- Singapore Immunology Network (SIgN), Agency of Science, Technology and Research (A*STAR), Singapore, Singapore
Candidemia is a bloodstream fungal infection caused by Candida species and is most commonly observed in hospitalized patients. Even with proper antifungal drug treatment, mortality rates remain high at 40–50%. Therefore, prophylactic or preemptive antifungal medications are currently recommended in order to prevent infections in high-risk patients. Moreover, the majority of women experience at least one episode of vulvovaginal candidiasis (VVC) throughout their lifetime and many of them suffer from recurrent VVC (RVVC) with frequent relapses for the rest of their lives. While there currently exists no definitive cure, the only available treatment for RVVC is again represented by antifungal drug therapy. However, due to the limited number of existing antifungal drugs, their associated side effects and the increasing occurrence of drug resistance, other approaches are greatly needed. An obvious prevention measure for candidemia or RVVC relapse would be to immunize at-risk patients with a vaccine effective against Candida infections. In spite of the advanced and proven techniques successfully applied to the development of antibacterial or antiviral vaccines, however, no antifungal vaccine is still available on the market. In this review, we first summarize various efforts to date in the development of anti-Candida vaccines, highlighting advantages and disadvantages of each strategy. We next unfold and discuss general hurdles encountered along these efforts, such as the existence of large genomic variation and phenotypic plasticity across Candida strains and species, and the difficulty in mounting protective immune responses in immunocompromised or immunosuppressed patients. Lastly, we review the concept of “trained immunity” and discuss how induction of this rapid and nonspecific immune response may potentially open new and alternative preventive strategies against opportunistic infections by Candida species and potentially other pathogens.
Introduction
Due to advances in medicine and surgery, over the past century there has been a rising population of immunocompromised patients, which are at an elevated risk to suffer from opportunistic infections (1, 2). While hospital-acquired fungal infections are less frequent than bacterial ones, they disproportionately account for higher mortality rates, longer hospitalization times and increased healthcare costs (3). Risk factors for fungal infections are very broad and nonspecific, and they include chronic respiratory disease, cancer, HIV infection, organ transplantation, neutropenia, presence of central venous catheters, prolonged hospital stay, administration of total parenteral nutrition, exposure to invasive procedures, chemotherapy, hemodialysis, gastric acid suppression, and use of broad-spectrum antibiotics (4, 5). Candidiasis (caused by Candida species) is the most common opportunistic fungal infection and the fourth most common nosocomial bloodstream infection (6, 7). Despite the best available standards of care, the incidence of candidemia, a sign of invasive or systemic candidiasis, is on the rise in the US (8) and mortality rates often exceed 50% despite use of antifungal drugs (9). This is especially true in intensive care units and in immunocompromised patients, where Candida bloodstream infections are estimated to strike ~400,000 patients a year, with an associated mortality of 46–75% (10). For all these reasons, and because the hospitalization and treatment cost of these patients is very high (3, 11), CDC guidelines recommend prophylactic or preemptive antifungal treatment in patients considered at high risk of candidemia (12).
Mucosal fungal infections, though almost never life-threatening, are much more common than invasive ones and can be associated with high morbidity, socioeconomic impact, and low quality of life. The most common infection sites are the oral cavity and the genitourinary tract (13). Vulvovaginal candidiasis (VVC) is the most common mucosal fungal infection, with different studies estimating that 50–70% of women suffer an episode of VVC at least once in their lifetime and 5–8% of women suffer from recurrent VVC (RVVC) (14). To this date, there still exists no definitive cure for RVVC, which is commonly treated with over-the-counter antifungal medications but continues to relapse ≥4 times a year for the rest of a woman’s life (15).
Antifungal agents available for the treatment of systemic or mucosal candidiasis are restricted to only a few classes of compounds, such as azoles, polyenes, allylamines, and echinocandins (16). However, adverse side effects, toxicity, and emergence of drug resistance limit the use of these drugs (10). In particular, various Candida species can acquire resistance to different antifungals or, even worse, to more than one drug (17–20). In fact, the emergence of drug resistance in Candida spp. has been on a growing trend over the past decade (21), with multidrug-resistant Candida spp. now being reported all over the world (22, 23). Finally, the recent discovery of a novel multidrug resistant species of Candida (C. auris) poses a further threat to our ability to use antifungal drugs to treat candidiasis (24).
To reduce the incidence and mortality of opportunistic fungal infections, experts agree that we need (i) improved diagnostics to allow more rapid implementation of appropriate therapies, (ii) more effective antifungal agents associated with less severe side effects, and (iii) to develop immunotherapies based on our mounting knowledge of antifungal immunity (10, 25). These same strategies could be used also to reduce the recurrence and severity of RVVC episodes. We hereby argue that an anti-Candida vaccine, as long as it was effective in the populations of patients it is intended to protect, could help reduce the global burden of both systemic and vaginal candidiasis bringing about substantial socioeconomic benefits.
In this review, we first provide an up-to-date overview of various efforts that have been attempted so far in the quest of an anti-Candida vaccine, which has remained elusive. We next critically assess potential common problems that might have hindered such efforts and finally propose a few tentative solutions to overcoming those problems.
Current Anti-Candida Vaccine Landscape
In the last decades, several different anti-Candida vaccines have been proposed but only few of them have been tested in clinical trials (Table 1). Here, we will summarize the current anti-Candida vaccine landscape and discuss advantages and disadvantages of each vaccination strategy.
Historically, vaccines are developed to boost antigen-specific immune responses and elicit protective immunological memory against specific pathogenic strains. In the case of Candida species, most of the efforts have been focused around the most common species, C. albicans. Different strategies have been used to immunize hosts against candidiasis: (i) live-attenuated strains, (ii) recombinant proteins, and (iii) glycoconjugates.
Live-Attenuated Vaccines
The first ever reported vaccine, Edward Jenner’s famous vaccinia virus, was a live virus causing cowpox in cattle but protecting humans against smallpox (49). By all definitions, it was a live-attenuated vaccine, which caused only a very mild form of the disease in humans while at the same time raising long-lasting protective immune memory. Up to this date, most currently available antiviral vaccines (e.g., yellow fever, measles, varicella) and some antibacterial ones (e.g., BCG) are essentially live-attenuated versions of their pathogenic counterparts. Because of this long history of success, it is not surprising that several studies have ascribed various attenuated strains of C. albicans the ability to confer protection against candidiasis.
The morphogenetic transition between the conidial (yeast) and hyphal (filamentous) form is one of the most important virulence factors in C. albicans (50); for this reason, the absence of filamentation is a common characteristic of attenuated strains of this species. Several C. albicans mutants associated with no or low virulence, including PCA-2 (incapable of yeast-hyphae conversion), CNC13 (deleted in the MAP kinase HOG1), RML2U [deleted in the cell wall protein (CWP) gene ECM33], and tet-NRG1 (in which the filamentation repressor NRG1 can be overexpressed by doxycycline), have been used to immunize mice and shown to protect them from a subsequent lethal systemic infection with a virulent strain (26–29). Also, the C. albicans double-mutant cph1/efg1, which is severely impaired in hyphal morphogenesis, partially protects mice from systemic infection by a wild-type C. albicans strain (30).
Not only C. albicans attenuated strains have been used to protect against systemic candidiasis, but also the generally regarded-as-safe baker’s yeast Saccharomyces cerevisiae has been successfully used to this end. Stevens and colleagues have described the use of heat-killed or live S. cerevisiae as a protective vaccine against C. albicans infection, but also against A. fumigatus, Cryptococcus grubii, and Coccidioides infection in a dose-dependent manner (31–34).
Despite these success stories at the basic research level, none of these vaccine candidates has progressed to clinical trials. Possible reasons include the fact that characterization of these strains is complex, that the stability of the virulence-attenuating mutations is not guaranteed, and that the use of live-attenuated vaccines is currently not recommended in immunocompromised patients due to their weaker immune defense and the higher probability to develop a disease.
Recombinant Proteins
In contrast to live Candida vaccines (how attenuated they may be), recombinant vaccines are generally perceived as potentially safer to the human host, because they do not contain any infectious agent. This characteristic makes recombinant protein vaccines more suitable especially for the immunocompromised individuals that would most benefit from such a vaccine. In general, this strategy has focused on proteins expressed on the surface of the fungus, such as CWPs or adhesion proteins, to ensure that epitopes would be easily accessible and “visible” by the immune system. Efforts also often concentrated on proteins critical to virulence, such as hyphal-specific effectors, in order to mount immune responses specifically against the pathogenic forms of the fungus. Besides, current work has shown that the strongest adaptive immune memory responses are often directed toward hyphal-associated proteins.
Agglutinin-Like Sequence Proteins
Agglutinin-like sequence (Als) proteins are located at the surface of C. albicans and play important roles in the adhesion to human endothelial cells and in the development of invasive candidiasis (51). Because these proteins are both exposed on the fungal surface and important for invasion of host cells, several vaccines have been proposed against invasive candidiasis using recombinant versions of various Als proteins, including Als1p and Als3p, formulated with or without adjuvants.
The recombinant N-terminus of Als1 (rAls1p-N) was produced and purified from S. cerevisiae and used in combination with Complete Freund’s Adjuvant (CFA) subcutaneously and boosted with different doses of rAls1p-N with Incomplete Freund’s Adjuvant (IFA) at day 21 post-immunization; lethal challenge with live C. albicans showed a survival rate of 50–57% and a decrease in fungal burden (36). This vaccine was deemed effective and improved survival in both immunocompetent and neutropenic mice and in murine models of oropharyngeal candidiasis and Candida vaginitis (35).
Vaccination with the recombinant N-terminus of Als3 (rAls3p-N) induced a stronger antibody response and survival rate compared with the rAls1p-N vaccine, and was more effective than rAls1p-N in the murine model of oropharyngeal and vaginal candidiasis (37). Interestingly, it showed also protection against S. aureus infection (38), suggesting the existence of shared immune epitopes between these distantly related species; consistently, Candida Als3p is structurally similar to a clumping factor of S. aureus (52).
Bar et al. identified an antigenic peptide by immunoproteomic approaches called pAls, widely conserved in many non-Candida species; this epitope mediates T-cell-dependent protection from invasive candidiasis. This pAls epitope is found in the Als3 protein and the authors suggest an implication of this epitope in the protective effect of the Als3 vaccine (53).
