- 1VIB Laboratory of Translational Immunomodulation, Center for Inflammation Research, Hasselt University, Diepenbeek, Belgium
- 2Biomedical Research Institute, Hasselt University, and School of Life Sciences, Transnationale Universiteit Limburg, Hasselt, Belgium
B cells possess a predominant role in adaptive immune responses via antibody-dependent and -independent functions. The microbiome of the gastrointestinal tract is currently being intensively investigated due to its profound impact on various immune responses, including B cell maturation, activation, and IgA antibody responses. Recent findings have demonstrated the interplay between dietary components, gut microbiome, and autoantibody production. “Western” dietary patterns, such as high fat and high salt diets, can induce alterations in the gut microbiome that in turn affects IgA responses and the production of autoantibodies. This could contribute to multiple pathologies including autoimmune and inflammatory diseases. Here, we summarize current knowledge on the influence of various dietary components on B cell function and (auto)antibody production in relation to the gut microbiota, with a particular focus on the gut–brain axis in the pathogenesis of multiple sclerosis.
Introduction
B cells are involved in humoral and cell-mediated immunity. They secrete antibodies following differentiation into plasma cells, produce cytokines, and regulate T cell responses via antigen presentation and costimulation (1–3). B cells develop in the bone marrow from hematopoietic stem cells to immature B cells that further mature in the periphery into transitional and mature naïve B cells (4). Following activation, short-lived plasma cells are generated that produce low-affinity immunoglobulin (Ig)M antibodies for a few days (4). A fraction of the responding B cells undergoes a germinal center response, which results in the generation of memory B cells and long-lived Ig class-switched plasma cells that produce high-affinity IgG, IgA, or IgE antibodies.
Autoantibodies can originate from autoreactive B cells that escape tolerance mechanisms following molecular mimicry of infectious antigens with autoantigens, bystander activation, novel autoantigen presentation, or recognition of circulating autoantigens. They can clear target cells via antibody-dependent cell-mediated cytotoxicity or complement activation (5, 6). In addition, B cells are highly effective antigen-presenting cells, effectively activating antigen-specific CD4+ T helper (Th) cells (2, 7). Depending on the cytokine profile, B cells can stimulate pro- and anti-inflammatory immune responses (8–10).
The humoral immune response in the gastrointestinal tract is mediated by IgA+ memory B cells and IgA-producing plasma cells in the gut-associated lymphoid tissue (GALT). The protective and nutrient-rich environment of the gastrointestinal tract accommodates an extremely dense and diverse bacterial community (11) that in turn provides metabolic advantages and serves as a natural defense against colonization with pathogens (12, 13). Commensal bacteria act as critical stimuli, playing an important role for the maturation of the GALT and further induce IgA production by B cells (14). Class switching to IgA-producing plasma cells occurs in the Peyer’s patches and lamina propria, following T cell-dependent or -independent mechanisms (15). The secreted IgA (SIgA) into the gut provides a first-line defense against pathogens mainly by blocking toxins and pathogens from adhering to the intestinal epithelium at the earliest steps of the infection process (16).
In this review, we describe the interrelation of dietary components, microbiome and B cell function with a focus on the production of (auto)antibodies. Special emphasis is placed on multiple sclerosis (MS) and its animal model experimental autoimmune encephalomyelitis (EAE).
Dietary Influences on B Cell Homeostasis and Function
Modern nutritional patterns, collectively termed “Western-diet,” are characterized by high energy density, animal protein, total and saturated fats, sugars and salt but low levels of plant-derived fibers. This “Western-diet” has a profound influence on the prevalence of autoantibodies, although changes in antibody-independent B cell functions have been reported as well. Additionally, a “Western-diet” may influence the balanced composition of the gut microbiome leading to perturbed immune responses, including effects on B cell production, activity, and maturation (17, 18) (Figure 1).
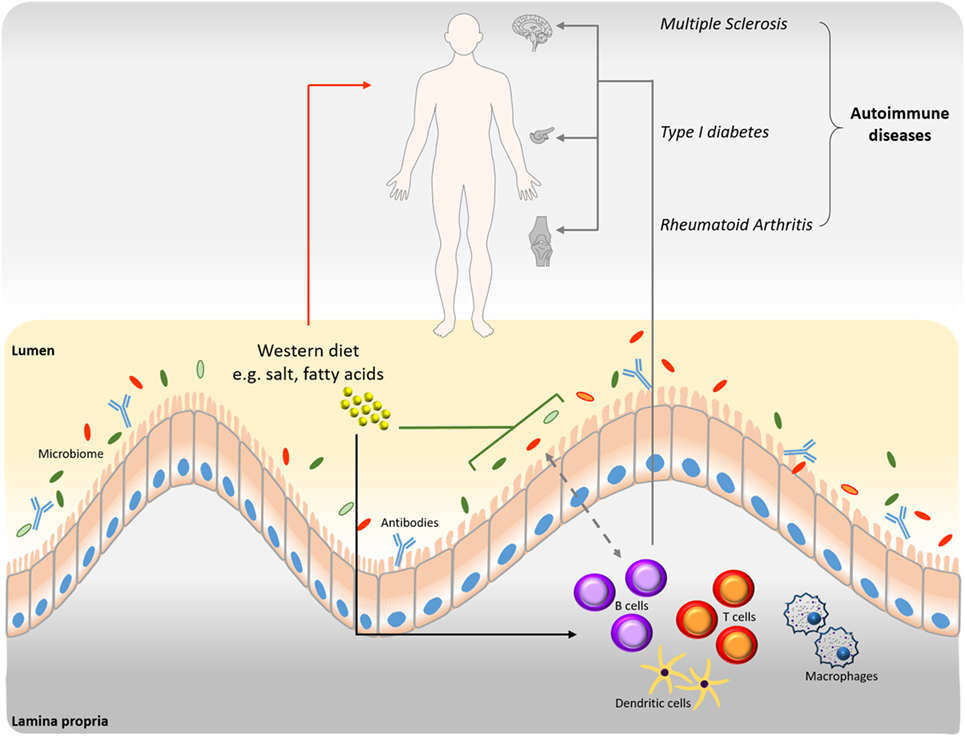
Figure 1. Interrelation among B cells, microbiome, and diet in disease progression. Western type nutritional patterns influence the composition of the intestinal microbiome (green line). Alterations of the gut microbiome induced by nutrient components impact homeostasis and the onset of various diseases (red arrow). Western diet dietary components influence B cell function and production of autoantibodies (black arrow), which are involved in disease progression (gray arrows). The connection between B cells and microbiome is bidirectional (dashed gray arrow). B cell-derived antibodies modulate the intestinal microbiome and vice versa.
Effects of a high-fat diet (HFD) on B cell function have mostly been studied in diet-induced obesity models. Here, B cells contribute to pro-inflammatory reactions in the adipose tissue mediating insulin insensitivity and diminished glucose clearance. More specifically, B cells secrete pathogenic IgG antibodies and pro-inflammatory cytokines, which could interfere with macrophage polarization, CD4+ T cell function, such as regulatory T cell inhibition or Th17 cell polarization, and CD8+ T cell activation (19, 20). Reduced systemic antibody production has been demonstrated following influenza infection and/or ex vivo stimulation in a HFD-induced obesity mouse model and in obese individuals (21–23). Underlying mechanisms could involve effects on the responding plasma cells and molecular deregulation. Yet, autoreactive and pro-inflammatory antibodies were increased in obese humans and HFD-fed mice (20, 24, 25), probably through CD40 ligand (CD40L) signaling. CD40L has been shown to induce inflammatory cytokine production in adipose cells in vitro and in vivo (26, 27). The increased natural autoreactive IgM antibodies under HFD formed an immune complex with apoptosis inhibitor of macrophage, which promoted IgG autoantibody production (28). Increased B cell frequencies and IgG levels were found in mouse obese white adipose tissue and obese humans, who additionally demonstrated a positive correlation between IgM levels and body mass index (21). Furthermore, obese humans displayed reduced IL-10+ regulatory B cell levels in subcutaneous adipose tissue, which could contribute to the occurrence of autoantibodies (29). Mouse models further indicated diverse roles for different B cell subtypes in obesity-associated pro-inflammatory responses (20, 29–31). Thus, B cells might play a crucial role in secondary inflammation following obesity and constitute a potential therapeutic target in diet-induced obesity.
