- Cancer Sciences Unit, Faculty of Medicine, University of Southampton, Southampton, United Kingdom
Head and neck cancer (HNC) is a heterogeneous group of squamous cell cancers that affect the oral cavity, pharynx, and larynx. Worldwide, it is the sixth most common cancer but in parts of Southern and South-East Asia, HNC is one of the most common cancers. A significant proportion of HNC is driven by human papillomavirus (HPV) infection, whereas HPV-independent HNC is associated with alcohol, smoking, and smokeless tobacco consumption. Here, we review the past and present experience of targeting HNC with vaccination focusing on HPV-derived antigens as well as non-viral antigens for HPV-negative HNC. Novel therapeutic approaches for HNC will focus not only on effective vaccine platforms but will also target the stroma-rich immunosuppressive microenvironment found in those tumours.
Introduction
Head and neck cancer (HNC) is a heterogeneous group of squamous cell cancers that affect the oral cavity, pharynx, and larynx. Overall, it is the sixth most common cancer worldwide with an annual estimated incidence of 550,000 cases and around 300,000 deaths (1–3). In parts of Southern and South-East Asia, HNC is one of the most common cancers, and the actual incidence in these developing countries is probably underestimated (1, 4, 5). While the aetiology of HNC is usually associated with smoking and alcohol, a significant subset of oropharyngeal cancers is driven by human papillomavirus (HPV) infection, and these cancers account, at least in part, for the significant increase in HNC in recent years (3, 6, 7).
Combinations of surgery, chemotherapy, and radiotherapy form the standard current first-line treatment regimens for HNC. But despite improvements, these are associated with significant morbidity and a relatively static 5-year survival rate of around 40–50% (1). HPV-positive HNCs have a better prognosis than HPV-negative HNC. Recent clinical trials have demonstrated a clear survival advantage in advanced head and neck squamous cell carcinoma patients treated with immune checkpoint blockade [for review, see Ref. (8)]. In a recent KEYNOTE 012 trial, treating HNC patients with anti-PD1 produced an overall response rate of 24.8% (9). Most patients (around 80%), however, do not respond to checkpoint inhibitor monotherapy aimed to boost pre-existing anti-tumour immune responses. The focus is now on induction of anti-tumour immune responses using cancer vaccines.
HPV-Positive Versus HPV-Negative HNC
The incidence of HNC has risen dramatically since the later 1970s and this has been linked to HPV infection. Around 25–50% of HNCs are HPV-driven with a higher percentage in developed countries (10–14). This percentage is expected to increase in coming years due to many patient cohorts being infected before prophylactic vaccination against HPV started. HPV-positive HNC predominantly tend to be restricted to the oropharynx, conversely most oropharyngeal cancers are reportedly HPV-positive (11).
Human papillomavirus is an asymptomatic, sexually transmitted DNA virus that infects squamous epithelium via micro abrasions which expose the deeper basal epithelial cells (15). Most of HPV-positive HNC (90%) are driven by high-risk HPV16 in contrast to 70% of cervical cancers that are linked to either HPV16 or 18 (11, 13, 16, 17). Other high-risk types involved include HPV 18, 31, and 33. The HPV genome encodes eight genes which are expressed early (E1, E2, E4, E5, E6, and E7) or late (L1 and L2) in the virus life cycle. E6 and E7 are the first viral proteins expressed following infection (15). They inhibit tumour suppressors p53 and pRb, respectively, resulting in uncontrolled host DNA synthesis and cell division; the first step towards malignant transformation (16). E2 protein differentially regulates E6/E7 expression through control of their transcription (18, 19). E5 is known to play an anti-apoptotic role and is thought to contribute to the early stages of oncogenesis (20, 21) by cooperating with E6 and E7 to immortalize cells (22). E5 is not necessary for the maintenance of the transformed phenotype and is often lost. L1 and L2 are structural proteins and form the viral capsid required for infectious viral particles (23).
For HPV-negative HNC incidence, habitual and cultural factors play a major role. In high-income countries, smoking and alcohol [70 and 30%, respectively, or 80% combined (24)] contribute, while in developing countries of Southern and South-Central Asia, HNC and in particular oral squamous cell carcinoma (OSCC), are primarily linked to smokeless tobacco and paan (1). Chewing of paan or betel quid has been strongly attributed to both OSCC and oral premalignancy (25). Besides tobacco, areca nut included in betel quid is also a known carcinogen and the mixture of tobacco, areca nut, and slaked lime forms a potent carcinogenic combination.
HPV-negative HNC also differs from HPV-positive genetically, and common genetic alterations which lead to inactivation of cell-cycle suppressors p53 and p16 and amplification of CCND1 (cyclin D) have been found in the HPV-negative HNC subset. Further alterations in the genes associated with smoking such as those involved in oxidative stress CUL3, KEAP1, and NFE2L2 are also associated with the HPV-negative subset (26–28).
Prophylactic Vaccination Against HPV
Several prophylactic vaccines including Cervarix, Gardasil® and more recently Gardasil®9 have been approved by the FDA to protect from HPV infection as well as HPV-associated diseases such as genital warts and cancer (Figure 1A) (29–32). The prophylactic effect specifically on HNC is assumed without relevant epidemiological studies available at present.
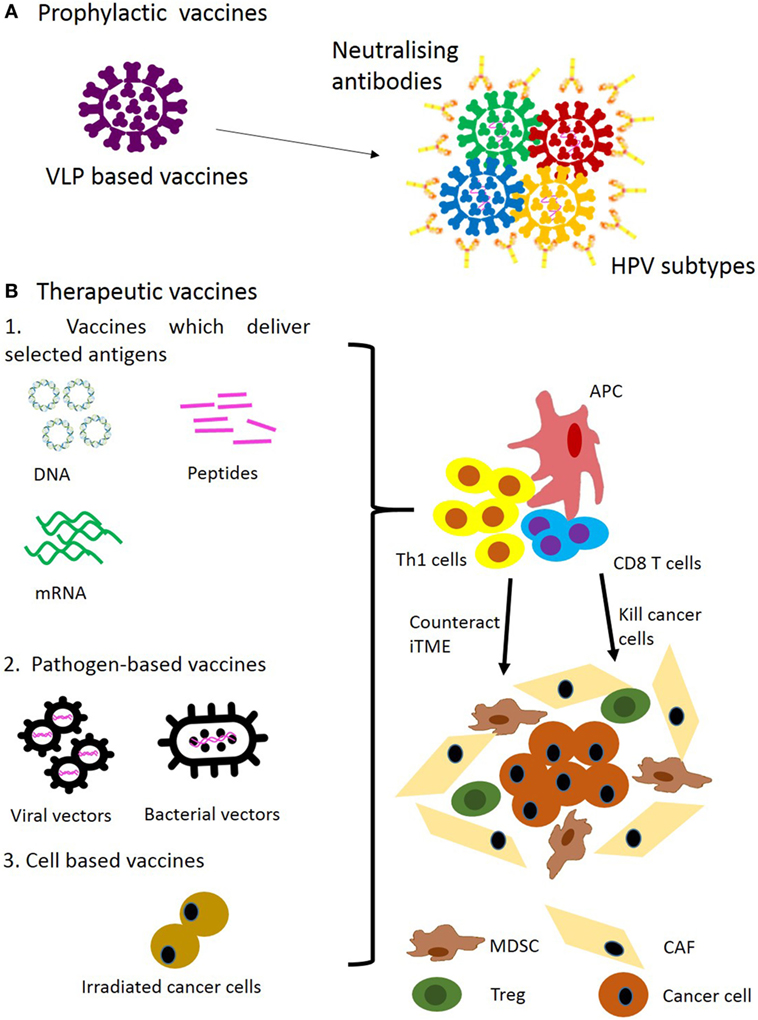
Figure 1. Approaches for prophylactic (A) and therapeutic (B) vaccination for head and neck cancer. Abbreviations: VLP, virus-like particle; iTME, immunosuppressive tumour microenvironment; MDSC, myeloid-derived suppressor cell; Treg, regulatory T cell; CAF, cancer-associated fibroblast.
These vaccines are based on virus-like particles (VLPs) consisting of different HPV capsid proteins L1 (33). For example, Gardasil consists of VLP derived from genital warts-inducing HPV6 and 11, and oncogenic strains of HPV16 and 18. One VLP is made of one type L1 molecule. When L1 is expressed using recombinant protein expression systems it self-assembles into VLPs in vitro (34–36). Superior properties of VLPs in induction of antibody are largely accounted for by their multimeric structure, and their ability to stimulate naive B cells has been demonstrated (37). Prophylactic HPV vaccines target the viral infection itself by inducing neutralising antibody and are effective in preventing HPV-induced malignancies but are not effective in treating them (38). The vaccine target L1 is not expressed during the oncogenic process. Hence, antigens expressed in the tumour have to be targeted by therapeutic vaccination.
Therapeutic Cancer Vaccines
Target Antigens
For HPV-positive cancers, the expressed viral antigens are available. For HPV-negative cancers, other antigens have to be considered. Cancer antigens can be broadly classed into two categories: tumour-specific antigens (TSAs) and tumour-associated antigens (TAAs). Here, we describe TSAs as proteins only expressed in cancer cells and mutated self-proteins (neoepitopes), and TAAs as unmutated self-proteins such as glycosylated proteins MUC1 and CEA or cancer testis antigens (CTAs) (39). TSAs often generate strong immune responses, but are comparatively less available than TAAs. TAAs are generally well conserved in populations, but tend to generate a weaker immune response (40, 41).