NDV-3A, a rAls3p-N vaccine formulated with Alhydrogel adjuvant, has been tested in a phase-I clinical trial. The trial was performed on 40 healthy volunteers and showed an increase in antibody titers at two different doses, as well as increased cytokine responses and IgG and IgA1 titers in the revaccinated subjects (39). A few adverse events have been described, but overall the vaccine was well tolerated by the subjects and hence the results were deemed as promising. Like the version of rAls3p-N vaccine without adjuvant, NDV-3 also showed activity against S. aureus infection, further corroborating the idea that the vaccination with Candida antigens containing epitope homologs found in other organisms can be harnessed to generate “convergent immunity.” NDV-3A is now in a phase-II clinical trial to test the immunotherapeutic effect of the vaccine in women with RVVC.1
Secreted Aspartil Proteases
Secreted Aspartil Proteases (SAP) constitute a family of 10 proteins secreted by C. albicans, which are required for adhesion, epithelial, and endothelial invasion and fungal cell metabolism (54, 55). Sap2p is the most abundantly expressed SAP in C. albicans and its recombinant form has been used to immunize rats intravaginally or intranasally, either using cholera toxin as an adjuvant or without an adjuvant; the vaccination resulted in the clearance of the Candida vaginal infection (40). The same research group also developed PEV7, a truncated version of Sap2p (amino acids 77–400) incorporated into the lipid bilayer of influenza virosomes. Because preclinical data demonstrated that intramuscularly vaccinated rats showed protection against vaginal candidiasis (41), PEV7 has progressed to clinical trials as a therapeutic vaccine for the treatment of RVVC. In a randomized phase-I trial, the subjects, whether immunized via intramuscular injections or by intravaginal capsules, showed a strong B-cell-mediated immune response in vaginal and cervical samples. All volunteers showed a mucosal immune response with consistently high titers across the groups, the response was dose-dependent and no serious adverse events were reported.2
Heat Shock Protein 90 (Hsp90p)
Heat shock protein 90 is a highly conserved stress-induced chaperone, with key functions in setting cellular responses to stressful stimuli, which is indispensable for yeast viability and located in the cell wall of C. albicans. The 47-kDa carboxyl fragment of C. albicans Hsp90 had been identified as a Candida antigen in different studies (56). This antigen is very abundant and immunogenic, and the presence of antibodies against Hsp90 correlates with good prognosis, whereas low levels are associated with mortality (57). Various Hsp90 epitopes (LSREM, LKVIRK, and DEPAGE) have been identified using a phage-display library and shown to induce Hsp90-specific serum antibodies and to prolong survival in a mouse model of systemic candidiasis (42). A recombinant protein called Mycograb, consisting of cross-linked Hsp90 NKILKVIRKNIVKK peptide-binding variable domains of human antibody heavy and light chains, was constructed and expressed in Escherichia coli. When combined with amphotericin B, Mycograb produced significant improvement in patients with invasive candidiasis (58, 59). However, the drug was refused by the European Medicines Agency on the grounds of product safety and quality, and its ability to potentiate the effects of amphotericin B were later found to be non Hsp90-specific (60).
Hyphally Regulated Proteins
Hyphally regulated 1 (Hyr1) is a glycosyl phosphatidylinositol (GPI)-anchor mannoprotein that is expressed during hyphal formation on the cell wall of C. albicans. A recombinant version of the N-terminus of Hyr1 (rHyr1p-N) was produced in E. coli and used to immunize mice via subcutaneous injection with either CFA or aluminum hydroxide and boosted on day 21 with IFA. After 2 weeks, vaccinated mice were challenged with a lethal dose of C. albicans and non-albicans Candida (NAC) species. The vaccine was effective against infections by C. albicans, C. glabrata, C. krusei, C. parapsilosis, and C. tropicalis in both immunocompetent and neutropenic mice (43).
CWP Extracts
Not only purified recombinant proteins, but also crude C. albicans cell wall extracts, seem to be effective to protect against invasive candidiasis. In fact, subcutaneous immunization with β-mercaptoethanol-extracted C. albicans CWPs in combination with Ribi Adjuvant System (RAS) R-700 followed by a booster injected 21 days after the first immunization, conferred protection in 75% of immunized mice after a lethal challenge with C. albicans (44). However, such crude preparations are unlikely to progress to clinical trials due to the difficulty in characterizing and standardizing such complex vaccine formulations.
Glycoconjugates
An alternative to protein-based vaccines is to use glycans commonly found in fungal cell walls but absent in host cells. In fact, the Candida cell wall represents a hub of pathogen-associated molecular pattern (PAMP)–pathogen recognition receptor (PRR) interactions that dictate downstream immune responses (61). For these reasons, various cell wall polysaccharides have been tested as vaccine targets against systemic candidiasis.
Mannans and Derivative Peptide Conjugates
Mannans are polymers of O-linked or N-linked mannosides attached to CWPs and constitute the outermost, and hence most accessible, layer of the C. albicans cell wall. While O-mannans are predominately recognized by TLR4 (62), N-mannans are recognized by a multitude of receptors, including the Mannose Receptor (MR), DC-SIGN, Dectin-2, Galectin-3, and Mincle (63). Classically, Candida mannans have been attributed immunesuppressive properties (64); but the discovery that N-linked mannans are critically required for recognition by DC-SIGN and MR on human dendritic cells (DCs) has established a rational for conjugating them to antigenic peptides. Consistent with this idea, mannosylated antigens were shown to be presented more effectively than non-mannosylated forms (65).
In one example, researchers have used computational epitope searches to select 6 peptides in C. albicans CWPs (fructose-bisphosphate aldolase; methyltetrahydropteroyltriglutamate; hyphal wall protein-1; enolase; glyceraldehyde-3-phosphate dehydrogenase; and phosphoglycerate kinase) and conjugated these peptides with β-mannans to generate the first series of fully synthetic glycopeptide vaccines against systemic candidiasis. Depending on the specific peptide, the immunized mice showed 80–100% survival and reduced kidney fungal burden after the challenge with C. albicans (45).
More recent studies showed than the vaccination with BSA-based conjugates bearing synthetic α-1,6-branched oligomannosides induced humoral responses in mice and induced production of potentially protective antibodies (46). Immunization with Candida mannan-derived branched 3,6-di-O-substituted α-oligomannosides conjugated to BSA (M5-BSA and M6-BSA conjugates) induced similar levels of CD19+ B lymphocytes, but the candidacidal activity of polymorphonuclear leukocytes induced by opsonization with M6-BSA antisera was higher than that with M5-BSA, thus revealing some important differences in their ability to induce an effective and protective immune response against Candida infection (66). A better mechanistic understanding of the type of immune responses elicited by various Candida mannans is therefore required before these compounds can progress to clinical studies.
β-Glucan and Derivative Conjugate Vaccines
Directly underneath mannans lies a hidden layer of β-glucans, which is an essential component of the Candida cell wall (67) and elicits Dectin-1-dependent innate immune responses that are critical for host protection against fungi (68). Moreover, β-glucans from the C. albicans cell wall are highly immunogenic, leading to epigenetic and metabolic reprogramming of monocytes/macrophages, elevated cytokine responses and to innate host protection against systemic candidiasis (69–71). This phenomenon was termed “trained immunity” (72) and is discussed further in Section “Trained Immunity—Toward the Next Generation of Vaccines?”
When used in combination with the MF59 adjuvant (oil-in-water emulsion of squalene oil), fungal-derived β-glucan showed protection against murine vaginal candidiasis but is currently not approved for use in humans (47). The conjugation of the β-glucan preparation Laminarin (a soluble Dectin-1 ligand derived from seaweed) with diphtheria toxoid (Lam-CRM197) resulted, in murine models, in significant protection against systemic candidiasis, as well as cross-protection against aspergillosis (48). In further studies, researchers conjugated the diphtheria toxoid with various other β-glucan preparations, such as curdlan (a high-molecular-weight β-1,3-linked polymer produced by bacteria) or synthetic oligosaccharides that contained either only linear β-1,3 linkages or also branched β-1,6 linkages, and combined it with the MF59 adjuvant. Interestingly, conjugates raising antibodies against β-1,3-glucans were protective in a mouse model of systemic candidiasis, while anti-β-1,6-antibodies appeared to reverse this effect (73). Overall these studies reveal the high immunogenicity of β-glucans but also a surprisingly complex relationship between antifungal immune responses and host protection against fungal infections. Moreover, different Candida species expose β-glucan on their surface to different degrees (74), questioning how broadly protective such vaccines would be against various candidiasis-causing agents. These complexities will need to be better understood, before these polysaccharides can be translated into safe and effective antifungal vaccines for human use.
Common Challenges Faced so Far
The fact that C. albicans is thought to have coevolved with humans for at least the past 2,000 years (75), that it is a lifelong inhabitant of humans that colonizes the gastrointestinal (GI) tract since birth (76) and that is then transmitted between family members (77), poses a few conceptual and technical challenges in the development of an anti-Candida vaccine. On one hand, this long interaction history with the human GI tract suggests that these fungi might have evolved a series of mechanisms to escape various host immune defenses, including a high genetic, phenotypic and morphological plasticity (78). On the other hand, humans might have learned to recognize Candida species as commensals and might have therefore developed immune tolerance toward them—breaking this tolerance is expected to be both difficult and in some cases even counterproductive (79). Lastly, the highest risk group for Candida-related infections is represented by immunocompromised and immunosuppressed patients, i.e., a class of individuals that is intrinsically less responsive to immunization. Here, we will outline each of these issues in greater detail, and later we will attempt to offer some possible ways forward based on recent learning points.
Zeroing in on a Moving Target
Morphological and Phenotypic Plasticity of C. albicans
A first difficulty encountered when designing an anti-Candida vaccine lies in the morphological and phenotypic plasticity of these fungi (Figure 1), which makes them almost “moving targets.” In fact, C. albicans is a polymorphic fungus that can reversibly transition between yeast, pseudohyphal and hyphal forms—a property that is closely linked to its evolutionary adaptation to life inside the human host. When C. albicans grows in its unicellular yeast form, it is commonly regarded as a harmless colonizer, while its switching to the hyphal form is related to pathogenesis, in that hyphae adhere to and invade epithelial cells resulting in extensive damage to host cells (80).
