- 1HKU Pasteur Research Pole, The University of Hong Kong, Pokfulam, Hong Kong
- 2WHO Collaborating Centre for Infectious Disease Epidemiology and Control, School of Public Health, The University of Hong Kong, Pokfulam, Hong Kong
Influenza viruses circulate worldwide causing annual epidemics that have a substantial impact on public health. This is despite vaccines being in use for over 70 years and currently being administered to around 500 million people each year. Improvements in vaccine design are needed to increase the strength, breadth, and duration of immunity against diverse strains that circulate during regular epidemics, occasional pandemics, and from animal reservoirs. Universal vaccine strategies that target more conserved regions of the virus, such as the hemagglutinin (HA)-stalk, or recruit other cellular responses, such as T cells and NK cells, have the potential to provide broader immunity. Many pre-pandemic vaccines in clinical development do not utilize new vaccine platforms but use “tried and true” recombinant HA protein or inactivated virus strategies despite substantial leaps in fundamental research on universal vaccines. Significant hurdles exist for universal vaccine development from bench to bedside, so that promising preclinical data is not yet translating to human clinical trials. Few studies have assessed immune correlates derived from asymptomatic influenza virus infections, due to the scale of a study required to identity these cases. The realization and implementation of a universal influenza vaccine requires identification and standardization of set points of protective immune correlates, and consideration of dosage schedule to maximize vaccine uptake.
Introduction
Influenza A viruses have over 18 different hemagglutinin (HA) subtypes, and continual antigenic drift of seasonal H3N2 and H1N1 viruses generates new variants. In addition, there are distinct lineages of influenza B viruses that also exhibit antigenic drift, meaning there is a plethora of influenza viruses that pose a threat to public health (1). Reports of global influenza infection rates estimate that up to 18% of the population can be infected during annual influenza epidemics (2), causing excess morbidity and mortality resulting in projected economic losses of nearly US$87 billion (3). Influenza vaccines are the most widely used vaccines in the world due to annual updates on circulating strains and health authority recommendations for at risk groups (4). The groups most commonly targeted for influenza vaccination programs are children and elderly, pregnant women, immunocompromised, and healthcare workers (HCWs). Inactivated influenza vaccines (IIV) administered intramuscularly have been available since the 1940s and progressive developments have been made to increase breadth of immunity provided by the vaccine, from monovalent to bivalent and then trivalent, to most recently quadrivalent formulations (5). The use of split and subunit vaccines has provided a more purified formulation, and the use of improved adjuvants with reduced side effects in recent years, such as MF59 and AS03 has enabled antigen sparing and increased immunogenicity of vaccine antigens (5, 6). One important advance was the release of live-attenuated influenza vaccines (LAIV) by MedImmune to American markets in 2003, delivered as a nasal spray (7). The quadrivalent cell-grown recombinant HA protein vaccines, FluCelVax (Seqirus) available from 2012 (8) and FluBlok (Sanofi Pasteur) available from 2013 (9), provide an expedient pipeline for pandemic vaccine responsiveness and avoid egg adaptations generated during vaccine production.
A combination of issues exists for the current influenza vaccines (10), including egg adaptations (11), lag between strain selection and vaccine availability (10), and breadth and duration of immunity (12). Annual vaccine effectiveness (VE) is variable and contingent upon antigenic distance between vaccine and circulating strains and the individual’s immune history (13). Shortcomings in VE for IIV and LAIV are repeatedly reported with a recent average VE found to be 78.4% and 30.7% against H1N1pdm09 infections, respectively, in 2- to 17-year olds in 2015/2016 in the UK, US, Canada, and Finland (14), while IIV VE reported by the CDC ranges from 10 to 60% from 2004 to 2016 (15). Therefore, current vaccines are not effective enough, with negative or low VE reported, and LAIV does not appear to improve upon VE over IIV consistently (16–18), hence, the need for universal vaccines. Furthermore, current IIV VE decreased over time by one-third from 3 to 6 months post vaccination (19), and reduced VE estimates over time were seen for LAIV (20, 21). Targeting the elderly for vaccination is a logical step as they are the demographic that have the highest morbidity and mortality risk from an influenza virus infection; however, current vaccines are even less effective at conferring protection within this susceptible age group (22).
Criteria for Design of Next-Generation Universal Influenza Vaccines
WHO published in 2017 the Preferred Product Characteristics for Next-Generation Influenza Vaccines which lays out the targets for influenza vaccine development over the next 5 and 10 years (23). In the first 5 years, the WHO encourages the evaluation of currently available vaccine and vaccine technologies to achieve greater protection against vaccine-matched or drifted influenza strains and protection against severe influenza for at least 1 year. In 10 years, by 2027, the WHO encourages research and development in next-generation vaccines to provide universal protection against severe influenza A illness for at least 5 years. In addition, a strategic work plan for the design of a universal vaccine has been outlined by the NIH NIAID (10, 24, 25). To achieve the goal of a universal influenza vaccine capable of providing protection beyond 1 year and with broader immunity against antigenically diverse strains, the work plan identified areas for expanded research efforts to address this goal, with an emphasis on research in the areas of (1) influenza transmission, natural history, and pathogenesis; (2) development of influenza immunity and correlates of protection; and (3) rational design of universal influenza vaccines.
Ultimately, an ideal universal influenza vaccine would provide protection (1) against seasonal influenza epidemics by drift variants between seasons or pan-influenza A and B viruses, (2) against influenza pandemics with limited prior population immunity, and (3) against zoonotic (e.g., avian) influenza infections with severe disease outcomes. However, this “ideal” vaccine is still stuck at the laboratory bench (26), with fundamental questions about immune correlates of protection required for universal protection against influenza viruses yet to be answered.
Strategies to Increase the Strength, Duration, and Breadth of Vaccine-Induced Immune Responses
Timing of Priming for T Cell Immunity
Existing inactivated virus-based vaccine technologies could be improved to increase the strength and duration of the vaccine-induced responses to overcome seasonal influenza epidemics of drifted variants. While natural infection may generate protective immunity for 2–10 years (2, 27), it has been reported that IIV sero-protection fell below 60% one year after vaccination (28). Therefore, bridging the gap between protection afforded by infection and vaccination, by defining immune correlates of protection (Table 1) associated with better outcomes of natural infection, severity of infection, and the protection afforded by current and other next-generation vaccines in clinical development (Table 2) is critical to future vaccine design.