To cover antigens which have been targeted to date, current clinical trials for HNC were queried at the NIH ClinicalTrials.gov database utilising “Head and Neck Cancer” or “Oropharyngeal Cancer” or “Oral Cancer” as the disease, and “Vaccine” as the search string, yielding 55 studies. Terminated, withdrawn, suspended trials as well as trials with unknown recruitment statuses were excluded and the results are summarised in Table 1. The WHO International Clinical Trials Registry Platform was also queried with the same search strings yielding two additional trials not included on the NIH ClinicalTrials.gov database, but registered on the Japanese UMIN-Clinical Trials Registry (UMIN000008379, UMIN000000976).
Viral Antigens
Viral proteins are considered to be good targets since they are foreign, and hence, the available T-cell repertoire has not been subjected to central tolerance. Most vaccination strategies for HNC target the HPV-positive subset where HPV antigens can be used. HPV E6 and E7 play a critical role in carcinogenesis of HNCs, similar to ano-genital cancers. During malignant transformation when HPV frequently integrates into the host genome, E6 and E7 are thought to be the only proteins expressed and hence have been targeted by many types of vaccines. Vaccines against these antigens have demonstrated efficacy in HPV-induced cervical dysplasia (42–46) and are currently in clinical trials for both cervical cancer (e.g., NCT02128126) and HNC (Table 1).
Other potential targets are E2 and E5. E2 has been successfully targeted in ano-genital intraepithelial lesions (47). In HNC, E2 is not always lost, and can be retained in episomal HPV DNA (48) (and unpublished data from our lab). A number of vaccines targeting E5 are in preclinical development (49–52). No clinical data on targeting E2 and E5 in HNC are currently available.
HPV-negative HNC lacks the immunogenic HPV viral proteins of HPV-associated HNC and appears less responsive to current treatments (53). A viral target which may be present in HPV-negative cases is EBV, which is strongly associated with nasopharyngeal cancer (54), though only around 6–21% of HNC cases express EBV RNA (55, 56). Vaccines targeting EBV are currently in phase I clinical trials (Table 1; NCT01147991), and appear safe and well tolerated, inducing only grade I/II adverse events, while reporting increased circulating CD4 cells and antigen-specific T cell responses (57, 58).
Neoepitopes
Despite earlier reports that HPV-negative HNC had a greater mutation rate than HPV-positive (59), more recent reports have found no significant difference to the mutation rate as a result of HPV status (26, 27). However, these reports do find significant differences in the mutational spectrum based on HPV status, which influence vaccine-targetable mutations. Targeting p53 and RAS is more likely to benefit HPV-negative cases, as these proteins are mutated in HPV-negative cases, but degraded in HPV-positive cases (60). Early trials targeting mutated p53 or RAS have been completed (61). The RAS phase II trial was completed in 2007, but no results have been reported to date (Table 1; NCT00019331).
Conventional mutation targeting in cancer therapy focuses upon driver mutations, but in the last decade has arisen a view that other mutations may be relevant and make for potential vaccine targets (62, 63). Advances in immunotherapeutics and bioinformatics in recent years have increased the practicality of targeting these neoantigens via vaccination. However, as each case exhibits its own unique mutanome (64), each vaccine must be created for the specific individual, making it expensive and time consuming. Recent studies reported around 100 days are required for production and analytical testing (NCT02035956, NCT01970358) (65, 66). Despite the current great expense of both time and money, early results suggest great efficacy from targeting neoantigens (65, 66).
Tumour-Associated Antigens
Well-characterised antigens including MUC1 and CEA have demonstrated immunogenicity in patients and their potential is being translated into clinical efficacy (67, 68). In HNC, phase I/II trials targeting MUC1 are on-going (Table 1; NCT02544880), while trials targeting CEA (Table 1; NCT00924092, NCT00027534) have been completed but have yet to report results. The p53 phase I trial targeting wt p53 T-cell epitopes completed with modest improvement to 2-year disease-free survival (DFS) [Table 1; NCT00404339 (69)], but has yet to progress to a phase II trial. A phase I trial targeting Survivin-2B has been performed in oral cancer (Table 1; UMIN000000976), but demonstrated low efficacy (70). The NANT vaccine (Table 1; NCT03169764) is a novel combination immunotherapy combining metronomic chemo-radiotherapy with vaccines targeting well-established and molecularly confirmed TAAs, and off-the-shelf NK cell therapy. This experimental therapy is part of the Cancer Breakthroughs 2020 global initiative.
Cancer testis antigens are a class of TAAs that make for promising vaccine targets. While there is evidence of central tolerance for CTAs (71), CTA expression in the periphery is normally restricted to healthy male germ cells, immune privileged cells lacking MHC I (72). Thus, CTAs are only presented to the immune system in the periphery by cancer cells (73), and thus frequently demonstrate immunogenicity (74–76).
Using either SEREX or TIL-derived T cells, many CTAs have been described over the past decades, most prominently the MAGE family, BAGE family, SSX family, PRAME, and NY-ESO1 (77–79). A recent analysis of HNC selected several potential CTA targets for further preclinical study, although they did not differentiate between HPV-associated and HPV-negative cases (80). A phase I clinical trial targeting MAGE-A3 and HPV antigens in HNC is on-going (Table 1; NCT00257738) (81).
Novel CTAs including LY6K, CDCA1, and IMP3 have been identified through genome wide microarray analysis of various cancer tissues (82–84). A multivalent vaccine targeting HLA-A24 restricted LY6K, CDCA1, and IMP3-derived peptides recently was tested in phase I and phase II clinical trials in oesophageal cancer (85, 86), and significantly improved DFS in HLA-A24 cases was reported. This success encouraged targeting of these antigens in HNC (Table 1; UMIN000008379) (87), which increased overall survival (OS) when administered to HLA-A24 patients with advanced refractory HNC (HPV-negative with the exception of one patient) and correlated with peptide specific CTL responses.
Immune Mechanisms for Cancer Attack
Most target antigens in HNC including HPV antigens, neoepitopes as well as the majority of TAA are intracellular antigens. Intracellular antigens are generally presented as 8-11-mer peptides bound to MHC I on cancer cells and these are targeted by CD8 cytotoxic T cells (CTLs). CTLs are powerful effector cells which directly kill target cells via a variety of mechanisms including perforin/granzyme and Fas-mediated attack. For induction of long-lasting CTLs, CD4 T helper (Th)1 cells must also be co-induced (88). These provide T-cell help via dendritic cells (DC)-licensing by binding to 12-15mer or longer peptides presented in the context of MHC II on DCs which leads to DC activation (89, 90). These Th1 subsets do not have to be specific for the target cancer antigen. Approaches for recruiting Th1 cells specific for foreign antigens have been extensively explored in the development of cancer vaccines (91–94). The advantage of this approach is that these Th cells escape tolerogenic mechanisms and are therefore available to provide help to CTLs specific for cancer-derived antigenic peptides [for review, see Ref. (95)]. Tetanus-derived Dom helper sequence has been used to recruit CD4 T cell for induction of CD8+ CTLs using DNA vaccine (96). A recent phase II clinical data demonstrated this approach led to induction of CD8+ T-cells response to CEA detected post-vaccine with indication of clinical benefits (67).
For effective cancer attack, Th1 cells that play not only a helper role to CTLs but a more direct role in anti-tumour immunity are important (97–99). Those specific for the target antigen Th1 cell subsets may be involved in recruitment of tumouricidal macrophages or reprogramming of the tumour microenvironment (100, 101). In HPV-associated cancer, targeting co-induction of broad CD4 and CD8 T-cell responses correlated with vaccine efficacy (44). This is in keeping with the data obtained in other solid tumours (65, 66).
Vaccine Platforms
Delivering antigenic epitopes in an immunogenic context which leads to the induction of a durable T-cell response is the goal. The available vaccine platforms are illustrated in Figure 1B. DNA and RNA vaccines encoding selected tumour antigens or synthetic long peptides (SLPs) vaccines co-delivering CD4 and CD8 epitopes have recently been highlighted as optimal cancer vaccine modalities (97). These focus on delivery of selected target antigens without co-delivering the backbone-encoded antigens as in the case of pathogen-derived bacterial or viral vectors. The latter often generate strong pathogen-derived CD8 epitopes and can focus the immune response on the vector itself (96). Nevertheless, several viral and bacterial vaccines have demonstrated induction of CD8 responses against dysplastic disease and cancer together with clinical efficacy (47, 68).
DNA Vaccines
DNA vaccines represent a simple approach of directly injecting a plasmid DNA encoding one or more antigens driven by a eukaryotic promoter. Not only is the antigen made directly in the body but the DNA backbone also acts as an immunological adjuvant (102). Multiple innate sensors for plasmid DNA have been identified including endosomal toll-like receptor (TLR) 9 as well as several cytosolic sensors DAI, AIM2, cGAS-STING, and others (103). Flexibility, simplicity of preparation, stability, and safety are the advantages. However, low immunogenicity in patients has been highlighted in early clinical trials (104). The situation improved upon combination of DNA vaccine injection and in vivo electroporation [EP, for review, see Ref. (105)]. In vivo EP increased cellular DNA uptake leading to generation of more antigen available for immunisation and potentially made DNA more visible to the cytosolic innate sensors. This led to significant increase in immunogenicity. Combination of DNA and EP induced durable antibody and T-cell responses in cancer patients (42, 67, 104, 106). Other methods of DNA delivery including liposomes, tattooing and cationic polymers have also been also investigated (107, 108).
