- 1Department of Immunology, School of Basic Medical Sciences, Fudan University, Shanghai, China
- 2Department of Integrative Medicine and Neurobiology, School of Basic Medical Sciences; Institute of Acupuncture and Moxibustion, Fudan Institutes of Integrative Medicine, Fudan University, Shanghai, China
- 3Deutsches Rheuma-Forschungszentrum Berlin, Berlin, Germany
- 4Department of Immunology, Medical Research Institute, Tokyo Medical and Dental University, Tokyo, Japan
The marginal zone B cells (MZB) are located at the interface between the circulation and lymphoid tissue and as a gatekeeper play important roles in both innate and adaptive immune responses. We have previously found that MZB are significantly reduced in mice deficient in the IgM Fc receptor (FcμR) but how FcμR regulates the development and function of MZB remains unknown. In this study, we found that both marginal zone precursor (MZP) and MZB were decreased in FcμR−/− mice. The reduction of MZP and MZB was not due to impaired proliferation of these cells but rather due to their increased death. Further analysis revealed that FcμR−/− MZB had reduced tonic BCR signal, as evidenced by their decreased levels of phosphorylated SYK and AKT relative to WT MZB. MZB in FcμR−/− mice responded poorly to LPS in vivo when compared with MZB in WT mice. Consistent with the reduced proportion of MZB and their impaired response to LPS, antibody production against the type 1 T-independent Ag, NP-LPS, was significantly reduced in FcμR−/− mice. Moreover, FcμR−/− mice were highly susceptible to Citrobacter rodentium-induced sepsis. These results reveal a critical role for FcμR in the survival and activation of MZB and in protection against acute bacterial infection.
Introduction
Mature B lymphocytes can be divided into B1, follicular B (FOB), and marginal zone B cells (MZB) (1). MZB are distinguished from FOB in that they are non-circulating mature B cells and located as a gatekeeper at the interface between the circulation and lymphoid tissues. MZB can efficiently bind to the blood-borne antigens (Ag) and rapidly differentiate into antibody-secreting plasmablasts in the presence of costimulatory signals from innate or adaptive immune cells (2). MZB can also transport blood-borne Ag to B cell follicles to initiate adaptive responses. Therefore, this innate-like B cell subset helps to bridge the spatiotemporal gap between the innate immunity and a primary, T-cell dependent, adaptive antibody response (3). Besides, unlike FOB that primarily express monoreactive BCR, MZB express polyreactive BCR that can recognize multiple microbial molecular patterns (4–6).
Marginal zone B cells are thought to derive from bone marrow and not fully formed until 2–3 weeks after birth in rodents (7). Currently, it is considered that MZB mainly develop through the following stages: transitional B1 (T1), transitional B2 (T2), MZ precursor (MZP), and finally MZB (1, 8–10). MZB fate decision depends on BCR (8), Notch2 (11, 12), and BAFF receptor (BAFFR) signaling (13). BCR signaling might be integrated with BAFFR and Notch2 signaling during the commitment to MZB or FOB lineage (14–17).
Marginal zone B cells and FOB exhibit different gene expression profiles (18, 19), which contribute to their differential localization and function. MZB have an IgMhighIgDlowCD21highCD23−CD1dhigh phenotype (20), which is distinct from FOB that are IgMlowIgDhighCD21lowCD23highCD1d−. MZB also express higher levels of MHC class II, CD80, and CD86 compared with FOB (6, 21). Moreover, our previous study shows that both MZB and FOB express Fcμ receptor (FcμR) (22), a bona fide FcμR specific for pentameric IgM (23, 24). FcμR is predominantly expressed by B cells in mice and B, T, and NK cells in humans (22–27). In addition, FcμR has been shown to regulate the activation of monocytes, macrophages, and granulocytes (28), the differentiation and activation of dendritic cells (29, 30), and the function of human T and NK cells (31). Both Honjo et al. (25) and Ouchida et al. (22) demonstrated that mice lacking FcμR exhibited reduced MZB population, elevated serum IgM levels, impaired humoral immune responses to a T-dependent Ag and autoantibody production. More recently, Nguyen et al. generated B cell-specific FcμR-deficient mice and found that FcμR constrained surface BCR expression and its absence in B cells resulted in elevated BCR levels and enhanced tonic BCR signaling (27). They also found increased numbers of B-1 cells in the spleen, which showed enhanced activation and differentiation into antibody-secreting cells (27). Consistent with previous studies (22, 25), mice with B cell-specific FcμR deficiency produced autoantibodies and exhibited reduced antiviral IgG production (27, 32). We have recently found that FcμR interacts and cooperates with the BCR to promote the survival of splenic B cells in mice (33). Clinically, FcμR is highly expressed on B cell chronic lymphocytic leukemia (23, 34–36), which suggests a role for FcμR in promoting the survival of such malignant cells. Intriguingly, MZB are significantly decreased in the spleen of FcμR−/− mice (22, 25, 37). It has been suggested that reduced numbers of MZB in FcμR−/− mice resulted from their rapid differentiation into plasma cells (37) but how FcμR regulates MZB development and function remains unknown.
In this study, we have analyzed the role of FcμR in the development, survival, and activation of MZB. We found a reduction of both MZP and MZB in FcμR−/− mice from 6 weeks of age when compared with WT mice. The reduction of MZB in FcμR−/− mice was associated with an increased death of these cells. Further analysis revealed that FcμR−/− MZB had reduced tonic BCR signal. FcμR−/− MZB had impaired response in vivo to LPS and consistently the mutant mice exhibited a severe impairment in antibody production against the T-independent (T-I) Ag NP-LPS. FcμR−/− mice were also highly susceptible to bacterial sepsis. These results demonstrate a critical role for FcμR in tonic BCR signaling in MZB and their survival and LPS response, and in protection against acute bacterial infection.
Materials and Methods
Mice
WT and FcμR−/− mice (22) were maintained in specific pathogen-free conditions in the Department of Laboratory Animal Science, Fudan University. All animal experiments were approved by the Animal Committee of the School of Basic Medical Sciences, Fudan University. Mice under 12 weeks of age were used for all the experiments.
Flow Cytometry (FACS)
Mouse spleen or bone marrow were obtained from euthanized mice and single-cell suspension was prepared after lysing the red blood cells. For analyzing FcμR expression in various B-cell subpopulations, cells were first incubated with aggregated human IgG (prepared by incubating the antibody in a 60°C water bath for 30 min and then chilling in ice water) to block all FcγRs and then stained with biotin-MM3 anti-FcμR mAb (25, 37), followed by PE-labeled streptavidin. For staining with other specific mAbs, cells were first incubated with rat IgG2b anti-mouse CD16/CD32 monoclonal antibody (clone 2.4G2; BD Biosciences) to block FcγR and then stained with FITC-, PE-, allophyocyanin-, PerCP-Cyanine5.5-, or PE/Cy7-conjugated antibodies against various surface molecules expressed during B cell development and differentiation (38). For intracellular staining, cells were fixed in 2% paraformaldehyde at 37°C for 10 min and then blocked with rat IgG2b anti-mouse CD16/CD32 for 20 min. The cells were then stained with antibodies against phosphorylated SYK (pSYK) (pY348) and AKY (pS473) (diluted in 1× PBS containing 10% FBS and 0.1% Triton X-100) for 20 min in the dark, and washed with 1× PBS containing 4% FBS. The stained cells were analyzed on a FACSVerse flow cytometer (BD Biosciences) using the FACSuite software. Antibodies used in this study are listed in Table S1 in Supplementary Material.