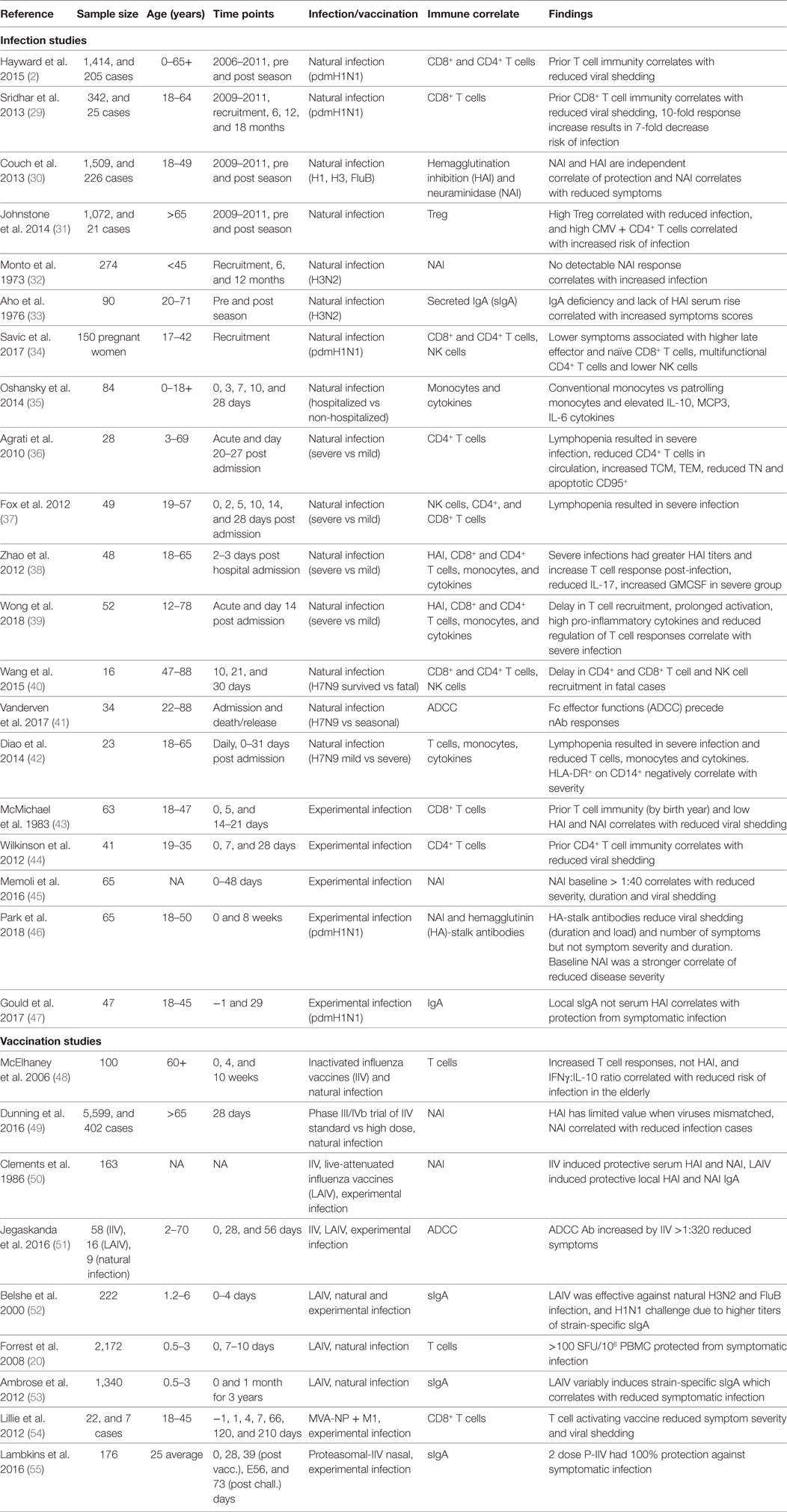
Table 1. Broadly reactive correlates of protection from symptomatic influenza virus infection from human studies.
Live-attenuated influenza vaccines have been shown to induce T cell responses in children but not adults (58), and in particular children under 10 years of age have greater T cell boosting (59). In mice, early-priming preserves optimal influenza-specific CD8+ T cell function and diversity and protects against age-related immune decline (60), with similar age-associated effects of VE observed for LAIV (20). Furthermore, the thymus involutes during puberty greatly reducing naïve T cell output, while “inflammaging” impacts T cell priming (61). In another study, memory CD8+ T cells have been observed and stable longitudinally for over more than a 10-year period, most likely due to multiple re-infections since childhood (27). It is likely that repeated boosting of T cell immunity to conserved, immunodominant epitopes of NP and M1 antigens (62) results in long-term maintenance of T cell responses associated with protection from symptomatic infection (2). In older adults, individuals who received 3–4 years of annual repeated IIV vaccination, rather than single vaccination, had higher response magnitude, long-term durability, and multifunctional quality cross-reactive memory CD4+ T cells (63). Indeed, the T cell-based modified vaccinia virus Ankara (MVA) vector expressing NP + M1 influenza vaccine (MVA-NP + M1) could boost antigen-specific T cell memory responses in adults over 65 years of age (64). The promising MVA-NP + M1, which is currently in phase II clinical trials (Table 2), has been proposed to be used in conjunction with current IIV (65) and shown to broaden both humoral and cellular immunity. Therefore, a window for priming optimal T cell immunity with longevity exists and could be considered for vaccine design to maintain effective T cell immunity.
In humans, the lungs are enriched with CD8+ T resident memory (TRM) that rapidly generate effector cytokines upon influenza infection (66). Furthermore, prime-pull strategies have been tested in mouse studies to seed local TRM responses (67), whereby a vaccine is given first parentally, i.e., intramuscularly like traditional vaccination route, to prime the T cell responses; and then inflammatory or secondary vaccine is given locally, i.e., intranasally, to pull responses to the lung, but this has had limited effect in the lung where cognate antigen presentation is required to maintain TRM (68).
However, the protective efficacy of localized TRM responses vs. circulating responses can only be tested in animal models. A 7-month limitation of protection has been identified by lung-resident TRM in mouse models (69), but may not reflect the decay of human peripheral T cell memory in labeling and tracking studies (70). Whether lung TRM have a similar critical role in modulating disease outcome in humans is unknown, but it is essential to be understood for optimal vaccine design.
Stalling of the HA-Stalk
Development of next-generation vaccines that provide broader immune responses will be needed to protect against influenza pandemics and zoonotic influenza infections. A consensus on immune arms which are capable of providing broader immunity are split over a dichotomy, which are focused on either the anti-HA-stalk antibodies or T cell immunity. Theoretically, a HA-stalk vaccine has an exciting and promising potential, with subtype specific, multigroup, and even pan-influenza A and B antibodies being identified (71). Impressive in vitro and animal studies have shown the breadth of HA-stalk antibodies, yet passive transfer in human clinical trials have shown high concentrations are needed but with little therapeutic effect (72). While HA-stalk antibodies have been found to be enriched in some individuals infected with the 2009 H1N1 pandemic virus, these antibodies are notoriously low in frequency with universal antibodies such as F10 representing only 0.001% of circulating antibodies (73). There is a paucity of data on the protective role of HA-stalk antibodies in human infection studies (46) (Table 1). A human challenge study found that higher baseline level hemagglutination inhibition (HAI) antibodies were accompanied by increased HA-stalk-specific antibodies and reduced viral shedding but not symptom severity, while anti-neuraminidase (NAI) antibodies were the strongest correlate of protection (CoP) for symptomatic infection (46). Therefore, the independent role of HA-stalk antibodies remains to be defined separately from HAI and NAI antibodies. Ultimately, harnessing HA-stalk-specific B cells capable of universal immunity may also require repeated boosting to overcome immune waning, which limits the duration of current IIV.
Alternative Strategies in Development
There are a large number of universal vaccine strategies in development in animal models, and only 61 in phase I and 189 in phase II clinical trials (Table 2) which are designed for pandemic potential viruses (56), and in total only 12.8% of these are designed to be effectively T cell stimulating (74). Apart from HA, additional viral proteins including the NP, NA, M1, and M2, are proposed to be possible targets for universal vaccines (75). Cross-reactive antibodies against these viral proteins from different subtypes have been identified and they are shown to have heterosubtypic protective effects in animals and humans. Various strategies using recombinant proteins/peptides, recombinant DNA, recombinant RNA, virus-like particles, viral vectors, and synthetic viruses for inducing heterosubtypic protective effects have been reported. Some of these approaches do not only aim at inducing broadly reactive antibodies but also cross-reactive T cell immunity against influenza infections. Clinical trials of experimental vaccines, such as proteasomal adjuvanted IIV by nasal delivery and MVA-NP + M1 have been assessed by experimental challenge and immune correlates of protection evaluated (Table 1). Previous reports also show many experimental vaccines are not undergoing clinical trials or approved for human use, suggesting a bottleneck to preclinical development (76), which could be attributed to limitations of some animal models to show vaccine efficacy or support needed from industry funding for increasing scale of clinical studies (Table 2).
Hurdles in Extending Experimental Findings to Community: Identification of Immune Correlates of Protection
Correlating Immune Responses to Infection and Illness Severity
Hemagglutination inhibition and single radial hemolysis assays are the only accepted serological methods used both in the US and Europe for accelerated licensure of seasonal IIV and only recognized immune CoPs for influenza currently (77–79). Other candidates of CoPs (Table 1) have been evaluated against experimental or natural human influenza virus infections and vaccine efficacy studies. The route of vaccination (intramuscular or intranasal) determines systemic vs. local immunity, and variability in sampling techniques at the mucosa may hinder the precise evaluation of mucosal antibody responses (53). Furthermore, different CoPs may be identified depending on the outcome measure that is used across the spectrum of severity, for example, from asymptomatic infection to severe illness leading to hospitalization. Therefore, the context under which each CoP was determined should be considered, and a comprehensive analytical approach is needed for clinical studies (80).
