the mouse model (24, 27, 28, 40–42). Our previous studies using the trivalent formulation (rBmHAT plus alum) in rhesus macaque showed that a Th2 biased response was predominant with a weak IFN-γ response, which we believed was the reason for poor vaccine-induced protection in macaques (34). Thus, in the current study we hypothesized that including BmTPX-2 as an additional vaccine antigen could improve the Th1 response after vaccination. Our results confirmed this notion. The tetravalent formulation was found to significantly increase the levels of IFN-γ-producing antigen-specific memory cells in vaccinated animals irrespective of the adjuvants tested. Further evidence comes from the analysis of the spleen cells, where the spleen cells from rBmHAXT-vaccinated animals were shown to secrete IL-4, IL-10, and IFN-γ in response to rBmHAXT suggesting the generation of a balanced Th1/Th2 response. There was also an increase in IL-17-secreting cells in rBmHAXT-vaccinated animals. IL-17 has been shown to be critical for promoting vaccine-induced protection in several systems (55–61).
One of our recent studies suggested that including GLA-SE/alum (a synthetic toll-like receptor 4 agonist on alhydrogel, AL019) as an adjuvant along with the trivalent formulation (rBmHAT) promoted a balanced Th1/Th2 response in the mouse model and gave better protection (30). GLA-SE is shown to be an excellent adjuvant for several human vaccine formulations (62, 63) and other helminth vaccine formulations (64). Therefore, in this study we evaluated if AL019 is a better adjuvant for the tetravalent (rBmHAXT) formulation. Our results showed that including AL019 as an adjuvant along with rBmHAXT gave significantly better protection (~88%) than alum as adjuvant that gave slightly less (79%) protection rate.
Similarly, we also tested another adjuvant formulation, MCA. One of our recent studies showed that MCA is a potential adjuvant for the trivalent (rBmHAT) formulation conferring close to 88% protection in mice (30). However, in this study when we used MCA as an adjuvant for the tetravalent formulation, the protection rate was slightly low (79% protection) but significant. One of the reasons for testing MCA as an adjuvant in this study is because of its potential to support the vaccine as an oral delivery platform, which may be advantageous for clinical use. Nevertheless, results from this study showed that MCA is as good as alum in generating the protective immune responses, especially when the first dose of MCA plus the vaccine antigen was given subcutaneously and the subsequent booster doses were given orally. However, when all the MCA plus vaccine antigen doses (prime and booster doses) were given orally, MCA was not as effective as an adjuvant for the rBmHAXT immunizations. Thus, our results showed that AL019 may be a better adjuvant for rBmHAXT vaccine formulation compared to alum and MCA.
Analysis of the titer of IgG antibodies in the serum and peritoneal fluids showed that alum and AL019 elicited the maximum titers of IgG antibodies compared to MCA adjuvant. Similarly, all three adjuvants elicited a balanced Th1/Th2 antibody response (IgG1, IgG2a, and IgG2b). These findings are similar to our previous report [30] and correlated well with the protective responses observed in this study. rBmHAXT vaccination did not elicit any IgE responses as was reported before for the rBmHAT vaccination in rhesus macaque (34) and mice (30). Surprisingly, oral delivery of vaccine antigen along with MCA (prime and booster doses) did not yield any antibody responses in the serum or peritoneal fluids suggesting that oral route alone may not be a viable vaccine delivery approach for rBmHAXT + MCA vaccination.
In conclusion, our present study show that AL019 is a better adjuvant formulation for rBmHAXT vaccination in the mouse model, based on the higher rate of protection and the strong immune responses generated following immunization. Further studies need to be performed in the non-human primate models with the tetravalent formulation plus AL019.
Ethics Statement
Use of mice and the experimental procedures performed in this study were reviewed and approved by the IACUC committee at the University of Illinois College of Medicine at Rockford.
Author Contributions
NC, VK, and RK planned the experiments. NC, VK, and RK interpreted the results and analyzed the data. NC, VK, and RK performed immunization and challenge experiments. NC, PB, and VK performed the flow cytometry and proliferation assays. VK performed the ADCC and MPO assays. NC, PB, and VK performed all the cell-based assays. All authors contributed to the writing of the manuscript.