High-fat diet also induces changes in the gut microbiota that are related to the development of obesity and diabetes. Obesity is associated with a decreased intestinal abundance of Bacteroidetes and an increased proportion of Firmicutes, both in mice and humans (32–34). It has been shown that germ-free (GF) mice may be protected against diet-induced obesity and the mechanism possibly involves the fasting-induced adipose factor (Fiaf) in the intestinal epithelium and the AMP-activated protein kinase in skeletal muscle and liver (35). In addition, colonization of GF mice with microbiota harvested from conventionally raised animals resulted in a 60% increase in body fat content and insulin resistance (36). However, more research is necessary to unravel the link between HFD-mediated alterations of gut microbiota and B cell function or autoantibody production.
Another factor associated with “Western-diet” is the high salt content in processed foods and so-called “fast foods.” High salt diets have been shown to exert profound effects on animal models of autoimmunity by affecting Th cell populations and macrophages promoting a pro-inflammatory environment (37, 38). However, if high salt also affects B cells is less well understood. Hybridoma cells under hyperosmotic stress exert suppressed cell growth but enhanced specific antibody production (39–41). Increased differentiation of Th17 and follicular helper T (Tfh) cells was demonstrated following a high salt diet in EAE and a lupus mouse model (42, 43). Tfh cells are involved in the selection of high-affinity B cells during the germinal center response. The mechanism involved in the high salt-mediated Th17 activation is dependent on nuclear factor of activated T cells 5 (NFAT5), p38/MAPK, and the serum/glucocorticoid-regulated kinase 1 (SGK1). SGK1 expression is induced upon salt treatment and its activation depends on p38/MAPK. Silencing of SGK1 reverts the effect of salt on IL-17 levels. To exclude the possibility that high osmolarity mediates the enhanced Th17 pro-inflammatory profile, mannitol and MgCl2 were tested along and proved to have only a slight effect (42). Furthermore, high salt conditions result in cellular osmotic stress that is regulated via the guanine nucleotide exchange factor Brx-induced expression of NFAT5 (44). Interestingly, Brx was shown to be necessary for B cell differentiation in high salt conditions via NFAT5-mediated production of B cell activating factor (BAFF) that regulates splenic B cell differentiation and Ig production. A recent study described the correlation between salt intake and gut microbiome changes in EAE. More specifically, salt intake decreased the population of Lactobacillus murinus, while supplementation of L. murinus reduced the salt-induced EAE clinical scores and Th17 cell frequencies (45).
By contrast, dietary supplementation with n − 3 polyunsaturated fatty acids (PUFAs) derived from fish oils could impact B cell function and suppress pro-inflammatory responses (46). Results from mouse models for obesity, colitis, peritonitis, and systemic lupus erythematosus indicated that dietary administration of fish oil containing n-3 PUFAs elevated splenic B cell numbers, increased B cell cytokine and IgM production while reducing autoantibodies (47–51). Monthly consumption of fish oil by postpartum women led to lower levels of anti-thyroid autoantibodies (52). In individuals at risk for rheumatoid arthritis, the use of n-3 PUFA food supplements and n-3 PUFA levels in red blood cell membranes were inversely associated with anti-cyclic citrullinated peptide and rheumatoid factor positivity (53, 54). Specialized pro-resolving lipid mediators (SPMs) that are endogenously synthesized from n-3 and n-6 PUFAs play a role in suppressing adipose tissue inflammation. In obese humans, selected SPMs were declined in adipose tissue (55). 17-hydroxydosahexaenoic acid (17-HDHA), DHA and resolving D1 stimulated increased Ig production in humans or mice with diet-induced obesity (21, 56). Furthermore, DHA and eicosapentaenoic acid (EPA) induced differential effects on B cell cytokine production and on distinct B cell subtypes that correlated with increased natural serum IgM and cecal IgA in murine obesity (57). Opposed to this, lipoxin A4 decreased (antigen-specific) IgM and IgG production and inhibited memory B cell function in an ovalbumin immunized mouse model (58). Thus, n − 3 PUFAs and their derived SPMs can have profound effects on B cell function. More research is needed to clarify the differential effects associated with different types of PUFAs and to mechanistically link the effects to inflammation in obesity. Of note, in MS, EPA and DHA had no beneficial effects on disease activity (OFAMS study) (59).
Furthermore, a diet rich in short-chain fatty acids (SCFAs) could positively impact gut microbiota and inflammatory processes (37). The microbiome converts non-digestible carbohydrates (dietary fibers) to SCFAs, including acetate, butyrate, and propionate, which reduce the risk of inflammatory diseases, type 2 diabetes, obesity, heart disease, and other conditions (60). Non-obese diabetic mice on a diet rich in acetate were characterized by decreased IL-12-producing marginal zone B cells, a B cell subtype linked to the disruption of immune tolerance, in the spleen and the Payer’s patches that additionally showed decreased expression of major histocompatibility complex I and CD86 (61). At the transcriptional level, changes were detected in genes associated with B cell costimulation, antigen presentation, proliferation, and differentiation. Thus, SCFAs and in particular acetate could affect the ability of B cells to expand autoreactive T cells in vivo and the development of type 1 diabetes. Butyrate was also suggested to protect against the development of anti-islet cell autoantibodies involved in type 1 diabetes (62). Early introduction of a non-milk diet in infants increased the risk for autoantibody production by reduced butyrate production and was associated with high Bacteroides levels. A milk-based diet resulted in a competitive advantage of acetogens compared to sulfate reducing bacteria, thereby leading to increased butyrate production via co-fermentation of acetate.
Dietary components such as gluten (63, 64), selenium (65), and iodine [reviewed in Ref. (66)] have been shown to increase autoantibody production. Additionally, impaired protein intake alters IgA responses, attenuating the protective efficacy of vaccination against cholera and Salmonella enterica serovar Typhimurium in mice (67). On the contrary, a cocoa-rich diet decreases autoantibody production and confers beneficial immune function (68–72).
Cross-Talk Between Microbiome and B Cells
Studies in various animal models of impaired microbial control [including GF, antibiotic-treated mice, mice with restricted flora and activation-induced cytidine deaminase knockout (AID−/−) mice], but also in humans, have demonstrated that gastrointestinal bacteria participate in B cell differentiation, maturation, and activation (73–76). A proof-of-principle study in Pakistani infants living in impoverished areas showed an accelerated maturation of the salivary IgA system compared with healthy Swedish infants (77). In contrast, in Swedish infants, the gut microbiota took longer to establish and was characterized by a lower diversity (78–80). B cell maturation in Swedish infants was shaped by the intestinal bacterial colonization pattern, mainly by Escherichia coli and Bifidobacteria (74).