For targeting of HPV, oncogenes E6 and E7 by DNA vaccines modifications have been made to their sequences to prevent E6 and E7 binding to p53 and pRb, respectively (42, 109, 110). Most HPV-targeting DNA vaccines so far have been trialled in the setting of cervical intraepithelial neoplasia (CIN). In the recent phase IIb clinical trial, VGX-3100 DNA vaccine encoding E6 and E7 in combination with DNA vaccine encoding IL-2 administered i.m. with EP has shown promising clinical results in women with HPV16- and 18-associated CIN2/3. Robust T-cell responses were induced and regression of premalignant lesions was demonstrated in 50% of vaccinated women (42). VGX-3100 was subsequently moved to the HNC setting where it was also delivered with EP plus DNA vaccine encoding IL-12 (the combined treatment defined as INO-3112; Table 1; NCT02163057). Initial results from the study were very promising. HPV E6/E7-specific antibody was successfully generated in four of the five HNC patients analyzed. Increased HPV-specific cellular responses were observed in nine out of 10 evaluable patients by ELISPOT. Seven of eight evaluable patients had HPV-specific granzyme/perforin positive CD8 T cells by flow cytometry (111). A phase I/II trial to assess the vaccine (now called MEDI-0457, MedImmune) safety and anti-tumour efficacy in combination with PD-L1-blocking mAb Durvalumab is now recruiting HPV-positive HNC patients (Table 1; NCT03162224).
DNA vaccine targeting HPV16 E7, pNGVL4a-CRT/E7 (detox), based on E7-calreticulin (CRT) fusion demonstrated the ability to enhance MHC I presentation and exhibited an anti-angiogenic effect (112). In a preclinical study, E7-specific antibody and T-cell responses were generated with protection from the TC-1 tumour challenge (113). A pilot clinical study using pNGVL4a-CRT/E7 (detox) for the treatment of patients with HPV16-associated CIN2/3 was recently conducted (114). EP was not used in this study but one arm investigated the particle-mediated epidermal delivery using a needle free ND10 delivery system. The results demonstrated mild but manageable toxicity predominantly localised to the injection site but only a small increase in systemic T-cell responses was observed with no increase in regression above the control. A phase I trial assessing safety and feasibility of this DNA vaccine in combination with cyclophosphamide in HPV16-associated HNC patients has been terminated (Table 1).
Immunogenicity and efficacy of a novel linear closed end DNA vaccine, doggybone (db) DNA (dbDNA™, Touchlight Genetics), have recently been demonstrated in the HPV E6 and E7 tumour model (115). dbDNA™ vaccine was developed using a bacteria-free manufacturing platform which relies on bacteriophage Phi29 polymerase for amplification. Minimal purification is required and safety is improved because of exclusion of antibiotic-resistant genes irrelevant for this platform. dbDNA™ vaccine operated through STING-mediating pathways but was independent of TLR9 recognition. Importantly, HPV16 E6 and E7 dbDNA™ vaccine and conventional plasmid DNA delivered with EP generated similar levels of CD4 and CD8 T cells as well as antibody. dbDNA™ was also able to suppress established TC-1 tumours similar to plasmid DNA. This novel DNA vaccine represents a promising alternative to a plasmid DNA vaccine for targeting of HPV E6 and E7 antigens.
mRNA Vaccines
mRNA vaccines are becoming increasingly attractive in recent years. They can accumulate at high concentration in the cytoplasm ensuring high antigen expression. mRNA is a natural ligand for TLR3, TLR7/8, and several cytosolic sensors (i.e., RIG-I, MDA5), which induce innate immune response to enhance vaccine efficacy [for review, see Ref. (116)]. For in vivo delivery, mRNA is complexed with a lipid carrier that protects from degradation as well as targets DCs (117). The efficacy of a first-in-human personalised mRNA vaccine targeting patients’ mutanome in melanoma patients has been reported recently (65). T-cell responses against multiple mutanome-derived neoepitopes were induced in all patients. Four out of five patients with progressing metastasis at the start of vaccination demonstrated either a partial or a complete clinical response after vaccination. Interestingly, one patient benefited from vaccination followed by anti-PD1 mAb. A phase I/II clinical trial using an mRNA vaccine targeting HPV16 E6 and E7 has recently started recruiting at our institution (University of Southampton, led by Prof C. Ottensmeier and Dr E. King; NCT03418480). The vaccine will be given to patients with HPV-positive HNC intradermally either alone or in combination with anti-CD40 costimulatory antibody.
Peptide Vaccines
Peptide-based vaccines are safe and easy to produce, but they are also expensive and poorly immunogenic by themselves. They are often CD8+ epitopes predicted for a particular HLA allele or include long single or overlapping peptides which often contain both CD8 and CD4 epitopes. The latter approach circumvents HLA restriction issues. Unlike DNA and RNA vaccines peptides do not carry “inbuilt” adjuvants. The efforts to enhance their immunogenicity have focused on combining with appropriate adjuvants. MF59®, emulsion of squalene oil approved in both Europe and USA, has been used in earlier trials (97). A number of clinical trials have used SLPs targeting HPV antigens combined with oil-in-water adjuvants for treatment of CIN and vulvar neoplasia which resulted in regression of premalignant lesions (43–46). However, this approach has not been successful when tested in recurrent cervical cancer with low T-cell responses and no survival benefit (118). This failure highlighted the need to use more potent adjuvants and combinational therapeutic approaches to overcome the tumour microenvironment (119).
Several peptide-based vaccines against HPV-associated HNCs are now in clinical trials. A phase I/II trial to assess safety and efficacy of a short peptide-based vaccine targeting HPV16 E7 (11–19), in combination with low dose of cyclophosphamide intending to deplete regulatory T cells, has started recently in HLA-A2 patients with incurable HPV16-associated oropharyngeal, cervical, and anal cancer (Table 1; NCT02865135). In another clinical trial, patients with advanced HPV-associated cancers were vaccinated weekly with a SLP derived from p16 (27, 37–62), the tumour suppressor induced as a result of HPV-linked transformation, after the completion of a standard treatment. The vaccine containing both CD8 and CD4 epitopes was emulsified with Montanide™ ISA-51 VG (oil-in-water adjuvant, SEPPIC). Both cellular and humoral responses to the peptide were induced with no unexpected serious adverse reactions. Out of 14 evaluated patients, nine had stable disease as their best overall response and five patients developed progressive disease [Table 1; NCT01462838 (120)]. A subsequent on-going trial using the same vaccine has been evaluating different routes of vaccination, i.e., subcutaneous and intradermal (Table 1; NCT02526316). Two phase II clinical trials using 13 HPV16 E6/E7 overlapping SLPs in combination with anti-PD-1 antibody (Nivolumab) or in combination with anti-CD137 immuno-stimulatory antibody (Utomilumab) have been initiated to treat patients with HPV16-associated HNC as well as other HPV-associated malignancies (ISA101/ISA101b; Table 1; NCT02426892 and NCT03258008) (119). A phase I trial using two HPV16 E6 SLPs (ISA201) together with TLR1/2 agonist adjuvant Amplivant® (ISA pharmaceutical), for HPV16-positive tumours and premalignant lesions has also been initiated [Table 1; NCT02821494 (121)].
A multivalent vaccine targeting HLA-A24 restricted short peptides from three CTAs (LY6K, CDCA1, and IMP3) in combination with IFA injected subcutaneously has recently cleared phase II clinical trials in HNC patients in Japan [Table 1; UMIN000008379 (87)]. The vaccine increased OS when administered to HLA-A24 patients, which was correlated to peptide specific CTL responses. Interestingly, those patients that demonstrated response to all three peptides had extended OS versus those who responded to one or two peptides only.
Viral and Bacterial Vector-Based Vaccines
Viral vector-based vaccines employ attenuated viruses that deliver antigen of interest in the infected cells. Alphaviruses, adenoviruses, and vaccinia viruses are the examples that have been explored to deliver HPV-associated antigens.
Replication-deficient alphaviruses including Semliki Forest virus (SFV) and Venezuelan equine encephalitis virus (VEE) have been demonstrated to be safe [for review, see Ref. (122)]. These RNA viruses preferentially infect APCs and are able to efficiently activate the adaptive immune system [for review, see Ref. (123)]. SFV- and VEE-based vaccines against HPV16 E6/E7 have demonstrated the ability to induce specific CTLs that can kill HPV16 E6/E7-expressing tumour cells in vitro and clear tumours in mouse models (124–126), including in the HLA-A*0201 transgenic mice (127, 128).
The first clinical trial of a recombinant vaccinia virus targeting HPV was conducted more than 20 years ago. The TA-HPV vaccine was based on a live vaccinia virus and was engineered to express E6 and E7 proteins from HPV16 and 18. Two patients with advanced cervical cancer remained tumour free 15 and 21 months after vaccination, in one of them an HPV-specific T-cell response was also induced (129). Two more clinical trials using the same vaccine to treat HPV-associated advanced cervical cancer and vulval/vaginal intraepithelial neoplasia had been reported with partly successful results, but also some side effects manifesting as erythema and swelling followed by ulceration with scab formation at the site of vaccination (130, 131). Safety concerns related to the use of live vaccinia virus prompted the development of vaccines based on the attenuated virus, i.e., modified vaccinia virus Ankara (MVA) (132, 133). MVA also preferentially infects APCs (134) and through recognition of its viral DNA by TLR9 and cytosolic DNA sensors is able to activate APCs leading to effective activation of T-cell immunity. The safety as a result of restricted replication has been demonstrated in many clinical trials (132).