Conflict of Interest Statement
The authors declare that the research was conducted in the absence of any commercial or financial relationships that could be construed as a potential conflict of interest.
Acknowledgments
This work was supported by the National Institutes of Health, National Institute of Allergy and Infectious Diseases grants R01AI072613 (RK). The infective larval stage (L3) of B. malayi was obtained from the NIH/NIAID Filariasis research reagent resource center, College of Veterinary Medicine, University of Georgia, Athens, GA under NIAID supply contract AI no. 30022.
References
1. Brady MGlobal Alliance to Eliminate Lymphatic Filariasis. Seventh meeting of the global alliance to eliminate lymphatic filariasis: reaching the vision by scaling up, scaling down, and reaching out. Parasit Vectors (2014) 7:46. doi:10.1186/1756-3305-7-46
2. Saxena A. Communicable Diseases of the Developing World. Topics in Medicinal Chemistry. Cham, Switzerland: Springer (2016). p. 97–124.
3. World Health Organization. Progress Report 2000-2009 and Strategic Plan 2010-2020 of the Global Programme to Eliminate Lymphatic Filariasis: Halfway Towards Eliminating Lymphatic Filariasis. (2010). Available from: http://whqlibdoc.who.int/publications/2010/9789241500722_eng.pdf (Accessed: January 19, 2018).
4. World Health Organization. Lymphatic Filariasis. Geneva, Switzerland: World Health Organization (2018). http://www.who.int/lymphatic_filariasis/elimination-programme/en (Accessed: February 15, 2018).
5. Rao RU, Samarasekera SD, Nagodavithana KC, Dassanayaka TDM, Punchihewa MW, Ranasinghe USB, et al. Reassessment of areas with persistent lymphatic filariasis nine years after cessation of mass drug administration in Sri Lanka. PLoS Negl Trop Dis (2017) 11(10):e0006066. doi:10.1371/journal.pntd.0006066
6. Ortu G, Williams O. Neglected tropical diseases: exploring long term practical approaches to achieve sustainable disease elimination and beyond. Infect Dis Poverty (2017) 6(1):147. doi:10.1186/s40249-017-0361-8
7. Stolk WA, Stone C, Vlas SJD. Modelling lymphatic filariasis transmission and control: modelling frameworks, lessons learned and future directions. Adv Parasitol (2015) 87:249–91. doi:10.1016/bs.apar.2014.12.005
8. Patel P. Mass drug administration coverage evaluation survey for lymphatic filariasis in Bagalkot and Gulbarga districts. Indian J Community Med (2012) 37(2):101. doi:10.4103/0970-0218.96095
9. Nujum Z, Rajmohanan K, Remadevi S, Indu P, Nair S, Nirmala C. Factors determining noncompliance to mass drug administration for lymphatic filariasis elimination. Trop Parasitol (2012) 2(2):109. doi:10.4103/2229-5070.105175
10. Nujum ZT. Coverage and compliance to mass drug administration for lymphatic filariasis elimination in a district of Kerala, India. Int Health (2011) 3(1):22–6. doi:10.1016/j.inhe.2010.12.001
11. Talbot JT, Viall A, Direny A, de Rochars MB, Addiss D, Streit T, et al. Predictors of compliance in mass drug administration for the treatment and prevention of lymphatic filariasis in Leogane, Haiti. Am J Trop Med Hyg (2008) 78(2):283–8. doi:10.4269/ajtmh.2008.78.283
12. Verma SK, Arora A, Murthy PK. Recombinant calponin of human filariid Brugia malayi: secondary structure and immunoprophylactic potential. Vaccine (2017) 35(38):5201–8. doi:10.1016/j.vaccine.2017.07.105