On the other hand, intestinal IgA can influence the gut microbiota composition. Natural and specific IgA antibodies in breast milk were capable of binding commensal bacteria and might be involved in establishing the newborn’s microbiome (81). High-affinity IgA, generated via T cell-dependent mechanisms, was essential in mice for the protection from invasive commensal species, such as segmented filamentous bacteria (SFB), and from true pathogens, such as Salmonella typhimurium and Enterobacter cloacae (82). Of note, SFB increased IgA+ B cells in vivo (83, 84). Moreover, SIgA promoted the establishment of host–microbial relationships by modulating bacterial epitopes and modifying bacterial metabolism, as demonstrated by the downregulation of bacterial genes involved in the metabolism of oxidative products, i.e., Bacteroides thetaiotaomicron (85). An alternative mechanism proposed a mouse monoclonal IgA which was reactive against multiple commensal but not beneficial bacteria by specific recognition of an epitope in serine hydroxymethyltransferase, a bacterial metabolic enzyme (86). Oral administration of this IgA antibody in vivo effectively prevented the development of colitis in several mouse models (86, 87).
Probiotics, live microbial food ingredients, have been demonstrated to affect B cell function by stimulating systemic and mucosal IgA production in humans (88, 89). More specifically, probiotic strains such as Bifidobacterium lactis and Saccharomyces boulardii enhanced IgA production through alteration of the gut mucosa cytokine milieu in preterm infants and mice, respectively (90, 91). Probiotic bacteria can induce TGF-β, IL-10, and IL-6 expression by epithelial cells, which potentiate IgA production through B cell maturation and class switching to IgA (92, 93). Finally, probiotics augment the expression of polymeric Ig receptors on the basolateral surface of intestinal epithelial cells enhancing IgA transcytosis into the gut lumen (94). Not only supplementation of preterm infants with B. lactis but also administration of Lactobacillus casei in mice resulted in increased IgA-producing cells (95, 96). Pretreatment of mice with the Bifidobacterium species B. bifidum and B. infantis increased gut mucosal pathogen-specific IgA antibody titers and reduced illness after challenge with rotavirus (97, 98). Similar results were described in infant rabbits and gnotobiotic pigs, pinpointing the effects of several commensals on IgA production (99, 100). Dietary components, shown to directly affect microbiome composition with subsequent influence on human’s immunity and health, include proteins, fats, carbohydrates, and polyphenols. Data from clinical studies prove that plant-derived proteins, non-digestible carbohydrates (prebiotics), and restricted fat consumption and polyphenols increase the intestinal numbers of beneficial bacteria such as Bifodobacterium and Lactobacillus. Interestingly, contrary to animal-derived proteins that can lead to inflammatory bowel disease and cardiovascular diseases, plant-derived proteins increase SCFAs and regulatory T cells, counteracting inflammatory responses (101).
Therefore, a “Western-diet” lacking components of high nutrient value such as probiotics may negatively modulate immune responses, thereby leading to decreased immune tolerance as well as disease and infection progression.
Interplay of B Cells and Microbiome in MS
B cells are important players in MS pathogenesis via antibody-dependent and -independent mechanisms (1). Bidirectional trafficking of B cells has been demonstrated between the periphery and CNS, where they could locally produce (auto)antibodies (102). IgA antibodies, that mediate humoral immunity in the gastrointestinal tract, have been described to play a role in MS as well. Increased serum IgA antibodies directed against myelin basic protein (MBP), myelin oligodendrocyte glycoprotein (MOG), plant and human aquaporins, and S100B have been described in MS (103, 104). Anti-MBP IgA antibodies were able to catalyze MBP hydrolysis, which could contribute to demyelination (105). Intrathecal IgG, IgA, and IgM synthesis correlated with the presence of anti-MBP or anti-proteolipid protein-secreting cells (106). Interestingly, IgA antibodies were found to be associated with a progressive disease course (103). More specifically, cerebrospinal fluid (CSF) IgA synthesis was correlated with the yearly disease progression rate in primary progressive MS (107). In addition, IgA antibodies directed against gliadin, gluten, and casein were increased in MS patients (108). In the CNS, IgA was reported as a major component of immune responses in MS with IgA+ plasma cells showing signs of clonal expansion, intraclonal diversification, and anti-axonal reactivity (109–111). An important correlation was found between CSF levels of chemokine C-X-C motif ligand (CXCL)13 and the extent of intrathecal IgA synthesis (112).
In addition, mounting evidence highlights the implication of the gut environment in MS onset and progression. Recently, microbiome analysis indicated altered levels of several commensals in MS patients (113–115). Possible mechanisms employed by microbiota to induce MS could potentially include low-grade microbial translocation such as peptidoglycan, a bacterial cell wall component, from the gut to the CNS (115, 116). Additionally, gut microbiota can lead to disruption of the blood–brain barrier (117), microglia activation (118), limited astrocyte pathogenicity (119), and expression of myelinating genes (120). Interestingly, microbiota transplantation of MS patients to GF mice resulted in more severe EAE symptoms and reduced IL-10+ regulatory T cells compared to mice transplanted with selected healthy human microbiomes (113, 121). Commensal microbiota is necessary for disease development in spontaneous and actively induced EAE models (122, 123). MOG-immunized GF mice showed reduced anti-MOG antibodies that could be increased by colonization with microbiota from MS-affected twins. Furthermore, GF housing conditions resulted in impaired B cell recruitment to brain-draining lymph nodes and reduced MOG-specific IgG2a antibodies in spontaneously developing EAE (121). In line with this, an antibiotic mixture orally administered before EAE induction impaired EAE development due to increased regulatory T cells in the mesenteric and cervical lymph nodes and increased IL-10-producing CD5+ B cells in cervical lymph nodes (124). The induced B cells were able to reduce EAE severity when adoptively transferred into naïve recipient mice by causing a shift from a Th1/Th17 toward a Th2 cytokine profile (125). Thus, antibiotic treatment stimulated both regulatory T and B cells, which both contributed to the protection against EAE.
In addition, dietary interventions have been tested in EAE models. A prophylactic diet of 66% caloric restriction protected Lewis rats from developing EAE as evident by reduced splenic CD8+ T cells and B cells, lymphoid and thymic CD4+ T cells and B cells, and IFN-γ production (126). Other dietary interventions that demonstrated efficacy in reducing EAE symptoms are SCFAs, low fat diets, and zinc aspartate (127). However, currently no information is available on the effects of these dietary components on B cell function or autoantibody production. Moreover, some vitamins, i.e., A, E, and D are important immune regulators and have been shown to limit EAE progression (116). Of note, vitamin D, which is mainly produced by sun exposure but is also contained in food such as salmon, beef meet, and egg yolks, has been shown to decrease EAE manifestations in vivo. Administration of 1,25-dihydroxyvitamin D3 to mice, the active form of vitamin D, prevented EAE development and significantly reduced serum anti-MBP antibody production (128). In MS patients, different dietary interventions have been studied although mostly unsuccessful or causality could not be demonstrated (116). However, preliminary data from a recent study indicated that a fasting mimicking diet or chronic ketogenic diet could be safe, feasible, and potentially effective in MS treatment (129).
Conclusion
Increasing evidence is being gathered for the interplay between diet, microbiome, and autoantibody production. Deregulation of this system could contribute to different pathologies, including MS. A “Western-diet” consisting among others of high fat and high salt content has been associated with increased autoantibody production, obesity, inflammatory disorders, and autoimmune diseases. Gut bacteria have been shown to modulate B cell differentiation, maturation, and activation with a profound influence on IgA responses (Figure 1). Dietary interventions and the use of probiotics could restore immune deregulation that is seen in case of diet-induced microbiome alterations. They thus may represent valuable tools for improving the treatment of inflammatory and autoimmune disorders. However, more research is needed to clarify the mechanisms underlying the effects of dietary components on autoantibody production and its relation to disease development in order to obtain a more efficient and preventive treatment line. In MS patients, IgA antibodies against several autoantigens have been described. Additionally, a disturbed microbiome has been observed in MS patients and animal studies have supported a possible link between the microbiome and the disease. However, the exact role of diet and the microbiome in B cell-mediated pathology in MS, along with the respective mechanisms, remain to be determined.
