- 1Faculdade de Medicina de Petrópolis (FMP-FASE), Petrópolis, Brazil
- 2Instituto de Biofísica Carlos Chagas Filho, Universidade Federal do Rio de Janeiro, Rio de Janeiro, Brazil
- 3Laboratório de Imunoparasitologia, Instituto Oswaldo Cruz, Fundação Oswaldo Cruz, Rio de Janeiro, Brazil
- 4Faculdade de Medicina, Universidade Federal do Rio de Janeiro, Rio de Janeiro, Brazil
- 5Laboratório de Microbiologia Celular, Instituto Oswaldo Cruz, Fundação Oswaldo Cruz, Rio de Janeiro, Brazil
- 6Departamento de Bioquímica e Imunologia, Universidade Federal de Minas Gerais, Belo Horizonte, Brazil
- 7Instituto de Biologia, Universidade Federal Fluminense, Niterói, Brazil
Few studies investigate the major protein antigens targeted by the antibody diversity of infected mice with Trypanosoma cruzi. To detect global IgG antibody specificities, sera from infected mice were immunoblotted against whole T. cruzi extracts. By proteomic analysis, we were able to identify the most immunogenic T. cruzi proteins. We identified three major antigens as pyruvate phosphate dikinase, Hsp-85, and β-tubulin. The major protein band recognized by host IgG was T. cruzi β-tubulin. The T. cruzi β-tubulin gene was cloned, expressed in E. coli, and recombinant T. cruzi β-tubulin was obtained. Infection increased IgG reactivity against recombinant T. cruzi β-tubulin. A single immunization of mice with recombinant T. cruzi β-tubulin increased specific IgG reactivity and induced protection against T. cruzi infection. These results indicate that repertoire analysis is a valid approach to identify antigens for vaccines against Chagas disease.
Introduction
Chagas disease is caused by the protozoan parasite Trypanosoma cruzi and imposes a heavy burden on human health in Latin America. Infection with T. cruzi leads to parasitemia and spread of the parasite to host tissues. Control of T. cruzi infection depends on cells of innate and acquired immunity, which produce cytokines, inflammatory mediators, and antibodies (1, 2). However, tissue infection persists indefinitely at low levels (1, 2). Several studies indicate the importance of antibodies in protection against T. cruzi infection (3, 4, 5, 6), but the precise role of humoral immunity in host defense remains incompletely understood.
Purified T. cruzi proteins induce protection in mice challenged with live parasites. These antigens include cruzipain (7), trans-sialidase (8), members of mucin-associated surface protein family (9), and excretory-secretory antigens (10). However, most studies amplify detection by using recombinant antigens, or probing with antibodies from immunized animals. New studies have characterized novel parasites antigens that could be targeted in vaccine studies (11, 12). An alternative approach to the testing of randomly purified molecules is the identification of T. cruzi antigens which are targeted by the host antibody diversity in the course of infection. Antibody diversity can be analyzed by an immunoblot technique which detects global antibody reactivity against whole protein extracts (13). This method detects autoantibodies produced in autoimmune diseases (14, 15) and identifies repertoire changes linked to resistance against T. cruzi infection (16).
Microtubules are cytoskeletal structures composed of α/β tubulin heterodimers that are found in eukaryotic cells and are abundant in trypanosomatid parasites from the order Kinetoplastide (17). These structures have important functions in cell division, maintenance of cellular morphology, motility, intracellular transport, and signal transduction (18). In trypanosomes microtubules have two α-tubulin isoforms and one β-tubulin isoform (19) and are found underneath the plasma membrane (the subpellicular microtubules), in the flagellum, and as a component of mitotic spindle apparatus (20). The microtubules function as a perfect target for many compounds with trypanocidal activity, blocking tubulin activity (21). Therefore, a microtubule component is a suitable target to be considered as an effective vaccine candidate to protect from trypanosome infections (22, 23). It was previously shown that mice vaccinated with native tubulin purified from Trypanosoma brucei were protected against T. brucei, Trypanosoma congolense, and Trypanosoma rhodesiense infection (22). Previous report showed that mice vaccinated with the microtubule-associated protein (MAP) p52 of T. brucei, together with aldolase, GAPDH, and MAP p15, were protected after challenge with a homologous infection (24). Furthermore, rabbit antibodies to tubulin-rich fractions from T. brucei inhibit the growth of trypanosomes in culture (25).
Given the paucity of information regarding the subject, this work addresses the identification of prominent T. cruzi antigens targeted by IgG antibodies during infection. Here, we describe that acute and chronic infection of BALB/c mice induced limited changes in the antibody diversity. Using a proteomic approach, we identified T. cruzi β-tubulin (TcβTUB) as one of the major antigens targeted by antibodies. The T. cruzi β-tubulin gene was isolated, cloned, and expressed. Recombinant TcβTUB was recognized by sera from infected mice, and immunization of naïve mice with a single dose of recombinant TcβTUB induced protection against infection. These results indicate the importance of selecting candidate vaccines antigens from analysis of unbiased Ab reactivities from infected mice.
Materials and Methods
Mice, Parasite, and Infection
Male wild-type (WT) BALB/c and Fas-L mutant BALB/c. gld (gld) mice aging 6–8 weeks, weighing 25–30 g were from the Oswaldo Cruz Institute Animal Care facility, Rio de Janeiro. BALB. gld mice (26) were produced at the National Institutes of Health, Bethesda, MD, USA by serially backcrossing the gld gene onto a BALB/c background for 15 generations.
All mouse studies followed the guidelines set by the National Institutes of Health, United States. The study was approved by the Research Ethics Committee of Federal University of Rio de Janeiro (protocol 062/14). Protocols for animal were approved by the Institutional Ethical Committees in accordance with international guidelines. All animal experimentation was performed in accordance with the terms of the Brazilian guidelines for the animal welfare regulations.
Mice were infected with intraperitoneal injection (i.p.) with 105 chemically induced metacyclic forms of T. cruzi clone Dm28c (17, 18). Chemically induced and insect-derived metacyclic forms induce a similar infection as demonstrated previously (18). Acute infection was evaluated after 23–33 days of infection, while chronic infection was evaluated after 150 days.