Immunofluorescence
Whole spleens were frozen in Tissue-Tek OCT compound (SAKURA). Cryosections (8-µm thick) were mounted onto slides, air dried for 30 min, fixed in ice-cold acetone/methanol (1:1) for 10 min, rehydrated in PBS, and blocked for 60 min with blocking buffer (PBS containing 1% BSA and 0.1% Tween 20), followed by three 5-min washes in PBS. Sections were stained with FITC-conjugated rat anti-mouse B220 (BD Biosciences) and biotinylated rat anti-mouse metallophilic macrophage (MOMA-1, MCA947G; Serotec) diluted in blocking buffer, incubated at 4°C overnight, and then stained with Cy3-labeled streptavidin at room temperature for 1 h, followed by three 5-min washes in PBS. The slides were finally mounted with low fluorescent glycerol and coverslip protection, and observed with a Nikon Eclipse Ti-S inverted microscope.
EdU Incorporation Assay
EdU incorporation was performed using a Click-iT EdU Alexa Fluor kit (C10424, ThermoFisher) according to the manufacturer’s instruction. Briefly, WT and FcμR−/− mice (12 weeks old) were injected intraperitoneal (i.p.) with 200 µl of (1 mg/ml) EdU. EdU incorporation was analyzed 24 h later by FACS.
Immunization and ELISA Assay
These experiments were performed essentially as described elsewhere (38, 39).
Infection Experiment
Citrobacter rodentium suspended in 200 µl of PBS or PBS alone were injected into the tail vein of WT and FcμR−/− mice as described previously (40) and monitored for their survival.
Statistical Analysis
Statistical significance was assessed by an unpaired t-test or log-rank test (*p < 0.05; **p < 0.01; ***p < 0.005).
Results
Decreased Numbers of MZB and Reduced MZB Area in the Spleen of FcμR−/− Mice
We previously found the reduced MZB compartment in FcμR−/− mice when compared with WT mice (22, 25). To confirm this result and further clarify the reduction in the absolute numbers of MZB, we analyzed eight pairs of WT and FcμR−/− mice at 8–12 weeks of age. FACS analysis of CD23 and CD21 expression confirmed the significant reduction in the proportion of the CD21highCD23− MZB (Figure 1A). The absolute numbers of MZB in the spleen of each mouse were also greatly reduced in FcμR−/− mice (Figure 1B, left panel) while the total numbers of spleen cells were not different between WT and FcμR−/− mice (Figure 1B, right panel). To further verify the reduction of MZB, we stained spleen sections with MOMA-1, which is expressed by the metallophilic macrophages adjacent to the MZ of the spleen, and B220. As shown in Figure 1C, the spleen of FcμR−/− mice contained a significantly reduced MZB area compared with that of WT mice. These results demonstrate that the absence of FcμR results in reduction of MZB cell numbers, consistent with the previous findings (22, 25, 37).
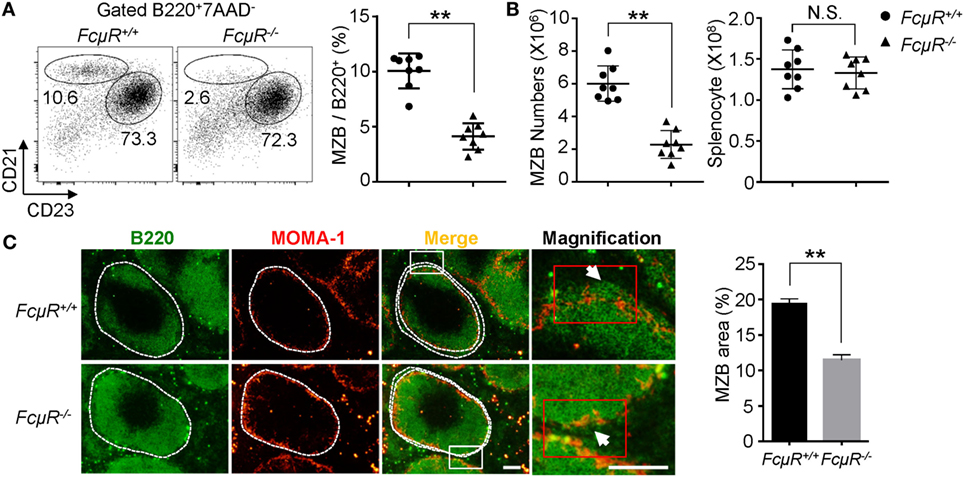
Figure 1. Decreased numbers of marginal zone B cells (MZB) and reduced MZB area in the spleen of FcμR−/− mice. (A) Reduced proportion of the B220+CD21highCD23low MZB in FcμR−/− mice. Left, representative FACS profiles; right, summary of eight pairs of WT and FcμR−/− mice. (B) Absolute numbers of MZB (left) and total spleen cells (right). The results of eight pairs of mice are shown. (C) Spleen sections of WT and FcμR−/− mice were stained for B220 (green) and mouse metallophilic macrophage (MOMA-1) (red). Scale bars, 100 µm. The proportion of MZB area was calculated by dividing the B220+ area outside the MOMA-1+ ring by the total B220+ area (n = 32; four MZB areas per section, four sections per mouse, two mice per group) using the Image J software. Representative staining results (left) and the mean MZB area (%) ± SD are shown. *p < 0.05; **p < 0.01.
MZB Development in FcμR−/− Mice
To explore the mechanism for the reduced MZB population in FcμR−/− mice, we first analyzed FcμR expression at different stages of MZB development by FACS. The gating strategy is shown in Figure S1 in Supplementary Material. FcμR expression was found to be low at T1, increased at T2, and further upregulated at MZ precursor (MZP), and then slightly reduced at MZB stage (Figure 2A). To explore the role of FcμR in MZB development, we next analyzed the proportion of T1, T2, MZP, and MZB in mice of 3, 6, and 9 weeks of age during which period MZB are formed. In WT mice, T1 decreased and T2 increased at 6 weeks of age compared with 3 weeks of age, and then both population remained unchanged during 6–9 weeks of age (Figure 2B). Compared with WT mice, FcμR−/− mice had a higher proportion of T1 at 3 weeks of age, which continued decreasing at 6 and 9 weeks of age accompanied by a gradual increase of T2 at 6 and 9 weeks of age (Figure 2B). These observations suggest that the decrease of T1 and the increase of T2 were both delayed in FcμR−/− mice when compared with WT mice. In WT mice, the proportion of MZP was transiently decreased at 6 weeks of age and then increased at 9 weeks of age (Figure 2C, left panel), accompanied by a similar increase of MZB at 9 weeks of age (Figure 2C, middle panel). While the proportion of MZP was similarly decreased at 6 weeks of age in FcμR−/− mice, it only slightly increased at 9 weeks of age (Figure 2C, left panel) and was not accompanied by an increase of MZB at 9 weeks of age (Figure 2C, middle panel). Therefore, the proportion of MZP and MZB both failed to recover at 9 weeks of age in FcμR−/− mice. Collectively, absence of FcμR resulted in a partial reduction of T2 and a severe reduction of MZP and MZB at 9 weeks of age. In contrast, the proportion of FOB was quite similar between WT and FcμR−/− mice at 3, 6, and 9 weeks of age (Figure 2C, right panel), indicating that FcμR specifically affected MZB cell development.