Author Contributions
IP, JF, VS, and MK wrote the manuscript. All authors approved the work for publication.
Conflict of Interest Statement
The authors declare the absence of any commercial or financial relationships that could be construed as a potential conflict of interest.
Funding
MK was supported by the European Research Council (ERC) under the European Union’s Horizon 2020 research and innovation program (640116), by a SALK-grant from the government of Flanders, Belgium, and by an Odysseus grant of the Research Foundation Flanders, Belgium (FWO). JF is a postdoctoral fellow of the FWO.
References
1. Claes N, Fraussen J, Stinissen P, Hupperts R, Somers V. B cells are multifunctional players in multiple sclerosis pathogenesis: insights from therapeutic interventions. Front Immunol (2015) 6:642. doi:10.3389/fimmu.2015.00642
2. Fraussen J, Claes N, Van Wijmeersch B, van Horssen J, Stinissen P, Hupperts R, et al. B cells of multiple sclerosis patients induce autoreactive proinflammatory T cell responses. Clin Immunol (2016) 173:124–32. doi:10.1016/j.clim.2016.10.001
3. Rajewsky K. Clonal selection and learning in the antibody system. Nature (1996) 381(6585):751–8. doi:10.1038/381751a0
4. Pieper K, Grimbacher B, Eibel H. B-cell biology and development. J Allergy Clin Immunol (2013) 131(4):959–71. doi:10.1016/j.jaci.2013.01.046
5. Bhakdi S, Tranum-Jensen J. Complement lysis: a hole is a hole. Immunol Today (1991) 12(9):318–20. doi:10.1016/0167-5699(91)90007-G
6. Esser AF. Big MAC attack: complement proteins cause leaky patches. Immunol Today (1991) 12(9):316–8. doi:10.1016/0167-5699(91)90006-F
7. Lanzavecchia A. Antigen-specific interaction between T and B cells. Nature (1985) 314(6011):537–9. doi:10.1038/314537a0
8. Bar-Or A, Fawaz L, Fan B, Darlington PJ, Rieger A, Ghorayeb C, et al. Abnormal B-cell cytokine responses a trigger of T-cell-mediated disease in MS? Ann Neurol (2010) 67(4):452–61. doi:10.1002/ana.21939
9. Fillatreau S, Sweenie CH, McGeachy MJ, Gray D, Anderton SM. B cells regulate autoimmunity by provision of IL-10. Nat Immunol (2002) 3(10):944–50. doi:10.1038/ni833
10. Shen P, Roch T, Lampropoulou V, O’Connor RA, Stervbo U, Hilgenberg E, et al. IL-35-producing B cells are critical regulators of immunity during autoimmune and infectious diseases. Nature (2014) 507(7492):366–70. doi:10.1038/nature12979
11. Sonnenburg JL, Angenent LT, Gordon JI. Getting a grip on things: how do communities of bacterial symbionts become established in our intestine? Nat Immunol (2004) 5(6):569–73. doi:10.1038/ni1079
12. O’Hara AM, Shanahan F. The gut flora as a forgotten organ. EMBO Rep (2006) 7(7):688–93. doi:10.1038/sj.embor.7400731
13. Turnbaugh PJ, Ley RE, Hamady M, Fraser-Liggett CM, Knight R, Gordon JI. The human microbiome project. Nature (2007) 449(7164):804–10. doi:10.1038/nature06244
14. Round JL, Mazmanian SK. The gut microbiota shapes intestinal immune responses during health and disease. Nat Rev Immunol (2009) 9(5):313–23. doi:10.1038/nri2515
15. Suzuki K, Ha SA, Tsuji M, Fagarasan S. Intestinal IgA synthesis: a primitive form of adaptive immunity that regulates microbial communities in the gut. Semin Immunol (2007) 19(2):127–35. doi:10.1016/j.smim.2006.10.001
16. Helander A, Miller CL, Myers KS, Neutra MR, Nibert ML. Protective immunoglobulin A and G antibodies bind to overlapping intersubunit epitopes in the head domain of type 1 reovirus adhesin sigma1. J Virol (2004) 78(19):10695–705. doi:10.1128/JVI.78.19.10695-10705.2004
17. Shaikh SR, Haas KM, Beck MA, Teague H. The effects of diet-induced obesity on B cell function. Clin Exp Immunol (2015) 179(1):90–9. doi:10.1111/cei.12444
18. Conlon MA, Bird AR. The impact of diet and lifestyle on gut microbiota and human health. Nutrients (2014) 7(1):17–44. doi:10.3390/nu7010017
19. DeFuria J, Belkina AC, Jagannathan-Bogdan M, Snyder-Cappione J, Carr JD, Nersesova YR, et al. B cells promote inflammation in obesity and type 2 diabetes through regulation of T-cell function and an inflammatory cytokine profile. Proc Natl Acad Sci U S A (2013) 110(13):5133–8. doi:10.1073/pnas.1215840110
20. Winer DA, Winer S, Shen L, Wadia PP, Yantha J, Paltser G, et al. B cells promote insulin resistance through modulation of T cells and production of pathogenic IgG antibodies. Nat Med (2011) 17(5):610–7. doi:10.1038/nm.2353
21. Kosaraju R, Guesdon W, Crouch MJ, Teague HL, Sullivan EM, Karlsson EA, et al. B cell activity is impaired in human and mouse obesity and is responsive to an essential fatty acid upon murine influenza infection. J Immunol (2017) 198(12):4738–52. doi:10.4049/jimmunol.1601031
22. Milner JJ, Sheridan PA, Karlsson EA, Schultz-Cherry S, Shi Q, Beck MA. Diet-induced obese mice exhibit altered heterologous immunity during a secondary 2009 pandemic H1N1 infection. J Immunol (2013) 191(5):2474–85. doi:10.4049/jimmunol.1202429
23. Sheridan PA, Paich HA, Handy J, Karlsson EA, Hudgens MG, Sammon AB, et al. Obesity is associated with impaired immune response to influenza vaccination in humans. Int J Obes (Lond) (2012) 36(8):1072–7. doi:10.1038/ijo.2011.208
24. Marzullo P, Minocci A, Tagliaferri MA, Guzzaloni G, Di Blasio A, De Medici C, et al. Investigations of thyroid hormones and antibodies in obesity: leptin levels are associated with thyroid autoimmunity independent of bioanthropometric, hormonal, and weight-related determinants. J Clin Endocrinol Metab (2010) 95(8):3965–72. doi:10.1210/jc.2009-2798
25. Rosenbloom AL. Obesity, insulin resistance, beta-cell autoimmunity, and the changing clinical epidemiology of childhood diabetes. Diabetes Care (2003) 26(10):2954–6. doi:10.2337/diacare.26.10.2954
26. Wolf D, Jehle F, Ortiz Rodriguez A, Dufner B, Hoppe N, Colberg C, et al. CD40L deficiency attenuates diet-induced adipose tissue inflammation by impairing immune cell accumulation and production of pathogenic IgG-antibodies. PLoS One (2012) 7(3):e33026. doi:10.1371/journal.pone.0033026