Efficacy of an MVA-based vaccine encoding modified HPV16 E6 and E7 together with IL-2 TG4001 (Transgene) was demonstrated in patients with HPV16-related CIN2/3. In 7 out of 10 patients who were evaluated as clinical responders, cytological and colposcopic regression together with HPV16 mRNA clearance was observed (135). A phase I/II clinical trial assessing TG4001 in HPV16-associated HNC patients has recently started recruiting (Table 1; NCT03260023).
A well-characterized attenuated facultative intracellular bacterium Listeria monocytogenes (Lm) incorporating a non-toxic version of the listerionlysin O (LLO) as adjuvant has been utilised to target HPV16 E7 oncoprotein (Lm-LLO-E7 also known as AXAL, ADXS11-001) [(136); for review, see Ref. (137)]. Several clinical trials using this vaccine in cervical and anal cancers are on-going, including one which progressed to phase III last year in women with high-risk locally advanced cervical cancer (NCT02853604). With regard to HNC, one phase I clinical trial in oropharyngeal cancer was terminated in 2016 as one patient suffered a dose limitation toxicity (NCT01598792). Two more phase II trials using the vaccine in combination with anti-PD-L1 mAb (Table 1; NCT02291055) or surgery (Table 1; NCT02002182) are still active and recruiting. The latter trial has recently reported specific T-cell responses in the blood and increased T-cell infiltration in the tumour in five out of eight and four out of eight patients, respectively (138).
Cellular Vaccines
Using autologous tumour cells as vaccines ensures that patients are vaccinated with cells containing the same tumour antigens that their tumour expresses saving time and effort needed to identify TSAs. Irradiated cells are used but undesired immune responses are still a potential safety concern. To enhance immunogenicity cells genetically modified to express costimulatory molecules, TLR ligands or cytokines have been utilised (139). Phase I/II trials are underway for personalised HNC vaccines Allovax and MVX-ONCO-1, utilising allogeneic tumour cells as a source of antigen (Table 1; NCT02999646, NCT01998542, NCT02624999). MVX-ONCO-1 contains irradiated autologous tumours cells expressing GM-CSF combined with encapsulated cellular technology that allows continuous supply of GM-CSF (140). It has demonstrated reasonable safety in a phase I trial for solid tumours, with no systemic serious adverse events (NCT02193503) (141). AlloVax™ is Charperone Rich cell lysate combined with AlloStim™ cells which are allogeneic Th1 effector cells [Allostim, Immunovative Therapies Ltd. (142)].
The Role of the Immunosuppressive Tumour Microenvironment: Cancer-Associated Fibroblasts (CAFs)
Several immunosuppressive immune subsets have been found in HNC including tumour-associated macrophages, myeloid-derived suppressor cells, and regulatory B and T cells. The role of these have been reviewed elsewhere (143, 144), but it is clear that vaccination must induce the correct immune milieu for therapy to be effective. Similarly, we have identified several features of the tumour microenvironment, including tumour cell glycolysis/hypoxia and a CAF-rich stroma that are associated with “immune cold” HNC (145, 146). It has been suggested that CAFs suppress T-cell infiltration into cancers, and also through secretion and activation of TGF-β, modulate multiple types of immune cells towards a more suppressive phenotype, including tolerization of CD4 T cells and promotion of a regulatory T cell phenotype. Tumours with this type of stromal response may not be effectively targeted by vaccination; it is possible, however, that such evasion mechanisms could be targeted as part of a vaccination strategy; for example, we have shown recently that CAFs can be specifically targeted by inhibiting the NADPH oxidase, NOX4 (146).
Concluding Remarks
Incidence of both HPV-positive and -negative HNC is on the increase. The trend is unlikely to change at least in the near future, particular for HPV-independent HNC, especially OSCC, where the habitual and cultural causes are unlikely to disappear.
Human papillomavirus targets E6 and E7 are considered to be less challenging because of their foreign nature. Their targeting by DNA, peptides, and other vaccines has already demonstrated clinical efficacy in HPV-driven dysplasia. These vaccines are now in clinical trials for HPV-driven cancers including HNC. On the contrary, HPV-independent HNC has received less attention largely because such targets have not been available. A number of interesting antigenic targets has started coming through; these include personalised mutanome-derived neoepitopes but also novel TAAs (87). The mutanome-based approach has started demonstrating clinical efficacy but it is unlikely to have an impact on the disease in the developing parts of the world. Therefore, the focus is on TAAs.
More affordable vaccine modalities such as DNA vaccines combined with a simple in vivo delivery are promising. However, these will still need to be developed within a strategy that overcomes a suppressive tumour microenvironment and further work is required to develop these therapeutic approaches.
Author Contributions
CW, JD, RS, and NS wrote sections on target cancer antigens, immune mechanisms, and vaccine platforms. CP and GT wrote sections on HNC and tumour microenvironment. All authors contributed to discussion of the manuscript.
Conflict of Interest Statement
The authors declare that the research was conducted in the absence of any commercial or financial relationships that could be construed as a potential conflict of interest.
Funding
This work was supported by Medical Research Council, UK grants MR/PO13414/1 and MR/P024351/1.
References
1. Jemal A, Bray F, Center MM, Ferlay J, Ward E, Forman D. Global cancer statistics. CA Cancer J Clin (2011) 61(2):69–90. doi:10.3322/caac.20107
2. Warnakulasuriya S. Living with oral cancer: epidemiology with particular reference to prevalence and life-style changes that influence survival. Oral Oncol (2010) 46(6):407–10. doi:10.1016/j.oraloncology.2010.02.015
3. Chaturvedi AK, Anderson WF, Lortet-Tieulent J, Curado MP, Ferlay J, Franceschi S, et al. Worldwide trends in incidence rates for oral cavity and oropharyngeal cancers. J Clin Oncol (2013) 31(36):4550–9. doi:10.1200/JCO.2013.50.3870
4. Mishra A, Meherotra R. Head and neck cancer: global burden and regional trends in India. Asian Pac J Cancer Prev (2014) 15(2):537–50. doi:10.7314/APJCP.2014.15.2.537
5. Chye GLC, Rampal S, Yahaya H, Latif AZA, Jalil AA, Bustam A, et al. Cancer Incidence in Peninsular Malaysia, 2003–2005. Kuala Lumpur: National Cancer Registry (2008). p. 38–176.
6. Kreimer AR, Clifford GM, Boyle P, Franceschi S. Human papillomavirus types in head and neck squamous cell carcinomas worldwide: a systematic review. Cancer Epidemiol Biomarkers Prev (2005) 14(2):467–75. doi:10.1158/1055-9965.EPI-04-0551
7. Hashibe M, Brennan P, Chuang SC, Boccia S, Castellsague X, Chen C, et al. Interaction between tobacco and alcohol use and the risk of head and neck cancer: pooled analysis in the International head and neck cancer epidemiology consortium. Cancer Epidemiol Biomarkers Prev (2009) 18(2):541–50. doi:10.1158/1055-9965.EPI-08-0347
8. Szturz P, Vermorken JB. Immunotherapy in head and neck cancer: aiming at EXTREME precision. BMC Med (2017) 15(1):110. doi:10.1186/s12916-017-0879-4
9. Chow LQM, Haddad R, Gupta S, Mahipal A, Mehra R, Tahara M, et al. Antitumor Activity of pembrolizumab in biomarker-unselected patients with recurrent and/or metastatic head and neck squamous cell carcinoma: results from the phase Ib KEYNOTE-012 expansion cohort. J Clin Oncol (2016) 34(32):3838–45. doi:10.1200/JCO.2016.68.1478
10. Franceschi S, Munoz N, Bosch XF, Snijders PJ, Walboomers JM. Human papillomavirus and cancers of the upper aerodigestive tract: a review of epidemiological and experimental evidence. Cancer Epidemiol Biomarkers Prev (1996) 5(7):567–75.
11. D’Souza G, Kreimer AR, Viscidi R, Pawlita M, Fakhry C, Koch WM, et al. Case-control study of human papillomavirus and oropharyngeal cancer. N Engl J Med (2007) 356(19):1944–56. doi:10.1056/NEJMoa065497
12. Attner P, Du J, Nasman A, Hammarstedt L, Ramqvist T, Lindholm J, et al. The role of human papillomavirus in the increased incidence of base of tongue cancer. Int J Cancer (2010) 126(12):2879–84. doi:10.1002/ijc.24994
13. Goon PK, Stanley MA, Ebmeyer J, Steinstrasser L, Upile T, Jerjes W, et al. HPV & head and neck cancer: a descriptive update. Head Neck Oncol (2009) 1:36. doi:10.1186/1758-3284-1-36
14. Schache AG, Powell NG, Cuschieri KS, Robinson M, Leary S, Mehanna H, et al. HPV-related oropharynx cancer in the United Kingdom: an evolution in the understanding of disease etiology. Cancer Res (2016) 76(22):6598–606. doi:10.1158/0008-5472.CAN-16-0633
15. Schiffman M, Doorbar J, Wentzensen N, de Sanjose S, Fakhry C, Monk BJ, et al. Carcinogenic human papillomavirus infection. Nat Rev Dis Primers (2016) 2:16086. doi:10.1038/nrdp.2016.86
16. Munoz N, Castellsague X, de Gonzalez AB, Gissmann L. Chapter 1: HPV in the etiology of human cancer. Vaccine (2006) 24(Suppl 3):S3/1–10. doi:10.1016/j.vaccine.2006.05.115
17. Rettig E, Kiess AP, Fakhry C. The role of sexual behavior in head and neck cancer: implications for prevention and therapy. Expert Rev Anticancer Ther (2015) 15(1):35–49. doi:10.1586/14737140.2015.957189
18. Bechtold V, Beard P, Raj K. Human papillomavirus type 16 E2 protein has no effect on transcription from episomal viral DNA. J Virol (2003) 77(3):2021–8. doi:10.1128/JVI.77.3.2021-2028.2003
19. McBride AA. The papillomavirus E2 proteins. Virology (2013) 445(1–2):57–79. doi:10.1016/j.virol.2013.06.006
20. Conrad M, Bubb VJ, Schlegel R. The human papillomavirus type 6 and 16 E5 proteins are membrane-associated proteins which associate with the 16-kilodalton pore-forming protein. J Virol (1993) 67(10):6170–8.