13. Kumar R, Doharey PK, Saxena JK, Rathaur S. Molecular cloning, purification and characterization of Brugia malayi phosphoglycerate kinase. Protein Expr Purif (2017) 132:152–63. doi:10.1016/j.pep.2017.02.005
14. Thirugnanam S, Pandiaraja P, Ramaswamy K, Murugan V, Gnanasekar M, Nandakumar K, et al. Brugia malayi: comparison of protective immune responses induced by Bm-alt-2 DNA, recombinant Bm-ALT-2 protein and prime-boost vaccine regimens in a jird model. Exp Parasitol (2007) 116(4):483–91. doi:10.1016/j.exppara.2007.02.017
15. Andure D, Pote K, Khatri V, Amdare N, Padalkar R, Reddy MVR. Immunization with Wuchereria bancrofti glutathione-s-transferase elicits a mixed th1/th2 type of protective immune response against filarial infection in Mastomys. Indian J Clin Biochem (2016) 31(4):423–30. doi:10.1007/s12291-016-0556-y
16. Singh PK, Kushwaha S, Rana AK, Misra-Bhattacharya S. Cofactor independent phosphoglycerate mutase of Brugia malayi induces a mixed th1/th2 type immune response and inhibits larval development in the host. Biomed Res Int (2014) 2014:1–19. doi:10.1155/2014/590281
17. Arunkumar C, Pandiaraja P, Prince P, Kaliraj P. Immunological characterization of recombinant Wuchereria bancrofti cuticular collagen (COL-4) as putative vaccine candidate for human lymphatic filariasis. Asian Pac J Trop Med (2014) 7(7):505–12. doi:10.1016/S1995-7645(14)60084-5
18. Arumugam S, Wei J, Ward D, Abraham D, Lustigman S, Zhan B, et al. Vaccination with a genetically modified Brugia malayi cysteine protease inhibitor-2 reduces adult parasite numbers and affects the fertility of female worms following a subcutaneous challenge of Mongolian gerbils (Meriones unguiculatus) with B. malayi infective larvae. Int J Parasitol (2014) 44(10):675–9. doi:10.1016/j.ijpara.2014.05.003
19. Veerapathran A, Dakshinamoorthy G, Gnanasekar M, Reddy MVR, Kalyanasundaram R. Evaluation of Wuchereria bancrofti GST as a vaccine candidate for lymphatic filariasis. PLoS Negl Trop Dis (2009) 3(6):e457. doi:10.1371/journal.pntd.0000457
20. Kushwaha S, Singh PK, Rana AK, Misra-Bhattacharya S. Immunization of Mastomys coucha with Brugia malayi recombinant trehalose-6-phosphate phosphatase results in significant protection against homologous challenge infection. PLoS One (2013) 8(8):e72585. doi:10.1371/journal.pone.0072585
21. Gregory WF, Atmadja AK, Allen JE, Maizels RM. The abundant larval transcript-1 and -2 genes of Brugia malayi encode stage-specific candidate vaccine antigens for filariasis. Infect Immun (2000) 68(7):4174–9. doi:10.1128/IAI.68.7.4174-4179.2000
22. Dakshinamoorthy G, Samykutty AK, Munirathinam G, Shinde GB, Nutman T, Reddy MV, et al. Biochemical characterization and evaluation of a Brugia malayi small heat shock protein as a vaccine against lymphatic filariasis. PLoS One (2012) 7(4):e34077. doi:10.1371/journal.pone.0034077
23. Dakshinamoorthy G, Munirathinam G, Stoicescu K, Reddy MV, Kalyanasundaram R. Large extracellular loop of tetraspanin as a potential vaccine candidate for filariasis. PLoS One (2013) 8(10):e77394. doi:10.1371/journal.pone.0077394
24. Anugraha G, Jeyaprita P, Madhumathi J, Sheeba T, Kaliraj P. Immune responses of B. malayi thioredoxin (TRX) and venom allergen homologue (VAH) chimeric multiple antigen for lymphatic filariasis. Acta Parasitol (2013) 58(4):468–77. doi:10.2478/s11686-013-0160-8
25. Kalyanasundaram R, Balumuri P. Multivalent vaccine formulation with BmVAL-1 and BmALT-2 confer significant protection against challenge infections with Brugia malayi in mice and jirds. Res Rep Trop Med (2011) 2011(2):45–56. doi:10.2147/RRTM.S13679