27. Missiou A, Wolf D, Platzer I, Ernst S, Walter C, Rudolf P, et al. CD40L induces inflammation and adipogenesis in adipose cells – a potential link between metabolic and cardiovascular disease. Thromb Haemost (2010) 103(4):788–96. doi:10.1160/TH09-07-0463
28. Arai S, Maehara N, Iwamura Y, Honda S, Nakashima K, Kai T, et al. Obesity-associated autoantibody production requires AIM to retain the immunoglobulin M immune complex on follicular dendritic cells. Cell Rep (2013) 3(4):1187–98. doi:10.1016/j.celrep.2013.03.006
29. Nishimura S, Manabe I, Takaki S, Nagasaki M, Otsu M, Yamashita H, et al. Adipose natural regulatory B cells negatively control adipose tissue inflammation. Cell Metab (2013) S1550–4131(13):00386. doi:10.1016/j.cmet.2013.09.017
30. van Dam AD, van Beek L, Pronk ACM, van den Berg SM, Van den Bossche J, de Winther MPJ, et al. IgG is elevated in obese white adipose tissue but does not induce glucose intolerance via Fcgamma-receptor or complement. Int J Obes (Lond) (2017) 42(2):260–9. doi:10.1038/ijo.2017.209
31. Nus M, Sage AP, Lu Y, Masters L, Lam BYH, Newland S, et al. Marginal zone B cells control the response of follicular helper T cells to a high-cholesterol diet. Nat Med (2017) 23(5):601–10. doi:10.1038/nm.4315
32. Ley RE, Backhed F, Turnbaugh P, Lozupone CA, Knight RD, Gordon JI. Obesity alters gut microbial ecology. Proc Natl Acad Sci U S A (2005) 102(31):11070–5. doi:10.1073/pnas.0504978102
33. Turnbaugh PJ, Backhed F, Fulton L, Gordon JI. Diet-induced obesity is linked to marked but reversible alterations in the mouse distal gut microbiome. Cell Host Microbe (2008) 3(4):213–23. doi:10.1016/j.chom.2008.02.015
34. Turnbaugh PJ, Ley RE, Mahowald MA, Magrini V, Mardis ER, Gordon JI. An obesity-associated gut microbiome with increased capacity for energy harvest. Nature (2006) 444(7122):1027–31. doi:10.1038/nature05414
35. Backhed F, Manchester JK, Semenkovich CF, Gordon JI. Mechanisms underlying the resistance to diet-induced obesity in germ-free mice. Proc Natl Acad Sci U S A (2007) 104(3):979–84. doi:10.1073/pnas.0605374104
36. Backhed F, Ding H, Wang T, Hooper LV, Koh GY, Nagy A, et al. The gut microbiota as an environmental factor that regulates fat storage. Proc Natl Acad Sci U S A (2004) 101(44):15718–23. doi:10.1073/pnas.0407076101
37. Jorg S, Grohme DA, Erzler M, Binsfeld M, Haghikia A, Muller DN, et al. Environmental factors in autoimmune diseases and their role in multiple sclerosis. Cell Mol Life Sci (2016) 73(24):4611–22. doi:10.1007/s00018-016-2311-1
38. Manzel A, Muller DN, Hafler DA, Erdman SE, Linker RA, Kleinewietfeld M. Role of “Western diet” in inflammatory autoimmune diseases. Curr Allergy Asthma Rep (2014) 14(1):404. doi:10.1007/s11882-013-0404-6
39. Bibila TA, Ranucci CS, Glazomitsky K, Buckland BC, Aunins JG. Monoclonal antibody process development using medium concentrates. Biotechnol Prog (1994) 10(1):87–96. doi:10.1021/bp00025a011
40. Reddy S, Bauer KD, Miller WM. Determination of antibody content in live versus dead hybridoma cells: analysis of antibody production in osmotically stressed cultures. Biotechnol Bioeng (1992) 40(8):947–64. doi:10.1002/bit.260400811
41. Soo Ryu J, Min Lee G. Effect of hypoosmotic stress on hybridoma cell growth and antibody production. Biotechnol Bioeng (1997) 55(3):565–70. doi:10.1002/(SICI)1097-0290(19970805)55:3<565::AID-BIT14>3.0.CO;2-F
42. Kleinewietfeld M, Manzel A, Titze J, Kvakan H, Yosef N, Linker RA, et al. Sodium chloride drives autoimmune disease by the induction of pathogenic TH17 cells. Nature (2013) 496(7446):518–22. doi:10.1038/nature11868
43. Wu H, Huang X, Qiu H, Zhao M, Liao W, Yuan S, et al. High salt promotes autoimmunity by TET2-induced DNA demethylation and driving the differentiation of Tfh cells. Sci Rep (2016) 6:28065. doi:10.1038/srep28065
44. Kino T, Takatori H, Manoli I, Wang Y, Tiulpakov A, Blackman MR, et al. Brx mediates the response of lymphocytes to osmotic stress through the activation of NFAT5. Sci Signal (2009) 2(57):ra5. doi:10.1126/scisignal.2000081
45. Wilck N, Matus MG, Kearney SM, Olesen SW, Forslund K, Bartolomaeus H, et al. Salt-responsive gut commensal modulates TH17 axis and disease. Nature (2017) 551(7682):585–9. doi:10.1038/nature24628
46. Kim J, Li Y, Watkins BA. Fat to treat fat: emerging relationship between dietary PUFA, endocannabinoids, and obesity. Prostaglandins Other Lipid Mediat (2013) 10(4–105):32–41. doi:10.1016/j.prostaglandins.2012.11.005
47. Gurzell EA, Teague H, Harris M, Clinthorne J, Shaikh SR, Fenton JI. DHA-enriched fish oil targets B cell lipid microdomains and enhances ex vivo and in vivo B cell function. J Leukoc Biol (2013) 93(4):463–70. doi:10.1189/jlb.0812394
48. Pestka JJ, Vines LL, Bates MA, He K, Langohr I. Comparative effects of n-3, n-6 and n-9 unsaturated fatty acid-rich diet consumption on lupus nephritis, autoantibody production and CD4+ T cell-related gene responses in the autoimmune NZBWF1 mouse. PLoS One (2014) 9(6):e100255. doi:10.1371/journal.pone.0100255
49. Rockett BD, Salameh M, Carraway K, Morrison K, Shaikh SR. n-3 PUFA improves fatty acid composition, prevents palmitate-induced apoptosis, and differentially modifies B cell cytokine secretion in vitro and ex vivo. J Lipid Res (2010) 51(6):1284–97. doi:10.1194/jlr.M000851
50. Teague H, Fhaner CJ, Harris M, Duriancik DM, Reid GE, Shaikh SR. n-3 PUFAs enhance the frequency of murine B-cell subsets and restore the impairment of antibody production to a T-independent antigen in obesity. J Lipid Res (2013) 54(11):3130–8. doi:10.1194/jlr.M042457
51. Tomasdottir V, Thorleifsdottir S, Vikingsson A, Hardardottir I, Freysdottir J. Dietary omega-3 fatty acids enhance the B1 but not the B2 cell immune response in mice with antigen-induced peritonitis. J Nutr Biochem (2014) 25(2):111–7. doi:10.1016/j.jnutbio.2013.09.010
52. Benvenga S, Vigo MT, Metro D, Granese R, Vita R, Le Donne M. Type of fish consumed and thyroid autoimmunity in pregnancy and postpartum. Endocrine (2016) 52(1):120–9. doi:10.1007/s12020-015-0698-3