Recombinant T. cruzi β-Tubulin
Genomic DNA was extracted from T. cruzi Dm28c epimastigotes (1 × 108; Rapidprep isolation kit, Pharmacia) and used as template for amplification of T. cruzi β-tubulin gene (19) by touchdown PCR (27). Touchdown PCR was carried out in 50 µL of 20 mM Tris–HCl (pH 8.8), 2 mM MgSO4, 10 mM KCl, 10 mM (NH4)2SO4, 0.1% (v/v) Triton X-100, 0.1 mg/mL nuclease-free BSA, 100 ng DNA, 0.5 mM dNTPs, 0.4 µM of primers TcβTubF (5′-ATCATATGCGTGAGATTGTGTGCG) and TcβTubR (5′-ATGAATTCTTAGTACTGCTCCTCCTC), and a mixture of 2.0 U Pfu (Fermentas) and 0.25 U Taq (Biotools) DNA polymerases. The resulting 1,342 bp fragment was cloned into pTZ57R/T (Fermentas), sequenced and sub-cloned, yielding pET-28a-TcβTUB expression plasmid. pET-28a-TcβTUB was introduced into BL21 (DE3) E. coli strain, and transfectants were induced with 0.5 mM isopropyl 1-thio-β-d-galactopyranoside (Invitrogen, USA) for 18 h at 20°C. Cells were suspended in 300 µg/mL lysozyme (Sigma) in 50 mM Tris–HCl (pH 7.5), 200 mM NaCl, 5% (w/v) glycerol, 1 mM DTT, and protease inhibitor mix (Sigma), and left 30 min on ice. After addition of 10 U DNAse I (Fermentas) and 5 mM MgCl2, the suspension was incubated for 30 min on ice and lysed by sonication. The lysate was centrifuged at 30,900 g for 40 min at 4°C, and SDS-PAGE indicated the presence of recombinant T. cruzi β-tubulin (TcβTUB). The pellet was solubilized in 2% Triton X-100, 2 M Urea, 100 mM Tris–HCl (pH 7.5), 5 mM Na2EDTA, 5 mM DTT, 5 mM imidazole, and protease inhibitor mix. The supernatant containing TcβTUB was applied onto a His-Trap HP (GE Healthcare) column pre-equilibrated with 2 M Urea, 100 mM Tris–HCl (pH 7.5), 0.5 M NaCl containing 5 mM imidazole (wash buffer). Recombinant TcβTUB was eluted with wash buffer containing 0.25 M imidazole, analyzed for purity on SDS-PAGE, and quantified by the method of Bradford (28) using BSA as standard.
ELISA
For determination of serum IgG, microplates (Greiner BiOne) were coated with 2 µg/mL goat anti-mouse IgG (Southern Biotechnology) for 16 h at 4°C. Concentrations were calculated based on standard curves generated with purified mouse IgG (Southern). In addition, microplates were coated with dsDNA from calf thymus, histone from calf thymus, myosin from rabbit heart, KLH (5 µg/mL; all from Sigma), or T. cruzi antigen diluted at 1:1,000. T. cruzi antigen was obtained by lysing epimastigotes in buffer containing 200 mM Tris, 400 mM NaCl, 40 mM EDTA, 20 mM iodoacetamide, and 1 mM PMSF. ELISA tests for IgG against recombinant TcβTUB (0.5 µg/mL) were performed. Plates were blocked with PBS-1% gelatin (Vetec, Brazil) for 1 h and washed with PBS containing 0.05% Tween-20. Sera were kept at −20°C until use. Data show the results with 1:100 serum dilution. Binding was determined following incubation with alkaline phosphatase-conjugated goat anti-mouse IgG (Southern). Reaction was developed with PnPP substrate (Southern) diluted in Tris–MgCl2 buffer. Absorbance was read at 405 nm.
Immunoblots
For antibody repertoire analysis, mouse hearts were lysed in extraction buffer (2% SDS, 5% 2-mercaptoethanol, and 62.5 mM Tris, pH 6.8) on ice, without protease inhibitors, at a proportion of 1 g/10 mL buffer. T. cruzi epimastigotes (clone Dm28c) were lysed in 10 mL extraction buffer (5 × 106/mL). Extracts were sonicated for 10 min, boiled for 10 min, centrifuged at 1,000 g, and then at 10,000 g. Supernatants were stored at −20°C. IgG reactivities against heart and T. cruzi polypeptides were identified by a modified immunoblot technique (13). Briefly, extracts (600 µg/mL) were subjected to SDS-PAGE, and proteins were transferred to nitrocellulose. Membranes were blocked for 18 h with PBS-0.2% Tween-20 at RT and incubated for 4 h with sera adjusted for 100 µg/mL IgG concentration, using the Cassette Miniblot System (Immunetics, Cambridge, MA, USA). Alkaline phosphatase-conjugated secondary goat anti-mouse IgG antibody (Southern) was added for 90 min. After washing, immunoreactivities were revealed with nitroblue-tetrazolium/bromo-chloro-indolyl-phosphate (NBT/BCIP; Promega) and analyzed by densitometry. Blotted proteins were stained with colloidal gold (Bio-Rad) and subjected to a second densitometry. The immunoblot and protein scans were superimposed and rescaled to correct migration irregularities. Adjusted profiles were divided into sections representing an IgG reactivity. Section reactivities were quantified as the average optical density expressed as peak values and subjected to multiparametric statistical analysis (13). Data analysis was performed on an iMac computer using the Igor software (WaveMetrics, Lake Oswego, OR, USA) (13, 16). The statistical test employed comparison between curves, rather than individual bands. Densitometric profiles represent the mean of 5–8 individual sera. Immunoblots against recombinant TcβTUB were performed with either anti-mouse β-tubulin III monoclonal antibody 5G8 (Promega) or anti-β-tubulin rabbit monoclonal antibody 9F3 (Cell Signaling). Anti-α-tubulin monoclonal antibody B-5-1-2 (29) was used as control. Mouse brain extract (MBE) (25 µg protein/lane) was used as source of mouse tubulin (30). Blots were incubated with 1:10,000 Alexa-680-labeled anti-mouse IgG (Invitrogen) or IRDye-800-labeled anti-rabbit IgG (Li-Cor), diluted in TBS with 3% BSA for 1 h at RT. Fluorescence was imaged using an Odyssey® infrared scanner (LI-COR Inc.). Blots were scanned and processed using Adobe Photoshop CS and Image J 1.43m software.
Mass Spectrometry
Trypanosoma cruzi extracts were subjected to SDS-PAGE in parallel mirror lanes. One lane was blotted and reacted with serum to identify the major bands. Following precise location, the mirror lane was excised in the gel. Excision and processing of protein spots were performed as previously described (31). Digestions were done with sequencing grade porcine trypsin (Promega, Madison, WI, USA) at 37°C for 16 h. Tryptic peptides (0.5 mL) were mixed with a saturated solution of CHCA matrix in 50% ACN, 1% TFA (0.5 mL), spotted onto an MALDI sample plate, and allowed to crystallize at room temperature. MALDI/TOF-TOF peptide sequencing, operated in reflectron-delayed extraction mode with high resolution for the 800–4,000 Da range, was performed by precursor ion fragmentation, using N2 gas in the collision cell at 2.8 × 10−6 torr, in a 4700 Explorer Proteomics Analyzer (Applied Biosystems). Protein identification was carried out against the NCBI database using the MASCOT software (www.matrixscience.com) and the following parameters: cys-carbamidomethylation as fixed modification; methionine oxidation as variable modification; one missing trypsin cleavage, monoisotopic masses, peptide, and ion tolerances at 0.2 Da. Under these conditions, a probability based score 81 was considered significant (p < 0.05). The other criteria for identification were a minimum of 20% of protein coverage and four peptides with hits in the database (for PMF data) or two peptide sequence tags (for MS/MS).