21. Borzacchiello G, Roperto F, Campo MS, Venuti A. 1st International workshop on papillomavirus E5 oncogene-a report. Virology (2010) 408(2):135–7. doi:10.1016/j.virol.2010.09.015
22. Jiang P, Yue Y. Human papillomavirus oncoproteins and apoptosis (review). Exp Ther Med (2014) 7(1):3–7. doi:10.3892/etm.2013.1374
23. Munger K, Howley PM. Human papillomavirus immortalization and transformation functions. Virus Res (2002) 89(2):213–28. doi:10.1016/S0168-1702(02)00190-9
24. Danaei G, Vander Hoorn S, Lopez AD, Murray CJ, Ezzati M; Comparative Risk Assessment Collaborating Group (Cancers). Causes of cancer in the world: comparative risk assessment of nine behavioural and environmental risk factors. Lancet (2005) 366(9499):1784–93. doi:10.1016/S0140-6736(05)67725-2
25. Goldenberg D, Lee J, Koch WM, Kim MM, Trink B, Sidransky D, et al. Habitual risk factors for head and neck cancer. Otolaryng Head Neck (2004) 131(6):986–93. doi:10.1016/j.otohns.2004.02.035
26. Seiwert TY, Zuo Z, Keck MK, Khattri A, Pedamallu CS, Stricker T, et al. Integrative and comparative genomic analysis of HPV-positive and HPV-negative head and neck squamous cell carcinomas. Clin Cancer Res (2015) 21(3):632–41. doi:10.1158/1078-0432.CCR-13-3310
27. Lawrence MS, Sougnez C, Lichtenstein L, Cibulskisl K, Lander E, Gabriel SB, et al. Comprehensive genomic characterization of head and neck squamous cell carcinomas. Nature (2015) 517(7536):576–82. doi:10.1038/nature14129
28. Beck TN, Golemis EA. Genomic insights into head and neck cancer. Cancers Head Neck (2016) 1(1):1. doi:10.1186/s41199-016-0003-z
29. US FDA. Cervarix. (2009). Available from: https://www.fda.gov/biologicsbloodvaccines/vaccines/approvedproducts/ucm186957.htm
30. US FDA. Gardasil. (2011). Available from: https://www.fda.gov/BiologicsBloodVaccines/Vaccines/ApprovedProducts/UCM094042
31. US FDA. Gardasil 9. (2014). Available from: https://www.fda.gov/biologicsbloodvaccines/vaccines/approvedproducts/ucm426445.htm
32. Frazer IH. Prevention of cervical cancer through papillomavirus vaccination. Nat Rev Immunol (2004) 4(1):46–54. doi:10.1038/nri1260
33. Lowy DR, Schiller JT. Prophylactic human papillomavirus vaccines. J Clin Invest (2006) 116(5):1167–73. doi:10.1172/JCI28607
34. Zhou J, Sun XY, Stenzel DJ, Frazer IH. Expression of vaccinia recombinant HPV 16 L1 and L2 ORF proteins in epithelial cells is sufficient for assembly of HPV virion-like particles. Virology (1991) 185(1):251–7. doi:10.1016/0042-6822(91)90772-4
35. Kirnbauer R, Taub J, Greenstone H, Roden R, Durst M, Gissmann L, et al. Efficient self-assembly of human papillomavirus type 16 L1 and L1-L2 into virus-like particles. J Virol (1993) 67(12):6929–36.
36. Scolnick EM. A vaccine to prevent cervical cancer: academic and industrial collaboration and a Lasker award. Clin Transl Immunol (2018) 7(1):e1002. doi:10.1002/cti2.1002
37. Bachmann MF, Jennings GT. Vaccine delivery: a matter of size, geometry, kinetics and molecular patterns. Nat Rev Immunol (2010) 10(11):787–96. doi:10.1038/nri2868
38. Schiller JT, Castellsague X, Garland SM. A review of clinical trials of human papillomavirus prophylactic vaccines. Vaccine (2012) 30(Suppl 5):F123–38. doi:10.1016/j.vaccine.2012.04.108
39. Coulie PG, Van den Eynde BJ, van der Bruggen P, Boon T. Tumour antigens recognized by T lymphocytes: at the core of cancer immunotherapy. Nat Rev Cancer (2014) 14(2):135–46. doi:10.1038/nrc3670
40. Bright RK, Bright JD, Byrne JA. Overexpressed oncogenic tumor-self antigens. Hum Vaccin Immunother (2014) 10(11):3297–305. doi:10.4161/hv.29475
41. Schumacher TN, Schreiber RD. Neoantigens in cancer immunotherapy. Science (2015) 348(6230):69–74. doi:10.1126/science.aaa4971
42. Trimble CL, Morrow MP, Kraynyak KA, Shen X, Dallas M, Yan J, et al. Safety, efficacy, and immunogenicity of VGX-3100, a therapeutic synthetic DNA vaccine targeting human papillomavirus 16 and 18 E6 and E7 proteins for cervical intraepithelial neoplasia 2/3: a randomised, double-blind, placebo-controlled phase 2b trial. Lancet (2015) 386(10008):2078–88. doi:10.1016/S0140-6736(15)00239-1
43. Kenter GG, Welters MJ, Valentijn AR, Lowik MJ, Berends-van der Meer DM, Vloon AP, et al. Vaccination against HPV-16 oncoproteins for vulvar intraepithelial neoplasia. N Engl J Med (2009) 361(19):1838–47. doi:10.1056/NEJMoa0810097
44. Welters MJ, Kenter GG, de Vos van Steenwijk PJ, Lowik MJ, Berends-van der Meer DM, Essahsah F, et al. Success or failure of vaccination for HPV16-positive vulvar lesions correlates with kinetics and phenotype of induced T-cell responses. Proc Natl Acad Sci U S A (2010) 107(26):11895–9. doi:10.1073/pnas.1006500107
45. de Vos van Steenwijk PJ, van Poelgeest MI, Ramwadhdoebe TH, Lowik MJ, Berends-van der Meer DM, van der Minne CE, et al. The long-term immune response after HPV16 peptide vaccination in women with low-grade pre-malignant disorders of the uterine cervix: a placebo-controlled phase II study. Cancer Immunol Immunother (2014) 63(2):147–60. doi:10.1007/s00262-013-1499-2
46. van Poelgeest MI, Welters MJ, Vermeij R, Stynenbosch LF, Loof NM, Berends-van der Meer DM, et al. Vaccination against oncoproteins of HPV16 for noninvasive vulvar/vaginal lesions: lesion clearance is related to the strength of the T-cell response. Clin Cancer Res (2016) 22(10):2342–50. doi:10.1158/1078-0432.CCR-15-2594
47. Rosales R, Lopez-Contreras M, Rosales C, Magallanes-Molina JR, Gonzalez-Vergara R, Arroyo-Cazarez JM, et al. Regression of human papillomavirus intraepithelial lesions is induced by MVA E2 therapeutic vaccine. Hum Gene Ther (2014) 25(12):1035–49. doi:10.1089/hum.2014.024
48. Venuti A, Paolini F. HPV detection methods in head and neck cancer. Head Neck Pathol (2012) 6(Suppl 1):S63–74. doi:10.1007/s12105-012-0372-5
49. Liao S, Zhang W, Hu X, Wang W, Deng D, Wang H, et al. A novel “priming-boosting” strategy for immune interventions in cervical cancer. Mol Immunol (2015) 64(2):295–305. doi:10.1016/j.molimm.2014.12.007
50. Liao SJ, Deng DR, Zeng D, Zhang L, Hu XJ, Zhang WN, et al. HPV16 E5 peptide vaccine in treatment of cervical cancer in vitro and in vivo. J Huazhong Univ Sci Technolog Med Sci (2013) 33(5):735–42. doi:10.1007/s11596-013-1189-5
51. Paolini F, Curzio G, Cordeiro MN, Massa S, Mariani L, Pimpinelli F, et al. HPV 16 E5 oncoprotein is expressed in early stage carcinogenesis and can be a target of immunotherapy. Hum Vaccin Immunother (2017) 13(2):291–7. doi:10.1080/21645515.2017.1264777
52. Venuti A, Curzio G, Mariani L, Paolini F. Immunotherapy of HPV-associated cancer: DNA/plant-derived vaccines and new orthotopic mouse models. Cancer Immunol Immunother (2015) 64(10):1329–38. doi:10.1007/s00262-015-1734-0
53. Whang SN, Filippova M, Duerksen-Hughes P. Recent progress in therapeutic treatments and screening strategies for the prevention and treatment of HPV-associated head and neck cancer. Viruses (2015) 7(9):5040–65. doi:10.3390/v7092860
54. Prabhu SR, Wilson DF. Evidence of Epstein-Barr virus association with head and neck cancers: a review. J Can Dent Assoc (2016) 82:g2.