Cellular immunity is important for protection from clinical disease. For example, the Flu Watch study highlighted NP-specific CD4+ and CD8+ T cells correlated with lower nasal viral shedding (2). Other studies identified dysregulation of cytokines (namely, IL-10, MCP3, and IL-6) (35) and reduced cellular responses (including T, NK, and MAIT cells) (39, 40, 42) are associated with severe disease. The baseline presence and increasing titer of secreted IgA (33, 47) and NAI (30, 32, 45, 46) have also been identified across studies and appear as more effective correlates of protection from symptomatic infection than HAI. In addition, reports are emerging that HA-stalk (46) and antibody dependent cellular cytotoxicity (ADCC)-activating antibodies (51) have been associated with reduced viral titers upon infection. de Vries et al. showed HA-stalk-specific ADCC responses were boosted in children post-infection (81), while H7-cross-reactive ADCC antibodies were cumulative and detectable from 2 years of age but plateauing by 17 years of age (82). Early exposures to influenza boost ADCC antibodies, while older adults have limited rises in ADCC antibodies post-infection (83). A titer of HA-specific ADCC antibodies >320 correlated with reduced risk of infection, symptom scores, and viral shedding in a human challenge study (51).
While a HAI titer of 40 is believed to provide 50% protection from symptomatic infection (84), new thresholds are being defined for T cell immunity. IIV does not effectively boost T cell immunity, hence the need for new universal vaccines. The longevity derived from memory generated by natural infection is also limited, with repeated infection during our lifetime, estimated every 2–10 years (2, 27). Therefore, universal vaccines will need to do better than nature to provide longer duration immunity from symptomatic infection. From community cohort studies with baseline samples prior to symptomatic infection, Hayward et al. (2) defined the protective threshold for symptomatic infection as >20 SFU/106 PBMCs (by stimulation with overlapping peptides for NP/M1); and from a LAIV children cohort study Forrest et al. (20) defined protective T cell threshold for symptomatic influenza infection as evaluated by ELISPOT was >100 SFU/106 PBMCs. Inactivated vaccination from the study by Koutsakos et al. resulted in a modest boost of influenza-specific CD4+ T cells, while CD8+ T cells were not boosted (85). While CD4+ T follicular helper cells (Tfh), correlate with greater antibody production and HAI titers (86), and are therefore important for current IIV efficacy. Future universal vaccines need to overcome limited immunogenicity of inactivated and LAIV vaccines by more immunogenic vaccine vectors (74). While universal vaccines, such as MVA-NP + M1, which uses systemic vaccination of a one-step replication vector encoding conserved NP and M1 proteins, boosted influenza-specific CD8+ T cells in adults over >65 years of age (64), a notoriously difficult population for increased cellular immunity. Therefore, universal vaccines in development already show improved ability to establish T cell memory.
The immune correlates of protection from influenza are mostly derived from the comparison of infected subjects on a spectrum of severity (Table 1). However, there is a difference between correlates of protection against all infection vs. correlates of protection against symptomatic infection. Furthermore, studies of current IIV for boosting of T cell responses as correlates of protection are not ideal as IIV is not designed to stimulate cellular immunity and can impinge the cellular immunity that is being developed during natural infection (87). Rather, studies of uninfected but exposed and asymptomatic cases (low or no viral shedding) from naturally acquired infection could define immune correlates on a larger scale than possible with human challenge studies (Table 1) (2, 29, 30).
Limitations by Prior Immunity
Prior immunity may impact vaccine efficacy, original antigenic sin, and similarly “HA-imprinting” may skew antibody and CD4+ T cell helper profiles by the viral subtype in the first exposure (88, 89). Furthermore, the level of neutralizing antibodies in a population will affect influenza transmission, and Bolton et al. proposed that T cell activating vaccines will have different efficacy depending on the population’s prior immunity (90). For example, due to prior immunity to seasonal H3N2 viruses but not to avian H7N9 viruses, a T cell-activating vaccine would be more efficacious for H7N9 viruses. Vaccinating an immune population with biased prior immunity may reduce vaccine efficacy, and universal vaccine strategies may differ by age group due to HA imprinting and immunosenescence. Therefore, use of a universal vaccine in younger demographics could exploit immunological imprinting to their advantage. Previously, high-antibody titers generated from childhood influenza infections which were maintained have been seen to be cross-reactive to antigenically drifted strains (91, 92).
On the other hand, seasonal influenza vaccination history may not always play a positive role in heterologous protection against subsequent influenza infection. Bodewes et al. (87) have compared the influenza A virus-specific cellular and humoral responses between 14 annually immunized children with cystic fibrosis and 27 unvaccinated healthy control children during winter season 2009–2010. A similar level of influenza-specific CD4+ T cell responses and neutralizing antibody titers were found between vaccinated and unvaccinated groups of children, but an age-dependent increase in the frequency of virus-specific CD8+ T cells were only observed in unvaccinated children. These findings indicated repeated annual influenza vaccinations might hamper the development of influenza A virus-specific CD8+ T cell immunity. One report in mice recently from Rowell et al. (93) also addressed such issue, which presented varied heterologous protection from a candidate universal influenza vaccine (A/NP + M2-rAd) following a history of conventional IIV vaccination. Interestingly, they found that humoral and cellular responses induced by universal vaccine could be enhanced, inhibited, or unaffected by selected prior vaccinations, and such variations may be affected by many factors including vaccine preparation and specific vaccine components.
Standardization of Assays and Findings Across Studies
Community cohort studies to identify natural influenza virus infections and measure immunity are established in the UK (2), US (94), Vietnam (95), Hong Kong (96, 97), China (98), and Nicaragua (99) with recruitment and experiments ongoing, making this area of research an exciting area to watch. The seasonality of influenza and year-to-year variation in infectivity of viruses requires these studies to span multiple years to generate robust data, for example, the Flu Watch study spanned 2006–2011 to capture 205 infections from baseline responses (2). Peripheral blood sampling will continue to be a proxy for cellular immune correlates for influenza virus infection, and simplified and standardized assays for immune signatures or biomarkers may aid future vaccine trials (Table 2).
One of the challenges in conducting studies to identify new correlates of protection is the sample size required. Typical community-based studies can follow up more than a thousand people over multiple years (2, 30, 97), measuring immune status before the season as baseline immunity and then identifying infections after influenza activity. Dunning et al. commented that data from 1,000 to 2,000 persons may be needed for a reasonably precise estimate of an influenza CoP (49). However, such sample size is logistically challenging, and the size scale of existing studies ranged from 16 to 226 infected individuals to stratify cases by severity to derive immune correlates (Table 1), and community cohort studies such as those by Sridhar et al. (25 cases from 342 participants) (29), Hayward et al. (205 cases from 1,414 participants) (2), and Couch et al. (226 cases from 1,509 participants) (30). The scale of vaccine efficacy trials precludes many vaccine studies, especially considering the need to show an improved standard of care from current IIV, which can be reasonable when well-matched viruses are in circulation but are limited for novel viruses.
The French Interior Milieu project (100) has provided baseline immune responses of 1,000 individuals over 2 time-points, sampled the individual’s genetic background, skin biopsy, nasal swab, urine, and fecal samples, and uses 10 unique panels by flow cytometry, and 40 stimuli for characterizing adaptive and innate cellular responses. The panels measure in parallel innate cells and adaptive cells, including innate lymphoid cells, NK cells, mucosal associated invariant T cells, dendritic cells, neutrophils, B cells, and T cells (1, 2, 17, reg). Stimulation determines the individual’s ability to respond to viral, microbial, agonists, and ligands, such as influenza and Sendai viruses, Helicobacter pylori, Poly I:C, Flagellin, TNFα, and CD3 + CD28 (101). The implementation of standardized assays, such as the stimulation of PBMCs with influenza viruses directly at blood collection by TruCulture tubes, was essential for multicenter experimental success (102). However, due to the use of an archetypal and outdated laboratory strain, A/Puerto Rico 8/1934, the results in regard to determining relevant baseline influenza virus-specific immunity were obsolete. The study protocols from the Interior Milieu project are now being extrapolated to other ethnicities and countries to provide a spectrum of a “healthy” baseline immune system and may provide a model for assays on a larger scale beyond HAI needed for universal vaccine design. The feasibility and scale of larger community cohort studies is beyond the capacity of a single research group for processing, storage, and experimental measures (80, 103) and needs commercial partners. Therefore, consensus and synergy with other established cohorts and network design to share expertise is essential to get past the bench to define quantifiable thresholds of immune correlates of protection.