Immunization Assays
BALB/c mice were injected with PBS, BSA, or recombinant TcβTUB (both 20 µg/animal) emulsified in Complete Freund Adjuvant (CFA, Thermo Scientific) in the hind footpads. After 14 days, mice were infected with 105 metacyclic trypomastigotes as above. Parasitemia was evaluated by tail vein puncture; viable parasites were counted in a Neubauer chamber. Mice were sacrificed at day 32 of infection, and individual spleens were weighted. Individual sera were collected and analyzed by western blotting for reactivity against T. cruzi and mouse tubulins.
Statistical Analysis
Data were analyzed by Student’s t-test for independent samples and ANOVA test, using SigmaPlot™ for Windows. Data from parasitemia were normalized by log transformation before statistical testing. Data from densitometric analysis were analyzed by Mann–Whitney test, using Graph Pad InStat 3.01 for Windows. Differences with a p value < 0.05 or lower were considered significant.
Results
Antibody Reactivities Against Exogenous and Autologous Antigens in T. cruzi Infection
We infected BALB/c mice with chemically induced metacyclic forms of T. cruzi clone Dm28c (17, 18). Chemically induced and insect-derived metacyclic forms induce a similar infection (18). Acute and chronic infection with T. cruzi increased the concentrations of serum IgG (data not shown). We measured IgG reactivities against a panel of exogenous and autologous antigens (T. cruzi, KLH, myosin, dsDNA, and histone) by ELISA in the sera of control and infected mice. Only IgG reactivities against T. cruzi antigens increased after acute (Figure 1A, left) or chronic infection (Figure 1B, left). As a positive control for autoreactivity, we used sera from lupus-prone gld mice for comparison (Figures 1A,B, right). Our results show that a highly focused Ab response against T. cruzi antigens, and not autoreactivity, is the final outcome following infection.
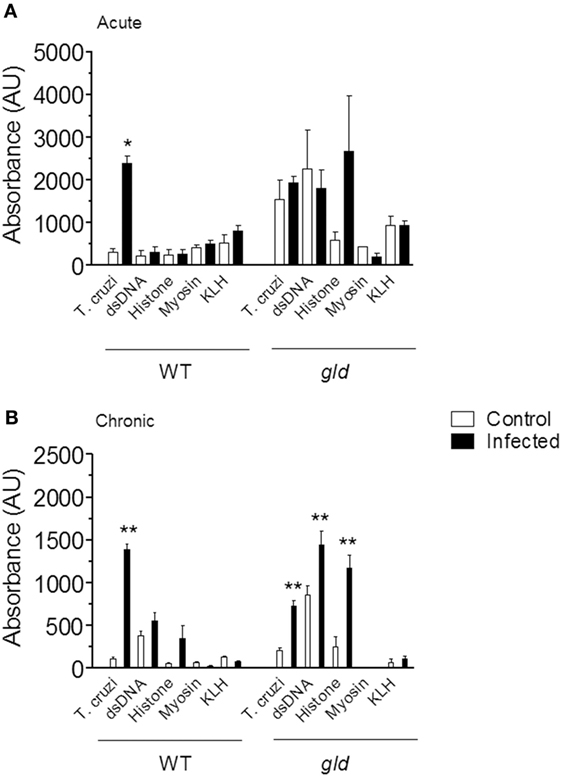
Figure 1. Analysis of serum IgG specificities in wild-type (WT) and gld BALB/c mice during acute (A) and chronic (B) infection with Trypanosoma cruzi. Serum IgG specificities from control (open bars) and infected mice (closed bars) were assayed by ELISA on plates coated with the indicated antigens. All sera were diluted 1:100. Data are mean and SEM of 3–12 mice per group. Statistical analysis was performed by t-test from representative results of three similar experiments (*p < 0.05; **p < 0.01).
Global Analysis of IgG Reactivities Against T. cruzi and Cardiac Polypeptides
We analyzed global IgG antibody reactivities with a semiquantitative immunoblot technique against T. cruzi epimastigote extracts (13–16). Western blots of individual sera from chronic infection showed that IgG reactivities were directed to a restricted set of antigens (Figure 2A, right). The strongest reactivity was directed to a 50–55 kDa band, which was detected with sera from all infected mice (Figure 2A). This reactivity colocalized with the most abundant band present on Coomassie blue-stained gels of T. cruzi extracts (Figure 2B, left). We quantified the immunoblots from all animals at both acute and chronic infection and showed the average optical density profiles of the IgG reactivities against T. cruzi proteins (Figures 3A,B, left). Global IgG reactivity against T. cruzi was focused on a few bands of the parasite extract (Figures 3A,B, left). The most prominent antibody reactivity against T. cruzi was represented by a band located at positions 600 and 800 in the blots of acute and chronic stages, respectively, depending on conditions of the electrophoresis assay (arrows on Figures 3A,B, left). Reacting T. cruzi extract with a mixture of acute and chronic sera on the same membrane yielded a single band, suggesting that it was the same reactivity (not shown). Acutely infected gld mice gave the same prominent IgG reactivity (Figure S1 in Supplementary Material). In addition, experiments with trypomastigote enriched extracts indicated the presence of an intense 50–55 kDa IgG reactivity (data not shown).
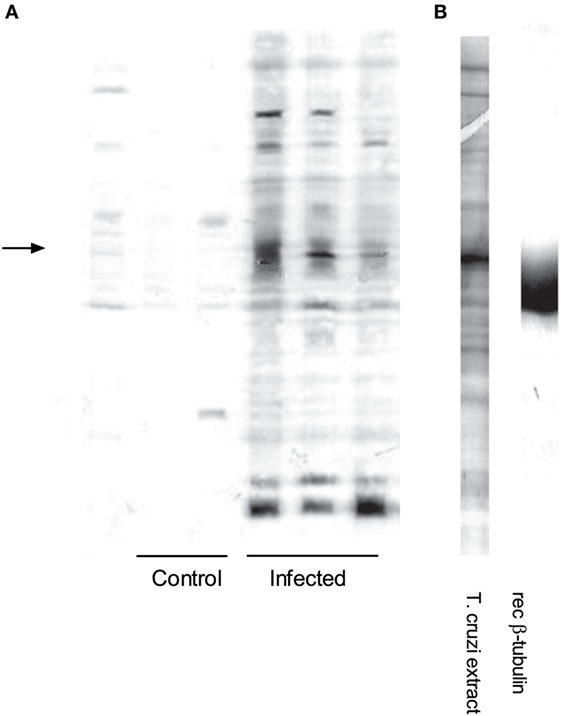
Figure 2. Infection induces a distinct antibody repertoire: a major reactivity elicited against Trypanosoma cruzi is directed to T. cruzi β-tubulin. (A) Immunoblot showing individual serum IgG reactivities from control (n = 3) and chronically infected BALB/c mice (n = 4) against T. cruzi extracts. The left arrow indicates the major 50–55 kDa protein band in the protein extract. IgG concentrations of all sera were adjusted to 100 µg/mL. (B) Left lane: Coomassie blue staining of SDS-PAGE showing the protein profile of T. cruzi extract. (B) Right lane: immunoblot of recombinant TcβTUB probed with anti-mouse β-tubulin III monoclonal antibody 5G8.