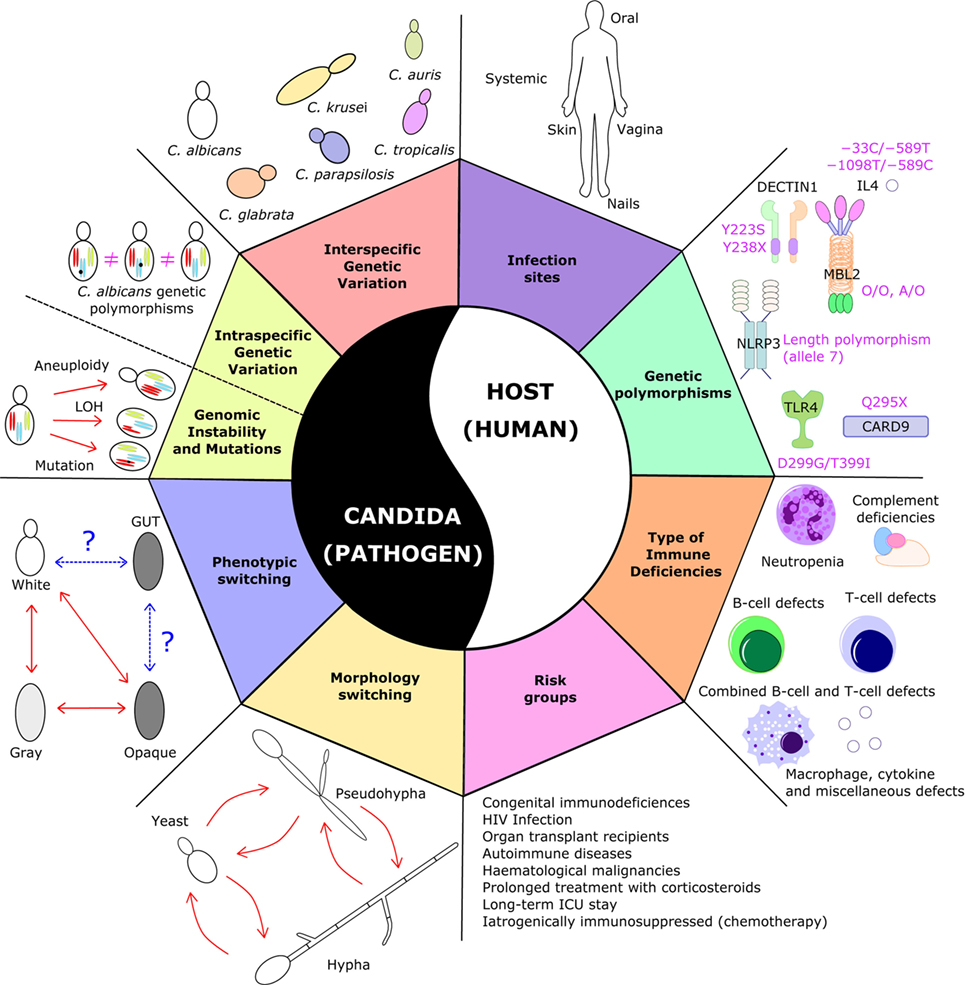
Figure 1. Sources of variability in Candida-related infections. The figure illustrates the plethora of variations at the level of the pathogen and the host, which pose serious challenges in the development of an antifungal vaccine. On the pathogen side, Candida species display large phenotypic, morphological, and genetic variation and dynamics; on the host side, candidiasis can occur in different types of patients, with different types of immune system dysfunction or carrying different genetic polymorphisms, and in different anatomical sites. Taken together, this broad and diverse clinical spectrum of the disease makes it difficult to design a “one-size-fits-all” vaccine that would protect all these different patients from all these different types of fungal infection.
Interestingly, C. albicans isolated from the blood of patients with candidiasis often matches those recovered from the GI tract of the same patients (81), suggesting that C. albicans invades the bloodstream directly from the GI tract. How exactly the GI barrier is crossed is currently not completely understood, but it is thought to be mediated by or facilitated by epithelial tissue damage; and that C. albicans hyphal formation might be critical for this ability (82). Therefore, hyphal-specific or hyphal-associated CWPs such as Hyr1, Hwp2, Plb5, and Sod5 have all been proposed as vaccine target candidates (83).
However, vaccines against hyphal CWPs alone may not be sufficient to provide immune protection. While direct penetration into the mucosal surfaces by the hyphal form is important for invasion, widespread dissemination through the bloodstream and to the organs is thought to be facilitated by the yeast form (84). As both yeast and hyphae are detected in patients with candidiasis (85), and some mutants defective in filamentation displayed similar virulence as the wild type (86), it is the plasticity of this morphological switch, rather than the hyphal form alone, that appears to critically underlie the virulence of C. albicans. The variety of different forms of C. albicans that exist in various organs may represent a further challenge in vaccine development. For example, in mouse infection models, while C. albicans is mainly observed in the hyphal form in the kidney, it actually persists as a yeast in the spleen and the liver (87). To achieve sterile protection, the ideal vaccine should be able to stimulate clearance of the fungus not only in the former but also in the latter organs.
A second form of plasticity in C. albicans is a heritable white-to-opaque phenotypic switch. Smooth and white colonies are observed during the white phase, while elongated and rod-like cells giving rise to flattened gray colonies appear in the opaque phase (88). Opaque cells are less virulent than white cells in mouse bloodstream infection model (89, 90) and undergo filamentation in response to stimuli that are distinct form white cells (91). The switching between white and opaque phenotypes may facilitate C. albicans to evade from host immune response, as opaque cells are less susceptible to be phagocytosed by macrophages (92) and also capable to evade killing by neutrophils (93).
Another phenotypic switch, known as gastrointestinally induced transition (GUT) and found as a result of WOR1 overexpression, was recently described to confer increased fitness of C. albicans in the GI tract of antibiotic-treated mice (94). While GUT cells share some resemblance with opaque cells, such as a reduced virulent in mouse bloodstream infections, they appear to be transcriptionally distinct from both white and opaque cells, therefore likely representing an independent epigenetic state. Whether GUT cells also arise in mucosal or systemic infections in humans and whether these cells express specific factors that are important for disease remains to be determined, but their discovery nonetheless sheds light on additional layers of complexity in the morphological and phenotypic plasticity of this fungal pathogen.
As a whole, morphological and phenotypic plasticity renders C. albicans a “skilled transformer” (78). Therefore, vaccines directed against single targets expressed only in a particular fungal form may not be sufficient to raise protective immune responses against the entire arsenal of antigens and virulence factors that are dynamically presented to the host in different organs at different times.
Genomic Plasticity of Candida albicans
Candida albicans, apart from morphological and phenotypic transitions, also displays a high degree of genomic plasticity including gross chromosome rearrangement, aneuploidy and loss of heterozygosity when exposed to different stresses. Such genomic changes equip C. albicans to rapidly adapt to an adverse environment by changing the copy number of specific genes on a given chromosome (95). One of the well-known examples is the identification of an isochromosome composed of two copies of the left arm of chromosome 5, which is associated with azole resistance (17) due to amplification of two resistance genes, ERG11 and TAC1 (96). Genomic variations are commonly observed in clinical C. albicans isolates, which includes copy number variations, chromosomal inversions, subtelomeric hypervariation, loss of heterozygosity and aneuploidy (97). Moreover, C. albicans with increased DNA content (aneuploidy) occurs at a higher frequency in clinical blood isolates when compared with mucosal isolates (98). This suggests that acquisition of aneuploidy, and the consequent copy number alteration of specific genes, may provide a rapid mechanism to modify the expression pattern of certain antigens that were originally targeted by a certain vaccine.
Non-albicans Candida Species
The genus Candida represents a highly heterogeneous group of >50 known species. Nevertheless, >90% of the invasive Candida infections are caused by C. albicans, C. glabrata, C. parapsilosis, C. tropicalis or C. krusei (99). While this figure has remained largely unchanged, the relative ranking between these species has seen significant shifts in different regions over the past decades (100). While C. albicans is still considered the most common species causing candidemia, increasing rates of NAC species in candidemia have been reported worldwide (18, 101, 102). Moreover, significant variations of Candida species are detected in different patient groups and geographical regions (103). For instance, C. glabrata has emerged as an important pathogen in northern Europe, the United States and Canada with a higher rate of incidence in adults than in children, and lower in neonates (104). C. parapsilosis, instead, is more prominent in southern Europe, Asia, and South America and is mostly associated with low-birth-weight neonates and transplant recipients (105). Finally, C. tropicalis constitutes 20–45% of Candida isolates in the Asia-Pacific region (18) and invasive candidiasis due to this species is commonly associated with patients with neutropenia and malignancy (106). Recently, the emergence of C. auris, first reported in 2009 in Japan, highlights a new challenge of antifungal treatment, as C. auris is often multi-drug resistant and also difficult to be diagnosed with standard laboratory methods, which is contributing to its rapid spreading over multiple countries (107). In addition to NAC, multispecies candidemia is also emerging as a threat (108). Taken together, these observations suggest that vaccines specifically against C. albicans alone may not be sufficient to provide protection against candidiasis caused by other emerging NAC species or mixed Candida species infections.
Breaking Immune Tolerance Toward Fungi
A microbial ecosystem in the human intestine, known as the gut microbiome, harbors more than 100 trillion microorganisms and thus immunological tolerogenic responses are required in order to maintain gut homeostasis and prevent chronic inflammation (109). Immune tolerance toward human gut commensals, such as Bacteroides fragilis and certain Clostridia species, is maintained by regulatory T (Treg) cell responses (110–112). Apart from bacteria, Candida species are the most common fungal species found in the GI tract; it is therefore reasonable to assume that similar tolerance mechanisms might have evolved to regulate the commensal relationship between humans and fungi. One such mechanism, which likely resulted from the coevolution of bacterial microbiota, commensal fungi and host immune system, relies on the metabolic tryptophan-AhR pathway and 2,3-indoleamine dioxygenase (IDO) (113, 114). C. albicans, being a lifelong inhabitant of humans that colonizes the GI tract since birth (76), is able to induce IDO expression in DCs. IDO-expressing DCs then promote tolerogenic Treg responses, probably facilitating the switch from pathogenicity to commensalism in C. albicans (115).
The existence of immunological tolerance toward C. albicans and probably other Candida species poses two serious challenges for anti-Candida vaccine development. First, unlike obligate pathogens with no commensal relationship with humans, this tolerance represents an additional hurdle toward establishment of effective and protective immunological memory. Second, much of the clinical manifestations of Candida-related infections are more due to host-derived immunopathology than to pathogen-derived host damage (116, 117). For instance, mice lacking the chemokine receptor Ccr1, which is critical for neutrophil recruitment, display improved renal function during invasive candidiasis (118) and administration of Ccr1 antagonists reduces renal tissue injury and improves survival in mice challenged with systemic candidiasis (119).