53. Gan RW, Demoruelle MK, Deane KD, Weisman MH, Buckner JH, Gregersen PK, et al. Omega-3 fatty acids are associated with a lower prevalence of autoantibodies in shared epitope-positive subjects at risk for rheumatoid arthritis. Ann Rheum Dis (2017) 76(1):147–52. doi:10.1136/annrheumdis-2016-209154
54. Gan RW, Young KA, Zerbe GO, Demoruelle MK, Weisman MH, Buckner JH, et al. Lower omega-3 fatty acids are associated with the presence of anti-cyclic citrullinated peptide autoantibodies in a population at risk for future rheumatoid arthritis: a nested case-control study. Rheumatology (Oxford) (2016) 55(2):367–76. doi:10.1093/rheumatology/kev266
55. Claria J, Dalli J, Yacoubian S, Gao F, Serhan CN. Resolvin D1 and resolvin D2 govern local inflammatory tone in obese fat. J Immunol (2012) 189(5):2597–605. doi:10.4049/jimmunol.1201272
56. Ramon S, Gao F, Serhan CN, Phipps RP. Specialized proresolving mediators enhance human B cell differentiation to antibody-secreting cells. J Immunol (2012) 189(2):1036–42. doi:10.4049/jimmunol.1103483
57. Teague H, Harris M, Fenton J, Lallemand P, Shewchuk BM, Shaikh SR. Eicosapentaenoic and docosahexaenoic acid ethyl esters differentially enhance B-cell activity in murine obesity. J Lipid Res (2014) 55(7):1420–33. doi:10.1194/jlr.M049809
58. Ramon S, Bancos S, Serhan CN, Phipps RP. Lipoxin A(4) modulates adaptive immunity by decreasing memory B-cell responses via an ALX/FPR2-dependent mechanism. Eur J Immunol (2014) 44(2):357–69. doi:10.1002/eji.201343316
59. Torkildsen O, Wergeland S, Bakke S, Beiske AG, Bjerve KS, Hovdal H, et al. omega-3 fatty acid treatment in multiple sclerosis (OFAMS Study): a randomized, double-blind, placebo-controlled trial. Arch Neurol (2012) 69(8):1044–51. doi:10.1001/archneurol.2012.283
60. Rios-Covian D, Ruas-Madiedo P, Margolles A, Gueimonde M, de Los Reyes-Gavilan CG, Salazar N. Intestinal short chain fatty acids and their link with diet and human health. Front Microbiol (2016) 7:185. doi:10.3389/fmicb.2016.00185
61. Marino E, Richards JL, McLeod KH, Stanley D, Yap YA, Knight J, et al. Gut microbial metabolites limit the frequency of autoimmune T cells and protect against type 1 diabetes. Nat Immunol (2017) 18(5):552–62. doi:10.1038/ni.3713
62. Endesfelder D, Engel M, Davis-Richardson AG, Ardissone AN, Achenbach P, Hummel S, et al. Towards a functional hypothesis relating anti-islet cell autoimmunity to the dietary impact on microbial communities and butyrate production. Microbiome (2016) 4:17. doi:10.1186/s40168-016-0163-4
63. Kumar J, Kumar M, Pandey R, Chauhan NS. Physiopathology and management of gluten-induced celiac disease. J Food Sci (2017) 82(2):270–7. doi:10.1111/1750-3841.13612
64. Lebwohl B, Sanders DS, Green PHR. Coeliac disease. Lancet (2017) 391(10115):70–81. doi:10.1016/S0140-6736(17)31796-8
65. McLachlan SM, Aliesky H, Banuelos B, Que Hee SS, Rapoport B. Variable effects of dietary selenium in mice that spontaneously develop a spectrum of thyroid autoantibodies. Endocrinology (2017) 158(11):3754–64. doi:10.1210/en.2017-00275
66. Burek CL, Talor MV. Environmental triggers of autoimmune thyroiditis. J Autoimmun (2009) 33(3–4):183–9. doi:10.1016/j.jaut.2009.09.001
67. Rho S, Kim H, Shim SH, Lee SY, Kim MJ, Yang BG, et al. Protein energy malnutrition alters mucosal IgA responses and reduces mucosal vaccine efficacy in mice. Immunol Lett (2017) 190:247–56. doi:10.1016/j.imlet.2017.08.025
68. Abril-Gil M, Massot-Cladera M, Perez-Cano FJ, Castellote C, Franch A, Castell M. A diet enriched with cocoa prevents IgE synthesis in a rat allergy model. Pharmacol Res (2012) 65(6):603–8. doi:10.1016/j.phrs.2012.02.001
69. Abril-Gil M, Perez-Cano FJ, Franch A, Castell M. Effect of a cocoa-enriched diet on immune response and anaphylaxis in a food allergy model in Brown Norway rats. J Nutr Biochem (2016) 27:317–26. doi:10.1016/j.jnutbio.2015.09.022
70. Camps-Bossacoma M, Massot-Cladera M, Abril-Gil M, Franch A, Perez-Cano FJ, Castell M. Cocoa diet and antibody immune response in preclinical studies. Front Nutr (2017) 4:28. doi:10.3389/fnut.2017.00028
71. Perez-Berezo T, Ramiro-Puig E, Perez-Cano FJ, Castellote C, Permanyer J, Franch A, et al. Influence of a cocoa-enriched diet on specific immune response in ovalbumin-sensitized rats. Mol Nutr Food Res (2009) 53(3):389–97. doi:10.1002/mnfr.200700396
72. Ramos-Romero S, Perez-Cano FJ, Castellote C, Castell M, Franch A. Effect of cocoa-enriched diets on lymphocytes involved in adjuvant arthritis in rats. Br J Nutr (2012) 107(3):378–87. doi:10.1017/S0007114511003035
73. Fagarasan S, Muramatsu M, Suzuki K, Nagaoka H, Hiai H, Honjo T. Critical roles of activation-induced cytidine deaminase in the homeostasis of gut flora. Science (2002) 298(5597):1424–7. doi:10.1126/science.1077336
74. Lundell AC, Bjornsson V, Ljung A, Ceder M, Johansen S, Lindhagen G, et al. Infant B cell memory differentiation and early gut bacterial colonization. J Immunol (2012) 188(9):4315–22. doi:10.4049/jimmunol.1103223
75. Ouwehand A, Isolauri E, Salminen S. The role of the intestinal microflora for the development of the immune system in early childhood. Eur J Nutr (2002) 41(Suppl 1):I32–7. doi:10.1007/s00394-002-1105-4
76. Wei B, Su TT, Dalwadi H, Stephan RP, Fujiwara D, Huang TT, et al. Resident enteric microbiota and CD8+ T cells shape the abundance of marginal zone B cells. Eur J Immunol (2008) 38(12):3411–25. doi:10.1002/eji.200838432
77. Mellander L, Carlsson B, Jalil F, Soderstrom T, Hanson LA. Secretory IgA antibody response against Escherichia coli antigens in infants in relation to exposure. J Pediatr (1985) 107(3):430–3. doi:10.1016/S0022-3476(85)80528-X
78. Adlerberth I, Carlsson B, de Man P, Jalil F, Khan SR, Larsson P, et al. Intestinal colonization with Enterobacteriaceae in Pakistani and Swedish hospital-delivered infants. Acta Paediatr Scand (1991) 80(6–7):602–10. doi:10.1111/j.1651-2227.1991.tb11917.x
79. Adlerberth I, Wold AE. Establishment of the gut microbiota in Western infants. Acta Paediatr (2009) 98(2):229–38. doi:10.1111/j.1651-2227.2008.01060.x