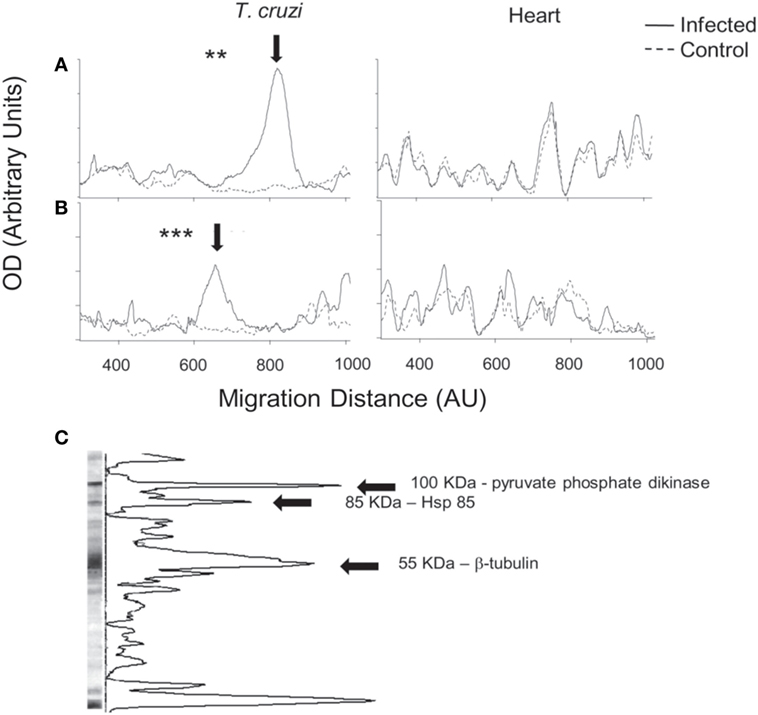
Figure 3. Global serum IgG reactivities against Trypanosoma cruzi and autologous heart polypeptides in infected BALB/c mice with T. cruzi. Mean densitometric profiles of IgG reactivities during acute (A) or chronic (B) infection on immunoblots against T. cruzi (left) and autologous heart polypeptides (right). Individual serum IgG concentrations were adjusted to 100 µg/mL. Arrow indicates the most prominent band of reactivity observed in the sera of acutely and chronically infected mice. Densitometric profiles represent the mean of 5–8 individual sera (see Materials and Methods) (**p < 0.01; ***p < 0.001). Immunoblots against heart polypeptides did not show significant differences. Data are representative of at least three independent experiments with five to eight mice per group. (C) A representative immunoblot of chronic serum against T. cruzi extract. Arrows indicate the three major T. cruzi protein bands, which were identified by mass spectrometry analysis as pyruvate phosphate dikinase (100 kDa), Hsp-85 (85 kDa), and β-tubulin (55 kDa). We could not identify the small-molecular weight band that also displayed a pronounced peak.
Infection with T. cruzi results in myocarditis (18). We therefore analyzed IgG antibody reactivities against autologous heart polypeptides (13–16). Global IgG reactivity against cardiac polypeptides was not significantly increased following acute or chronic infection with T. cruzi (Figures 3A,B, right).
Identification of T. cruzi β-Tubulin as a Major Antigenic Target for Antibodies in Infection
To identify the 50–55 kDa band reacting with sera from infection, the band was located in the gel with the help of the blot of a mirror lane, excised and subjected to mass spectrometry analysis. As shown in Table 1 and Figure 3C, the 50–55 kDa band was identified as T. cruzi β-tubulin (TcβTUB). We also identified two additional major T. cruzi antigens targeted by antibodies. The 100 kDa band was identified as pyruvate phosphate dikinase, and the 85 kDa band was identified as Hsp-85 (Figure 3C and data not shown).
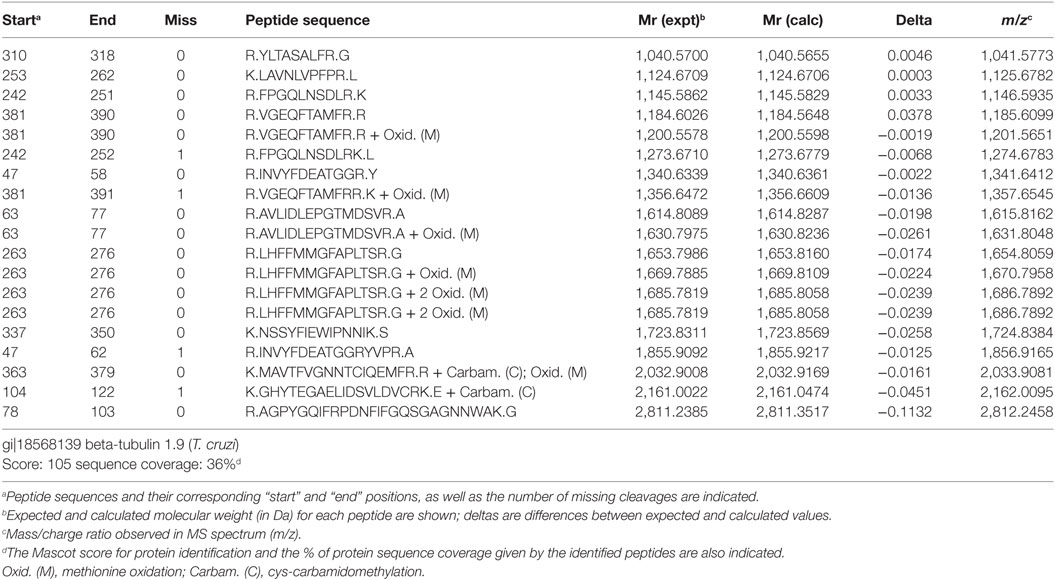
Table 1. Mass spectrometry analysis of peptide fragments of the major 50–55 kDa band of Trypanosoma cruzi extract corresponding to T. cruzi β-tubulin.
The β-tubulin coding region (19) was PCR amplified from T. cruzi Dm28c genomic DNA. The resulting fragment was cloned, sequenced and sub-cloned into pET-28a-TcβTUB expression plasmid. Recombinant TcβTUB was expressed and purified (Figure S2 in Supplementary Material). Following reaction with anti-β-tubulin monoclonal antibody, recombinant TcβTUB gave a single band compatible with the major band expressed in T. cruzi extracts (Figure 2B right). Analysis by ELISA confirmed that chronic infection with T. cruzi increased the production of IgG reactive with TcβTUB (Figure 4).