Maximizing the Use and Effectiveness of Influenza Vaccines in the Community
Once a vaccine has been licensed to be truly effective within a population a certain coverage threshold must be reached. In 2009, the European Centre for Disease Prevention and Control set out to achieve 75% influenza vaccination coverage in the elderly and those suffering from chronic medical conditions by the winter season 2014/15. However, this target was only reached by one EU Member State in the 2013/14 season and during the 2014/15 influenza season no Member States were able to reach this coverage rate (104). Whereas the vaccination coverage rate for adults aged 18-64 years is even lower, reaching only 36.7% during 2013–2014 in the US (105). This demonstrates that current approach to vaccination, in the case of influenza, is insufficient and even with the development of novel vaccines, strategies for their implementation needs to be carefully considered.
Considerations and Strategies to Increase Accessibility and Uptake
The live-attenuated influenza vaccine only represents 8% of the vaccine market share (106), and production in the US has been threatened by low VE in recent years. Other enhanced influenza vaccines, such as QIV Fluzone by intradermal vaccination (107), have also been threatened by a dwindling market share. Combination and heterologous approaches may complicate adherence to vaccine schedules. Various methods have been developed to stimulate HA-stalk antibodies, such as HA-headless or chimeric HA, and combination strategies of prime boost for four doses (108). However, anti-HA-stalk antibody-stimulating vaccine regimes by heterologous prime boost used in mouse studies to elicit HA-stalk antibody responses may not be feasible in practice in the community, with each regime requiring separate licensure and multiple doses reducing vaccine adherence. The human papilloma virus (HPV) and hepatitis B virus (HBV) vaccines both require a homologous 3-dose regime within 2 years for optimal sero-protection, and HBV also requires a 10-year dose booster. Adherence to HPV vaccine 3-dose schedule is only 28% (109), and similarly 29% for HBV vaccination (110). A comprehensive vaccination record system will be instrumental for orderly vaccination schedules.
An increasingly difficult barrier to successful vaccination strategies is “vaccine hesitancy.” The WHO Strategic Advisory Group of Experts (SAGE) on Immunization has defined vaccine hesitancy as a “delay in acceptance or refusal of vaccination despite availability of vaccination services” (111). Vaccine hesitancy can develop into refusal and the encouragement of others to refuse vaccination, leading to unvaccinated clusters within a community and severe public health consequences. One of the more concerning effects of vaccine hesitancy is the effect on vaccination coverage in HCWs. Vaccination for HCWs is recommended in most countries but mandatory vaccination programs vary. A survey of HCWs in China found a coverage rate of only 9.5% in the seasons of 2009/2010 to 2011/2012 (112). However, vaccination in HCWs in the US has increased since the 2010/2011 season, reaching 64.8% in 2014/2015 (113), demonstrating this issue varies greatly by country due to policy decisions and cultural factors. If HCWs themselves are hesitant about current vaccines, novel vaccines that utilize “non-traditional” approaches for universal immunity may require extensive explanation and promotion to HCWs to encourage self-vaccination and increase vaccine recommendations to patients.
Indirect Protection in the Community With Vaccine Uptake
Many studies have shown that increasing vaccination uptake in children and younger adults reduces influenza burden in older adults (114–116, 117). Older children and adolescents have been shown to be the key age groups affecting the initial spread of influenza infections within a community (118). Elderly individuals often come into contact with children and young adults in household and urban settings, public areas and transportation. One of the clearest examples of this was seen in Japan, when influenza vaccination of school children ceased in 1994, leading to an increase in elderly mortality rates (119). A study analyzing US vaccine data also found that in areas where there was ≥31% vaccine coverage in younger adults, the elderly had a 20.6% lower chance of being diagnosed with influenza than in areas with a ≤15% coverage rate (120). Vaccination of healthy children could also form the basis of establishing early T cell memory and broader HA imprinting from an immunological perspective.
Conclusion
Universal influenza vaccine research is a growing trend (Figure 1), with first reports in the 1970s of heterologous immunity in the absence of antibodies for recombinant vaccines being developed following the antigenic switch from H1N1 to H3N2 viruses (121). A large increase in the universal vaccine research field has been seen since 2003, coinciding with zoonotic infections from avian and equine sources and pandemic viruses becoming a real threat to public health. Therefore, the drive for increased breadth of coverage for influenza vaccine has been a long-term objective, and the recent NIAID push has been a “call to arms” to address this issue. An increasing number of immune biomarkers that are associated with protection against influenza virus infection and disease severity in vitro and in vivo have been identified, leading to vaccines designed to elicit these immune markers at different stages of clinical trials. Such a strategy assumes that these markers are correlates of protection in humans, but whether such assumptions hold is yet to be confirmed in large epidemiological studies. Ultimately, immune correlates should be compared in parallel and defined within a weighted hierarchy to drive vaccine design which can stimulate multiple immune arms effectively. Alternatively, despite measurable influenza-specific T and B cell immunity, all healthy adults experience repeat infections in their lifetime. Additionally the WHO goals to promote longevity of responses may also require a vaccine that elicits a “better than nature” response. With increased attention and funding for this area, particularly from the National Institutes of Health, there is real hope for the successful development of universal influenza vaccines.
Author Contributions
SV, NL, MB, YLM, AL, LP and BC wrote and prepared the review. SV and NL prepared the figures and tables.
Conflict of Interest Statement
BC has received research funding from Sanofi Pasteur, and honoraria from Sanofi Pasteur and Roche. The authors report no other potential conflicts of interest.
Funding
This work was supported in part by the NIAID Centers of Excellence for Influenza Research and Surveillance (CEIRS Contract No. HHSN272201400006C), the Theme-based Research Scheme (TRS) from the Research Grants Council of the Hong Kong Special Administrative Region, China (Project No. T11-705/14N), and Health and Medical Research Fund (Project No. 14130672).