It has thus been argued that a careful balance between immunity and tolerance must be established to maintain commensalism (79). Breaking host tolerance against fungi and the self-regulated equilibrium between Th17 and Treg responses might lead to undesired consequences, such as exacerbating fungal disease progression or other underlying inflammatory or autoimmune conditions.
Vaccinating Patients With No Immunity
Fungal infections, especially invasive ones, most frequently occur in individuals with compromised or suppressed immunity. Hence, it appears that the patients that would mostly benefit from a future antifungal vaccine are also those least likely to respond to it. Is such an effort then even justified? Or is it doomed to fail from the start? Answers to these questions are more complex than one might expect.
Efficacy and safety are always a primary concern in any vaccine development effort, and especially so in vulnerable subjects such as patients with lowered immune defenses. As mentioned, the risk of Candida infection is especially high in patients with neutropenia, hematological malignancies, solid-organ transplants, prolonged treatment with corticosteroids, long-term ICU stay, chemotherapy and HIV infection (120). The ideal anti-Candida vaccine should possess all of the following properties: (i) zero risk of causing a Candida-related infection or to exacerbate the immunopathology associated with an ongoing or future fungal infection, (ii) be immunogenic enough to elicit protection in patients with little or no immunity, (iii) do not increase the risk of aggravating any underlying disease. While this may sound like a “catch-22,” vaccinating immunocompromised patients has been attempted with various degrees of success in the past for other kinds of infection.
For instance, a single-dose of the pneumococcal vaccine was recently deemed safe and immunogenic in children under active immunosuppressive therapy (121). However, low immunogenicity of the meningococcal vaccine was reported in solid-organ transplant recipients (122). Lower immunogenicity against the influenza vaccine is generally reported in immunocompromised patients when compared with healthy individuals (123). However, using a high-dose influenza vaccine was reported to be more immunogenic than the standard dose in children and young adults with leukemia or solid tumors, although not in those with HIV (124).
What is progressively becoming clearer is that a “one-size-fits-all” anti-Candida vaccine may never see the light. For example, a vaccine effective in preventing candidemia in neutropenic cancer patients might not be effective in T-cell-deficient HIV or transplant patients and vice versa. As a whole, one of the greatest challenges of any antifungal vaccine will be to deal with the large diversity of underlying disease states and of the associated types of immunosuppression that characterizes this highly heterogeneous risk group (Figure 1).
Back to the Future: New Vaccine Strategies on the Horizon?
Multivalent Vaccines
Some monovalent vaccines, i.e., those directed against a single strain or a single antigen, are very effective. A good example is represented by the measles vaccine, which has almost completely eradicated the disease in countries where it has been employed consistently throughout the population (125). However, the most recent vaccines tend to be multivalent, i.e., they carry multiple antigens of two or more strains/serotypes of the same pathogen. An example is the quadrivalent meningococcal vaccine that protects against 4 serogroups (A, C, W-135, and Y) of meningococci. The quadrivalent vaccine was shown to be more effective in reducing invasive meningococcal disease incidence when compared with the monovalent C vaccine (126). Another example is the 13-valent pneumococcal conjugate vaccine (PCV13) containing antigens from 13 serotypes of pneumococci. A replacement of the seven-valent pneumococcal conjugate vaccine (PCV7) with PCV13 covering additional six of the most prevalent serotypes that are not included in PCV7 successfully reduced the burden of pneumococcal disease in pediatric populations (127).
As C. albicans itself expresses a range of virulence factors and several NAC species also carry their own species-specific virulence factors, the recent development of univalent subunit vaccines may face practical obstacles. Considering above success stories of multivalent vaccines applied to bacterial infections, it has been argued that a better approach toward the development of an anti-Candida vaccine would be to simultaneously target multiple unrelated virulence-associated antigens (78). The predicted advantages include the lower probability of selecting “escape mutants” and a higher selectively against the pathogenic form of C. albicans, thus sparing the commensal—and perhaps beneficial—form of this gut inhabitant. In particular, it was proposed to combine a few of the univalent vaccines that have so far progressed furthest in clinical trials, such as the Als3 and the Sap2 vaccines (78).
More recently, a study reported that a newly identified cytolytic peptide toxin, Candidalysin, is secreted from C. albicans to induce epithelial cell damage and inflammation and to facilitate tissue invasion (128). Intriguingly, the mechanism of action of this novel virulence factor is partially uncoupled from the hyphal morphogenesis program, suggesting that a vaccine targeting both the former and the latter would be more effective than one targeting only one or the other. In general, as more knowledge is gained on the pathogenesis of C. albicans, further targets could be added to the list of antigens to be included in a multivalent formulation. At the same time, we can also predict that as the complexity of the vaccine increases, so will regulatory hurdles and manufacturing challenges. Nevertheless, testing the efficacy and safety of a combination of more and more antigens is likely going to be crucial for better vaccines against Candida infections.
Trained Immunity—Toward the Next Generation of Vaccines?
The classic vaccination paradigm is based on adaptive immunity and the raising of long-lasting, protective B- and/or T-cell memory responses (129). As mentioned above, mounting protective antibodies or T-cell responses across different Candida strains and species may be challenging due to their large genetic, phenotypic and morphological variation and plasticity. In addition, the capacity to mount B- and T-memory responses may be impaired in immunocompromised or immunosuppressed patients such as HIV/AIDS or transplant recipients who are among the highest risk groups for candidiasis. Though innate immunity lacks most of the properties of classical immunological memory, various studies have demonstrated that certain vaccines induce a type of innate immune memory known as “trained immunity” (Figure 2), which is mediated by monocytes, macrophages or NK cells (72). For instance, BCG vaccination reduces mortality in low-birth-weight children (130, 131) and vaccination against measles reduces all-cause mortality in childhood (132), suggesting non-specific cross-protection against other infections (133).
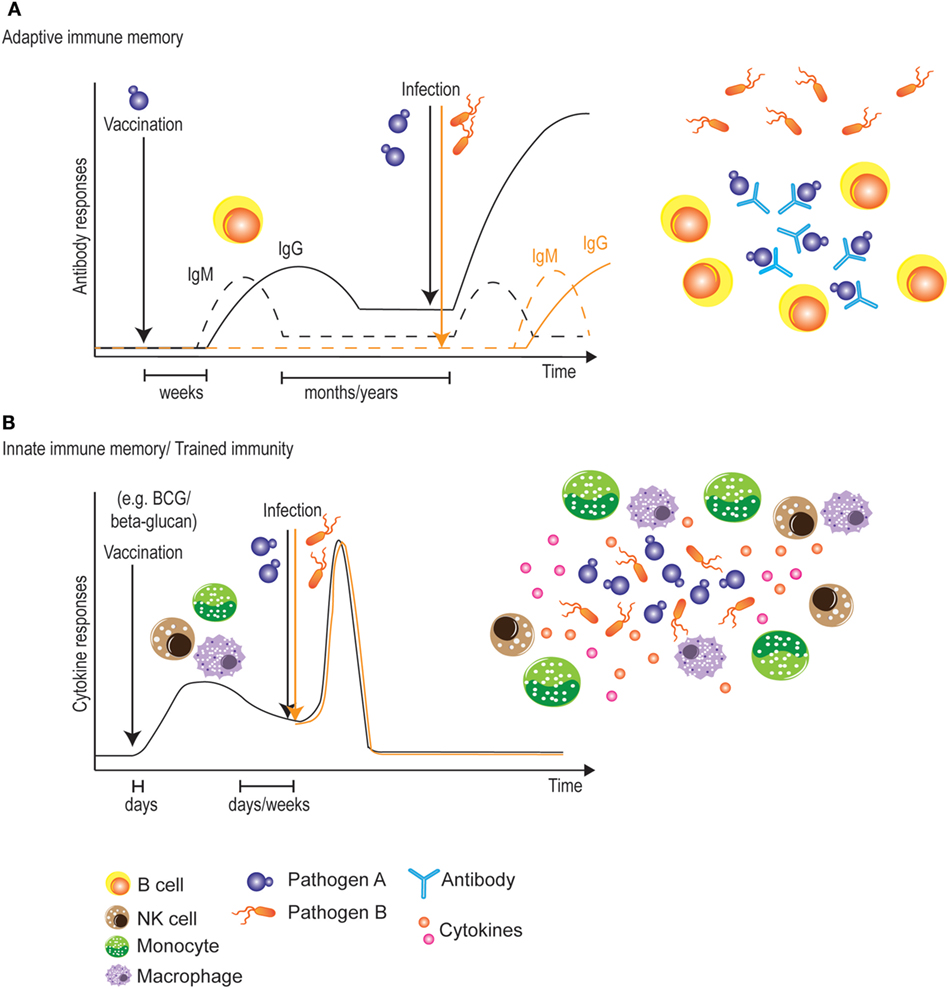
Figure 2. Vaccination strategies targeting adaptive or innate immune memory. (A) Classical vaccines initially induce a slow adaptive immune response. During this primary response, however, adaptive immune memory is raised, allowing for a more rapid and stronger secondary response upon encounter of the targeted pathogen. This protection is very long-lived, but is limited to the antigens present in the vaccine. (B) Most vaccines also induce a very rapid innate immune response. During this primary response, some vaccines also “train” innate immune cells, allowing for a stronger secondary innate response against pathogens. One advantage of this innate immune memory is the non-specificity of the secondary response, which could potentially lead to broadly cross-protective vaccines. A potential disadvantage would be the relatively shorter time window of protection.
Testing whether such innate memory responses could be stimulated also in adults, PBMCs obtained 2 weeks or 3 months after BCG vaccination were found to produce higher levels of pro-inflammatory cytokines such as TNF-α and IL-1β when restimulated in vitro with C. albicans (134). Such increased cytokine production capacity involved epigenetic changes mediated by increased H3K4 trimethylation in monocytes and was dependent on NOD2 and Rip2. Similarly, SCID mice vaccinated by BCG are protected against lethal C. albicans infection through a T and B lymphocyte-independent mechanism (134). Apart from the increased production of pro-inflammatory cytokines in monocytes, another study reported that BCG vaccination also enhances IL-1β production in human NK cells when stimulated with C. albicans. BCG-induced protection against disseminated C. albicans infection was shown to be partially dependent on NK cells in NOD-SCID-IL2Rγ−/− mice (135). These studies warrant clinical trials to test if BCG vaccination could reduce the risk of candidiasis in high-risk patients.