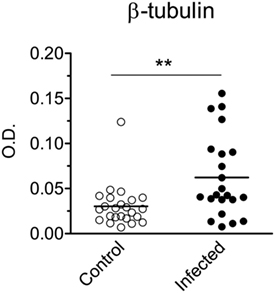
Figure 4. Infection with Trypanosoma cruzi increases IgG reactivity against T. cruzi β-tubulin. Serum IgG reactivities from control uninfected (open circles) or chronically infected (closed circles) BALB/c mice were assayed by ELISA on plates coated with recombinant TcβTUB. All IgG concentrations in sera were adjusted to 100 µg/mL. Each symbol corresponds to one individual mouse. Statistical analysis was performed by t-test from representative results of three similar experiments (**p < 0.01).
Immunization With T. cruzi β-Tubulin Induced Protection Against Infection
Naïve BALB/c mice were immunized with a single dose of recombinant TcβTUB in CFA and challenged with T. cruzi after 2 weeks. Control groups were immunized with PBS/CFA and BSA/CFA. All mice were sacrificed after 32 days of infection. Parasitemia was markedly reduced in mice immunized with TcβTUB/CFA, compared with control groups (Figure 5A). Spleen cellularity, which correlates with parasite burden in T. cruzi infection (32), was reduced in mice immunized with TcβTUB/CFA, compared with controls (Figure 5B). As expected, mice immunized with TcβTUB/CFA before infection produced higher amounts of anti-TcβTUB IgG, compared with controls (Figure 5C).
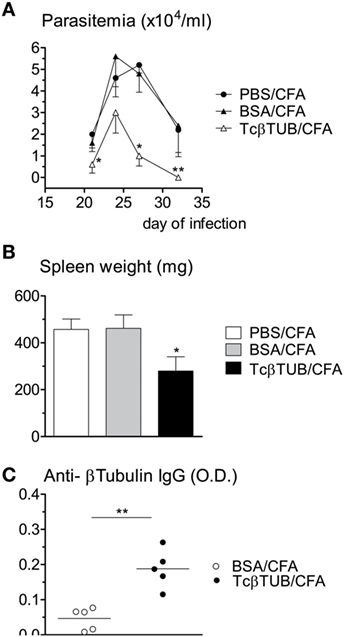
Figure 5. Immunization of naïve BALB/c mice with recombinant TcβTUB induces protection against Trypanosoma cruzi infection. (A) Kinetics of parasitemia. Groups of mice (n = 5 each) were immunized with PBS, BSA, or recombinant TcβTUB in CFA and were infected with T. cruzi Dm28c clone 14 days after immunization. Parasitemia was followed up to 32 days of infection. Data represent mean and SEM of groups. (B) Spleen weights of the same three groups after 32 days of infection. (C) After 32 days of infection, IgG levels against TcβTUB were determined for animals previously immunized with either BSA or TcβTUB in CFA. Statistical analysis was performed by two-way RM ANOVA test (A), one-way ANOVA test (B), and by t-test (C) from representative results of three similar experiments (*p < 0.05; **p < 0.01).
IgG reactivities of control and immunized mice were compared by western blotting against mouse tubulin (MBE), recombinant TcβTUB, and native T. cruzi tubulin (Figure 6). IgG from mice immunized with BSA/CFA before infection reacted with mouse tubulin, TcβTUB and reacted weakly with native T. cruzi tubulin (Figure 6A). IgG from mice immunized with TcβTUB/CFA before infection reacted to TcβTUB and to native T. cruzi tubulin (Figure 6B). In addition, IgG from TcβTUB/CFA group showed increased reactivity to mouse tubulin (Figure 6B). These differences were confirmed by densitometric analysis and are shown in Figure 6E. Blots with monoclonal antibodies indicated the contents of α- and β-tubulin in mouse brain and T. cruzi extracts, confirmed that the recombinant antigen was β-tubulin, and identified the expected 2 kDa molecular weight shift due to an additional histidine tail in recombinant TcβTUB (Figures 6C,D). Taken together, our data on repertoire analysis indicated that TcβTUB is an immunodominant antigen in T. cruzi infection and that previous immunization with TcβTUB elicited a protective host immune response against infection.
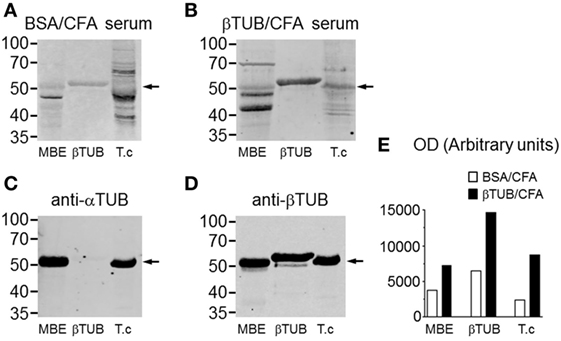
Figure 6. Increased IgG reactivity against β-tubulin in mice immunized with recombinant TcβTUB. (A) Immunoblots from mice immunized with BSA/CFA before infection and (B) mice immunized with TcβTUB/CFA before infection. Sera were blotted against mouse tubulin from mouse brain extract (MBE), recombinant TcβTUB and native tubulin from Trypanosoma cruzi extract. (C) Immunoblots of MBE, recombinant TcβTUB, and T. cruzi extract with monoclonal antibodies against α-tubulin; and (D) against β-tubulin. The shift in molecular weight of recombinant TcβTUB, compared with native mouse and T. cruzi β-tubulins, is due to an additional poly-histidine tail. (E) Densitometric profiles of the staining profiles presented in panels (A,B).
Discussion
Several proteins purified from T. cruzi induce protective immune responses. However, few studies identify the major protein antigens targeted by the antibody response of infected hosts. Here we investigated global changes in the antibody diversity of infected mice with T. cruzi. We employed a non-biased immunoblot technique against total parasite extracts. Under these selective conditions, the infection induced significant antibody reactivity against a range of T. cruzi antigens, which could be identified by proteomic analysis of the target proteins. We identified the major protein band recognized by IgG as TcβTUB. We cloned and expressed T. cruzi TcβTUB, and demonstrated that a single immunization with recombinant TcβTUB induced protection against T. cruzi infection. Therefore, our results indicated that repertoire analysis is a valid approach to identify new candidate antigens for a vaccine against Chagas disease.
Infection with T. cruzi can lead to polyclonal B lymphocyte activation, hypergammaglobulinemia, and production of autoantibodies (33, 34). We compared parasite-specific versus non-specific antibody reactivities elicited by infection. Since FasL-deficient mice undergo hypergammaglobulinemia, lymphoproliferation, and autoantibody production (35), we also compared antibody production by infected WT- and FasL-deficient gld mice. We used a limited set of exogenous and autologous antigens, and this approach did not reveal any evidence of autoreactivity or polyclonal lymphocyte activation. Our results might also reflect the use of Dm28c isolate, as parasite genetic diversity can influence the profile of immunoglobulins produced (36). On the other hand, age-matched FasL-deficient mice showed high levels of natural antibodies against T. cruzi and dsDNA, which did not increase following acute infection. Therefore, lymphocyte activation elicited by T. cruzi is weaker than that induced by the gld mutation. Chronic infection of gld mice increased the production of antibodies against T. cruzi, dsDNA and histone. The reason for increased responses to nuclear autoantigens is unknown but could be related to increased lymphoproliferation precipitated by T. cruzi infection. Production of antibodies against nuclear autoantigens correlates with increased apoptosis (37). Infection with T. cruzi exacerbates lymphocyte apoptosis (38, 39), which is partially dependent on the Fas/FasL death pathway (40–43), but apoptosis is also increased in gld mice (40).