References
1. Fauci AS. Pandemic influenza threat and preparedness. Emerg Infect Dis (2006) 12(1):73–7. doi:10.3201/eid1201.050983
2. Hayward AC, Wang L, Goonetilleke N, Fragaszy EB, Bermingham A, Copas A, et al. Natural T cell-mediated protection against seasonal and pandemic influenza. Results of the Flu Watch Cohort Study. Am J Respir Crit Care Med (2015) 191(12):1422–31. doi:10.1164/rccm.201411-1988OC
3. Molinari NA, Ortega-Sanchez IR, Messonnier ML, Thompson WW, Wortley PM, Weintraub E, et al. The annual impact of seasonal influenza in the US: measuring disease burden and costs. Vaccine (2007) 25(27):5086–96. doi:10.1016/j.vaccine.2007.03.046
4. Palache A, Abelin A, Hollingsworth R, Cracknell W, Jacobs C, Tsai T, et al. Survey of distribution of seasonal influenza vaccine doses in 201 countries (2004-2015): the 2003 World Health Assembly resolution on seasonal influenza vaccination coverage and the 2009 influenza pandemic have had very little impact on improving influenza control and pandemic preparedness. Vaccine (2017) 35(36):4681–6. doi:10.1016/j.vaccine.2017.07.053
5. Wong SS, Webby RJ. Traditional and new influenza vaccines. Clin Microbiol Rev (2013) 26(3):476–92. doi:10.1128/CMR.00097-12
6. Del Giudice G, Rappuoli R. Inactivated and adjuvanted influenza vaccines. Curr Top Microbiol Immunol (2015) 386:151–80. doi:10.1007/82_2014_406
7. Jin H, Subbarao K. Live attenuated influenza vaccine. Curr Top Microbiol Immunol (2015) 386:181–204. doi:10.1007/82_2014_410
8. Manini I, Domnich A, Amicizia D, Rossi S, Pozzi T, Gasparini R, et al. Flucelvax (Optaflu) for seasonal influenza. Expert Rev Vaccines (2015) 14(6):789–804. doi:10.1586/14760584.2015.1039520
9. Dunkle LM, Izikson R, Patriarca P, Goldenthal KL, Muse D, Callahan J, et al. Efficacy of recombinant influenza vaccine in adults 50 years of age or older. N Engl J Med (2017) 376(25):2427–36. doi:10.1056/NEJMoa1608862
10. Paules CI, Sullivan SG, Subbarao K, Fauci AS. Chasing seasonal influenza – the need for a universal influenza vaccine. N Engl J Med (2018) 378(1):7–9. doi:10.1056/NEJMp1714916
11. Manini I, Trombetta CM, Lazzeri G, Pozzi T, Rossi S, Montomoli E. Egg-independent influenza vaccines and vaccine candidates. Vaccines (Basel) (2017) 5(3):E18. doi:10.3390/vaccines5030018
12. Xu C, Thompson MG, Cowling BJ. Influenza vaccination in tropical and subtropical areas. Lancet Respir Med (2017) 5(12):920–2. doi:10.1016/S2213-2600(17)30377-6
13. Skowronski DM, Chambers C, Sabaiduc S, De Serres G, Winter AL, Dickinson JA, et al. Beyond antigenic match: possible agent-host and immuno-epidemiological influences on influenza vaccine effectiveness during the 2015-2016 season in Canada. J Infect Dis (2017) 216(12):1487–500. doi:10.1093/infdis/jix526
14. Pebody R, McMenamin J, Nohynek H. Live attenuated influenza vaccine (LAIV): recent effectiveness results from the USA and implications for LAIV programmes elsewhere. Arch Dis Child (2018) 103(1):101–5. doi:10.1136/archdischild-2016-312165
15. CDC. Seasonal Influenza Vaccine Effectiveness, 2005-2018 [Online]. (2018). Available from: https://www.cdc.gov/flu/professionals/vaccination/effectiveness-studies.htm (Accessed: February 24, 2018).
16. Caspard H, Mallory RM, Yu J, Ambrose CS. Live-attenuated influenza vaccine effectiveness in children from 2009 to 2015–2016: a systematic review and meta-analysis. Open Forum Infect Dis (2017) 4(3):ofx111. doi:10.1093/ofid/ofx111
17. McLean HQ, Caspard H, Griffin MR, Poehling KA, Gaglani M, Belongia EA, et al. Effectiveness of live attenuated influenza vaccine and inactivated influenza vaccine in children during the 2014-2015 season. Vaccine (2017) 35(20):2685–93. doi:10.1016/j.vaccine.2017.03.085
18. Poehling KA, Caspard H, Peters TR, Belongia EA, Congeni B, Gaglani M, et al. 2015-2016 vaccine effectiveness of live attenuated and inactivated influenza vaccines in children in the United States. Clin Infect Dis (2018) 66(5):665–72. doi:10.1093/cid/cix869
19. Young B, Sadarangani S, Jiang L, Wilder-Smith A, Chen MI. Duration of influenza vaccine effectiveness: a systematic review, meta-analysis, and meta-regression of test-negative design case-control studies. J Infect Dis (2018) 217(5):731–41. doi:10.1093/infdis/jix632
20. Forrest BD, Pride MW, Dunning AJ, Capeding MR, Chotpitayasunondh T, Tam JS, et al. Correlation of cellular immune responses with protection against culture-confirmed influenza virus in young children. Clin Vaccine Immunol (2008) 15(7):1042–53. doi:10.1128/CVI.00397-07
21. Petrie JG, Ohmit SE, Truscon R, Johnson E, Braun TM, Levine MZ, et al. Modest waning of influenza vaccine efficacy and antibody titers during the 2007–2008 influenza season. J Infect Dis (2016) 214(8):1142–9. doi:10.1093/infdis/jiw105
22. Beyer WE, McElhaney J, Smith DJ, Monto AS, Nguyen-Van-Tam JS, Osterhaus AD. Cochrane re-arranged: support for policies to vaccinate elderly people against influenza. Vaccine (2013) 31(50):6030–3. doi:10.1016/j.vaccine.2013.09.063
23. Ortiz JR, Hickling J, Jones R, Donabedian A, Engelhardt OG, Katz JM, et al. Report on eighth WHO meeting on development of influenza vaccines that induce broadly protective and long-lasting immune responses: Chicago, USA, 23-24 August 2016. Vaccine (2018) 36(7):932–8. doi:10.1016/j.vaccine.2017.11.061
24. Paules CI, Marston HD, Eisinger RW, Baltimore D, Fauci AS. The pathway to a universal influenza vaccine. Immunity (2017) 47(4):599–603. doi:10.1016/j.immuni.2017.09.007
25. Erbelding EJ, Post D, Stemmy E, Roberts PC, Augustine AD, Ferguson S, et al. A universal influenza vaccine: the strategic plan for the National Institute of Allergy and Infectious Diseases. J Infect Dis (2018). doi:10.1093/infdis/jiy103
26. Rajao DS, Perez DR. Universal vaccines and vaccine platforms to protect against influenza viruses in humans and agriculture. Front Microbiol (2018) 9:123. doi:10.3389/fmicb.2018.00123
27. van de Sandt CE, Hillaire ML, Geelhoed-Mieras MM, Osterhaus AD, Fouchier RA, Rimmelzwaan GF. Human influenza A virus-specific CD8+ T cell response is long-lived. J Infect Dis (2015) 212(1):81–5. doi:10.1093/infdis/jiv018
28. Young B, Zhao X, Cook AR, Parry CM, Wilder-Smith A, MC IC. Do antibody responses to the influenza vaccine persist year-round in the elderly? A systematic review and meta-analysis. Vaccine (2017) 35(2):212–21. doi:10.1016/j.vaccine.2016.11.013
29. Sridhar S, Begom S, Bermingham A, Hoschler K, Adamson W, Carman W, et al. Cellular immune correlates of protection against symptomatic pandemic influenza. Nat Med (2013) 19(10):1305–12. doi:10.1038/nm.3350