55. Deng ZY, Uehara T, Maeda H, Hasegawa M, Matayoshi S, Kiyuna A, et al. Epstein-Barr virus and human papillomavirus infections and genotype distribution in head and neck cancers. PLoS One (2014) 9(11). doi:10.1371/journal.pone.0113702
56. Turunen A, Rautava J, Grenman R, Syrjanen K, Syrjanen S. Epstein-Barr virus (EBV)-encoded small RNAs (EBERs) associated with poor prognosis of head and neck carcinomas. Oncotarget (2017) 8(16):27328–38. doi:10.18632/oncotarget.16033
57. Si YF, Deng ZX, Lan GP, Du HJ, Wang YL, Si JY, et al. The safety and immunological effects of rAd5-EBV-LMP2 vaccine in nasopharyngeal carcinoma patients: a phase I clinical trial and two-year follow-up. Chem Pharm Bull (2016) 64(8):1118–23. doi:10.1248/cpb.c16-00114
58. Taylor GS, Jia H, Harrington K, Lee LW, Turner J, Ladell K, et al. A recombinant modified vaccinia ankara vaccine encoding Epstein-Barr virus (EBV) target antigens: a phase I trial in UK patients with EBV-positive cancer. Clin Cancer Res (2014) 20(19):5009–22. doi:10.1158/1078-0432.CCR-14-1122-T
59. Stransky N, Egloff AM, Tward AD, Kostic AD, Cibulskis K, Sivachenko A, et al. The mutational landscape of head and neck squamous cell carcinoma. Science (2011) 333(6046):1157–60. doi:10.1126/science.1208130
60. Gildener-Leapman N, Ferris RL, Bauman JE. Promising systemic immunotherapies in head and neck squamous cell carcinoma. Oral Oncol (2013) 49(12):1089–96. doi:10.1016/j.oraloncology.2013.09.009
61. Carbone DP, Ciernik IF, Kelley MJ, Smith MC, Nadaf S, Kavanaugh D, et al. Immunization with mutant p53- and K-ras-derived peptides in cancer patients: immune response and clinical outcome. J Clin Oncol (2005) 23(22):5099–107. doi:10.1200/JCO.2005.03.158
62. Segal NH, Parsons DW, Peggs KS, Velcutlescu V, Kinzler KW, Vogelstein B, et al. Epitope landscape in breast and colorectal cancer. Cancer Res (2008) 68(3):889–92. doi:10.1158/0008-5472.CAN-07-3095
63. Zolkind P, Dunn GP, Lin TX, Griffith M, Griffith OL, Uppaluri R. Neoantigens in immunotherapy and personalized vaccines: implications for head and neck squamous cell carcinoma. Oral Oncol (2017) 71:169–76. doi:10.1016/j.oraloncology.2016.09.010
64. Overwijk WW, Wang E, Marincola FM, Rammensee HG, Restifo NP. Mining the mutanome: developing highly personalized Immunotherapies based on mutational analysis of tumors. J Immunother Cancer (2013) 1:11. doi:10.1186/2051-1426-1-11
65. Sahin U, Derhovanessian E, Miller M, Kloke BP, Simon P, Lower M, et al. Personalized RNA mutanome vaccines mobilize poly-specific therapeutic immunity against cancer. Nature (2017) 547(7662):222–6. doi:10.1038/nature23003
66. Ott PA, Hu Z, Keskin DB, Shukla SA, Sun J, Bozym DJ, et al. An immunogenic personal neoantigen vaccine for patients with melanoma. Nature (2017) 547(7662):217–21. doi:10.1038/nature22991
67. McCann KJ, Mander A, Cazaly A, Chudley L, Stasakova J, Thirdborough S, et al. Targeting carcinoembryonic antigen with DNA vaccination: on-target adverse events link with immunologic and clinical outcomes. Clin Cancer Res (2016) 22(19):4827–36. doi:10.1158/1078-0432.CCR-15-2507
68. Quoix E, Lena H, Losonczy G, Forget F, Chouaid C, Papai Z, et al. TG4010 immunotherapy and first-line chemotherapy for advanced non-small-cell lung cancer (TIME): results from the phase 2b part of a randomised, double-blind, placebo-controlled, phase 2b/3 trial. Lancet Oncol (2016) 17(2):212–23. doi:10.1016/S1470-2045(15)00483-0
69. Schuler PJ, Harasymczuk M, Visus C, Deleo A, Trivedi S, Lei Y, et al. Phase I dendritic cell p53 peptide vaccine for head and neck cancer. Clin Cancer Res (2014) 20(9):2433–44. doi:10.1158/1078-0432.CCR-13-2617
70. Miyazaki A, Kobayashi J, Torigoe T, Hirohashi Y, Yamamoto T, Yamaguchi A, et al. Phase I clinical trial of survivin-derived peptide vaccine therapy for patients with advanced or recurrent oral cancer. Cancer Sci (2011) 102(2):324–9. doi:10.1111/j.1349-7006.2010.01789.x
71. Gotter J, Brors B, Hergenhahn M, Kyewski B. Medullary epithelial cells of the human thymus express a highly diverse selection of tissue-specific genes colocalized in chromosomal clusters. J Exp Med (2004) 199(2):155–66. doi:10.1084/jem.20031677
72. Janitz M, Fiszer D, Michalczak-Janitz K, Lukaszyk A, Fernandez N, Skorupski W, et al. Analysis of mRNA for class I HLA on human gametogenic cells. Mol Reprod Dev (1994) 38(2):231–7. doi:10.1002/mrd.1080380215
74. Andersen RS, Thrue CA, Junker N, Lyngaa R, Donia M, Ellebaek E, et al. Dissection of T-cell antigen specificity in human melanoma. Cancer Res (2012) 72(7):1642–50. doi:10.1158/0008-5472.CAN-11-2614
75. Tsuji T, Altorki NK, Ritter G, Old LJ, Gnjatic S. Characterization of preexisting MAGE-A3-specific CD4(+) T cells in cancer patients and healthy individuals and their activation by protein vaccination. J Immunol (2009) 183(7):4800–8. doi:10.4049/jimmunol.0900903
76. Valmori D, Scheibenbogen C, Dutoit V, Nagorsen D, Asemissen AM, Rubio-Godoy V, et al. Circulating tumor-reactive CD8(+) T cells in melanoma patients contain a CD45RA(+)CCR7(-) effector subset exerting ex vivo tumor-specific cytolytic activity. Cancer Res (2002) 62(6):1743–50.