Consistent with what reviewed in Section “Live-Attenuated Vaccines,” systemic infection of mice with an avirulent C. albicans strain was shown by Antonio Cassone’s group to confer protection against a subsequent challenge with a pathogenic C. albicans strain (26). The protection was shown to be non-specific, as cross-protection against C. tropicalis and Staphylococcus aureus was equally observed, and to be mediated by macrophage-like cells, as adoptive transfer of “plastic-adherent” cells was sufficient to confer protection against subsequent challenge with a virulent strain of C. albicans. Several years later, Mihai Netea’s group demonstrated that vaccination of both wild-type and RAG1−/− mice, but not CCR2−/− mice that lack circulating monocytes, with a sublethal dose of a virulent C. albicans strain similarly affords protection against reinfection with a lethal dose of the same strain, and dubbed the phenomenon “trained immunity” (69). Increased TNF-α and IL-6 levels from the trained monocytes were induced by β-glucan found in the C. albicans cell wall and sensed by Dectin-1 on host cells. In another study, mice trained with Candida-derived β-glucan showed increased serum levels of TNF-α and IL-6 when challenged by LPS four days later, but the response was transient and diminished after 20 days (136). To test the possibility of using β-glucan as a vaccine or “immune trainer” in humans, a pilot study was conducted by orally administrating β-glucan to healthy volunteers and subsequently testing innate immune responses in PBMCs restimulated in vitro with C. albicans (137). Enhanced innate immune responses as in the mouse model, however, were unfortunately not observed in this human study. This may probably be due to the solubility of β-glucan and the absorbability of β-glucan in the human GI tract. Further studies using different administration routes are still worth exploring, in order to establish whether β-glucan could one day be used as a vaccine to induce trained immunity against subsequent Candida infections (Figure 2).
Concluding Remarks
With the increasing reports of multidrug resistance in several Candida species, prophylactic vaccination of at-risk patients likely represents a more effective long-term measure to reduce the growing incidence of Candida-related infections. The main challenges faced by Candida vaccine developers are the large variation and plasticity of these fungi, the existence of preestablished immunological tolerance and the difficulty in raising protective memory responses in patients with impaired adaptive immunity. In addition to multivalent vaccines, we propose that future vaccine development efforts should harness the growing mechanistic understanding of trained innate immunity, which might provide not only protection against candidiasis but also potentially cross-protection against a wide range of opportunistic infections. Further research on the mechanism, efficacy, and safety of raising trained immunity especially in immunocompromised patients would pave the way toward the development of a new generation of vaccines against Candida-related and other nosocomial infections.
Author Contributions
GT and JR-C contributed equally as first authors. GT and JR-C conducted literature review and drafted the manuscript. NP conceptualized and oversaw the study and revised the manuscript. All authors read and approved the submitted version.
Conflict of Interest Statement
The authors are inventors on a patent application related to the topic of this article.
Acknowledgments
The authors are thankful to all authors of the cited literature for their invaluable contributions and apologize for any omissions that were due to lack of space.
Funding
This work was supported by an A*STAR Investigatorship to NP (JCO/1437a00117) and by core funding by the Singapore Immunology Network (SIgN), A*STAR.
Footnotes
- ^https://www.clinicaltrials.gov/ct2/show/NCT01926028 (Accessed: April 17, 2018).
- ^https://www.clinicaltrials.gov/ct2/show/NCT01067131 (Accessed: April 17, 2018).
References
1. Fishman JA. Infection in solid-organ transplant recipients. N Engl J Med (2007) 357:2601–14. doi:10.1056/NEJMra064928
2. Teoh F, Pavelka N. How chemotherapy increases the risk of systemic candidiasis in cancer patients: current paradigm and future directions. Pathogens (2016) 5:6. doi:10.3390/pathogens5010006
3. Menzin J, Meyers JL, Friedman M, Perfect JR, Langston AA, Danna RP, et al. Mortality, length of hospitalization, and costs associated with invasive fungal infections in high-risk patients. Am J Health Syst Pharm (2009) 66:1711–7. doi:10.2146/ajhp080325
4. Pfaller MA, Diekema DJ. Epidemiology of invasive candidiasis: a persistent public health problem. Clin Microbiol Rev (2007) 20:133–63. doi:10.1128/CMR.00029-06
5. Richardson M, Lass-Florl C. Changing epidemiology of systemic fungal infections. Clin Microbiol Infect (2008) 14(Suppl 4):5–24. doi:10.1111/j.1469-0691.2008.01978.x
6. Wisplinghoff H, Bischoff T, Tallent SM, Seifert H, Wenzel RP, Edmond MB. Nosocomial bloodstream infections in US hospitals: analysis of 24,179 cases from a prospective nationwide surveillance study. Clin Infect Dis (2004) 39:309–17. doi:10.1086/421946
7. Peman J, Canton E, Quindos G, Eraso E, Alcoba J, Guinea J, et al. Epidemiology, species distribution and in vitro antifungal susceptibility of fungaemia in a Spanish multicentre prospective survey. J Antimicrob Chemother (2012) 67:1181–7. doi:10.1093/jac/dks019
8. Cleveland AA, Farley MM, Harrison LH, Stein B, Hollick R, Lockhart SR, et al. Changes in incidence and antifungal drug resistance in candidemia: results from population-based laboratory surveillance in Atlanta and Baltimore, 2008-2011. Clin Infect Dis (2012) 55:1352–61. doi:10.1093/cid/cis697
9. Eliakim-Raz N, Babaoff R, Yahav D, Yanai S, Shaked H, Bishara J. Epidemiology, microbiology, clinical characteristics, and outcomes of candidemia in internal medicine wards-a retrospective study. Int J Infect Dis (2016) 52:49–54. doi:10.1016/j.ijid.2016.09.018
10. Brown GD, Denning DW, Gow NA, Levitz SM, Netea MG, White TC. Hidden killers: human fungal infections. Sci Transl Med (2012) 4:165rv113. doi:10.1126/scitranslmed.3004404
11. Smith PB, Morgan J, Benjamin JD, Fridkin SK, Sanza LT, Harrison LH, et al. Excess costs of hospital care associated with neonatal candidemia. Pediatr Infect Dis J (2007) 26:197–200. doi:10.1097/01.inf.0000253973.89097.c0
12. Pappas PG, Kauffman CA, Andes DR, Clancy CJ, Marr KA, Ostrosky-Zeichner L, et al. Clinical practice guideline for the management of candidiasis: 2016 update by the Infectious Diseases Society of America. Clin Infect Dis (2016) 62:e1–50. doi:10.1093/cid/civ1194
13. Havlickova B, Czaika VA, Friedrich M. Epidemiological trends in skin mycoses worldwide. Mycoses (2008) 51(Suppl 4):2–15. doi:10.1111/j.1439-0507.2008.01606.x
15. Sobel JD, Faro S, Force RW, Foxman B, Ledger WJ, Nyirjesy PR, et al. Vulvovaginal candidiasis: epidemiologic, diagnostic, and therapeutic considerations. Am J Obstet Gynecol (1998) 178:203–11. doi:10.1016/S0002-9378(98)80001-X
16. Cannon RD, Lamping E, Holmes AR, Niimi K, Baret PV, Keniya MV, et al. Efflux-mediated antifungal drug resistance. Clin Microbiol Rev (2009) 22:291–321. doi:10.1128/CMR.00051-08
17. Selmecki A, Forche A, Berman J. Aneuploidy and isochromosome formation in drug-resistant Candida albicans. Science (2006) 313:367–70. doi:10.1126/science.1128242
18. Pfaller MA, Diekema DJ, Gibbs DL, Newell VA, Ellis D, Tullio V, et al. Results from the ARTEMIS DISK Global Antifungal Surveillance Study, 1997 to 2007: a 10.5-year analysis of susceptibilities of Candida species to fluconazole and voriconazole as determined by CLSI standardized disk diffusion. J Clin Microbiol (2010) 48:1366–77. doi:10.1128/JCM.02117-09
19. Alexander BD, Johnson MD, Pfeiffer CD, Jimenez-Ortigosa C, Catania J, Booker R, et al. Increasing echinocandin resistance in Candida glabrata: clinical failure correlates with presence of FKS mutations and elevated minimum inhibitory concentrations. Clin Infect Dis (2013) 56:1724–32. doi:10.1093/cid/cit136
20. Reales-Calderon JA, Molero G, Gil C, Martinez JL. The fungal resistome: a risk and an opportunity for the development of novel antifungal therapies. Future Med Chem (2016) 8:1503–20. doi:10.4155/fmc-2016-0051
21. Fadda ME, Podda GS, Pisano MB, Deplano M, Cosentino S. Prevalence of Candida species in different hospital wards and their susceptibility to antifungal agents: results of a three year survey. J Prev Med Hyg (2008) 49:69–74. doi:10.15167/2421-4248/jpmh2008.49.2.119
22. Vermitsky JP, Edlind TD. Azole resistance in Candida glabrata: coordinate upregulation of multidrug transporters and evidence for a Pdr1-like transcription factor. Antimicrob Agents Chemother (2004) 48:3773–81. doi:10.1128/AAC.48.10.3773-3781.2004
23. Vermitsky JP, Earhart KD, Smith WL, Homayouni R, Edlind TD, Rogers PD. Pdr1 regulates multidrug resistance in Candida glabrata: gene disruption and genome-wide expression studies. Mol Microbiol (2006) 61:704–22. doi:10.1111/j.1365-2958.2006.05235.x
24. Navalkele BD, Revankar S, Chandrasekar P. Candida auris: a worrisome, globally emerging pathogen. Expert Rev Anti Infect Ther (2017) 15(9):819–27. doi:10.1080/14787210.2017.1364992
25. Denning DW, Perlin DS, Muldoon EG, Colombo AL, Chakrabarti A, Richardson MD, et al. Delivering on antimicrobial resistance agenda not possible without improving fungal diagnostic capabilities. Emerg Infect Dis (2017) 23:177–83. doi:10.3201/eid2302.152042
26. Bistoni F, Vecchiarelli A, Cenci E, Puccetti P, Marconi P, Cassone A. Evidence for macrophage-mediated protection against lethal Candida albicans infection. Infect Immun (1986) 51:668–74.