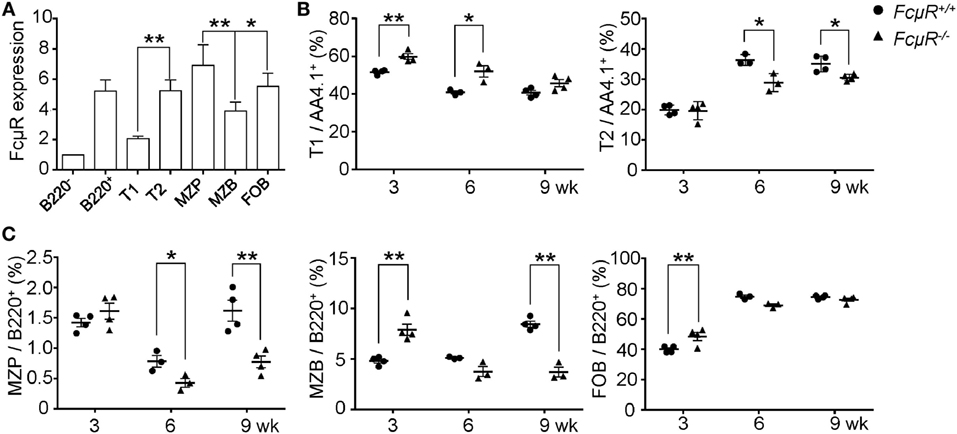
Figure 2. Marginal zone B cells (MZB) development in 3-, 6-, and 9-week-old WT and FcμR−/− mice. (A) Fc receptor (FcμR) expression at different stages of MZB development. MFI ± SD of the results from four 8- to 10-week-old mice are shown. MFI of the B220− cells was set to 1. (B) The proportion of T1 and T2 in 3-, 6-, and 9-week-old WT and FcμR−/− mice (n = 3 or 4). (C) The proportion of marginal zone precursor (MZP), MZB, and follicular B (FOB) in 3-, 6-, and 9-week-old WT and FcμR−/− mice (n = 3 or 4). The gating strategy is shown in Figure S1 in Supplementary Material. *p < 0.05; **p < 0.01.
MZB in FcμR−/− Mice Exhibit Increased Cell Death and Turnover In Vivo
We next sought to investigate the mechanism for the observed reduction in MZP and MZB in FcμR−/− mice. The decrease in MZB could be due to their increased death, decreased expansion, developmental arrest, or accelerated differentiation into plasma cells (37). To explore these possibilities, we first analyzed death of freshly isolated MZB and FOB. As shown in Figure S2 in Supplementary Material and Figure 3A (left panel), MZB in 6- and 9-week-old FcμR−/− mice exhibited significantly increased percentages of the 7AAD+ dead cells compared with MZB in WT mice. Consistently, the mutant MZB contained a greater proportion of cells positive for active caspase-3 (Figure 3B, left panel). In contrast, FOB in WT and FcμR−/− mice contained a similar percentage of the dead cells (Figure 3A, right panel) and cells positive for active caspase-3 (Figure 3B, right panel). These observations indicate that FcμR−/− MZB had increased spontaneous apoptosis in vivo compared with WT MZB.
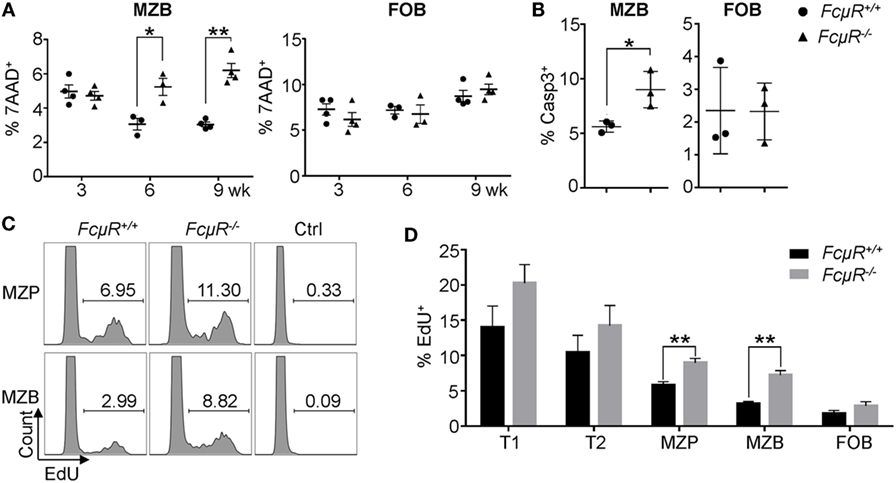
Figure 3. Increased death and turnover in marginal zone B cells (MZB) of FcμR−/− mice. (A) The proportion of 7AAD+ dead cells in gated MZB and follicular B (FOB) in the spleen. (B) Increased proportion of Casp3+ MZB in FcμR−/− mice. (C) Increased EdU+ marginal zone precursor (MZP) and MZB population in FcμR−/− mice. (D) Proportion of EdU+ cells at each stage of MZB development. Mean ± SD of the results from three to four mice are shown. For panels (B–D), 10- to 12 week-old mice were analyzed. *p < 0.05; **p < 0.01.
We then analyzed the proliferation in vivo of cells at different stages of MZB development by EdU incorporation assay. Mice were injected with EdU and 1 day later the proportion of EdU+ cells was analyzed. Both MZP and MZB in FcμR−/− mice contained a higher proportion of the EdU+ cells compared with those in WT mice (Figure 3C). In addition, T1 and T2 cells in FcμR−/− mice also contained a slightly increased proportion of the EdU+ cells compared with those in WT mice but the difference did not reach statistical significance (Figure 3D). In contrast, FOB in WT and FcμR−/− mice contained a similar proportion of the EdU+ cells. These results implicated a higher rate of turnover for cells at each stage of MZB development in FcμR−/− mice, possibly to compensate for the increased death.
Elevated IgD and MHC Class II Expression in MZB Cells of FcμR−/− Mice
To gain insight into the functional differences between MZB in WT and FcμR−/− mice, we compared the expression of various cell surface molecules that are known to play important roles in B cell function. We found that MZB in FcμR−/− mice expressed higher levels of IgD and MHC class II than did MZB in WT mice (Figure 4A). The levels of IgM, TLR4, CD80, CD86, CD40, FAS, and CD19 were not different between WT and FcμR−/− MZB (Figure 4A). The increased expression of IgD and MHC class II was observed in FcμR−/− mice at both 3 and 9 weeks of age (Figures 4B,C).