26. Anand S, Kodumudi K, Reddy M, Kaliraj P. A combination of two Brugia malayi filarial vaccine candidate antigens (BmALT-2 and BmVAH) enhances immune responses and protection in jirds. J Helminthol (2011) 85(2):442–52. doi:10.1017/S0022149X10000799
27. Vanam U, Pandey V, Prabhu PR, Dakshinamurthy G, Reddy MVR, Kaliraj P. Evaluation of immunoprophylactic efficacy of Brugia malayi transglutaminase (BmTGA) in single and multiple antigen vaccination with BmALT-2 and BmTPX for human lymphatic filariasis. Am J Trop Med Hyg (2009) 80(2):319–24. doi:10.4269/ajtmh.2009.80.319
28. Anand SB, Murugan V, Prabhu PR, Anandharaman V, Reddy MVR, Kaliraj P. Comparison of immunogenicity, protective efficacy of single and cocktail DNA vaccine of Brugia malayi abundant larval transcript (ALT-2) and thioredoxin peroxidase (TPX) in mice. Acta Trop (2008) 107(2):106–12. doi:10.1016/j.actatropica.2008.04.018
29. Dakshinamoorthy G, Samykutty AK, Munirathinam G, Reddy MV, Kalyanasundaram R. Multivalent fusion protein vaccine for lymphatic filariasis. Vaccine (2013) 31(12):1616–22. doi:10.1016/j.vaccine.2012.09.055
30. Chauhan N, Banerjee P, Khatri VK, Canciamille A, Gilles J, Kalyanasundaram R. Improving the efficacy of a prophylactic vaccine formulation against lymphatic filariasis. Parasitol Res (2017) 116(10):2821–30. doi:10.1007/s00436-017-5593-9
31. Dakshinamoorthy G, Kalyanasundaram R. Evaluating the efficacy of rBmHATαc as a multivalent vaccine against lymphatic filariasis in experimental animals and optimizing the adjuvant formulation. Vaccine (2013) 32(1):19–25. doi:10.1016/j.vaccine.2013.10.083
32. Samykutty A, Dakshinamoorthy G, Kalyanasundaram R. Multivalent vaccine for lymphatic filariasis. Procedia Vaccinol (2010) 3:12–8. doi:10.1016/j.provac.2010.11.003
33. Joseph SK, Ramaswamy K. Single multivalent vaccination boosted by trickle larval infection confers protection against experimental lymphatic filariasis. Vaccine (2013) 31:3320–6. doi:10.1016/j.vaccine.2013.05.077
34. Dakshinamoorthy G, Gegerfelt AV, Andersen H, Lewis M, Kalyanasundaram R. Evaluation of a multivalent vaccine against lymphatic filariasis in rhesus macaque model. PLoS One (2014) 9(11):e112982. doi:10.1371/journal.pone.0112982
35. Dimock KA, Eberhard ML, Lammie PJ. Th1-like antifilarial immune responses predominate in antigen-negative persons. Infect Immun (1996) 64(8):2962–7.
36. Giambartolomei GH, Lasater BL, Villinger FÃ, Dennis VA. Diminished production of T helper 1 cytokines and lack of induction of IL-2R+ T cells correlate with T-cell unresponsiveness in rhesus monkeys chronically infected with Brugia malayi. Exp Parasitol (1998) 90(1):77–85. doi:10.1006/expr.1998.4312
37. Li B-W, Wang Z, Rush AC, Mitreva M, Weil GJ. Transcription profiling reveals stage- and function-dependent expression patterns in the filarial nematode Brugia malayi. BMC Genomics (2012) 13(1):184. doi:10.1186/1471-2164-13-184
38. Wongkamchai S, Chiangjong W, Sinchaikul S, Chen S-T, Choochote W, Thongboonkerd V. Identification of Brugia malayi immunogens by an immunoproteomics approach. J Proteomics (2011) 74(9):1607–13. doi:10.1016/j.jprot.2011.06.012
39. Hewitson JP, Harcus YM, Curwen RS, Dowle AA, Atmadja AK, Ashton PD, et al. The secretome of the filarial parasite, Brugia malayi: proteomic profile of adult excretory-secretory products. Mol Biochem Parasitol (2008) 160(1):8–21. doi:10.1016/j.molbiopara.2008.02.007