80. Nowrouzian F, Hesselmar B, Saalman R, Strannegard IL, Aberg N, Wold AE, et al. Escherichia coli in infants’ intestinal microflora: colonization rate, strain turnover, and virulence gene carriage. Pediatr Res (2003) 54(1):8–14. doi:10.1203/01.PDR.0000069843.20655.EE
81. Sekirov I, Russell SL, Antunes LC, Finlay BB. Gut microbiota in health and disease. Physiol Rev (2010) 90(3):859–904. doi:10.1152/physrev.00045.2009
82. Harris NL, Spoerri I, Schopfer JF, Nembrini C, Merky P, Massacand J, et al. Mechanisms of neonatal mucosal antibody protection. J Immunol (2006) 177(9):6256–62. doi:10.4049/jimmunol.177.9.6256
83. Glenn JD, Mowry EM. Emerging concepts on the gut microbiome and multiple sclerosis. J Interferon Cytokine Res (2016) 36(6):347–57. doi:10.1089/jir.2015.0177
84. Wekerle H, Berer K, Krishnamoorthy G. Remote control-triggering of brain autoimmune disease in the gut. Curr Opin Immunol (2013) 25(6):683–9. doi:10.1016/j.coi.2013.09.009
85. Peterson DA, McNulty NP, Guruge JL, Gordon JI. IgA response to symbiotic bacteria as a mediator of gut homeostasis. Cell Host Microbe (2007) 2(5):328–39. doi:10.1016/j.chom.2007.09.013
86. Okai S, Usui F, Ohta M, Mori H, Kurokawa K, Matsumoto S, et al. Intestinal IgA as a modulator of the gut microbiota. Gut Microbes (2017) 8(5):486–92. doi:10.1080/19490976.2017.1310357
87. Okai S, Usui F, Yokota S, Hori IY, Hasegawa M, Nakamura T, et al. High-affinity monoclonal IgA regulates gut microbiota and prevents colitis in mice. Nat Microbiol (2016) 1(9):16103. doi:10.1038/nmicrobiol.2016.103
88. Fukushima Y, Kawata Y, Hara H, Terada A, Mitsuoka T. Effect of a probiotic formula on intestinal immunoglobulin A production in healthy children. Int J Food Microbiol (1998) 42(1–2):39–44. doi:10.1016/S0168-1605(98)00056-7
89. Kaila M, Isolauri E, Soppi E, Virtanen E, Laine S, Arvilommi H. Enhancement of the circulating antibody secreting cell response in human diarrhea by a human Lactobacillus strain. Pediatr Res (1992) 32(2):141–4. doi:10.1203/00006450-199208000-00002
90. Mohan R, Koebnick C, Schildt J, Mueller M, Radke M, Blaut M. Effects of Bifidobacterium lactis Bb12 supplementation on body weight, fecal pH, acetate, lactate, calprotectin, and IgA in preterm infants. Pediatr Res (2008) 64(4):418–22. doi:10.1203/PDR.0b013e318181b7fa
91. Rodrigues AC, Cara DC, Fretez SH, Cunha FQ, Vieira EC, Nicoli JR, et al. Saccharomyces boulardii stimulates sIgA production and the phagocytic system of gnotobiotic mice. J Appl Microbiol (2000) 89(3):404–14. doi:10.1046/j.1365-2672.2000.01128.x
92. He B, Xu W, Santini PA, Polydorides AD, Chiu A, Estrella J, et al. Intestinal bacteria trigger T cell-independent immunoglobulin A(2) class switching by inducing epithelial-cell secretion of the cytokine APRIL. Immunity (2007) 26(6):812–26. doi:10.1016/j.immuni.2007.04.014
93. Shang L, Fukata M, Thirunarayanan N, Martin AP, Arnaboldi P, Maussang D, et al. Toll-like receptor signaling in small intestinal epithelium promotes B-cell recruitment and IgA production in lamina propria. Gastroenterology (2008) 135(2):529–38. doi:10.1053/j.gastro.2008.04.020
94. Resendiz-Albor AA, Reina-Garfias H, Rojas-Hernandez S, Jarillo-Luna A, Rivera-Aguilar V, Miliar-Garcia A, et al. Regionalization of pIgR expression in the mucosa of mouse small intestine. Immunol Lett (2010) 128(1):59–67. doi:10.1016/j.imlet.2009.11.005
95. Galdeano CM, Perdigon G. The probiotic bacterium Lactobacillus casei induces activation of the gut mucosal immune system through innate immunity. Clin Vaccine Immunol (2006) 13(2):219–26. doi:10.1128/CVI.13.2.219-226.2006
96. Holscher HD, Faust KL, Czerkies LA, Litov R, Ziegler EE, Lessin H, et al. Effects of prebiotic-containing infant formula on gastrointestinal tolerance and fecal microbiota in a randomized controlled trial. JPEN J Parenter Enteral Nutr (2012) 36(1 Suppl):95S–105S. doi:10.1177/0148607111430087
97. Qiao H, Duffy LC, Griffiths E, Dryja D, Leavens A, Rossman J, et al. Immune responses in rhesus rotavirus-challenged BALB/c mice treated with Bifidobacteria and prebiotic supplements. Pediatr Res (2002) 51(6):750–5. doi:10.1203/00006450-200206000-00015
98. Shu Q, Gill HS. A dietary probiotic (Bifidobacterium lactis HN019) reduces the severity of Escherichia coli O157:H7 infection in mice. Med Microbiol Immunol (2001) 189(3):147–52. doi:10.1007/s430-001-8021-9
99. Ogawa M, Shimizu K, Nomoto K, Takahashi M, Watanuki M, Tanaka R, et al. Protective effect of Lactobacillus casei strain Shirota on Shiga toxin-producing Escherichia coli O157:H7 infection in infant rabbits. Infect Immun (2001) 69(2):1101–8. doi:10.1128/IAI.69.2.1101-1108.2001
100. Zhang W, Azevedo MS, Gonzalez AM, Saif LJ, Van Nguyen T, Wen K, et al. Influence of probiotic lactobacilli colonization on neonatal B cell responses in a gnotobiotic pig model of human rotavirus infection and disease. Vet Immunol Immunopathol (2008) 122(1–2):175–81. doi:10.1016/j.vetimm.2007.10.003
101. Singh RK, Chang HW, Yan D, Lee KM, Ucmak D, Wong K, et al. Influence of diet on the gut microbiome and implications for human health. J Transl Med (2017) 15(1):73. doi:10.1186/s12967-017-1175-y
102. Stern JN, Yaari G, Vander Heiden JA, Church G, Donahue WF, Hintzen RQ, et al. B cells populating the multiple sclerosis brain mature in the draining cervical lymph nodes. Sci Transl Med (2014) 6(248):248ra107. doi:10.1126/scitranslmed.3008879
103. Egg R, Reindl M, Deisenhammer F, Linington C, Berger T. Anti-MOG and anti-MBP antibody subclasses in multiple sclerosis. Mult Scler (2001) 7(5):285–9. doi:10.1191/135245801681137979
104. Vojdani A, Mukherjee PS, Berookhim J, Kharrazian D. Detection of antibodies against human and plant aquaporins in patients with multiple sclerosis. Autoimmune Dis (2015) 2015:905208. doi:10.1155/2015/905208
105. Polosukhina DI, Buneva VN, Doronin BM, Tyshkevich OB, Boiko AN, Gusev EI, et al. Hydrolysis of myelin basic protein by IgM and IgA antibodies from the sera of patients with multiple sclerosis. Med Sci Monit (2005) 11(8):BR266–72.