30. Couch RB, Atmar RL, Franco LM, Quarles JM, Wells J, Arden N, et al. Antibody correlates and predictors of immunity to naturally occurring influenza in humans and the importance of antibody to the neuraminidase. J Infect Dis (2013) 207(6):974–81. doi:10.1093/infdis/jis935
31. Johnstone J, Parsons R, Botelho F, Millar J, McNeil S, Fulop T, et al. Immune biomarkers predictive of respiratory viral infection in elderly nursing home residents. PLoS One (2014) 9(9):e108481. doi:10.1371/journal.pone.0108481
32. Monto AS, Kendal AP. Effect of neuraminidase antibody on Hong Kong influenza. Lancet (1973) 1(7804):623–5. doi:10.1016/S0140-6736(73)92196-X
33. Aho K, Pyhala R, Koistinen J. IgA deficiency and influenza infection. Scand J Immunol (1976) 5(10):1089–92. doi:10.1111/j.1365-3083.1976.tb00247.x
34. Savic M, Dembinski JL, Laake I, Hungnes O, Cox R, Oftung F, et al. Distinct T and NK cell populations may serve as immune correlates of protection against symptomatic pandemic influenza A(H1N1) virus infection during pregnancy. PLoS One (2017) 12(11):e0188055. doi:10.1371/journal.pone.0188055
35. Oshansky CM, Gartland AJ, Wong SS, Jeevan T, Wang D, Roddam PL, et al. Mucosal immune responses predict clinical outcomes during influenza infection independently of age and viral load. Am J Respir Crit Care Med (2014) 189(4):449–62. doi:10.1164/rccm.201309-1616OC
36. Agrati C, Gioia C, Lalle E, Cimini E, Castilletti C, Armignacco O, et al. Association of profoundly impaired immune competence in H1N1v-infected patients with a severe or fatal clinical course. J Infect Dis (2010) 202(5):681–9. doi:10.1086/655469
37. Fox A, Le NM, Horby P, van Doorn HR, Nguyen VT, Nguyen HH, et al. Severe pandemic H1N1 2009 infection is associated with transient NK and T deficiency and aberrant CD8 responses. PLoS One (2012) 7(2):e31535. doi:10.1371/journal.pone.0031535
38. Zhao Y, Zhang YH, Denney L, Young D, Powell TJ, Peng YC, et al. High levels of virus-specific CD4+ T cells predict severe pandemic influenza A virus infection. Am J Respir Crit Care Med (2012) 186(12):1292–7. doi:10.1164/rccm.201207-1245OC
39. Wong SS, Oshansky CM, Guo XJ, Ralston J, Wood T, Seeds R, et al. Severe influenza is characterized by prolonged immune activation: results from the SHIVERS Cohort Study. J Infect Dis (2018) 217(2):245–56. doi:10.1093/infdis/jix571
40. Wang Z, Wan Y, Qiu C, Quinones-Parra S, Zhu Z, Loh L, et al. Recovery from severe H7N9 disease is associated with diverse response mechanisms dominated by CD8(+) T cells. Nat Commun (2015) 6:6833. doi:10.1038/ncomms7833
41. Vanderven HA, Liu L, Ana-Sosa-Batiz F, Nguyen TH, Wan Y, Wines B, et al. Fc functional antibodies in humans with severe H7N9 and seasonal influenza. JCI Insight (2017) 2(13):92750. doi:10.1172/jci.insight.92750
42. Diao H, Cui G, Wei Y, Chen J, Zuo J, Cao H, et al. Severe H7N9 infection is associated with decreased antigen-presenting capacity of CD14+ cells. PLoS One (2014) 9(3):e92823. doi:10.1371/journal.pone.0092823
43. McMichael AJ, Gotch FM, Noble GR, Beare PA. Cytotoxic T-cell immunity to influenza. N Engl J Med (1983) 309(1):13–7. doi:10.1056/NEJM198307073090103
44. Wilkinson TM, Li CK, Chui CS, Huang AK, Perkins M, Liebner JC, et al. Preexisting influenza-specific CD4+ T cells correlate with disease protection against influenza challenge in humans. Nat Med (2012) 18(2):274–80. doi:10.1038/nm.2612
45. Memoli MJ, Shaw PA, Han A, Czajkowski L, Reed S, Athota R, et al. Evaluation of antihemagglutinin and antineuraminidase antibodies as correlates of protection in an influenza A/H1N1 virus healthy human challenge model. MBio (2016) 7(2):e417–416. doi:10.1128/mBio.00417-16
46. Park JK, Han A, Czajkowski L, Reed S, Athota R, Bristol T, et al. Evaluation of preexisting anti-hemagglutinin stalk antibody as a correlate of protection in a healthy volunteer challenge with influenza A/H1N1pdm virus. MBio (2018) 9(1):e02284-17. doi:10.1128/mBio.02284-17
47. Gould VMW, Francis JN, Anderson KJ, Georges B, Cope AV, Tregoning JS. Nasal IgA provides protection against human influenza challenge in volunteers with low serum influenza antibody titre. Front Microbiol (2017) 8:900. doi:10.3389/fmicb.2017.00900
48. McElhaney JE, Xie D, Hager WD, Barry MB, Wang Y, Kleppinger A, et al. T cell responses are better correlates of vaccine protection in the elderly. J Immunol (2006) 176(10):6333–9. doi:10.4049/jimmunol.176.10.6333
49. Dunning AJ, DiazGranados CA, Voloshen T, Hu B, Landolfi VA, Talbot HK. Correlates of protection against influenza in the elderly: results from an influenza vaccine efficacy trial. Clin Vaccine Immunol (2016) 23(3):228–35. doi:10.1128/CVI.00604-15
50. Clements ML, Betts RF, Tierney EL, Murphy BR. Serum and nasal wash antibodies associated with resistance to experimental challenge with influenza A wild-type virus. J Clin Microbiol (1986) 24(1):157–60.
51. Jegaskanda S, Luke C, Hickman HD, Sangster MY, Wieland-Alter WF, McBride JM, et al. Generation and protective ability of influenza virus-specific antibody-dependent cellular cytotoxicity in humans elicited by vaccination, natural infection, and experimental challenge. J Infect Dis (2016) 214(6):945–52. doi:10.1093/infdis/jiw262
52. Belshe RB, Gruber WC, Mendelman PM, Mehta HB, Mahmood K, Reisinger K, et al. Correlates of immune protection induced by live, attenuated, cold-adapted, trivalent, intranasal influenza virus vaccine. J Infect Dis (2000) 181(3):1133–7. doi:10.1086/315323
53. Ambrose CS, Wu X, Jones T, Mallory RM. The role of nasal IgA in children vaccinated with live attenuated influenza vaccine. Vaccine (2012) 30(48):6794–801. doi:10.1016/j.vaccine.2012.09.018
54. Lillie PJ, Berthoud TK, Powell TJ, Lambe T, Mullarkey C, Spencer AJ, et al. Preliminary assessment of the efficacy of a T-cell-based influenza vaccine, MVA-NP+M1, in humans. Clin Infect Dis (2012) 55(1):19–25. doi:10.1093/cid/cis327
55. Lambkin-Williams R, Gelder C, Broughton R, Mallett CP, Gilbert AS, Mann A, et al. An intranasal proteosome-adjuvanted trivalent influenza vaccine is safe, immunogenic & efficacious in the human viral influenza challenge model. Serum IgG & mucosal IgA are important correlates of protection against illness associated with infection. PLoS One (2016) 11(12):e0163089. doi:10.1371/journal.pone.0163089
56. WHO. Tables on Clinical Evaluation of Influenza Vaccines. (2017). Available from: http://www.who.int/immunization/diseases/influenza/clinical_evaluation_tables/en/