40. Madhumathi J, Prince PR, Anugraha G, Kiran P, Rao DN, Reddy MVR, et al. Identification and characterization of nematode specific protective epitopes of Brugia malayi TRX towards development of synthetic vaccine construct for lymphatic filariasis. Vaccine (2010) 28(31):5038–48. doi:10.1016/j.vaccine.2010.05.012
41. Anand SB, Rajagopal V, Kaliraj P. Brugia malayi thioredoxin peroxidase as a potential vaccine candidate antigen for lymphatic filariasis. Appl Biochem Biotechnol (2012) 167(5):1351–64. doi:10.1007/s12010-012-9643-6
42. Madhumathi J, Anugraha G, Prince PR, Pradiba D, Kaliraj P. Proliferative responses of Brugia malayi TPX-1 and its epitopic peptide 29-43 in an endemic population of human lymphatic filariasis. Microbes Infect (2011) 13(6):602–6. doi:10.1016/j.micinf.2011.01.008
43. Prince PR, Madhumathi J, Anugraha G, Jeyaprita P, Reddy M, Kaliraj P. Tandem antioxidant enzymes confer synergistic protective responses in experimental filariasis. J Helminthol (2013) 88(4):402–10. doi:10.1017/S0022149X13000333
44. Maggioli MF, Palmer MV, Thacker TC, Vordermeier HM, McGill JL, Whelan AO, et al. Increased TNF-α/IFN-γ/IL-2 and decreased TNF-α/IFN-γ production by central memory T cells are associated with protective responses against bovine tuberculosis following BCG vaccination. Front Immunol (2016) 7:421. doi:10.3389/fimmu.2016.00421
45. Williams DL, Bonilla M, Gladyshev VN, Salinas G. Thioredoxin glutathione reductase-dependent redox networks in platyhelminth parasites. Antioxid Redox Signal (2013) 19(7):735–45. doi:10.1089/ars.2012.4670
46. Donnelly S, O’neill SM, Sekiya M, Mulcahy G, Dalton JP. Thioredoxin peroxidase secreted by Fasciola hepatica induces the alternative activation of macrophages. Infect Immun (2005) 73(1):166–73. doi:10.1128/IAI.73.1.166-173.2005
47. Ghosh I, Eisinger SW, Raghavan N, Scott AL. Thioredoxin peroxidases from Brugia malayi. Mol Biochem Parasitol (1998) 91:207–20. doi:10.1016/S0166-6851(97)00213-2
48. Klimowski L, Chandrashekar R, Tripp CA. Molecular cloning, expression and enzymatic activity of a thioredoxin peroxidase from Dirofilaria. Mol Biochem Parasitol (1997) 90(1):297–306. doi:10.1016/S0166-6851(97)00167-9
49. Li J, Zhang W-B, Loukas A, Lin R-Y, Ito A, Zhang L-H, et al. Functional expression and characterization of Echinococcus granulosus thioredoxin peroxidase suggests a role in protection against oxidative damage. Gene (2004) 326:157–65. doi:10.1016/j.gene.2004.07.034
50. Chiumiento L, Bruschi F. Enzymatic antioxidant systems in helminth parasites. Parasitol Res (2009) 105(3):593–603. doi:10.1007/s00436-009-1483-0
51. Lu W, Egerton GL, Bianco AE, Williams SA. Thioredoxin peroxidase from Onchocerca volvulus: a major hydrogen peroxide detoxifying enzyme in filarial parasites. Mol Biochem Parasitol (1998) 91(2):221–35. doi:10.1016/S0166-6851(97)00230-2
52. Kunchithapautham K, Padmavathi B, Narayanan RB, Kaliraj P, Scott AL. Thioredoxin from Brugia malayi: defining a 16-kilodalton class of thioredoxins from nematodes. Infect Immun (2003) 71(7):4119–26. doi:10.1128/IAI.71.7.4119-4126.2003
53. Usui M, Masuda-Suganuma H, Fukumoto S, Angeles JMM, Hakimi H, Inoue N, et al. Effect of thioredoxin peroxidase-1 gene disruption on the liver stages of the rodent malaria parasite Plasmodium berghei. Parasitol Int (2015) 64(3):290–4. doi:10.1016/j.parint.2014.09.013