27. Fernandez-Arenas E, Molero G, Nombela C, Diez-Orejas R, Gil C. Low virulent strains of Candida albicans: unravelling the antigens for a future vaccine. Proteomics (2004) 4:3007–20. doi:10.1002/pmic.200400929
28. Martinez-Lopez R, Nombela C, Diez-Orejas R, Monteoliva L, Gil C. Immunoproteomic analysis of the protective response obtained from vaccination with Candida albicans ecm33 cell wall mutant in mice. Proteomics (2008) 8:2651–64. doi:10.1002/pmic.200701056
29. Saville SP, Lazzell AL, Chaturvedi AK, Monteagudo C, Lopez-Ribot JL. Efficacy of a genetically engineered Candida albicans tet-NRG1 strain as an experimental live attenuated vaccine against hematogenously disseminated candidiasis. Clin Vaccine Immunol (2009) 16:430–2. doi:10.1128/CVI.00480-08
30. Yang YL, Wang CW, Chen CT, Wang MH, Hsiao CF, Lo HJ. Non-lethal Candida albicans cph1/cph1 efg1/efg1 mutant partially protects mice from systemic infections by lethal wild-type cells. Mycol Res (2009) 113:388–90. doi:10.1016/j.mycres.2008.11.016
31. Capilla J, Clemons KV, Liu M, Levine HB, Stevens DA. Saccharomyces cerevisiae as a vaccine against coccidioidomycosis. Vaccine (2009) 27:3662–8. doi:10.1016/j.vaccine.2009.03.030
32. Liu M, Capilla J, Johansen ME, Alvarado D, Martinez M, Chen V, et al. Saccharomyces as a vaccine against systemic aspergillosis: ‘the friend of man’ a friend again? J Med Microbiol (2011) 60:1423–32. doi:10.1099/jmm.0.033290-0
33. Liu M, Clemons KV, Johansen ME, Martinez M, Chen V, Stevens DA. Saccharomyces as a vaccine against systemic candidiasis. Immunol Invest (2012) 41:847–55. doi:10.3109/08820139.2012.692418
34. Majumder T, Liu M, Chen V, Martinez M, Alvarado D, Clemons KV, et al. Killed Saccharomyces cerevisiae protects against lethal challenge of Cryptococcus grubii. Mycopathologia (2014) 178:189–95. doi:10.1007/s11046-014-9798-5
35. Spellberg BJ, Ibrahim AS, Avenissian V, Filler SG, Myers CL, Fu Y, et al. The anti-Candida albicans vaccine composed of the recombinant N terminus of Als1p reduces fungal burden and improves survival in both immunocompetent and immunocompromised mice. Infect Immun (2005) 73:6191–3. doi:10.1128/IAI.73.9.6191-6193.2005
36. Ibrahim AS, Spellberg BJ, Avanesian V, Fu Y, Edwards JE Jr. The anti-Candida vaccine based on the recombinant N-terminal domain of Als1p is broadly active against disseminated candidiasis. Infect Immun (2006) 74:3039–41. doi:10.1128/IAI.74.5.3039-3041.2006
37. Spellberg BJ, Ibrahim AS, Avanesian V, Fu Y, Myers C, Phan QT, et al. Efficacy of the anti-Candida rAls3p-N or rAls1p-N vaccines against disseminated and mucosal candidiasis. J Infect Dis (2006) 194:256–60. doi:10.1086/504691
38. Lin L, Ibrahim AS, Xu X, Farber JM, Avanesian V, Baquir B, et al. Th1-Th17 cells mediate protective adaptive immunity against Staphylococcus aureus and Candida albicans infection in mice. PLoS Pathog (2009) 5:e1000703. doi:10.1371/journal.ppat.1000703
39. Schmidt CS, White CJ, Ibrahim AS, Filler SG, Fu Y, Yeaman MR, et al. NDV-3, a recombinant alum-adjuvanted vaccine for Candida and Staphylococcus aureus, is safe and immunogenic in healthy adults. Vaccine (2012) 30:7594–600. doi:10.1016/j.vaccine.2012.10.038
40. De Bernardis F, Boccanera M, Adriani D, Girolamo A, Cassone A. Intravaginal and intranasal immunizations are equally effective in inducing vaginal antibodies and conferring protection against vaginal candidiasis. Infect Immun (2002) 70:2725–9. doi:10.1128/IAI.70.5.2725-2729.2002
41. De Bernardis F, Amacker M, Arancia S, Sandini S, Gremion C, Zurbriggen R, et al. A virosomal vaccine against candidal vaginitis: immunogenicity, efficacy and safety profile in animal models. Vaccine (2012) 30:4490–8. doi:10.1016/j.vaccine.2012.04.069
42. Yang Q, Wang L, Lu DN, Gao RJ, Song JN, Hua PY, et al. Prophylactic vaccination with phage-displayed epitope of C. albicans elicits protective immune responses against systemic candidiasis in C57BL/6 mice. Vaccine (2005) 23:4088–96. doi:10.1016/j.vaccine.2004.07.005
43. Luo G, Ibrahim AS, French SW, Edwards JE Jr, Fu Y. Active and passive immunization with rHyr1p-N protects mice against hematogenously disseminated candidiasis. PLoS One (2011) 6:e25909. doi:10.1371/journal.pone.0025909
44. Thomas DP, Viudes A, Monteagudo C, Lazzell AL, Saville SP, Lopez-Ribot JL. A proteomic-based approach for the identification of Candida albicans protein components present in a subunit vaccine that protects against disseminated candidiasis. Proteomics (2006) 6:6033–41. doi:10.1002/pmic.200600321
45. Xin H, Dziadek S, Bundle DR, Cutler JE. Synthetic glycopeptide vaccines combining beta-mannan and peptide epitopes induce protection against candidiasis. Proc Natl Acad Sci U S A (2008) 105:13526–31. doi:10.1073/pnas.0803195105
46. Paulovicova L, Paulovicova E, Karelin AA, Tsvetkov YE, Nifantiev NE, Bystricky S. Effect of branched alpha-oligomannoside structures on induction of anti-Candida humoral immune response. Scand J Immunol (2013) 77:431–41. doi:10.1111/sji.12044
47. Pietrella D, Rachini A, Torosantucci A, Chiani P, Brown AJ, Bistoni F, et al. A beta-glucan-conjugate vaccine and anti-beta-glucan antibodies are effective against murine vaginal candidiasis as assessed by a novel in vivo imaging technique. Vaccine (2010) 28:1717–25. doi:10.1016/j.vaccine.2009.12.021
48. Torosantucci A, Bromuro C, Chiani P, De Bernardis F, Berti F, Galli C, et al. A novel glyco-conjugate vaccine against fungal pathogens. J Exp Med (2005) 202:597–606. doi:10.1084/jem.20050749
49. Smith KA. Edward Jenner and the small pox vaccine. Front Immunol (2011) 2:21. doi:10.3389/fimmu.2011.00021
50. Lo HJ, Kohler JR, Didomenico B, Loebenberg D, Cacciapuoti A, Fink GR. Nonfilamentous C. albicans mutants are avirulent. Cell (1997) 90:939–49. doi:10.1016/S0092-8674(00)80358-X
51. Hoyer LL, Cota E. Candida albicans agglutinin-like sequence (Als) family vignettes: a review of Als protein structure and function. Front Microbiol (2016) 7:280. doi:10.3389/fmicb.2016.00280
52. Spellberg B, Ibrahim AS, Yeaman MR, Lin L, Fu Y, Avanesian V, et al. The antifungal vaccine derived from the recombinant N terminus of Als3p protects mice against the bacterium Staphylococcus aureus. Infect Immun (2008) 76:4574–80. doi:10.1128/IAI.00700-08
53. Bar E, Gladiator A, Bastidas S, Roschitzki B, Acha-Orbea H, Oxenius A, et al. A novel Th cell epitope of Candida albicans mediates protection from fungal infection. J Immunol (2012) 188:5636–43. doi:10.4049/jimmunol.1200594
54. Hube B, Naglik J. Candida albicans proteinases: resolving the mystery of a gene family. Microbiology (2001) 147:1997–2005. doi:10.1099/00221287-147-8-1997
55. Naglik JR, Challacombe SJ, Hube B. Candida albicans secreted aspartyl proteinases in virulence and pathogenesis. Microbiol Mol Biol Rev (2003) 67:400–28, table of contents. doi:10.1128/MMBR.67.3.400-428.2003
56. Matthews R, Burnie J. Cloning of a DNA sequence encoding a major fragment of the 47 kilodalton stress protein homologue of Candida albicans. FEMS Microbiol Lett (1989) 51:25–30. doi:10.1016/0378-1097(89)90071-2
57. Matthews RC, Burnie JP, Tabaqchali S. Isolation of immunodominant antigens from sera of patients with systemic candidiasis and characterization of serological response to Candida albicans. J Clin Microbiol (1987) 25:230–7.
58. Matthews RC, Rigg G, Hodgetts S, Carter T, Chapman C, Gregory C, et al. Preclinical assessment of the efficacy of mycograb, a human recombinant antibody against fungal HSP90. Antimicrob Agents Chemother (2003) 47:2208–16. doi:10.1128/AAC.47.7.2208-2216.2003
59. Pachl J, Svoboda P, Jacobs F, Vandewoude K, van der Hoven B, Spronk P, et al. A randomized, blinded, multicenter trial of lipid-associated amphotericin B alone versus in combination with an antibody-based inhibitor of heat shock protein 90 in patients with invasive candidiasis. Clin Infect Dis (2006) 42:1404–13. doi:10.1086/503428
60. Richie DL, Ghannoum MA, Isham N, Thompson KV, Ryder NS. Nonspecific effect of Mycograb on amphotericin B MIC. Antimicrob Agents Chemother (2012) 56:3963–4. doi:10.1128/AAC.00435-12
61. Netea MG, Brown GD, Kullberg BJ, Gow NA. An integrated model of the recognition of Candida albicans by the innate immune system. Nat Rev Microbiol (2008) 6:67–78. doi:10.1038/nrmicro1815
62. Netea MG, Gow NA, Munro CA, Bates S, Collins C, Ferwerda G, et al. Immune sensing of Candida albicans requires cooperative recognition of mannans and glucans by lectin and toll-like receptors. J Clin Invest (2006) 116:1642–50. doi:10.1172/JCI27114
63. Hall RA, Gow NA. Mannosylation in Candida albicans: role in cell wall function and immune recognition. Mol Microbiol (2013) 90:1147–61. doi:10.1111/mmi.12426
64. Nelson RD, Shibata N, Podzorski RP, Herron MJ. Candida mannan: chemistry, suppression of cell-mediated immunity, and possible mechanisms of action. Clin Microbiol Rev (1991) 4:1–19. doi:10.1128/CMR.4.1.1
65. Tan MC, Mommaas AM, Drijfhout JW, Jordens R, Onderwater JJ, Verwoerd D, et al. Mannose receptor-mediated uptake of antigens strongly enhances HLA class II-restricted antigen presentation by cultured dendritic cells. Eur J Immunol (1997) 27:2426–35. doi:10.1002/eji.1830270942
66. Paulovicova L, Paulovicova E, Karelin AA, Tsvetkov YE, Nifantiev NE, Bystricky S. Immune cell response to Candida cell wall mannan derived branched alpha-oligomannoside conjugates in mice. J Microbiol Immunol Infect (2015) 48:9–19. doi:10.1016/j.jmii.2013.08.020
67. Douglas CM, D’ippolito JA, Shei GJ, Meinz M, Onishi J, Marrinan JA, et al. Identification of the FKS1 gene of Candida albicans as the essential target of 1,3-beta-d-glucan synthase inhibitors. Antimicrob Agents Chemother (1997) 41:2471–9.