Studies with purified T. cruzi proteins amplify the ability of antibodies to detect antigen by employing recombinant antigens or serum from immunized hosts (44). Instead, our approach employed immunoblots of whole T. cruzi extracts to detect antibody diversity of animals infected with T. cruzi. In this way, we identified by proteomic analysis the most immunogenic T. cruzi proteins serving as targets for the antibody response. A very limited number of protein bands reacted with the sera. The most prominent reactivity was a 50–55 kDa band which colocalized with the most abundant protein band of the T. cruzi extract. This band was excised, digested, analyzed by mass spectrometry, and identified as TcβTUB. All sera from infected mice reacted strongly with TcβTUB. In addition, in spite of already detectable immunological abnormalities, all sera from acutely infected gld mice reacted strongly with TcβTUB. These results suggest a robust response. Two additional bands recognized by host IgG antibodies were identified as T. cruzi pyruvate phosphate dikinase (100 kDa) and T. cruzi Hsp-85 (85 kDa).
We investigated IgG reactivity against autologous heart extracts. Global IgG antibody reactivity against heart polypeptides did not differ between control and infected sera. We detected increased or induced IgM reactivities against a set of cardiac polypeptides were following infection (data not shown). These cardiac polypeptides deserve further characterization.
To investigate the immunogenicity of TcβTUB, we cloned the β-tubulin gene from T. cruzi Dm28c genomic DNA and expressed recombinant TcβTUB in E. coli. Recombinant TcβTUB reacted with monoclonal antibodies against β-tubulin, but not α-tubulin. Chronic infection increased IgG reactivity to TcβTUB, compared with uninfected mice. Immunization of mice with a single dose of TcβTUB in CFA protected against T. cruzi infection, as seen by markedly reduced parasitemia, compared with animals immunized with PBS or BSA. Although our data show a wide range in the levels of anti-β tubulin antibody title in the immunized group, we observed a correlation of host protective response with higher IgG reactivity against recombinant TcβTUB. Mice immunized with TcβTUB before infection also gave increased reactivity against parasite and mouse tubulins.
Although T. cruzi tubulin reacts with antibodies during infection, to our knowledge it has not been tested previously as an immunogen. Interestingly, immunizations of mice with native T. brucei tubulin or recombinant Trypanosoma evansi beta-tubulin confer broad spectrum protection against infection by African trypanosomes (22, 23, 25). Humoral and cellular mechanisms leading to immunoprotection following immunization with TcβTUB are unknown. TcβTUB is expressed both intracellularly and at the surface of live parasites (45). The majority of monoclonal antibodies reacting against the membrane of live T. cruzi parasites recognize a 50/55 kDa antigen related to tubulin (46). In addition, antibodies reactive against parasite tubulin cross-react with host tubulin (47). Infection with T. cruzi increases the amount and the affinity of naturally occurring antibodies against autologous tubulin and induces novel specificities against tubulin fragments (48, 49). We found slightly increased reactivity against mouse tubulin in the serum of mice immunized with TcβTUB. Additional studies are required to determine whether increased humoral reactivity against autologous tubulin plays any deleterious effect in the host. Taken together, our results indicate that non-biased identification of immunodominant parasite antigens through analysis of antibody diversity of infected hosts is a valid approach to identify candidate antigens for vaccines against Chagas disease.
Ethics Statement
This study was carried out in strict accordance with the recommendations in the Guide for the Care and Use of Laboratory Animals of the National Institutes of Health (USA). The protocol was approved by the Committee on the Ethics of Animal Experiments of the Health Science Center of the Federal University of Rio de Janeiro (CEUA-CCS, Permit Number: IBCCF 062/14), and all efforts were made to minimize suffering.
Author Contributions
Conceived and designed the experiments: CGF-de-L, GADR, FM, and DON. Performed the experiments: FM, DON, CK, NH and MN. Analyzed the data: FM, DON, GADR, RV, CGF-de-L, and MN. Contributed reagents/materials/analysis tools: LML, PMB. RV, LF-de-L, AM, MFL, SMT. AM, NH, GD, and CGF-de-L. Wrote the manuscript: GD, MN, CGF-de-L, and FM.
Conflict of Interest Statement
The authors declare that the research was conducted in the absence of any commercial or financial relationships that could be construed as a potential conflict of interest.
Acknowledgments
This work was supported by grants from Conselho Nacional de Desenvolvimento Científico e Tecnológico do Brasil (CNPq), Fundação de Amparo à Pesquisa do Estado do Rio de Janeiro (FAPERJ), and Programa Institutos Nacionais de Ciência e Tecnologia (INCT), CNPq, Brazil. FM was supported by CAPES/FAPERJ PNPD program. Technical assistance of Lindomar Miranda Silva to portion of this work is gratefully acknowledged. PMB, CGF-de-L, MFL, AM, SMT, NH and GADR are investigators from CNPq.
Supplementary Material
The Supplementary Material for this article can be found online at https://www.frontiersin.org/articles/10.3389/fimmu.2018.00671/full#supplementary-material.
References
1. Kurup SP, Tarleton RL. Perpetual expression of PAMPs necessary for optimal immune control and clearance of a persistent pathogen. Nat Commun (2013) 4:2616. doi:10.1038/ncomms3616
2. DosReis GA. Cell-mediated immunity in experimental Trypanosoma cruzi infection. Parasitol Today (1997) 13:335–42. doi:10.1016/S0169-4758(97)01073-9
3. Kierszenbaum F, Howard JG. Mechanisms of resistance against experimental Trypanosoma cruzi infection: the importance of antibodies and antibody-forming capacity in the biozzi high and low responder mice. J Immunol (1976) 116:1208–11.