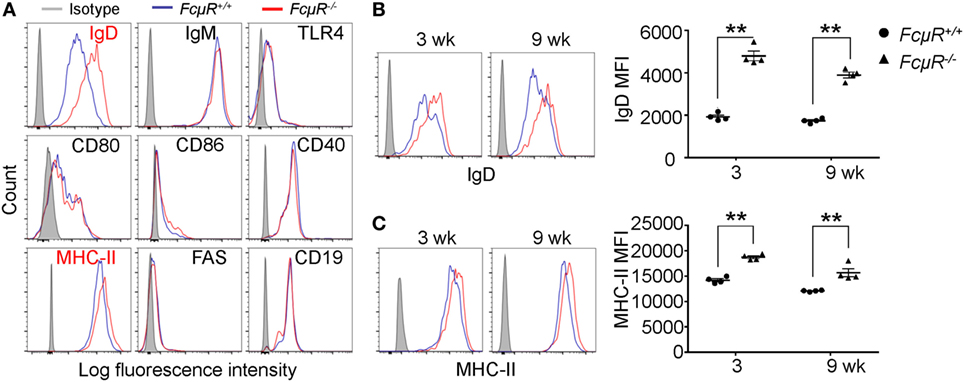
Figure 4. Elevated IgD and MHC class II expression in FcμR−/− marginal zone B cells (MZB) cells. (A) Expression of IgD, IgM, TLR4, CD80, CD86, CD40, MHC-II, FAS, and CD19 in WT (blue) and FcμR−/− (red) MZB. (B) IgD and (C) MHC-II expression in MZB from 3- and 9-week-old WT and FcμR−/− mice. Left panels, representative FACS profiles; right panels, the results of four WT and FcμR−/− mice. *p < 0.05; **p < 0.01.
Reduced Tonic BCR Signaling in FcμR−/− MZB
The strength of BCR and Notch2 signaling is important for MZB development. Tonic BCR signaling triggers phosphorylation of SYK and its downstream targets, including ERK and AKT kinases. Intracellular staining revealed significant reduction in the levels of pSYK and pAKT in FcμR−/− MZB when compared with WT MZB (Figure 5A; Figure S3 in Supplementary Material). These results suggest that MZB in FcμR−/− mice have reduced tonic BCR signal, consistent with their decreased survival (Figure 3A). In contrast, pSYK and pAKT levels were not significantly different between WT and FcμR−/− FOB (Figure 5B). In addition, we found normal Notch2 expression in FcμR−/− T2 and MZP and a moderately increased Notch2 level in FcμR−/− MZB relative to WT MZB (Figure 5C). Notch2 expression has been shown to be critical for the cell fate determination of MZB (11, 12). These observations suggest that the reduction of MZP and MZB in FcμR−/− mice is unlikely due to an impaired MZB fate decision but rather due to reduced tonic BCR signaling and increased cell death.
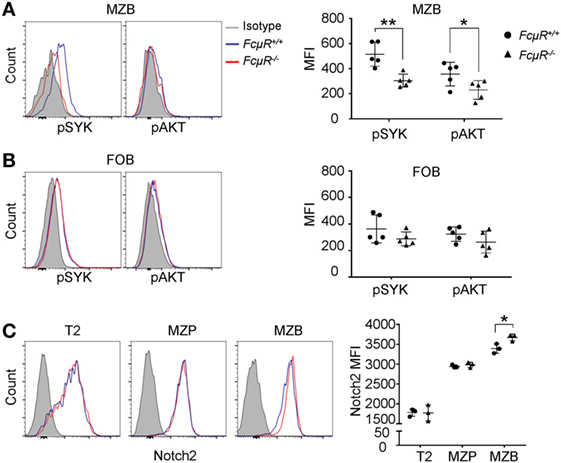
Figure 5. Reduced tonic BCR signaling and increased Notch2 expression in FcμR−/− marginal zone B cells (MZB). Reduced levels of phosphorylated SYK (pSYK) and AKT in MZB (A) but not follicular B (FOB) (B) of naïve FcμR−/− mice. Left panels, representative FACS profiles; right panels, quantitation of MFI of pSYK (pY348) and AKT (pS473) in MZB from five pairs of WT and FcμR−/− mice. (C) Elevated Notch2 expression in FcμR−/− MZB. Left panel, representative FACS profiles; right panel, results of three pairs of WT and FcμR−/− mice. *p < 0.05; **p < 0.01.
Decreased Antibody Production against Type 1 T-I Ag in FcμR−/− Mice
Marginal zone B cells and B1 cells are thought to be the major sources that produce antibodies in response to T-I and self Ags. To explore the function of MZB in FcμR−/− mice, we analyzed antibody production against type 1 T-I Ag NP-LPS. Consistent with the reduced MZB population, the production of NP-specific IgG3 antibody was significantly reduced in FcμR−/− mice (Figure 6A, right panel). The production of NP-specific IgM seemed unaffected (Figure 6A, left panel). However, our previous study has revealed that the basal serum IgM levels are elevated in FcμR−/− mice possibly due to the lack of FcμR-mediated IgM binding (22). Therefore, the seemingly normal production of the NP-specific IgM in FcμR−/− mice could be due to the lack of FcμR-mediated absorption of these antibodies. To analyze MZB and FOB activation in vivo in WT and FcμR−/− mice after the immunization, we compared their cell sizes as described by others (41). After i.v. injection of LPS, WT MZB increased their cell sizes significantly whereas FcμR−/− MZB did not (Figure 6B, upper left vs. right panels). By contrast, FOB in both groups of mice increased their cell sizes significantly (Figure 6B low panels). The results of three pairs of WT and FcμR−/− mice are summarized in Figure 6C. These results demonstrate that MZB in FcμR−/− mice responded poorly to LPS than did MZB in WT mice and suggest that FcμR is required for MZB to produce antibodies against NP-LPS.