57. NIH, Clinical trials. (2018). Available from: ClinicalTrials.gov (Accessed: April 24, 2018).
58. Hoft DF, Babusis E, Worku S, Spencer CT, Lottenbach K, Truscott SM, et al. Live and inactivated influenza vaccines induce similar humoral responses, but only live vaccines induce diverse T-cell responses in young children. J Infect Dis (2011) 204(6):845–53. doi:10.1093/infdis/jir436
59. Mohn KGI, Zhou F, Brokstad KA, Sridhar S, Cox RJ. Boosting of cross-reactive and protection-associated T cells in children after live attenuated influenza vaccination. J Infect Dis (2017) 215(10):1527–35. doi:10.1093/infdis/jix165
60. Valkenburg SA, Venturi V, Dang TH, Bird NL, Doherty PC, Turner SJ, et al. Early priming minimizes the age-related immune compromise of CD8(+) T cell diversity and function. PLoS Pathog (2012) 8(2):e1002544. doi:10.1371/annotation/e142f9de-7f30-4759-bda1-a651e86d5ba6
61. Kedzierska K, Valkenburg SA, Doherty PC, Davenport MP, Venturi V. Use it or lose it: establishment and persistence of T cell memory. Front Immunol (2012) 3:357. doi:10.3389/fimmu.2012.00357
62. Lee LY, Ha, do LA, Simmons C, de Jong MD, Chau NV, et al. Memory T cells established by seasonal human influenza A infection cross-react with avian influenza A (H5N1) in healthy individuals. J Clin Invest (2008) 118(10):3478–90. doi:10.1172/JCI32460
63. Trieu MC, Zhou F, Lartey S, Jul-Larsen A, Mjaaland S, Sridhar S, et al. Long-term maintenance of the influenza-specific cross-reactive memory CD4+ T-cell responses following repeated annual influenza vaccination. J Infect Dis (2017) 215(5):740–9. doi:10.1093/infdis/jiw619
64. Antrobus RD, Lillie PJ, Berthoud TK, Spencer AJ, McLaren JE, Ladell K, et al. A T cell-inducing influenza vaccine for the elderly: safety and immunogenicity of MVA-NP+M1 in adults aged over 50 years. PLoS One (2012) 7(10):e48322. doi:10.1371/journal.pone.0048322
65. Antrobus RD, Berthoud TK, Mullarkey CE, Hoschler K, Coughlan L, Zambon M, et al. Coadministration of seasonal influenza vaccine and MVA-NP+M1 simultaneously achieves potent humoral and cell-mediated responses. Mol Ther (2014) 22(1):233–8. doi:10.1038/mt.2013.162
66. Pizzolla A, Nguyen TH, Sant S, Jaffar J, Loudovaris T, Mannering SI, et al. Influenza-specific lung-resident memory T cells are proliferative and polyfunctional and maintain diverse TCR profiles. J Clin Invest (2018) 128(2):721–33. doi:10.1172/JCI96957
67. Shin H, Iwasaki A. A vaccine strategy that protects against genital herpes by establishing local memory T cells. Nature (2012) 491(7424):463–7. doi:10.1038/nature11522
68. Gilchuk P, Hill TM, Guy C, McMaster SR, Boyd KL, Rabacal WA, et al. A distinct lung-interstitium-resident memory CD8(+) T cell subset confers enhanced protection to lower respiratory tract infection. Cell Rep (2016) 16(7):1800–9. doi:10.1016/j.celrep.2016.07.037
69. Wu T, Hu Y, Lee YT, Bouchard KR, Benechet A, Khanna K, et al. Lung-resident memory CD8 T cells (TRM) are indispensable for optimal cross-protection against pulmonary virus infection. J Leukoc Biol (2014) 95(2):215–24. doi:10.1189/jlb.0313180
70. Akondy RS, Fitch M, Edupuganti S, Yang S, Kissick HT, Li KW, et al. Origin and differentiation of human memory CD8 T cells after vaccination. Nature (2017) 552(7685):362–7. doi:10.1038/nature24633
71. Corti D, Lanzavecchia A. Broadly neutralizing antiviral antibodies. Annu Rev Immunol (2013) 31:705–42. doi:10.1146/annurev-immunol-032712-095916
72. Sparrow E, Friede M, Sheikh M, Torvaldsen S, Newall AT. Passive immunization for influenza through antibody therapies, a review of the pipeline, challenges and potential applications. Vaccine (2016) 34(45):5442–8. doi:10.1016/j.vaccine.2016.08.057
73. Sui J, Sheehan J, Hwang WC, Bankston LA, Burchett SK, Huang CY, et al. Wide prevalence of heterosubtypic broadly neutralizing human anti-influenza A antibodies. Clin Infect Dis (2011) 52(8):1003–9. doi:10.1093/cid/cir121
74. Clemens EB, van de Sandt C, Wong SS, Wakim LM, Valkenburg SA. Harnessing the power of T cells: the promising hope for a universal influenza vaccine. Vaccines (Basel) (2018) 6(2). doi:10.3390/vaccines6020018
75. Kumar A, Meldgaard TS, Bertholet S. Novel platforms for the development of a universal influenza Vaccine. Front Immunol (2018) 9:600. doi:10.3389/fimmu.2018.00600
76. Lambert LC, Fauci AS. Influenza vaccines for the future. N Engl J Med (2010) 363(21):2036–44. doi:10.1056/NEJMra1002842
77. Russell SM, McCahon D, Beare AS. A single radial haemolysis technique for the measurement of influenza antibody. J Gen Virol (1975) 27(1):1–10. doi:10.1099/0022-1317-27-1-1
78. Trombetta CM, Montomoli E. Influenza immunology evaluation and correlates of protection: a focus on vaccines. Expert Rev Vaccines (2016) 15(8):967–76. doi:10.1586/14760584.2016.1164046
79. Weir JP, Gruber MF. An overview of the regulation of influenza vaccines in the United States. Influenza Other Respir Viruses (2016) 10(5):354–60. doi:10.1111/irv.12383
80. Ramilo O, Mejias A. It is time for a comprehensive analytical approach in clinical studies. Virus-versus-host interaction and influenza disease severity. Am J Respir Crit Care Med (2014) 189(4):385–6. doi:10.1164/rccm.201401-0008ED
81. de Vries RD, Nieuwkoop NJ, Pronk M, de Bruin E, Leroux-Roels G, Huijskens EGW, et al. Influenza virus-specific antibody dependent cellular cytoxicity induced by vaccination or natural infection. Vaccine (2017) 35(2):238–47. doi:10.1016/j.vaccine.2016.11.082
82. Terajima M, Co MD, Cruz J, Ennis FA. High antibody-dependent cellular cytotoxicity antibody titers to H5N1 and H7N9 Avian influenza A viruses in healthy US adults and older children. J Infect Dis (2015) 212(7):1052–60. doi:10.1093/infdis/jiv181
83. Valkenburg SA, Zhang Y, Chan KY, Leung K, Wu JT, Poon LL. Preexisting antibody-dependent cellular cytotoxicity-activating antibody responses are stable longitudinally and cross-reactive responses are not boosted by recent influenza exposure. J Infect Dis (2016) 214(8):1159–63. doi:10.1093/infdis/jiw346
84. Hobson D, Curry RL, Beare AS, Ward-Gardner A. The role of serum haemagglutination-inhibiting antibody in protection against challenge infection with influenza A2 and B viruses. J Hyg (Lond) (1972) 70(4):767–77. doi:10.1017/S0022172400022610
85. Koutsakos M, Wheatley AK, Loh L, Clemens EB, Sant S, Nussing S, et al. Circulating TFH cells, serological memory, and tissue compartmentalization shape human influenza-specific B cell immunity. Sci Transl Med (2018) 10(428):eaan8405. doi:10.1126/scitranslmed.aan8405
86. Bentebibel SE, Lopez S, Obermoser G, Schmitt N, Mueller C, Harrod C, et al. Induction of ICOS+CXCR3+CXCR5+ TH cells correlates with antibody responses to influenza vaccination. Sci Transl Med (2013) 5(176):176ra132. doi:10.1126/scitranslmed.3005191
87. Bodewes R, Fraaij PL, Geelhoed-Mieras MM, van Baalen CA, Tiddens HA, van Rossum AM, et al. Annual vaccination against influenza virus hampers development of virus-specific CD8(+) T cell immunity in children. J Virol (2011) 85(22):11995–2000. doi:10.1128/JVI.05213-11
88. Nayak JL, Richards KA, Yang H, Treanor JJ, Sant AJ. Effect of influenza A(H5N1) vaccine prepandemic priming on CD4+ T-cell responses. J Infect Dis (2015) 211(9):1408–17. doi:10.1093/infdis/jiu616
89. Gostic KM, Ambrose M, Worobey M, Lloyd-Smith JO. Potent protection against H5N1 and H7N9 influenza via childhood hemagglutinin imprinting. Science (2016) 354(6313):722–6. doi:10.1126/science.aag1322
90. Bolton KJ, McCaw JM, Brown L, Jackson D, Kedzierska K, McVernon J. Prior population immunity reduces the expected impact of CTL-inducing vaccines for pandemic influenza control. PLoS One (2015) 10(3):e0120138. doi:10.1371/journal.pone.0120138
91. Cobey S, Hensley SE. Immune history and influenza virus susceptibility. Curr Opin Virol (2017) 22:105–11. doi:10.1016/j.coviro.2016.12.004