54. Gretes MC, Poole LB, Karplus PA. Peroxiredoxins in parasites. Antioxid Redox Signal (2012) 17(4):608–33. doi:10.1089/ars.2011.4404
55. Anthony RM, Rutitzky LI, Urban JF, Stadecker MJ, Gause WC. Protective immune mechanisms in helminth infection. Nat Rev Immunol (2007) 7(12):975–87. doi:10.1038/nri2199
56. Priebe GP, Walsh RL, Cederroth TA, Kamei A, Coutinho-Sledge YS, Goldberg JB, et al. IL-17 is a critical component of vaccine-induced protection against lung infection by lipopolysaccharide-heterologous strains of Pseudomonas aeruginosa. J Immunol (2008) 181(7):4965–75. doi:10.4049/jimmunol.181.7.4965
57. Lin Y, Slight SR, Khader SA. Th17 cytokines and vaccine-induced immunity. Semin Immunopathol (2010) 32(1):79–90. doi:10.1007/s00281-009-0191-2
58. Kumar P, Chen K, Kolls JK. Th17 cell based vaccines in mucosal immunity. Curr Opin Immunol (2013) 25(3):373–80. doi:10.1016/j.coi.2013.03.011
59. Dann SM, Manthey CF, Le C, Miyamoto Y, Gima L, Abrahim A, et al. IL-17A promotes protective IgA responses and expression of other potential effectors against the lumen-dwelling enteric parasite Giardia. Exp Parasitol (2015) 156:68–78. doi:10.1016/j.exppara.2015.06.003
60. Cai CW, Blase JR, Zhang X, Eickhoff CS, Hoft DF. Th17 cells are more protective than th1 cells against the intracellular parasite Trypanosoma cruzi. PLoS Pathog (2016) 12(10):e1005902. doi:10.1371/journal.ppat.1005902
61. Maizels RM, Mcsorley HJ. Regulation of the host immune system by helminth parasites. J Allergy Clin Immunol (2016) 138(3):666–75. doi:10.1016/j.jaci.2016.07.007
62. Heeke DS, Lin R, Rao E, Woo JC, Mccarthy MP, Marshall JD. Identification of GLA/SE as an effective adjuvant for the induction of robust humoral and cell-mediated immune responses to EBV-gp350 in mice and rabbits. Vaccine (2016) 34(23):2562–9. doi:10.1016/j.vaccine.2016.04.012
63. Arias MA, Roey GAV, Tregoning JS, Moutaftsi M, Coler RN, Windish HP, et al. Glucopyranosyl lipid adjuvant (GLA), a synthetic TLR4 agonist, promotes potent systemic and mucosal responses to intranasal immunization with HIVgp140. PLoS One (2012) 7(7):e41144. doi:10.1371/journal.pone.0041144
Keywords: lymphatic filariasis, TLR-4, prophylactic vaccine, aluminum, multivalent antigen, mouse model
Citation: Chauhan N, Khatri V, Banerjee P and Kalyanasundaram R (2018) Evaluating the Vaccine Potential of a Tetravalent Fusion Protein (rBmHAXT) Vaccine Antigen Against Lymphatic Filariasis in a Mouse Model. Front. Immunol. 9:1520. doi: 10.3389/fimmu.2018.01520
Received: 25 April 2018; Accepted: 19 June 2018;
Published: 02 July 2018
Edited by:
Rashika El Ridi, Cairo University, EgyptReviewed by:
P. Kalpana Murthy, Council of Scientific and Industrial Research (CSIR), IndiaSamar Nagah El-Beshbishi, Mansoura University, Egypt
Copyright: © 2018 Chauhan, Khatri, Banerjee and Kalyanasundaram. This is an open-access article distributed under the terms of the Creative Commons Attribution License (CC BY). The use, distribution or reproduction in other forums is permitted, provided the original author(s) and the copyright owner are credited and that the original publication in this journal is cited, in accordance with accepted academic practice. No use, distribution or reproduction is permitted which does not comply with these terms.
*Correspondence: Ramaswamy Kalyanasundaram, cmFtc3dhbXkmI3gwMDA0MDt1aWMuZWR1
†These authors have contributed equally to this work.