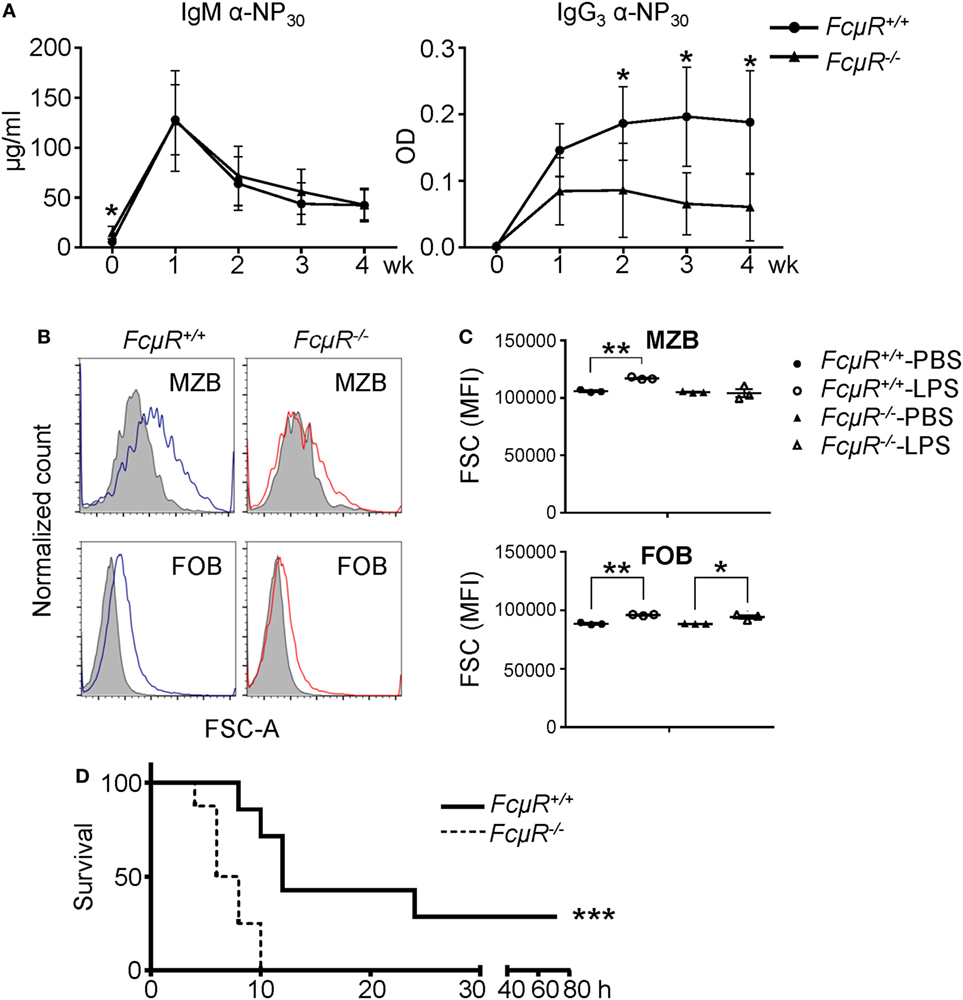
Figure 6. FcμR−/− mice are impaired in response to LPS and highly susceptible to Citrobacter rodentium infection. (A) Antibody production to a TI antigen, NP-LPS. Eight pairs of WT and FcμR−/− mice were immunized i.v. with 10 µg of NP-LPS and analyzed for the production of NP-specific IgM (left) and IgG3 (right) antibodies in the serum at 1–4 weeks after immunization. The mean values of WT and FcμR−/− mice at the indicated time points are shown. (B,C) Marginal zone B cells (MZB) in FcμR−/− mice responded poorly to LPS administration. WT and FcμR−/− mice were injected i.v. with 20 µg LPS or PBS as a control, and the cell size (FSC) of MZB and follicular B (FOB) was analyzed 24 h later. (B) FSC of MZB and FOB in WT (blue lines) and FcμR−/− (red lines) mice injected with LPS. Shaded areas, FSC of MZB and FOB in mice injected with PBS. (C) Summary of three mice per group. *p < 0.05; **p < 0.01 (unpaired t-test). (D) FcμR−/− mice are highly susceptible to acute bacterial infection. Eight pairs of WT and FcμR−/− mice were injected i.v. with C. rodentium (6 × 108 CFU) or PBS as a control and monitored for their survival. Kaplan–Meier survival plot for WT (solid line) and FcμR−/− (dotted line) mice are shown. ***p < 0.005 (log-rank test).
FcμR Protects Mice against Bacteria-Induced Sepsis
Having found that FcμR is required for MZB response to LPS, we next sought to investigate the role of FcμR in protecting mice against intravenous bacterial infection. We infected mice with C. rodentium, a Gram-negative bacterium that has LPS on its outer membrane. As shown in Figure 6D, FcμR−/− mice exhibited significantly accelerated and increased death after the infection compared with WT mice. Along with a previous study demonstrating a critical role for B cells in enhancing early innate immune responses and protecting mice from bacterial sepsis (42), our results suggest that FcμR contributes to B cell-mediated protection against acute bacterial infection.
Discussion
In this study, we have elucidated a critical role for FcμR in tonic BCR signaling in MZB and in their survival and LPS response in vivo. In addition, FcμR is required for antibody production against the T-I Ag NP-LPS and in protection against bacterial sepsis. Along with previous findings (22), these results demonstrate that FcμR is required for the survival and activation of both FOB and MZB and regulates both adaptive and innate immune responses.
While we found reduced MZB population in FcμR−/− mice [(22, 25), and current study], Nguyen et al. found reduced proportion of MZB but their absolute numbers were not decreased due to an increase in the total splenic B cells (27). The reason for this discrepancy is unclear but could be due to the difference in the age of mice. We have analyzed mice under 12-week-old, whereas the age of their mice used in the analysis of MZB was not indicated. In addition, we analyzed mice with a global FcμR deficiency whereas they analyzed mice with CD19-Cre-mediated B cell-specific FcμR deletion. Although FcμR is predominantly expressed by B cells (22–27), one cannot exclude the possibility that FcμR is also expressed at low levels by other cell types, which might have affected MZB. Furthermore, CD19 is a part of BCR coreceptor and it has been shown that humans carrying one mutated CD19 have defects in B cell development and activation (43, 44). CD19-Cre-mediated FcμR deletion also resulted in CD19 heterozygosity, which might have some effects on B cell development or function.
While FcμR−/− mice have reduced MZB population, mice lacking soluble IgM (Sμ−/−), the specific ligand for FcμR, showed a threefold increase in MZB numbers that is normalized by passive administration of polyclonal IgM (45). Similarly, Sμ−/− mice, but not FcμR−/− mice, exhibited enhanced B-1 cell development in the peritoneal cavity (46, 47). We have previously shown that FcμR physically associates with surface BCR in normal spleen B cells (33). FcμR therefore binds to both soluble pentameric IgM and membrane BCR. One intriguing possibility is that soluble IgM and BCR compete for the binding to FcμR. In Sμ−/− mice where soluble IgM is absent, FcμR may preferentially associate with BCR, leading to distinct phenotypes in MZB and B-1 development.
We show in this study that FcμR is required for the survival of MZB in vivo. Tonic BCR signal is essential for B cell survival. While WT and FcμR−/− MZB expressed the same levels of surface IgM BCR, we found reduced levels of pSYK and AKT in FcμR−/− MZB compared with WT MZB. SYK phosphorylation and activation occur during the early phase of BCR signaling. Therefore, the reduced SYK phosphorylation in FcμR−/− MZB suggests that FcμR is involved in the proximal BCR signaling, possibly upstream of SYK, in MZB. pSYK further activates downstream targets including AKT. Phosphorylated AKT promotes B cell survival by inhibiting FOXO1-mediated expression of pro-apoptotic genes (48). The reduced levels of pSYK and AKT suggest that FcμR promotes MZB survival in vivo by enhancing tonic BCR signaling. While we found that pSYK and pAKT levels were not significantly different between WT and FcμR−/− FOB, Nguyen et al. found increased pAKT levels in FOB of mice with B cell-specific FcμR deletion when compared with control littermates (27). As is the case for the discrepancy in MZB, differences in the age of mice, global vs. B cell-specific FcμR deficiency, or CD19 haploinsufficiency could have affected the results. Nevertheless, both their study and our previous study (22) revealed increased IgM levels in spleen B cells. In addition, the increases in pAKT levels and cell viability after anti-IgM stimulation were clearly compromised in FcμR−/− B cells compared with WT B cells (27), which is consistent with our study (22, 33) showing reduced B cell survival in FcμR−/− B cells after BCR crosslinking.