92. Cobey S, Gouma S, Parkhouse K, Chambers BS, Ertl HC, Schmader KE, et al. Poor immunogenicity, not vaccine strain egg adaptation, may explain the low H3N2 influenza vaccine effectiveness in 2012-13. Clin Infect Dis (2018). doi:10.1093/cid/ciy097
93. Rowell J, Lo CY, Price GE, Misplon JA, Epstein SL, Garcia M. Conventional influenza vaccines influence the performance of a universal influenza vaccine in mice. Vaccine (2018) 36(7):1008–15. doi:10.1016/j.vaccine.2017.11.065
94. Monto AS, Malosh RE, Petrie JG, Thompson MG, Ohmit SE. Frequency of acute respiratory illnesses and circulation of respiratory viruses in households with children over 3 surveillance seasons. J Infect Dis (2014) 210(11):1792–9. doi:10.1093/infdis/jiu327
95. Nguyen DNT, Mai LQ, Bryant JE, Hang NLK, Hoa LNM, Nadjm B, et al. Epidemiology and etiology of influenza-like-illness in households in Vietnam; it’s not all about the kids! J Clin Virol (2016) 82:126–32. doi:10.1016/j.jcv.2016.07.014
96. Cowling BJ, Ng S, Ma ES, Cheng CK, Wai W, Fang VJ, et al. Protective efficacy of seasonal influenza vaccination against seasonal and pandemic influenza virus infection during 2009 in Hong Kong. Clin Infect Dis (2010) 51(12):1370–9. doi:10.1086/657311
97. Cowling BJ, Perera RA, Fang VJ, Chan KH, Wai W, So HC, et al. Incidence of influenza virus infections in children in Hong Kong in a 3-year randomized placebo-controlled vaccine study, 2009-2012. Clin Infect Dis (2014) 59(4):517–24. doi:10.1093/cid/ciu356
98. Cowling BJ, Xu C, Tang F, Zhang J, Shen J, Havers F, et al. Cohort profile: the China Ageing REespiratory infections Study (CARES), a prospective cohort study in older adults in Eastern China. BMJ Open (2017) 7(10):e017503. doi:10.1136/bmjopen-2017-017503
99. Gordon A, Kuan G, Aviles W, Sanchez N, Ojeda S, Lopez B, et al. The Nicaraguan pediatric influenza cohort study: design, methods, use of technology, and compliance. BMC Infect Dis (2015) 15:504. doi:10.1186/s12879-015-1256-6
100. Thomas S, Rouilly V, Patin E, Alanio C, Dubois A, Delval C, et al. The Milieu Interieur study – an integrative approach for study of human immunological variance. Clin Immunol (2015) 157(2):277–93. doi:10.1016/j.clim.2014.12.004
101. Duffy D, Rouilly V, Libri V, Hasan M, Beitz B, David M, et al. Functional analysis via standardized whole-blood stimulation systems defines the boundaries of a healthy immune response to complex stimuli. Immunity (2014) 40(3):436–50. doi:10.1016/j.immuni.2014.03.002
102. Duffy D, Rouilly V, Braudeau C, Corbiere V, Djebali R, Ungeheuer MN, et al. Standardized whole blood stimulation improves immunomonitoring of induced immune responses in multi-center study. Clin Immunol (2017) 183:325–35. doi:10.1016/j.clim.2017.09.019
103. Pavlova S, D’Alessio F, Houard S, Remarque EJ, Stockhofe N, Engelhardt OG. Workshop report: immunoassay standardisation for “universal” influenza vaccines. Influenza Other Respir Viruses (2017) 11(3):194–201. doi:10.1111/irv.12445
104. European Centre for Disease Prevention and Control. Annual Epidemiological Report for 2015 – Seasonal Influenza [Internet]. Stockholm: ECDC (2017).
105. CDC. Influenza Season Vaccination Coverage Report [Online]. (2014). Available from: https://www.cdc.gov/flu/fluvaxview/reportshtml/reporti1314/reporti/index.html (Accessed: April 24, 2018).
106. CDC. ACIP Votes Down use of LAIV for 2016-2017 Flu Season. (2016). Available from: https://www.cdc.gov/media/releases/2016/s0622-laiv-flu.html (Accessed: April 24, 2018).
107. Gorse GJ, Falsey AR, Ozol-Godfrey A, Landolfi V, Tsang PH. Safety and immunogenicity of a quadrivalent intradermal influenza vaccine in adults. Vaccine (2015) 33(9):1151–9. doi:10.1016/j.vaccine.2015.01.025
108. Nachbagauer R, Kinzler D, Choi A, Hirsh A, Beaulieu E, Lecrenier N, et al. A chimeric haemagglutinin-based influenza split virion vaccine adjuvanted with AS03 induces protective stalk-reactive antibodies in mice. NPJ Vaccines (2016) 1:16015. doi:10.1038/npjvaccines.2016.15
109. Widdice LE, Bernstein DI, Leonard AC, Marsolo KA, Kahn JA. Adherence to the HPV vaccine dosing intervals and factors associated with completion of 3 doses. Pediatrics (2011) 127(1):77–84. doi:10.1542/peds.2010-0812
110. Trantham L, Kurosky S, Zhang D, Johnson K. Adherence to multi-dose hepatitis A and hepatitis B vaccine schedules in the United States. Open Forum Infect Dis (2017) 4(Suppl_1):S518. doi:10.1093/ofid/ofx163.1347
111. MacDonald NE; SAGE Working Group on Vaccine Hesitancy. Vaccine hesitancy: definition, scope and determinants. Vaccine (2015) 33(34):4161–4. doi:10.1016/j.vaccine.2015.04.036
112. Zhou L, Su Q, Xu Z, Feng A, Jin H, Wang S, et al. Seasonal influenza vaccination coverage rate of target groups in selected cities and provinces in China by season (2009/10 to 2011/12). PLoS One (2013) 8(9):e73724. doi:10.1371/journal.pone.0073724
113. Black CL, Yue X, Mps, Ball SW, Fink R, de Perio MA, et al. Influenza vaccination coverage among health care personnel – United States, 2016–17 influenza season. Morb Mortal Wkly Rep (2017) 66(38):1009–15. doi:10.15585/mmwr.mm6638a1
114. Piedra PA, Gaglani MJ, Kozinetz CA, Herschler G, Riggs M, Griffith M, et al. Herd immunity in adults against influenza-related illnesses with use of the trivalent-live attenuated influenza vaccine (CAIV-T) in children. Vaccine (2005) 23(13):1540–8. doi:10.1016/j.vaccine.2004.09.025
115. Cohen SA, Chui KK, Naumova EN. Influenza vaccination in young children reduces influenza-associated hospitalizations in older adults, 2002-2006. J Am Geriatr Soc (2011) 59(2):327–32. doi:10.1111/j.1532-5415.2010.03271.x
116. Pebody RG, Green HK, Andrews N, Boddington NL, Zhao H, Yonova I, et al. Uptake and impact of vaccinating school age children against influenza during a season with circulation of drifted influenza A and B strains, England, 2014/15. Euro Surveill (2015) 20(39):30029. doi:10.2807/1560-7917.ES.2015.20.39.30029
117. Monto AS, Davenport FM, Napier JA, Francis T Jr. Effect of vaccination of a school-age population upon the course of an A2-Hong Kong influenza epidemic. Bull World Health Organ (1969) 41(3):537–42.
118. Medlock J, Galvani AP. Optimizing influenza vaccine distribution. Science (2009) 325(5948):1705–8. doi:10.1126/science.1175570
119. Reichert TA, Sugaya N, Fedson DS, Glezen WP, Simonsen L, Tashiro M. The Japanese experience with vaccinating schoolchildren against influenza. N Engl J Med (2001) 344(12):889–96. doi:10.1056/NEJM200103223441204
120. Taksler GB, Rothberg MB, Cutler DM. Association of influenza vaccination coverage in younger adults with influenza-related illness in the elderly. Clin Infect Dis (2015) 61(10):1495–503. doi:10.1093/cid/civ630
Keywords: influenza virus, universal vaccine, T cell, hemagglutinin-stalk, clinical trials
Citation: Valkenburg SA, Leung NHL, Bull MB, Yan LM, Li APY, Poon LLM and Cowling BJ (2018) The Hurdles From Bench to Bedside in the Realization and Implementation of a Universal Influenza Vaccine. Front. Immunol. 9:1479. doi: 10.3389/fimmu.2018.01479
Received: 27 April 2018; Accepted: 14 June 2018;
Published: 02 July 2018
Edited by:
Alan Chen-Yu Hsu, University of Newcastle, AustraliaReviewed by:
Stephen Kent, University of Melbourne, AustraliaAlice Sijts, Utrecht University, Netherlands
Copyright: © 2018 Valkenburg, Leung, Bull, Yan, Li, Poon and Cowling. This is an open-access article distributed under the terms of the Creative Commons Attribution License (CC BY). The use, distribution or reproduction in other forums is permitted, provided the original author(s) and the copyright owner are credited and that the original publication in this journal is cited, in accordance with accepted academic practice. No use, distribution or reproduction is permitted which does not comply with these terms.
*Correspondence: Sophie A. Valkenburg, c29waGlldiYjeDAwMDQwO2hrdS5oaw==