- 1Frontier Research Institute for Interdisciplinary Sciences, Tohoku University, Sendai, Japan
- 2PRESTO, Japan Science and Technology Agency, Kawaguchi, Japan
Inhalation of exogenous crystals such as silica, asbestos, and carbon nanotubes can cause lung fibrosis and cancer. Endogenous crystals such as monosodium urate, cholesterol, and hydroxyapatite are associated with pathogenesis of gout, atherosclerosis, and osteoarthritis, respectively. These crystal-associated-inflammatory diseases are triggered by the macrophage NLRP3 inflammasome activation and cell death. Therefore, it is important to understand how macrophages recognize crystals. However, it is unlikely that macrophages have evolutionally acquired receptors specific for crystals or recently emerged nanoparticles. Several recent studies have reported that some crystal particles are negatively charged and are recognized by scavenger receptor family members in a charge-dependent manner. Alternatively, a model for receptor-independent phagocytosis of crystals has also been proposed. This review focuses on the mechanisms by which macrophages recognize crystals and nanoparticles.
Introduction
Phagocytosis of crystals such as silica, asbestos, monosodium urate (MSU), and hydroxyapatite by macrophages was initially observed by electron microscopy about 40 years ago (1–4). These early studies showed that upon phagocytosis, crystals are not digested but instead cause lysosomal damage. Although the underlying mechanism was unclear, this process was referred to as “frustrated phagocytosis” and was implicated in the pathogenesis of inflammatory diseases such as fibrosis and cancer (5).
Recent studies have revealed that silica and asbestos induce IL-1β secretion via NLRP3 inflammasome activation in macrophages (6–8). Likewise, various crystals such as MSU, hydroxyapatite, cholesterol, and alum crystals, and nanomaterials such as TiO2 nanoparticles and carbon nanotubes (CNTs) have also been reported to induce NLRP3 inflammasome activation in macrophages (7, 9–12). The molecular mechanism for inflammasome activation has been extensively studied and is well summarized in several recent reviews (6, 13–15). Briefly, at least two signals are required for the activation of NLRP3 inflammasome. The first signal (signal 1) is mediated via pathogen-associated molecular patterns, damage-associated molecular patterns (DAMPs), or cytokines that trigger nuclear factor-κB (NF-κB)-mediated upregulation of NLRP3 along with pro-IL-1β (Figure 1). The second signal (signal 2) stimulates the assembly of a complex of multiple proteins including NLRP3, ASC, and pro-caspase-1, resulting in the activation of caspase-1. Subsequently, active caspase-1 processes pro-IL-1β to mature IL-1β, which is then released into the extracellular environment through damaged membranes of dying macrophages (Figure 1).
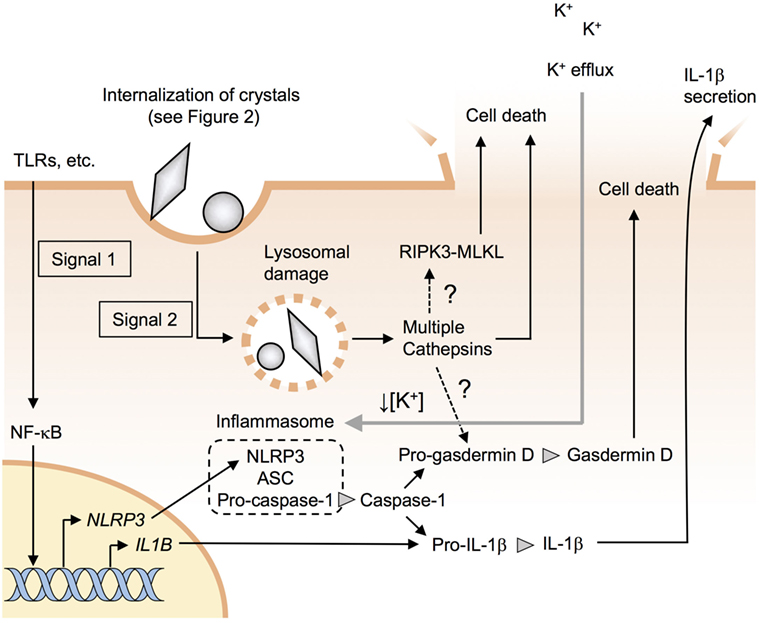
Figure 1. Particle-induced NLRP3 inflammasome activation and cell death. Signal 1 induces pro-IL-1β along with NLRP3 through the nuclear factor-kappa B (NF-κB) pathway. Signal 2 causes lysosomal damages and stimulates the assembly of a complex of multiple proteins including NLRP3, ASC, and procaspase-1, resulting in the formation of inflammasomes. Active caspase-1 processes pro-IL-1β and pro-gasdermin D to mature IL-1β and gasdermin D. Lysosomal damage results in the release of the lysosomal enzyme cathepsins, which may induce NLRP3 inflammasome-independent pyroptotic cell death. Receptor-interacting serine/threonine kinase-mixed-lineage kinase domain-like protein (RIPK3-MLKL) pathway is involved in crystal-induced necroptosis in epithelial cells but not in macrophages.