While FcμR is required for the survival of MZB in vivo in the absence of exogenous Ag stimulation, we have previously found that FcμR is required for the survival of FOB only after BCR crosslinking. Consistently, FcμR−/− mice have reduced MZB but normal FOB population. It has been suggested that MZB frequently recognize self Ag, for example Sm/RNP, which can be released from dead cells. Therefore, even in the absence of exogenous BCR stimulation, MZB may be constantly stimulated by self Ag. As MZB express much higher levels of IgM, but not IgD, BCR than FOB, self Ag stimulation may trigger a sufficiently strong signal in an FcμR-dependent manner. It is therefore conceivable that the “tonic” BCR signaling and the consequent SYK and AKT phosphorylation observed in MZB are actually a result of self Ag stimulation. In this regard, self Ag-stimulated MZB may resemble exogenous Ag-stimulated FOB. Therefore, FcμR may function similarly in FOB and MZB, i.e., promoting B cell survival after BCR stimulation, although the nature of the Ag may be different between FOB and MZB.
Fc receptor deficiency did not result in reduced expression of RBP-J/Notch2, which plays a critical role in the MZB fate determination. Notch2 expression was even slightly elevated in FcμR−/− MZB. This result suggests that FcμR is unlikely involved in MZB fate decision or commitment to the MZB lineage. In addition, we found a moderate but statistically significant increase in the levels of IgD and MHC-II. It has been shown that IgD BCR can only be activated by polyvalent Ag (49). Therefore, the elevated IgD levels in FcμR−/− MZB might affect their responses to Ag stimulation. MHC class II is normally upregulated in B cells upon activation. The moderate increase of MHC class II in FcμR−/− MZB may suggest that MZB in FcμR−/− mice might be at a slightly activated status. Alternatively, the elevated IgD, MHC-II, and Notch2 expression could be a result of the increased turnover of MZB observed in FcμR−/− mice, possibly to counteract against the reduction of MZB. A similar phenomenon has been observed in mice lacking c-myb where FOB were reduced in the periphery but these FOB exhibited increased turnover and expressed elevated MHC-II (50).
Recently, FcμR−/− mice were found to contain increased titters of the anti-Sm/RNP autoantibodies (37). Since antibodies against Sm/RNP are known to be produced by MZB (51), it was speculated that the reduction of MZB cells in FcμR−/− mice was caused by the rapid differentiation of MZB into plasma cells. To verify the accelerated plasma cell differentiation of MZB in FcμR−/− mice, we have attempted to immunize mice i.v. with Sm/RNP. However, we were unable to find an increased production of α-Sm/RNP antibodies in FcμR−/− mice (Figure S4 in Supplementary Material). We think that FcμR−/− mice already contain elevated titers of α-Sm/RNP antibodies, which may neutralize the immunized antigen quickly and prevent further production of the antibodies against these Ag. Based on these observations, we propose that FcμR has dual functions: it promotes antibody production by MZB against foreign T-I Ag yet restricts the production of autoantibodies such as α-Sm/RNP.
An unexpected finding is that FcμR is required for MZB to respond to LPS. LPS stimulates TLR4 and triggers survival and activation signals in B cells through MyD88-dependent and -independent pathways. Intriguingly, very recently, it has been reported that TLR4 signaling in B cells requires BCR and SYK (52). Their results suggest that TLR4 signals through two distinct pathways, one via BCR and the other via MYD88. Based on these findings, the poor response of FcμR−/− MZB to LPS may be attributable to the reduced BCR signaling in these cells. We also found reduced antibody production against the type 1 T-I Ag NP-LPS. MZB and B-1 cells are thought to be the major cell types that respond to the T-I Ag. FcμR−/− mice have reduced MZB but normal B-1 population, suggesting that the impaired antibody production against NP-LPS in FcμR−/− mice is more likely attributable to both the decreased MZB population and their impaired response to LPS. MZB are known to play a central role in capturing and responding to the blood-borne Ag (41). It has also been shown that B cells protect mice against bacterial sepsis by enhancing innate inflammatory cytokine and chemokine production (42). In agreement with this study, we found that FcμR−/− mice exhibited accelerated and increased death after intravenous bacterial infection compared with WT mice. We postulate that FcμR−/− mice were unable to efficiently capture the blood-borne C. rodentium due to reduced MZB population and produce inflammatory cytokines due to the impaired LPS response, resulting in increased death. However, it is also possible that defects in other B cell subpopulation or even in non-B cells might be involved in the accelerated death of FcμR−/− mice after i.v. inoculation of C. rodentium. In summary, this study revealed an important role for FcμR in the survival and activation of MZB and in protection against bacterial sepsis.
Ethics Statement
All animal experiments were approved by the Animal Committee of the School of Basic Medical Sciences, Fudan University.
Author Contributions
JL designed the study, performed the experiments, and contributed to the writing of the manuscript. HZ and JQ analyzed and interpreted data. EX and LZ helped animal experiments. Y-QW, YC, HK, and TT interpreted data and supervised the study. J-YW designed the study, interpreted data, and wrote the manuscript. All authors read and approved the final manuscript.
Conflict of Interest Statement
The authors declare that the research was conducted in the absence of any commercial or financial relationships that could be construed as a potential conflict of interest.
Acknowledgments
We thank Dapeng Yan for providing the Citrobacter rodentium bacterium and members in Wang lab for helpful discussions and suggestions.
Funding
This work was supported by the National Basic Research Program of China (2015CB943300 to J-YW), the National Natural Science Foundation of China (81373129 and 81571529 to J-YW), Development Project of Shanghai Peak Disciplines-Integrative Medicine (20150407) and a grant-in-aid for scientific research (C) from Japan Society for the Promotion of Science (17K08878 to J-YW).
Supplementary Material
The Supplementary Material for this article can be found online at http://www.frontiersin.org/articles/10.3389/fimmu.2018.00160/full#supplementary-material.