4. Brodskyn CI, da Silva AM, Takehara HA, Mota I. Characterization of antibody isotype responsible for immune clearance in mice infected with Trypanosoma cruzi. Immunol Lett (1988) 18:255–8. doi:10.1016/0165-2478(88)90171-X
5. Scott MT. The nature of immunity against Trypanosoma cruzi in mice recovered from acute infection. Parasite Immunol (1981) 3:209–18. doi:10.1111/j.1365-3024.1981.tb00400.x
6. Kumar S, Tarleton RL. The relative contribution of antibody production and CD8+ T cell function to immune control of Trypanosoma cruzi. Parasite Immunol (1998) 20:207–16. doi:10.1046/j.1365-3024.1998.00154.x
7. Cazorla SI, Frank FM, Becker PD, Arnaiz M, Mirkin GA, Corral RS, et al. Redirection of the immune response to the functional catalytic domain of the cystein proteinase cruzipain improves protective immunity against Trypanosoma cruzi infection. J Infect Dis (2010) 202:136–44. doi:10.1086/652872
8. Fontanella GH, De Vusser K, Laroy W, Daurelio L, Nocito AL, Revelli S, et al. Immunization with an engineered mutant trans-sialidase highly protects mice from experimental Trypanosoma cruzi infection: a vaccine candidate. Vaccine (2008) 26:2322–34. doi:10.1016/j.vaccine.2008.02.060
9. Serna C, Lara JA, Rodrigues SP, Marques AF, Almeida IC, Maldonado RA. A synthetic peptide from Trypanosoma cruzi mucin-like associated surface protein as candidate for a vaccine against Chagas disease. Vaccine (2014) 32:3525–32. doi:10.1016/j.vaccine.2014.04.026
10. Ouaissi MA, Taibi A, Cornette J, Velge P, Marty B, Loyens M, et al. Characterization of major surface and excretory-secretory immunogens of Trypanosoma cruzi trypomastigotes and identification of potential protective antigen. Parasitology (1990) 100:115–24. doi:10.1017/S0031182000060182
11. San Francisco J, Barría I, Gutiérrez B, Neira I, Muñoz C, Sagua H, et al. Decreased cruzipain and gp85/trans-sialidase family protein expression contributes to loss of Trypanosoma cruzi trypomastigote virulence. Microbes Infect (2017) 19:55–61. doi:10.1016/j.micinf.2016.08.003
12. González-Marcano E, Acosta H, Mijares A, Concepción JL. Kinetic and molecular characterization of the pyruvate phosphate dikinase from Trypanosoma cruzi. Exp Parasitol (2016) 165:81–7. doi:10.1016/j.exppara.2016.03.023
13. Haury M, Grandien A, Sundblad A, Coutinho A, Nobrega A. Global analysis of antibody repertoires. 1. An immunoblot method for the quantitative screening of a large number of reactivities. Scand J Immunol (1994) 39:79–87. doi:10.1111/j.1365-3083.1994.tb03343.x
14. Ronda N, Haury M, Nobrega A, Kaveri SV, Coutinho A, Kazatchkine MD. Analysis of natural and disease-associated autoantibody repertoires: anti-endothelial cell IgG autoantibody activity in the serum of healthy individuals and patients with systemic lupus erythematosus. Int Immunol (1994) 6:1651–60. doi:10.1093/intimm/6.11.1651
15. Sundblad A, Ferreira C, Nobrega A, Haury M, Ferreira E, Padua F, et al. Characteristic generated alterations of autoantibody patterns in idiopathic thrombocytopenic purpura. J Autoimmun (1997) 10:193–201. doi:10.1006/jaut.1996.0116
16. Santos-Lima EC, Vasconcellos R, Reina-San-Martín B, Fesel C, Cordeiro-Da-Silva A, Berneman A, et al. Significant association between the skewed natural antibody repertoire of xid mice and resistance to Trypanosoma cruzi infection. Eur J Immunol (2001) 31:634–45. doi:10.1002/1521-4141(200102)31:2<634::AID-IMMU634>3.0.CO;2-H
17. Contreras VT, Salles JM, Thomas N, Morel CM, Goldenberg S. In vitro differentiation of Trypanosoma cruzi under chemically defined conditions. Mol Biochem Parasitol (1985) 16:315–27. doi:10.1016/0166-6851(85)90073-8
18. Lopes MF, Cunha JM, Bezerra FL, Gonzalez MS, Gomes JE, Lapa e Silva JR, et al. Trypanosoma cruzi: both chemically induced and triatomine-derived metacyclic trypomastigotes cause the same immunological disturbances in the infected mammalian host. Exp Parasitol (1995) 80:194–204. doi:10.1006/expr.1995.1024
19. Bartholomeu DC, Silva RA, Galvão LM, el-Sayed NM, Donelson JE, Teixeira SM. Trypanosoma cruzi: RNA structure and post-transcriptional control of tubulin gene expression. Exp Parasitol (2002) 102:123–33. doi:10.1016/S0014-4894(03)00034-1
20. Robinson D, Beattie R, Sherwin J, Gull K. Microtubules, tubulin and microtubule associated proteins of trypanosomes. Methods Enzymol (1991) 196:285–99. doi:10.1016/0076-6879(91)96027-O
21. Sueth-Santiago V, Decote-Ricardo D, Morrot A, Freire-de-Lima CG, Lima ME. Challenges in the chemotherapy of Chagas disease: looking for possibilities related to the differences and similarities between the parasite and host. World J Biol Chem (2017) 26:57–80. doi:10.4331/wjbc.v8.i1.57
22. Lubega GW, Byarugaba DK, Prichard RK. Immunization with a tubulin-rich preparation from Trypanosoma brucei confers broad protection against African trypanosomosis. Exp Parasitol (2002) 102:9–22. doi:10.1016/S0014-4894(02)00140-6
23. Li SQ, Fung MC, Reid SA, Inoue N, Lun ZR. Immunization with recombinant beta-tubulin from Trypanosoma evansi induced protection against T. evansi, T. equiperdum and T. b. brucei infection in mice. Parasite Immunol (2007) 29:191–9. doi:10.1111/j.1365-3024.2006.00933.x
24. Balaban N, Waithaka HK, Njogu AR, Goldman R. Intracellular antigens (microtubule-associated protein copurified with glycosomal enzymes) – possible vaccines against trypanosomiasis. J Infect Dis (1995) 172:845–50. doi:10.1093/infdis/172.3.845
25. Lubega GW, Ochola DO, Prichard RK. Trypanosoma brucei: anti-tubulin antibodies specifically inhibit trypanosome growth in culture. Exp Parasitol (2002) 102:134–42. doi:10.1016/S0014-4894(03)00035-3
26. Takahashi T, Tanaka M, Brannan CI, Jenkins NA, Copeland NG, Suda T, et al. Generalized lymphoproliferative disease in mice, caused by a point mutation in the fas ligand. Cell (1994) 25:76.
27. Kerbie DJ, Mattick JS. Touchdown PCR for increasing specificity and sensitivity in PCR amplification. Nat Protoc (2008) 3:1452–6. doi:10.1038/nprot.2008.133
28. Bradford MM. A rapid and sensitive method for the quantitation of microgram quantities of protein utilizing the principle of protein-dye binding. Anal Biochem (1976) 72:248–54. doi:10.1016/0003-2697(76)90527-3
29. Piperno G, LeDizet M, Chang XJ. Microtubules containing acetylated alpha-tubulin in mammalian cells in culture. J Cell Biol (1987) 104:289–302. doi:10.1083/jcb.104.2.289
30. Fukushima N, Furuta D, Hidaka Y, Moriyama R, Tsujiuchi T. Post-translational modifications of tubulin in the nervous system. J Neurochem (2009) 109:683–93. doi:10.1111/j.1471-4159.2009.06013.x
31. von Krüger WM, Lery LM, Soares MR, de Neves-Manta FS, Batista e Silva CM, Neves-Ferreira AG, et al. The phosphate-starvation response in Vibrio cholerae O1 and phoB mutant under proteomic analysis: disclosing functions involved in adaptation, survival and virulence. Proteomics (2006) 6:1495–511. doi:10.1002/pmic.200500238
32. Marinho CR, D’Império Lima MR, Grisotto MG, Alvarez JM. Influence of acute-phase parasite load on pathology, parasitism, and activation of the immune system at the late chronic phase of Chagas’ disease. Infect Immun (1999) 67:308–18.