68. Taylor PR, Tsoni SV, Willment JA, Dennehy KM, Rosas M, Findon H, et al. Dectin-1 is required for beta-glucan recognition and control of fungal infection. Nat Immunol (2007) 8:31–8. doi:10.1038/ni1408
69. Quintin J, Saeed S, Martens JHA, Giamarellos-Bourboulis EJ, Ifrim DC, Logie C, et al. Candida albicans infection affords protection against reinfection via functional reprogramming of monocytes. Cell Host Microbe (2012) 12:223–32. doi:10.1016/j.chom.2012.06.006
70. Cheng SC, Quintin J, Cramer RA, Shepardson KM, Saeed S, Kumar V, et al. mTOR- and HIF-1alpha-mediated aerobic glycolysis as metabolic basis for trained immunity. Science (2014) 345:1250684. doi:10.1126/science.1250684
71. Saeed S, Quintin J, Kerstens HH, Rao NA, Aghajanirefah A, Matarese F, et al. Epigenetic programming of monocyte-to-macrophage differentiation and trained innate immunity. Science (2014) 345:1251086. doi:10.1126/science.1251086
72. Netea MG, Joosten LA, Latz E, Mills KH, Natoli G, Stunnenberg HG, et al. Trained immunity: a program of innate immune memory in health and disease. Science (2016) 352:aaf1098. doi:10.1126/science.aaf1098
73. Bromuro C, Romano M, Chiani P, Berti F, Tontini M, Proietti D, et al. Beta-glucan-CRM197 conjugates as candidates antifungal vaccines. Vaccine (2010) 28:2615–23. doi:10.1016/j.vaccine.2010.01.012
74. Sem X, Le GT, Tan AS, Tso G, Yurieva M, Liao WW, et al. Beta-glucan exposure on the fungal cell wall tightly correlates with competitive fitness of Candida species in the mouse gastrointestinal tract. Front Cell Infect Microbiol (2016) 6:186. doi:10.3389/fcimb.2016.00186
75. Barnett JA. A history of research on yeasts 12: medical yeasts part 1, Candida albicans. Yeast (2008) 25:385–417. doi:10.1002/yea.1595
76. Fujimura KE, Sitarik AR, Havstad S, Lin DL, Levan S, Fadrosh D, et al. Neonatal gut microbiota associates with childhood multisensitized atopy and T cell differentiation. Nat Med (2016) 22:1187–91. doi:10.1038/nm.4176
77. Bougnoux ME, Diogo D, Francois N, Sendid B, Veirmeire S, Colombel JF, et al. Multilocus sequence typing reveals intrafamilial transmission and microevolutions of Candida albicans isolates from the human digestive tract. J Clin Microbiol (2006) 44:1810–20. doi:10.1128/JCM.44.5.1810-1820.2006
78. Cassone A. Development of vaccines for Candida albicans: fighting a skilled transformer. Nat Rev Microbiol (2013) 11:884–91. doi:10.1038/nrmicro3156
79. Carvalho A, Cunha C, Bozza S, Moretti S, Massi-Benedetti C, Bistoni F, et al. Immunity and tolerance to fungi in hematopoietic transplantation: principles and perspectives. Front Immunol (2012) 3:156. doi:10.3389/fimmu.2012.00156
80. Kumamoto CA, Vinces MD. Contributions of hyphae and hypha-co-regulated genes to Candida albicans virulence. Cell Microbiol (2005) 7:1546–54. doi:10.1111/j.1462-5822.2005.00616.x
81. Miranda LN, van der Heijden IM, Costa SF, Sousa AP, Sienra RA, Gobara S, et al. Candida colonisation as a source for candidaemia. J Hosp Infect (2009) 72:9–16. doi:10.1016/j.jhin.2009.02.009
82. Koh AY, Kohler JR, Coggshall KT, Van Rooijen N, Pier GB. Mucosal damage and neutropenia are required for Candida albicans dissemination. PLoS Pathog (2008) 4:e35. doi:10.1371/journal.ppat.0040035
83. Heilmann CJ, Sorgo AG, Siliakus AR, Dekker HL, Brul S, De Koster CG, et al. Hyphal induction in the human fungal pathogen Candida albicans reveals a characteristic wall protein profile. Microbiology (2011) 157:2297–307. doi:10.1099/mic.0.049395-0
84. Saville SP, Lazzell AL, Monteagudo C, Lopez-Ribot JL. Engineered control of cell morphology in vivo reveals distinct roles for yeast and filamentous forms of Candida albicans during infection. Eukaryot Cell (2003) 2:1053–60. doi:10.1128/EC.2.5.1053-1060.2003
85. Di Carlo P, Di Vita G, Guadagnino G, Cocorullo G, D’arpa F, Salamone G, et al. Surgical pathology and the diagnosis of invasive visceral yeast infection: two case reports and literature review. World J Emerg Surg (2013) 8:38. doi:10.1186/1749-7922-8-38
86. Noble SM, French S, Kohn LA, Chen V, Johnson AD. Systematic screens of a Candida albicans homozygous deletion library decouple morphogenetic switching and pathogenicity. Nat Genet (2010) 42:590–8. doi:10.1038/ng.605
87. Lionakis MS, Lim JK, Lee CC, Murphy PM. Organ-specific innate immune responses in a mouse model of invasive candidiasis. J Innate Immun (2011) 3:180–99. doi:10.1159/000321157
88. Lohse MB, Johnson AD. White-opaque switching in Candida albicans. Curr Opin Microbiol (2009) 12:650–4. doi:10.1016/j.mib.2009.09.010
89. Kvaal CA, Srikantha T, Soll DR. Misexpression of the white-phase-specific gene WH11 in the opaque phase of Candida albicans affects switching and virulence. Infect Immun (1997) 65:4468–75.
90. Kvaal C, Lachke SA, Srikantha T, Daniels K, Mccoy J, Soll DR. Misexpression of the opaque-phase-specific gene PEP1 (SAP1) in the white phase of Candida albicans confers increased virulence in a mouse model of cutaneous infection. Infect Immun (1999) 67:6652–62.