References
1. Allman D, Pillai S. Peripheral B cell subsets. Curr Opin Immunol (2008) 20(2):149–57. doi:10.1016/j.coi.2008.03.014
2. Zouali M, Richard Y. Marginal zone B-cells, a gatekeeper of innate immunity. Front Immunol (2011) 2:63. doi:10.3389/fimmu.2011.00063
3. Lopes-Carvalho T, Kearney JF. Development and selection of marginal zone B cells. Immunol Rev (2004) 197:192–205. doi:10.1111/j.0105-2896.2004.0112.x
4. Janeway CA Jr, Medzhitov R. Innate immune recognition. Annu Rev Immunol (2002) 20:197–216. doi:10.1146/annurev.immunol.20.083001.084359
5. Bendelac A, Bonneville M, Kearney JF. Autoreactivity by design: innate B and T lymphocytes. Nat Rev Immunol (2001) 1(3):177–86. doi:10.1038/35105052
6. Martin F, Kearney JF. Marginal-zone B cells. Nat Rev Immunol (2002) 2(5):323–35. doi:10.1038/nri799
7. MacLennan IC, Bazin H, Chassoux D, Gray D, Lortan J. Comparative analysis of the development of B cells in marginal zones and follicles. Adv Exp Med Biol (1985) 186:139–44.
8. Pillai S, Cariappa A, Moran ST. Marginal zone B cells. Annu Rev Immunol (2005) 23:161–96. doi:10.1146/annurev.immunol.23.021704.115728
9. Newman R, Ahlfors H, Saveliev A, Galloway A, Hodson DJ, Williams R, et al. Maintenance of the marginal-zone B cell compartment specifically requires the RNA-binding protein ZFP36L1. Nat Immunol (2017) 18(6):683–93. doi:10.1038/ni.3724
10. Srivastava B, Quinn WJ III, Hazard K, Erikson J, Allman D. Characterization of marginal zone B cell precursors. J Exp Med (2005) 202(9):1225–34. doi:10.1084/jem.20051038
11. Witt CM, Won WJ, Hurez V, Klug CA. Notch2 haploinsufficiency results in diminished B1 B cells and a severe reduction in marginal zone B cells. J Immunol (2003) 171(6):2783–8. doi:10.4049/jimmunol.171.6.2783
12. Tan JB, Xu K, Cretegny K, Visan I, Yuan JS, Egan SE, et al. Lunatic and manic fringe cooperatively enhance marginal zone B cell precursor competition for delta-like 1 in splenic endothelial niches. Immunity (2009) 30(2):254–63. doi:10.1016/j.immuni.2008.12.016
13. Pillai S, Cariappa A. The follicular versus marginal zone B lymphocyte cell fate decision. Nat Rev Immunol (2009) 9(11):767–77. doi:10.1038/nri2656
14. Martin F, Kearney JF. Positive selection from newly formed to marginal zone B cells depends on the rate of clonal production, CD19, and btk. Immunity (2000) 12(1):39–49. doi:10.1016/S1074-7613(00)80157-0
15. Wen L, Brill-Dashoff J, Shinton SA, Asano M, Hardy RR, Hayakawa K. Evidence of marginal-zone B cell-positive selection in spleen. Immunity (2005) 23(3):297–308. doi:10.1016/j.immuni.2005.08.007
16. Carey JB, Moffatt-Blue CS, Watson LC, Gavin AL, Feeney AJ. Repertoire-based selection into the marginal zone compartment during B cell development. J Exp Med (2008) 205(9):2043–52. doi:10.1084/jem.20080559
17. Stadanlick JE, Kaileh M, Karnell FG, Scholz JL, Miller JP, Quinn WJ III, et al. Tonic B cell antigen receptor signals supply an NF-kappaB substrate for prosurvival BLyS signaling. Nat Immunol (2008) 9(12):1379–87. doi:10.1038/ni.1666
18. Kleiman E, Salyakina D, De Heusch M, Hoek KL, Llanes JM, Castro I, et al. Distinct transcriptomic features are associated with transitional and mature B-cell populations in the mouse spleen. Front Immunol (2015) 6:30. doi:10.3389/fimmu.2015.00030
19. King JK, Ung NM, Paing MH, Contreras JR, Alberti MO, Fernando TR, et al. Regulation of marginal zone B-cell differentiation by microRNA-146a. Front Immunol (2016) 7:670. doi:10.3389/fimmu.2016.00670
20. Cerutti A, Cols M, Puga I. Marginal zone B cells: virtues of innate-like antibody-producing lymphocytes. Nat Rev Immunol (2013) 13(2):118–32. doi:10.1038/nri3383
21. Song H, Cerny J. Functional heterogeneity of marginal zone B cells revealed by their ability to generate both early antibody-forming cells and germinal centers with hypermutation and memory in response to a T-dependent antigen. J Exp Med (2003) 198(12):1923–35. doi:10.1084/jem.20031498
22. Ouchida R, Mori H, Hase K, Takatsu H, Kurosaki T, Tokuhisa T, et al. Critical role of the IgM Fc receptor in IgM homeostasis, B-cell survival, and humoral immune responses. Proc Natl Acad Sci U S A (2012) 109(40):E2699–706. doi:10.1073/pnas.1210706109
23. Kubagawa H, Oka S, Kubagawa Y, Torii I, Takayama E, Kang DW, et al. Identity of the elusive IgM Fc receptor (FcµR) in humans. J Exp Med (2009) 206(12):2779–93. doi:10.1084/jem.20091107
24. Shima H, Takatsu H, Fukuda S, Ohmae M, Hase K, Kubagawa H, et al. Identification of TOSO/FAIM3 as an Fc receptor for IgM. Int Immunol (2010) 22(3):149–56. doi:10.1093/intimm/dxp121
25. Honjo K, Kubagawa Y, Jones DM, Dizon B, Zhu Z, Ohno H, et al. Altered Ig levels and antibody responses in mice deficient for the Fc receptor for IgM (FcµR). Proc Natl Acad Sci U S A (2012) 109(39):15882–7. doi:10.1073/pnas.1206567109
26. Choi SC, Wang H, Tian L, Murakami Y, Shin DM, Borrego F, et al. Mouse IgM Fc receptor, FCMR, promotes B cell development and modulates antigen-driven immune responses. J Immunol (2013) 190(3):987–96. doi:10.4049/jimmunol.1202227
27. Nguyen TT, Klasener K, Zurn C, Castillo PA, Brust-Mascher I, Imai DM, et al. The IgM receptor FcµR limits tonic BCR signaling by regulating expression of the IgM BCR. Nat Immunol (2017) 18(3):321–33. doi:10.1038/ni.3677
28. Lang KS, Lang PA, Meryk A, Pandyra AA, Boucher LM, Pozdeev VI, et al. Involvement of toso in activation of monocytes, macrophages, and granulocytes. Proc Natl Acad Sci U S A (2013) 110(7):2593–8. doi:10.1073/pnas.1222264110
29. Brenner D, Brustle A, Lin GH, Lang PA, Duncan GS, Knobbe-Thomsen CB, et al. Toso controls encephalitogenic immune responses by dendritic cells and regulatory T cells. Proc Natl Acad Sci U S A (2014) 111(3):1060–5. doi:10.1073/pnas.1323166111
30. Lang PA, Meryk A, Pandyra AA, Brenner D, Brustle A, Xu HC, et al. Toso regulates differentiation and activation of inflammatory dendritic cells during persistence-prone virus infection. Cell Death Differ (2015) 22(1):164–73. doi:10.1038/cdd.2014.138