33. d’Imperio Lima MR, Eisen H, Minoprio P, Joskowicz M, Coutinho A. Persistence of polyclonal B cell activation with undetectable parasitemia in late stages of experimental Chagas’ disease. J Immunol (1986) 137:353–6.
34. Bryan MA, Guyach SE, Norris KA. Specific humoral immunity versus polyclonal B cell activation in Trypanosoma cruzi infection of susceptible and resistant mice. PLoS Negl Trop Dis (2010) 4:e733. doi:10.1371/journal.pntd.0000733
35. Roths JB, Murphy ED, Eicher EM. A new mutation, gld, that produces lymphoproliferation and autoimmunity in C3H/HeJ mice. J Exp Med (1984) 159:1–20. doi:10.1084/jem.159.1.1
36. dos Santos DM, Talvani A, Guedes PM, Machado-Coelho GL, de Lana M, Bahia MT. Trypanosoma cruzi: genetic diversity influences the profile of immunoglobulins during experimental infection. Exp Parasitol (2009) 121:8–14. doi:10.1016/j.exppara.2008.09.012
37. Plotz PH. The autoantibody repertoire: searching for order. Nat Rev Immunol (2003) 3:73–8. doi:10.1038/nri976
38. Freire-de-Lima CG, Nascimento DO, Soares MB, Bozza PT, Castro-Faria-Neto HC, de Mello FG, et al. Uptake of apoptotic cells drives the growth of a pathogenic trypanosome in macrophages. Nature (2000) 13:199–203; Erratum in: Nature (2000) 20:904. doi:10.1038/35003208
39. Decote-Ricardo D, Nunes MP, Morrot A, Freire-de-Lima CG. Implication of apoptosis for the pathogenesis of Trypanosoma cruzi infection. Front Immunol (2017) 9:518. doi:10.3389/fimmu.2017.00518
40. Lopes MF, Nunes MP, Henriques-Pons A, Giese N, Morse HC III, Davidson WF, et al. Increased susceptibility of fas ligand-deficient gld mice to Trypanosoma cruzi infection due to a Th2-biased host immune response. Eur J Immunol (1999) 29:81–9. doi:10.1002/(SICI)1521-4141(199901)29:01<81::AID-IMMU81>3.0.CO;2-Y
41. Martins GA, Petkova SB, MacHado FS, Kitsis RN, Weiss LM, Wittner M, et al. Fas-FasL interaction modulates nitric oxide production in Trypanosoma cruzi-infected mice. Immunology (2001) 103:122–9. doi:10.1046/j.1365-2567.2001.01216.x
42. Zuniga E, Motran CC, Montes CL, Yagita H, Gruppi A. Trypanosoma cruzi infection selectively renders parasite-specific IgG+ B lymphocytes susceptible to Fas/Fas ligand-mediated fratricide. J Immunol (2002) 168:3965–73. doi:10.4049/jimmunol.168.8.3965
43. Guillermo LV, Silva EM, Ribeiro-Gomes FL, De Meis J, Pereira WF, Yagita H, et al. The Fas death pathway controls coordinated expansions of type 1 CD8 and type 2 CD4 T cells in Trypanosoma cruzi infection. J Leukoc Biol (2007) 81:942–51. doi:10.1189/jlb.1006643
44. Affranchino JL, Ibañez CF, Luquetti AO, Rassi A, Reyes MB, Macina RA, et al. Identification of a Trypanosoma cruzi antigen that is shed during the acute phase of Chagas’ disease. Mol Biochem Parasitol (1989) 34:221–8. doi:10.1016/0166-6851(89)90050-9
45. Alcina A, Hargreaves AJ, Avila J, Fresno M. A Trypanosoma cruzi monoclonal antibody that recognizes a superficial tubulin-like antigen. Biochem Biophys Res Commun (1986) 139:1176–83. doi:10.1016/S0006-291X(86)80301-1
46. Alcina A, Fresno M. A tubulin-related 55 kilodalton surface antigen recognized by different Trypanosoma cruzi stage-specific monoclonal antibodies from infected mice. Mol Biochem Parasitol (1988) 29:181–90. doi:10.1016/0166-6851(88)90073-4
47. Paulin JJ, Keith CH, Tarleton RL. A monoclonal antibody to alpha tubulin recognizes host cell and Trypanosoma cruzi tubulins. J Protozool (1988) 35:123–9. doi:10.1111/j.1550-7408.1988.tb04091.x
48. Ternynck T, Bleux C, Gregoire J, Avrameas S, Kanellopoulos-Langevin C. Comparison between autoantibodies arising during Trypanosoma cruzi infection in mice and natural autoantibodies. J Immunol (1990) 144:1504–11.
Keywords: Trypanosoma cruzi, antibody repertoires, beta-tubulin, lymphocyte activation, Chagas disease
Citation: Montalvão F, Nascimento DO, Nunes MP, Koeller CM, Morrot A, Lery LMS, Bisch PM, Teixeira SMR, Vasconcellos R, Freire-de-Lima L, Lopes MF, Heise N, DosReis GA and Freire-de-Lima CG (2018) Antibody Repertoires Identify β-Tubulin as a Host Protective Parasite Antigen in Mice Infected With Trypanosoma cruzi. Front. Immunol. 9:671. doi: 10.3389/fimmu.2018.00671
Received: 23 November 2017; Accepted: 19 March 2018;
Published: 13 April 2018
Edited by:
Amy Rasley, Lawrence Livermore National Laboratory (DOE), United StatesReviewed by:
Phileno Pinge-Filho, Universidade Estadual de Londrina, BrazilEden Ramalho Ferreira, Federal University of São Paulo, Brazil
Copyright: © 2018 Montalvão, Nascimento, Nunes, Koeller, Morrot, Lery, Bisch, Teixeira, Vasconcellos, Freire-de-Lima, Lopes, Heise, DosReis and Freire-de-Lima. This is an open-access article distributed under the terms of the Creative Commons Attribution License (CC BY). The use, distribution or reproduction in other forums is permitted, provided the original author(s) and the copyright owner are credited and that the original publication in this journal is cited, in accordance with accepted academic practice. No use, distribution or reproduction is permitted which does not comply with these terms.
*Correspondence: Célio Geraldo Freire-de-Lima, celio@biof.ufrj.br;
George A. DosReis, gdosreis@biof.ufrj.br
†These authors have contributed equally to this work.