91. Si H, Hernday AD, Hirakawa MP, Johnson AD, Bennett RJ. Candida albicans white and opaque cells undergo distinct programs of filamentous growth. PLoS Pathog (2013) 9:e1003210. doi:10.1371/journal.ppat.1003210
92. Lohse MB, Johnson AD. Differential phagocytosis of white versus opaque Candida albicans by Drosophila and mouse phagocytes. PLoS One (2008) 3:e1473. doi:10.1371/journal.pone.0001473
93. Sasse C, Hasenberg M, Weyler M, Gunzer M, Morschhauser J. White-opaque switching of Candida albicans allows immune evasion in an environment-dependent fashion. Eukaryot Cell (2013) 12:50–8. doi:10.1128/EC.00266-12
94. Pande K, Chen C, Noble SM. Passage through the mammalian gut triggers a phenotypic switch that promotes Candida albicans commensalism. Nat Genet (2013) 45:1088–91. doi:10.1038/ng.2710
95. Selmecki A, Forche A, Berman J. Genomic plasticity of the human fungal pathogen Candida albicans. Eukaryot Cell (2010) 9:991–1008. doi:10.1128/EC.00060-10
96. Selmecki A, Gerami-Nejad M, Paulson C, Forche A, Berman J. An isochromosome confers drug resistance in vivo by amplification of two genes, ERG11 and TAC1. Mol Microbiol (2008) 68:624–41. doi:10.1111/j.1365-2958.2008.06176.x
97. Hirakawa MP, Martinez DA, Sakthikumar S, Anderson MZ, Berlin A, Gujja S, et al. Genetic and phenotypic intra-species variation in Candida albicans. Genome Res (2015) 25:413–25. doi:10.1101/gr.174623.114
98. Mandelblat M, Frenkel M, Abbey D, Ben Ami R, Berman J, Segal E. Phenotypic and genotypic characteristics of Candida albicans isolates from bloodstream and mucosal infections. Mycoses (2017) 60:534–45. doi:10.1111/myc.12623
99. Pfaller MA, Diekema DJ. Epidemiology of invasive mycoses in North America. Crit Rev Microbiol (2010) 36:1–53. doi:10.3109/10408410903241444
100. Falagas ME, Apostolou KE, Pappas VD. Attributable mortality of candidemia: a systematic review of matched cohort and case-control studies. Eur J Clin Microbiol Infect Dis (2006) 25:419–25. doi:10.1007/s10096-006-0159-2
101. Eggimann P, Garbino J, Pittet D. Epidemiology of Candida species infections in critically ill non-immunosuppressed patients. Lancet Infect Dis (2003) 3:685–702. doi:10.1016/S1473-3099(03)00801-6
102. Bassetti M, Merelli M, Righi E, Diaz-Martin A, Rosello EM, Luzzati R, et al. Epidemiology, species distribution, antifungal susceptibility, and outcome of candidemia across five sites in Italy and Spain. J Clin Microbiol (2013) 51:4167–72. doi:10.1128/JCM.01998-13
103. Pfaller MA, Castanheira M, Messer SA, Moet GJ, Jones RN. Variation in Candida spp. distribution and antifungal resistance rates among bloodstream infection isolates by patient age: report from the SENTRY Antimicrobial Surveillance Program (2008-2009). Diagn Microbiol Infect Dis (2010) 68:278–83. doi:10.1016/j.diagmicrobio.2010.06.015
104. Guinea J. Global trends in the distribution of Candida species causing candidemia. Clin Microbiol Infect (2014) 20(Suppl 6):5–10. doi:10.1111/1469-0691.12539
105. Trofa D, Gacser A, Nosanchuk JD. Candida parapsilosis, an emerging fungal pathogen. Clin Microbiol Rev (2008) 21:606–25. doi:10.1128/CMR.00013-08
106. Nucci M, Colombo AL. Candidemia due to Candida tropicalis: clinical, epidemiologic, and microbiologic characteristics of 188 episodes occurring in tertiary care hospitals. Diagn Microbiol Infect Dis (2007) 58:77–82. doi:10.1016/j.diagmicrobio.2006.11.009
107. Spivak ES, Hanson KE. Candida auris: an emerging fungal pathogen. J Clin Microbiol (2018) 56:e01588-17. doi:10.1128/JCM.01588-17
108. Nace HL, Horn D, Neofytos D. Epidemiology and outcome of multiple-species candidemia at a tertiary care center between 2004 and 2007. Diagn Microbiol Infect Dis (2009) 64:289–94. doi:10.1016/j.diagmicrobio.2009.03.010
109. Nutsch KM, Hsieh CS. T cell tolerance and immunity to commensal bacteria. Curr Opin Immunol (2012) 24:385–91. doi:10.1016/j.coi.2012.04.009
110. Round JL, Mazmanian SK. Inducible Foxp3+ regulatory T-cell development by a commensal bacterium of the intestinal microbiota. Proc Natl Acad Sci U S A (2010) 107:12204–9. doi:10.1073/pnas.0909122107
111. Atarashi K, Tanoue T, Oshima K, Suda W, Nagano Y, Nishikawa H, et al. Treg induction by a rationally selected mixture of clostridia strains from the human microbiota. Nature (2013) 500:232–6. doi:10.1038/nature12331
112. Furusawa Y, Obata Y, Fukuda S, Endo TA, Nakato G, Takahashi D, et al. Commensal microbe-derived butyrate induces the differentiation of colonic regulatory T cells. Nature (2013) 504:446–50. doi:10.1038/nature12721
113. Zelante T, Iannitti RG, Cunha C, De Luca A, Giovannini G, Pieraccini G, et al. Tryptophan catabolites from microbiota engage aryl hydrocarbon receptor and balance mucosal reactivity via interleukin-22. Immunity (2013) 39:372–85. doi:10.1016/j.immuni.2013.08.003
114. Romani L, Zelante T, De Luca A, Iannitti RG, Moretti S, Bartoli A, et al. Microbiota control of a tryptophan-AhR pathway in disease tolerance to fungi. Eur J Immunol (2014) 44:3192–200. doi:10.1002/eji.201344406
115. Bonifazi P, Zelante T, D’angelo C, De Luca A, Moretti S, Bozza S, et al. Balancing inflammation and tolerance in vivo through dendritic cells by the commensal Candida albicans. Mucosal Immunol (2009) 2:362–74. doi:10.1038/mi.2009.17
116. Duggan S, Leonhardt I, Hunniger K, Kurzai O. Host response to Candida albicans bloodstream infection and sepsis. Virulence (2015) 6:316–26. doi:10.4161/21505594.2014.988096
117. Carpino N, Naseem S, Frank DM, Konopka JB. Modulating host signaling pathways to promote resistance to infection by Candida albicans. Front Cell Infect Microbiol (2017) 7:481. doi:10.3389/fcimb.2017.00481
118. Lionakis MS, Fischer BG, Lim JK, Swamydas M, Wan W, Richard Lee CC, et al. Chemokine receptor Ccr1 drives neutrophil-mediated kidney immunopathology and mortality in invasive candidiasis. PLoS Pathog (2012) 8:e1002865. doi:10.1371/journal.ppat.1002865
119. Lionakis MS, Albert ND, Swamydas M, Lee CR, Loetscher P, Kontoyiannis DP. Pharmacological blockade of the chemokine receptor CCR1 protects mice from systemic candidiasis of hematogenous origin. Antimicrob Agents Chemother (2017) 61:e02365-16. doi:10.1128/AAC.02365-16
120. Kullberg BJ, Arendrup MC. Invasive candidiasis. N Engl J Med (2015) 373:1445–56. doi:10.1056/NEJMra1315399
121. Hung TY, Kotecha RS, Blyth CC, Steed SK, Thornton RB, Ryan AL, et al. Immunogenicity and safety of single-dose, 13-valent pneumococcal conjugate vaccine in pediatric and adolescent oncology patients. Cancer (2017) 123:4215–23. doi:10.1002/cncr.30764
122. Wyplosz B, Derradji O, Hong E, Francois H, Durrbach A, Duclos-Vallee JC, et al. Low immunogenicity of quadrivalent meningococcal vaccines in solid organ transplant recipients. Transpl Infect Dis (2015) 17:322–7. doi:10.1111/tid.12359
123. Zbinden D, Manuel O. Influenza vaccination in immunocompromised patients: efficacy and safety. Immunotherapy (2014) 6:131–9. doi:10.2217/imt.13.171
124. Hakim H, Allison KJ, Van de Velde LA, Tang L, Sun Y, Flynn PM, et al. Immunogenicity and safety of high-dose trivalent inactivated influenza vaccine compared to standard-dose vaccine in children and young adults with cancer or HIV infection. Vaccine (2016) 34:3141–8. doi:10.1016/j.vaccine.2016.04.053
125. Holzmann H, Hengel H, Tenbusch M, Doerr HW. Eradication of measles: remaining challenges. Med Microbiol Immunol (2016) 205:201–8. doi:10.1007/s00430-016-0451-4
126. Vickers DM, Anonychuk AM, De Wals P, Demarteau N, Bauch CT. Evaluation of serogroup C and ACWY meningococcal vaccine programs: projected impact on disease burden according to a stochastic two-strain dynamic model. Vaccine (2015) 33:268–75. doi:10.1016/j.vaccine.2013.09.034
127. Daniels CC, Rogers PD, Shelton CM. A review of pneumococcal vaccines: current polysaccharide vaccine recommendations and future protein antigens. J Pediatr Pharmacol Ther (2016) 21:27–35. doi:10.5863/1551-6776-21.1.27
128. Moyes DL, Wilson D, Richardson JP, Mogavero S, Tang SX, Wernecke J, et al. Candidalysin is a fungal peptide toxin critical for mucosal infection. Nature (2016) 532:64–8. doi:10.1038/nature17625
129. Zepp F. Principles of vaccine design-lessons from nature. Vaccine (2010) 28(Suppl 3):C14–24. doi:10.1016/j.vaccine.2010.07.020
130. Aaby P, Roth A, Ravn H, Napirna BM, Rodrigues A, Lisse IM, et al. Randomized trial of BCG vaccination at birth to low-birth-weight children: beneficial nonspecific effects in the neonatal period? J Infect Dis (2011) 204:245–52. doi:10.1093/infdis/jir240
131. Biering-Sorensen S, Aaby P, Napirna BM, Roth A, Ravn H, Rodrigues A, et al. Small randomized trial among low-birth-weight children receiving bacillus Calmette-Guerin vaccination at first health center contact. Pediatr Infect Dis J (2012) 31:306–8. doi:10.1097/INF.0b013e3182458289
132. Aaby P, Martins CL, Garly ML, Bale C, Andersen A, Rodrigues A, et al. Non-specific effects of standard measles vaccine at 4.5 and 9 months of age on childhood mortality: randomised controlled trial. BMJ (2010) 341:c6495. doi:10.1136/bmj.c6495
133. Benn CS, Netea MG, Selin LK, Aaby P. A small jab––a big effect: nonspecific immunomodulation by vaccines. Trends Immunol (2013) 34:431–9. doi:10.1016/j.it.2013.04.004
134. Kleinnijenhuis J, Quintin J, Preijers F, Joosten LA, Ifrim DC, Saeed S, et al. Bacille Calmette-Guerin induces NOD2-dependent nonspecific protection from reinfection via epigenetic reprogramming of monocytes. Proc Natl Acad Sci U S A (2012) 109:17537–42. doi:10.1073/pnas.1202870109
135. Kleinnijenhuis J, Quintin J, Preijers F, Joosten LA, Jacobs C, Xavier RJ, et al. BCG-induced trained immunity in NK cells: role for non-specific protection to infection. Clin Immunol (2014) 155:213–9. doi:10.1016/j.clim.2014.10.005
136. Garcia-Valtanen P, Guzman-Genuino RM, Williams DL, Hayball JD, Diener KR. Evaluation of trained immunity by beta-1, 3 (d)-glucan on murine monocytes in vitro and duration of response in vivo. Immunol Cell Biol (2017) 95:601–10. doi:10.1038/icb.2017.13
Keywords: Candida, candidemia, candidiasis, vaccine, trained immunity, opportunistic infections, immunocompromised patients
Citation: Tso GHW, Reales-Calderon JA and Pavelka N (2018) The Elusive Anti-Candida Vaccine: Lessons From the Past and Opportunities for the Future. Front. Immunol. 9:897. doi: 10.3389/fimmu.2018.00897
Received: 15 February 2018; Accepted: 11 April 2018;
Published: 27 April 2018
Edited by:
Steven Templeton, Indiana University School of Medicine-Terre Haute, United StatesReviewed by:
Jeniel E. Nett, University of Wisconsin-Madison, United StatesRebecca Drummond, National Institutes of Health (NIH), United States
Copyright: © 2018 Tso, Reales-Calderon and Pavelka. This is an open-access article distributed under the terms of the Creative Commons Attribution License (CC BY). The use, distribution or reproduction in other forums is permitted, provided the original author(s) and the copyright owner are credited and that the original publication in this journal is cited, in accordance with accepted academic practice. No use, distribution or reproduction is permitted which does not comply with these terms.
*Correspondence: Norman Pavelka, bm9ybWFuX3BhdmVsa2EmI3gwMDA0MDtpbW11bm9sLmEtc3Rhci5lZHUuc2c=
†These authors have contributed equally as first authors.