106. Sellebjerg F, Christiansen M, Garred P. MBP, anti-MBP and anti-PLP antibodies, and intrathecal complement activation in multiple sclerosis. Mult Scler (1998) 4(3):127–31. doi:10.1191/135245898678909475
107. Abdelhak A, Hottenrott T, Mayer C, Hintereder G, Zettl UK, Stich O, et al. CSF profile in primary progressive multiple sclerosis: re-exploring the basics. PLoS One (2017) 12(8):e0182647. doi:10.1371/journal.pone.0182647
108. Reichelt KL, Jensen D. IgA antibodies against gliadin and gluten in multiple sclerosis. Acta Neurol Scand (2004) 110(4):239–41. doi:10.1111/j.1600-0404.2004.00303.x
109. Link H, Muller R. Immunoglobulins in multiple sclerosis and infections of the nervous system. Arch Neurol (1971) 25(4):326–44. doi:10.1001/archneur.1971.00490040052007
110. Olsson JE, Link H. Immunoglobulin abnormalities in multiple sclerosis. Relation to clinical parameters: exacerbations and remissions. Arch Neurol (1973) 28(6):392–9. doi:10.1001/archneur.1973.00490240052009
111. Zhang Y, Da RR, Hilgenberg LG, Tourtellotte WW, Sobel RA, Smith MA, et al. Clonal expansion of IgA-positive plasma cells and axon-reactive antibodies in MS lesions. J Neuroimmunol (2005) 167(1–2):120–30. doi:10.1016/j.jneuroim.2005.05.006
112. Kowarik MC, Cepok S, Sellner J, Grummel V, Weber MS, Korn T, et al. CXCL13 is the major determinant for B cell recruitment to the CSF during neuroinflammation. J Neuroinflammation (2012) 9:93. doi:10.1186/1742-2094-9-93
113. Cekanaviciute E, Yoo BB, Runia TF, Debelius JW, Singh S, Nelson CA, et al. Gut bacteria from multiple sclerosis patients modulate human T cells and exacerbate symptoms in mouse models. Proc Natl Acad Sci U S A (2017) 114(40):10713–8. doi:10.1073/pnas.1711235114
114. Jangi S, Gandhi R, Cox LM, Li N, von Glehn F, Yan R, et al. Alterations of the human gut microbiome in multiple sclerosis. Nat Commun (2016) 7:12015. doi:10.1038/ncomms12015
115. Mirza A, Mao-Draayer Y. The gut microbiome and microbial translocation in multiple sclerosis. Clin Immunol (2017) 183:213–24. doi:10.1016/j.clim.2017.03.001
116. van den Hoogen WJ, Laman JD, t Hart BA. Modulation of multiple sclerosis and its animal model experimental autoimmune encephalomyelitis by food and gut microbiota. Front Immunol (2017) 8:1081. doi:10.3389/fimmu.2017.01081
117. Braniste V, Al-Asmakh M, Kowal C, Anuar F, Abbaspour A, Toth M, et al. The gut microbiota influences blood-brain barrier permeability in mice. Sci Transl Med (2014) 6(263):263ra158. doi:10.1126/scitranslmed.3009759
118. Erny D, Hrabe de Angelis AL, Jaitin D, Wieghofer P, Staszewski O, David E, et al. Host microbiota constantly control maturation and function of microglia in the CNS. Nat Neurosci (2015) 18(7):965–77. doi:10.1038/nn.4030
119. Rothhammer V, Mascanfroni ID, Bunse L, Takenaka MC, Kenison JE, Mayo L, et al. Type I interferons and microbial metabolites of tryptophan modulate astrocyte activity and central nervous system inflammation via the aryl hydrocarbon receptor. Nat Med (2016) 22(6):586–97. doi:10.1038/nm.4106
120. Hoban AE, Stilling RM, Ryan FJ, Shanahan F, Dinan TG, Claesson MJ, et al. Regulation of prefrontal cortex myelination by the microbiota. Transl Psychiatry (2016) 6:e774. doi:10.1038/tp.2016.42
121. Berer K, Gerdes LA, Cekanaviciute E, Jia X, Xiao L, Xia Z, et al. Gut microbiota from multiple sclerosis patients enables spontaneous autoimmune encephalomyelitis in mice. Proc Natl Acad Sci U S A (2017) 114(40):10719–24. doi:10.1073/pnas.1711233114
122. Le Berre L, Rousse J, Gourraud PA, Imbert-Marcille BM, Salama A, Evanno G, et al. Decrease of blood anti-alpha1,3 galactose Abs levels in multiple sclerosis (MS) and clinically isolated syndrome (CIS) patients. Clin Immunol (2017) 180:128–35. doi:10.1016/j.clim.2017.05.006
123. Lee YK, Menezes JS, Umesaki Y, Mazmanian SK. Proinflammatory T-cell responses to gut microbiota promote experimental autoimmune encephalomyelitis. Proc Natl Acad Sci U S A (2011) 108(Suppl 1):4615–22. doi:10.1073/pnas.1000082107
124. Ochoa-Reparaz J, Mielcarz DW, Ditrio LE, Burroughs AR, Foureau DM, Haque-Begum S, et al. Role of gut commensal microflora in the development of experimental autoimmune encephalomyelitis. J Immunol (2009) 183(10):6041–50. doi:10.4049/jimmunol.0900747
125. Ochoa-Reparaz J, Mielcarz DW, Haque-Begum S, Kasper LH. Induction of a regulatory B cell population in experimental allergic encephalomyelitis by alteration of the gut commensal microflora. Gut Microbes (2010) 1(2):103–8. doi:10.4161/gmic.1.2.11515
126. Esquifino AI, Cano P, Jimenez-Ortega V, Fernandez-Mateos MP, Cardinali DP. Immune response after experimental allergic encephalomyelitis in rats subjected to calorie restriction. J Neuroinflammation (2007) 4:6. doi:10.1186/1742-2094-4-6
127. Haghikia A, Jorg S, Duscha A, Berg J, Manzel A, Waschbisch A, et al. Dietary fatty acids directly impact central nervous system autoimmunity via the small intestine. Immunity (2015) 43(4):817–29. doi:10.1016/j.immuni.2015.09.007
128. Lemire JM, Archer DC. 1,25-dihydroxyvitamin D3 prevents the in vivo induction of murine experimental autoimmune encephalomyelitis. J Clin Invest (1991) 87(3):1103–7. doi:10.1172/JCI115072
Keywords: B cells, autoantibodies, diet, microbiome, multiple sclerosis, experimental autoimmune encephalomyelitis
Citation: Petta I, Fraussen J, Somers V and Kleinewietfeld M (2018) Interrelation of Diet, Gut Microbiome, and Autoantibody Production. Front. Immunol. 9:439. doi: 10.3389/fimmu.2018.00439
Received: 22 December 2017; Accepted: 19 February 2018;
Published: 06 March 2018
Edited by:
Ralf J. Ludwig, University of Lübeck, GermanyReviewed by:
Anne L. Astier, UMR5282 Centre de Physiopathologie de Toulouse Purpan (CPTP), FranceMichael Kogut, Agricultural Research Service (USDA), United States
Copyright: © 2018 Petta, Fraussen, Somers and Kleinewietfeld. This is an open-access article distributed under the terms of the Creative Commons Attribution License (CC BY). The use, distribution or reproduction in other forums is permitted, provided the original author(s) and the copyright owner are credited and that the original publication in this journal is cited, in accordance with accepted academic practice. No use, distribution or reproduction is permitted which does not comply with these terms.
*Correspondence: Veerle Somers, dmVlcmxlLnNvbWVycyYjeDAwMDQwO3VoYXNzZWx0LmJl;
Markus Kleinewietfeld, bWFya3VzLmtsZWluZXdpZXRmZWxkJiN4MDAwNDA7dWhhc3NlbHQudmliLmJl
†These authors have contributed equally to this work.