77. Kienstra MA, Neel HB, Strome SE, Roche P. Identification of NY-ESO-1, MAGE-1, and MAGE-3 in head and neck squamous cell carcinoma. Head Neck (2003) 25(6):457–63. doi:10.1002/hed.10223
78. Szczepanski MJ, DeLeo AB, Luczak M, Molinska-Glura M, Misiak J, Szarzynska B, et al. PRAME expression in head and neck cancer correlates with markers of poor prognosis and might help in selecting candidates for retinoid chemoprevention in pre-malignant lesions. Oral Oncol (2013) 49(2):144–51. doi:10.1016/j.oraloncology.2012.08.005
79. Tureci O, Chen YT, Sahin U, Gure AO, Zwick C, Villena C, et al. Expression of SSX genes in human tumors. Int J Cancer (1998) 77(1):19–23. doi:10.1002/(SICI)1097-0215(19980703)77:1<19::AID-IJC4>3.0.CO;2-2
80. Zamuner FT, Karia BTR, de Oliveira CZ, dos Santos CR, Carvalho AL, Vettore AL. A comprehensive expression analysis of cancer testis antigens in head and neck squamous cell carcinoma revels MAGEA3/6 as a marker for recurrence. Mol Cancer Ther (2015) 14(3):828–34. doi:10.1158/1535-7163.MCT-14-0796
81. Voskens CJ, Sewell D, Hertzano R, DeSanto J, Rollins S, Lee M, et al. inducTION of mage-A3 and HPV-16 immunity by Trojan vaccines in patients with head and neck carcinoma. Head Neck (2012) 34(12):1734–46. doi:10.1002/hed.22004
82. Hirayama M, Nishimura Y. The present status and future prospects of peptide-based cancer vaccines. Int Immunol (2016) 28(7):319–28. doi:10.1093/intimm/dxw027
83. Yamabuki T, Daigo Y, Kato T, Hayama S, Tsunoda T, Miyamoto M, et al. Genome-wide gene expression profile analysis of esophageal squamous cell carcinomas. Int J Oncol (2006) 28(6):1375–84. doi:10.3892/ijo.28.6.1375
84. Suda T, Tsunoda T, Daigo Y, Nakamura Y, Tahara H. Identification of human leukocyte antigen-A24-restricted epitope peptides derived from gene products upregulated in lung and esophageal cancers as novel targets for immunotherapy. Cancer Sci (2007) 98(11):1803–8. doi:10.1111/j.1349-7006.2007.00603.x
85. Kono K, Iinuma H, Akutsu Y, Tanaka H, Hayashi N, Uchikado Y, et al. Multicenter, phase II clinical trial of cancer vaccination for advanced esophageal cancer with three peptides derived from novel cancer-testis antigens. J Transl Med (2012) 10:141. doi:10.1186/1479-5876-10-141
86. Kono K, Mizukami Y, Daigo Y, Takano A, Masuda K, Yoshida K, et al. Vaccination with multiple peptides derived from novel cancer-testis antigens can induce specific T-cell responses and clinical responses in advanced esophageal cancer. Cancer Sci (2009) 100(8):1502–9. doi:10.1111/j.1349-7006.2009.01200.x
87. Yoshitake Y, Fukuma D, Yuno A, Hirayama M, Nakayama H, Tanaka T, et al. Phase II clinical trial of multiple peptide vaccination for advanced head and neck cancer patients revealed induction of immune responses and improved OS. Clin Cancer Res (2015) 21(2):312–21. doi:10.1158/1078-0432.CCR-14-0202
88. Janssen EM, Lemmens EE, Wolfe T, Christen U, von Herrath MG, Schoenberger SP. CD4+ T cells are required for secondary expansion and memory in CD8+ T lymphocytes. Nature (2003) 421(6925):852–6. doi:10.1038/nature01441
89. Schoenberger SP, Toes RE, van der Voort EI, Offringa R, Melief CJ. T-cell help for cytotoxic T lymphocytes is mediated by CD40-CD40L interactions. Nature (1998) 393(6684):480–3. doi:10.1038/31002
90. Pasqual G, Chudnovskiy A, Tas JMJ, Agudelo M, Schweitzer LD, Cui A, et al. Monitoring T cell-dendritic cell interactions in vivo by intercellular enzymatic labelling. Nature (2018) 553(7689):496–500. doi:10.1038/nature25442
91. Alexander J, Sidney J, Southwood S, Ruppert J, Oseroff C, Maewal A, et al. Development of high potency universal DR-restricted helper epitopes by modification of high affinity DR-blocking peptides. Immunity (1994) 1(9):751–61. doi:10.1016/S1074-7613(94)80017-0
92. King CA, Spellerberg MB, Zhu D, Rice J, Sahota SS, Thompsett AR, et al. DNA vaccines with single-chain Fv fused to fragment C of tetanus toxin induce protective immunity against lymphoma and myeloma. Nat Med (1998) 4(11):1281–6. doi:10.1038/3266
93. Savelyeva N, Munday R, Spellerberg MB, Lomonossoff GP, Stevenson FK. Plant viral genes in DNA idiotypic vaccines activate linked CD4+ T-cell mediated immunity against B-cell malignancies. Nat Biotechnol (2001) 19(8):760–4. doi:10.1038/90816
94. Hung CF, Monie A, Alvarez RD, Wu TC. DNA vaccines for cervical cancer: from bench to bedside. Exp Mol Med (2007) 39(6):679–89. doi:10.1038/emm.2007.74
95. Stevenson FK, Ottensmeier CH, Johnson P, Zhu D, Buchan SL, McCann KJ, et al. DNA vaccines to attack cancer. Proc Natl Acad Sci U S A (2004) 101(Suppl 2):14646–52. doi:10.1073/pnas.0404896101
96. Rice J, Ottensmeier CH, Stevenson FK. DNA vaccines: precision tools for activating effective immunity against cancer. Nat Rev Cancer (2008) 8(2):108–20. doi:10.1038/nrc2326
97. Melief CJ, van Hall T, Arens R, Ossendorp F, van der Burg SH. Therapeutic cancer vaccines. J Clin Invest (2015) 125(9):3401–12. doi:10.1172/JCI80009
98. Inderberg-Suso EM, Trachsel S, Lislerud K, Rasmussen AM, Gaudernack G. Widespread CD4+ T-cell reactivity to novel hTERT epitopes following vaccination of cancer patients with a single hTERT peptide GV1001. Onco-immunology (2012) 1(5):670–86. doi:10.4161/onci.20426
99. Choi IK, Wang Z, Ke Q, Hong M, Qian Y, Zhao X, et al. Signaling by the Epstein-Barr virus LMP1 protein induces potent cytotoxic CD4(+) and CD8(+) T cell responses. Proc Natl Acad Sci U S A (2018) 115(4):E686–95. doi:10.1073/pnas.1713607115
100. Haabeth OA, Tveita AA, Fauskanger M, Schjesvold F, Lorvik KB, Hofgaard PO, et al. How do CD4(+) T cells detect and eliminate tumor cells that either lack or express MHC class II molecules? Front Immunol (2014) 5:174. doi:10.3389/fimmu.2014.00174
101. Kreiter S, Vormehr M, van de Roemer N, Diken M, Lower M, Diekmann J, et al. Mutant MHC class II epitopes drive therapeutic immune responses to cancer. Nature (2015) 520(7549):692–6. doi:10.1038/nature14426
102. Krieg AM. CpG motifs in bacterial DNA and their immune effects. Annu Rev Immunol (2002) 20:709–60. doi:10.1146/annurev.immunol.20.100301.064842
103. Dempsey A, Bowie AG. Innate immune recognition of DNA: a recent history. Virology (2015) 479-480:146–52. doi:10.1016/j.virol.2015.03.013
104. Low L, Mander A, McCann K, Dearnaley D, Tjelle T, Mathiesen I, et al. DNA vaccination with electroporation induces increased antibody responses in patients with prostate cancer. Hum Gene Ther (2009) 20(11):1269–78. doi:10.1089/hum.2009.067
105. Kraynyak KA, Bodles-Brakhop A, Bagarazzi M. Tapping the potential of DNA delivery with electroporation for cancer immunotherapy. Curr Top Microbiol Immunol (2017) 405:55–78. doi:10.1007/82_2015_431
106. Chudley L, McCann K, Mander A, Tjelle T, Campos-Perez J, Godeseth R, et al. DNA fusion-gene vaccination in patients with prostate cancer induces high-frequency CD8(+) T-cell responses and increases PSA doubling time. Cancer Immunol Immunother (2012) 61(11):2161–70. doi:10.1007/s00262-012-1270-0
107. Meleshko AN, Petrovskaya NA, Savelyeva N, Vashkevich KP, Doronina SN, Sachivko NV. Phase I clinical trial of idiotypic DNA vaccine administered as a complex with polyethylenimine to patients with B-cell lymphoma. Hum Vaccin Immunother (2017) 13(6):1–6. doi:10.1080/21645515.2017.1285477
108. Bolhassani A, Safaiyan S, Rafati S. Improvement of different vaccine delivery systems for cancer therapy. Mol Cancer (2011) 10:3. doi:10.1186/1476-4598-10-3
109. Boursnell ME, Rutherford E, Hickling JK, Rollinson EA, Munro AJ, Rolley N, et al. Construction and characterisation of a recombinant vaccinia virus expressing human papillomavirus proteins for immunotherapy of cervical cancer. Vaccine (1996) 14(16):1485–94. doi:10.1016/S0264-410X(96)00117-X
110. Pim D, Storey A, Thomas M, Massimi P, Banks L. Mutational analysis of HPV-18 E6 identifies domains required for p53 degradation in vitro, abolition of p53 transactivation in vivo and immortalisation of primary BMK cells. Oncogene (1994) 9(7):1869–76.
111. Aggarwal C, Cohen R, Morrow MP, Bauml J, Weinstein G, Boyer J, et al. Immunotherapy with VGX-3100 (HPV16 and HPV18 plasmids) + INO-9012 (DNA encoding IL-12) in human papillomavirus (HPV) associated head and neck squamous cell carcinoma (HNSCCa): interim safety and immunogenicity results. J Immunother Cancer (2015) 3(Suppl 2):426. doi:10.1186/2051-1426-3-S2-P426
112. Cheng WF, Hung CF, Chai CY, Hsu KF, He L, Ling M, et al. Tumor-specific immunity and antiangiogenesis generated by a DNA vaccine encoding calreticulin linked to a tumor antigen. J Clin Invest (2001) 108(5):669–78. doi:10.1172/JCI200112346
113. Best SR, Peng S, Juang CM, Hung CF, Hannaman D, Saunders JR, et al. Administration of HPV DNA vaccine via electroporation elicits the strongest CD8+ T cell immune responses compared to intramuscular injection and intradermal gene gun delivery. Vaccine (2009) 27(40):5450–9. doi:10.1016/j.vaccine.2009.07.005
114. Alvarez RD, Huh WK, Bae S, Lamb LS Jr, Conner MG, Boyer J, et al. A pilot study of pNGVL4a-CRT/E7(detox) for the treatment of patients with HPV16+ cervical intraepithelial neoplasia 2/3 (CIN2/3). Gynecol Oncol (2016) 140(2):245–52. doi:10.1016/j.ygyno.2015.11.026
115. Allen A, Wang C, Caproni LJ, Sugiyarto G, Harden E, Douglas LR, et al. Linear doggybone DNA vaccine induces similar immunological responses to conventional plasmid DNA independently of immune recognition by TLR9 in a pre-clinical model. Cancer Immunol Immunother (2018) 67(4):627–38. doi:10.1007/s00262-017-2111-y
116. Grunwitz C, Kranz LM. mRNA cancer vaccines-messages that prevail. Curr Top Microbiol Immunol (2017) 405:145–64. doi:10.1007/82_2017_509
117. Kranz LM, Diken M, Haas H, Kreiter S, Loquai C, Reuter KC, et al. Systemic RNA delivery to dendritic cells exploits antiviral defence for cancer immunotherapy. Nature (2016) 534(7607):396–401. doi:10.1038/nature18300
118. van Poelgeest MI, Welters MJ, van Esch EM, Stynenbosch LF, Kerpershoek G, van Persijn van Meerten EL, et al. HPV16 synthetic long peptide (HPV16-SLP) vaccination therapy of patients with advanced or recurrent HPV16-induced gynecological carcinoma, a phase II trial. J Transl Med (2013) 11:88. doi:10.1186/1479-5876-11-88
119. Welters MJ, van der Sluis TC, van Meir H, Loof NM, van Ham VJ, van Duikeren S, et al. Vaccination during myeloid cell depletion by cancer chemotherapy fosters robust T cell responses. Sci Transl Med (2016) 8(334):334ra52. doi:10.1126/scitranslmed.aad8307
120. Reuschenbach M, Rafiyan M, Pauligk C, Karbach J, Kloor M, Prigge ES, et al. Phase I/IIa trial targeting p16(INK4a) by peptide vaccination in patients with human papillomavirus-associated cancer. J Clin Oncol (2015) 33(15):e14030. doi:10.1200/jco.2015.33.15_suppl.e14030
121. Slingerland M, Speetjens F, Welters M, Gelderblom H, Roozen I, van der Velden LA, et al. A phase I study in patients with a human papillomavirus type 16 positive oropharyngeal tumor treated with second generation synthetic long peptide vaccine conjugated to a defined adjuvant. J Clin Oncol (2016) 34(15_suppl):TPS3113. doi:10.1200/JCO.2016.34.15_suppl.TPS3113
123. Ryman KD, Klimstra WB. Host responses to alphavirus infection. Immunol Rev (2008) 225:27–45. doi:10.1111/j.1600-065X.2008.00670.x
124. Daemen T, Riezebos-Brilman A, Regts J, Dontje B, van der Zee A, Wilschut J. Superior therapeutic efficacy of alphavirus-mediated immunization against human papilloma virus type 16 antigens in a murine tumour model: effects of the route of immunization. Antivir Ther (2004) 9(5):733–42.