31. Murakami Y, Narayanan S, Su S, Childs R, Krzewski K, Borrego F, et al. Toso, a functional IgM receptor, is regulated by IL-2 in T and NK cells. J Immunol (2012) 189(2):587–97. doi:10.4049/jimmunol.1200840
32. Nguyen TT, Graf BA, Randall TD, Baumgarth N. sIgM-FcµR interactions regulate early B cell activation and plasma cell development after influenza virus infection. J Immunol (2017) 199(5):1635–46. doi:10.4049/jimmunol.1700560
33. Ouchida R, Lu Q, Liu J, Li Y, Chu Y, Tsubata T, et al. FcµR interacts and cooperates with the B cell receptor to promote B cell survival. J Immunol (2015) 194(7):3096–101. doi:10.4049/jimmunol.1402352
34. Li FJ, Kubagawa Y, McCollum MK, Wilson L, Motohashi T, Bertoli LF, et al. Enhanced levels of both the membrane-bound and soluble forms of IgM Fc receptor (FcµR) in patients with chronic lymphocytic leukemia. Blood (2011) 118(18):4902–9. doi:10.1182/blood-2011-04-350793
35. Vire B, David A, Wiestner A. TOSO, the Fcmicro receptor, is highly expressed on chronic lymphocytic leukemia B cells, internalizes upon IgM binding, shuttles to the lysosome, and is downregulated in response to TLR activation. J Immunol (2011) 187(8):4040–50. doi:10.4049/jimmunol.1100532
36. Hancer VS, Diz-Kucukkaya R, Aktan M. Overexpression of Fc mu receptor (FCMR, TOSO) gene in chronic lymphocytic leukemia patients. Med Oncol (2012) 29(2):1068–72. doi:10.1007/s12032-011-9821-3
37. Honjo K, Kubagawa Y, Suzuki Y, Takagi M, Ohno H, Bucy RP, et al. Enhanced auto-antibody production and Mott cell formation in FcµR-deficient autoimmune mice. Int Immunol (2014) 26(12):659–72. doi:10.1093/intimm/dxu070
38. Ouchida R, Kurosaki T, Wang JY. A role for lysosomal-associated protein transmembrane 5 in the negative regulation of surface B cell receptor levels and B cell activation. J Immunol (2010) 185(1):294–301. doi:10.4049/jimmunol.1000371
39. Takahashi Y, Ohta H, Takemori T. Fas is required for clonal selection in germinal centers and the subsequent establishment of the memory B cell repertoire. Immunity (2001) 14(2):181–92. doi:10.1016/S1074-7613(01)00100-5
40. Van Schaik SM, Abbas AK. Role of T cells in a murine model of Escherichia coli sepsis. Eur J Immunol (2007) 37(11):3101–10. doi:10.1002/eji.200737295
41. Martin F, Oliver AM, Kearney JF. Marginal zone and B1 B cells unite in the early response against T-independent blood-borne particulate antigens. Immunity (2001) 14(5):617–29. doi:10.1016/S1074-7613(01)00129-7
42. Kelly-Scumpia KM, Scumpia PO, Weinstein JS, Delano MJ, Cuenca AG, Nacionales DC, et al. B cells enhance early innate immune responses during bacterial sepsis. J Exp Med (2011) 208(8):1673–82. doi:10.1084/jem.20101715
43. Artac H, Reisli I, Kara R, Pico-Knijnengurg I, Adin-Cinar S, Pekcan S, et al. B-cell maturation and antibody responses in individuals carrying a mutated CD19 allele. Genes Immun (2010) 11(7):523–30. doi:10.1038/gene.2010.22
44. Morbach H, Schickel JN, Cunningham-Rundles C, Conley ME, Reisli I, Franco JL, et al. CD19 controls toll-like receptor 9 responses in human B cells. J Allergy Clin Immunol (2016) 137(3):889–98. doi:10.1016/j.jaci.2015.08.040
45. Baker N, Ehrenstein MR. Cutting edge: selection of B lymphocyte subsets is regulated by natural IgM. J Immunol (2002) 169(12):6686–90. doi:10.4049/jimmunol.169.12.6686
46. Ehrenstein MR, O’Keefe TL, Davies SL, Neuberger MS. Targeted gene disruption reveals a role for natural secretory IgM in the maturation of the primary immune response. Proc Natl Acad Sci U S A (1998) 95(17):10089–93. doi:10.1073/pnas.95.17.10089
47. Boes M, Esau C, Fischer MB, Schmidt T, Carroll M, Chen J. Enhanced B-1 cell development, but impaired IgG antibody responses in mice deficient in secreted IgM. J Immunol (1998) 160(10):4776–87.
48. Srinivasan L, Sasaki Y, Calado DP, Zhang B, Paik JH, DePinho RA, et al. PI3 kinase signals BCR-dependent mature B cell survival. Cell (2009) 139(3):573–86. doi:10.1016/j.cell.2009.08.041
49. Ubelhart R, Hug E, Bach MP, Wossning T, Duhren-von Minden M, Horn AH, et al. Responsiveness of B cells is regulated by the hinge region of IgD. Nat Immunol (2015) 16(5):534–43. doi:10.1038/ni.3141
50. Thomas MD, Kremer CS, Ravichandran KS, Rajewsky K, Bender TP. c-Myb is critical for B cell development and maintenance of follicular B cells. Immunity (2005) 23(3):275–86. doi:10.1016/j.immuni.2005.08.005
51. Kishi Y, Higuchi T, Phoon S, Sakamaki Y, Kamiya K, Riemekasten G, et al. Apoptotic marginal zone deletion of anti-Sm/ribonucleoprotein B cells. Proc Natl Acad Sci U S A (2012) 109(20):7811–6. doi:10.1073/pnas.1204509109
Keywords: marginal zone B cell, Fcµ receptor, tonic BCR signal, apoptosis, humoral immune response
Citation: Liu J, Zhu H, Qian J, Xiong E, Zhang L, Wang Y-Q, Chu Y, Kubagawa H, Tsubata T and Wang J-Y (2018) Fcµ Receptor Promotes the Survival and Activation of Marginal Zone B Cells and Protects Mice against Bacterial Sepsis. Front. Immunol. 9:160. doi: 10.3389/fimmu.2018.00160
Received: 17 October 2017; Accepted: 18 January 2018;
Published: 05 February 2018
Edited by:
Harry W. Schroeder, University of Alabama at Birmingham, United StatesReviewed by:
Paolo Casali, The University of Texas Health Science Center San Antonio, United StatesClaude-Agnes Reynaud, Institut National de la Santé et de la Recherche Médicale, France
Copyright: © 2018 Liu, Zhu, Qian, Xiong, Zhang, Wang, Chu, Kubagawa, Tsubata and Wang. This is an open-access article distributed under the terms of the Creative Commons Attribution License (CC BY). The use, distribution or reproduction in other forums is permitted, provided the original author(s) and the copyright owner are credited and that the original publication in this journal is cited, in accordance with accepted academic practice. No use, distribution or reproduction is permitted which does not comply with these terms.
*Correspondence: Ji-Yang Wang, wang@fudan.edu.cn