125. Lin CT, Hung CF, Juang J, He L, Lin KY, Kim TW, et al. Boosting with recombinant vaccinia increases HPV-16 E7-specific T cell precursor frequencies and antitumor effects of HPV-16 E7-expressing Sindbis virus replicon particles. Mol Ther (2003) 8(4):559–66. doi:10.1016/S1525-0016(03)00238-7
126. Riezebos-Brilman A, Regts J, Freyschmidt EJ, Dontje B, Wilschut J, Daemen T. Induction of human papilloma virus E6/E7-specific cytotoxic T-lymphocyte activity in immune-tolerant, E6/E7-transgenic mice. Gene Ther (2005) 12(18):1410–4. doi:10.1038/sj.gt.3302536
127. Cassetti MC, McElhiney SP, Shahabi V, Pullen JK, Le Poole IC, Eiben GL, et al. Antitumor efficacy of Venezuelan equine encephalitis virus replicon particles encoding mutated HPV16 E6 and E7 genes. Vaccine (2004) 22(3–4):520–7. doi:10.1016/j.vaccine.2003.07.003
128. Eiben GL, Velders MP, Schreiber H, Cassetti MC, Pullen JK, Smith LR, et al. Establishment of an HLA-A*0201 human papillomavirus type 16 tumor model to determine the efficacy of vaccination strategies in HLA-A*0201 transgenic mice. Cancer Res (2002) 62(20):5792–9.
129. Borysiewicz LK, Fiander A, Nimako M, Man S, Wilkinson GW, Westmoreland D, et al. A recombinant vaccinia virus encoding human papillomavirus types 16 and 18, E6 and E7 proteins as immunotherapy for cervical cancer. Lancet (1996) 347(9014):1523–7. doi:10.1016/S0140-6736(96)90674-1
130. Baldwin PJ, van der Burg SH, Boswell CM, Offringa R, Hickling JK, Dobson J, et al. Vaccinia-expressed human papillomavirus 16 and 18 e6 and e7 as a therapeutic vaccination for vulval and vaginal intraepithelial neoplasia. Clin Cancer Res (2003) 9(14):5205–13.
131. Kaufmann AM, Stern PL, Rankin EM, Sommer H, Nuessler V, Schneider A, et al. Safety and immunogenicity of TA-HPV, a recombinant vaccinia virus expressing modified human papillomavirus (HPV)-16 and HPV-18 E6 and E7 genes, in women with progressive cervical cancer. Clin Cancer Res (2002) 8(12):3676–85.
132. Sutter G, Moss B. Nonreplicating vaccinia vector efficiently expresses recombinant genes. Proc Natl Acad Sci U S A (1992) 89(22):10847–51. doi:10.1073/pnas.89.22.10847
133. Sutter G, Staib C. Vaccinia vectors as candidate vaccines: the development of modified vaccinia virus Ankara for antigen delivery. Curr Drug Targets Infect Disord (2003) 3(3):263–71. doi:10.2174/1568005033481123
134. Chahroudi A, Chavan R, Kozyr N, Waller EK, Silvestri G, Feinberg MB. Vaccinia virus tropism for primary hematolymphoid cells is determined by restricted expression of a unique virus receptor. J Virol (2005) 79(16):10397–407. doi:10.1128/JVI.79.16.10397-10407.2005
135. Brun JL, Dalstein V, Leveque J, Mathevet P, Raulic P, Baldauf JJ, et al. Regression of high-grade cervical intraepithelial neoplasia with TG4001 targeted immunotherapy. Am J Obstet Gynecol (2011) 204(2):e1–8. doi:10.1016/j.ajog.2010.09.020
136. Miles B, Safran HP, Monk BJ. Therapeutic options for treatment of human papillomavirus-associated cancers – novel immunologic vaccines: ADXS11-001. Gynecol Oncol Res Pract (2017) 4:10. doi:10.1186/s40661-017-0047-8
137. Miles BA, Monk BJ, Safran HP. Mechanistic insights into ADXS11-001 human papillomavirus-associated cancer immunotherapy. Gynecol Oncol Res Pract (2017) 4:9. doi:10.1186/s40661-017-0046-9
138. Krupar R, Imai N, Miles B, Genden E, Misiukiewicz K, Saenger Y, et al. Abstract LB-095: HPV E7 antigen-expressing Listeria-based immunotherapy (ADXS11-001) prior to robotic surgery for HPV-positive oropharyngeal cancer enhances HPV-specific T cell immunity. Cancer Res (2016) 76(Suppl 14):am2016-lb-095. doi:10.1158/1538-7445.AM2016-LB-095
139. Keenan B, Jaffee EM. Whole cell vaccines—past progress and future strategies. Semin Oncol (2012) Section II:276–86. doi:10.1053/j.seminoncol.2012.02.007
140. Lathuiliere A, Mach N, Schneider BL. Encapsulated cellular implants for recombinant protein delivery and therapeutic modulation of the immune system. Int J Mol Sci (2015) 16(5):10578–600. doi:10.3390/ijms160510578
141. Mach N, Vernet R, Belkouch MC, Luy P, Ancrenaz V, Teta P, et al. MVX-ONCO-1 phase 1 final results of the first personalized cell-based immunotherapy using cell encapsulation technology. Ann Oncol (2016) 27(Suppl 6):1058P. doi:10.1093/annonc/mdw378.12
142. Allostim. Immunovative Therapies Ltd. (2015). Available from: http://www.immunovative.com/products/allostim
143. Duray A, Demoulin S, Hubert P, Delvenne P, Saussez S. Immune suppression in head and neck cancers: a review. Clin Dev Immunol (2010) 2010:701657. doi:10.1155/2010/701657
144. Gajewski TF, Schreiber H, Fu YX. Innate and adaptive immune cells in the tumor microenvironment. Nat Immunol (2013) 14(10):1014–22. doi:10.1038/ni.2703
145. Ottensmeier CH, Perry KL, Harden EL, Stasakova J, Jenei V, Fleming J, et al. Upregulated glucose metabolism correlates inversely with CD8+ T-cell infiltration and survival in squamous cell carcinoma. Cancer Res (2016) 76(14):4136–48. doi:10.1158/0008-5472.CAN-15-3121
Keywords: head and neck cancer, human papillomavirus, human papillomavirus independent, cancer antigens, cancer vaccines
Citation: Wang C, Dickie J, Sutavani RV, Pointer C, Thomas GJ and Savelyeva N (2018) Targeting Head and Neck Cancer by Vaccination. Front. Immunol. 9:830. doi: 10.3389/fimmu.2018.00830
Received: 01 February 2018; Accepted: 05 April 2018;
Published: 23 April 2018
Edited by:
Rasha Abu Eid, University of Aberdeen, United KingdomReviewed by:
Graham Robert Leggatt, The University of Queensland, AustraliaAngelika B. Riemer, Deutsches Krebsforschungszentrum (DKFZ), Germany
Copyright: © 2018 Wang, Dickie, Sutavani, Pointer, Thomas and Savelyeva. This is an open-access article distributed under the terms of the Creative Commons Attribution License (CC BY). The use, distribution or reproduction in other forums is permitted, provided the original author(s) and the copyright owner are credited and that the original publication in this journal is cited, in accordance with accepted academic practice. No use, distribution or reproduction is permitted which does not comply with these terms.
*Correspondence: Natalia Savelyeva, bnMxJiN4MDAwNDA7c290b24uYWMudWs=