Upon recognition of crystals, macrophage surface receptors transmit signal 1 and/or 2. It is also proposed that the receptor-independent recognition of crystals transmits signal 2. This review summarizes and discusses the recent findings regarding the recognition of crystals and nanoparticles by macrophages.
Macrophage Surface Receptors
Macrophages express a wide variety of cell-surface receptors in order to recognize and internalize pathogenic particles such as bacteria and apoptotic cells (16–20). For instance, class A scavenger receptors such as SR-A1 (also known as MSR) and MARCO (proposed to be renamed as SR-A6) and class B scavenger receptors such as SR-B1 and CD36 (proposed to be renamed as SR-B2) bind to various polyanionic particles such as bacteria and apoptotic cells (18, 21). Fc receptors such as FcγRIII and complement receptors (CRs) such as CR3 internalize IgG- and complement-opsonized particles, respectively (22, 23), while C-type lectins such as Dectin-1 (also called Clec7a), Mincle (also called Clec4e), and MICL (also called Clec12a) recognize fungi-, mycobacteria-, or dying host cell-associated molecules (17, 24).
Given that it is unlikely that macrophages have acquired the specific ability to recognize crystals and recently emerged nanoparticles through evolution, the abovementioned receptors and opsonins may be responsible for the recognition of such inorganic particles. A common feature of organic particles such as bacteria and apoptotic cells and inorganic particles such as MSU, silica, and titanium is that they have negatively charged surfaces (25, 26), which could be favored by class A and class B scavenger receptors. By contrast, while organic particles harbor various ligands (protein, lipid, etc.) on their surface, the surface of a crystal is remarkably uniform. Therefore, an alternative model for receptor-independent phagocytosis of crystals has also been proposed (27). The mechanisms underlying the recognition of each particle by macrophages are discussed below.
Recognition of Exogenous Crystals and Nanomaterials by Macrophages
Silica (SiO2) and Titanium (TiO2) Particles
Silica, which comprises about 60% of the Earth’s crust, is a major component of sand and rocks and thus is contained in dust and air pollutants (28, 29). Therefore, it can be assumed that most organisms are exposed to crystalline silica (30, 31), and prolonged inhalation of large amounts of crystalline silica dust is known to cause lung fibrosis and cancer (8).
Compared with crystalline silica, amorphous silica is biocompatible and is contained in various foods and medicines (32). However, recent studies have shown that nanoparticles (diameter <100 nm), but not micro-sized particles, can trigger inflammation (32–34). Silica and titanium nanoparticles are the most frequently used nanomaterials (35), and titanium nanoparticles have also been reported to trigger inflammation (36, 37). Under physiological conditions, nanoparticles tend to aggregate irreversibly, resulting in particles of submicron or micrometer size in order to reduce their high surface energy (26, 38, 39). Nevertheless, their toxicity largely depends on their primary size and not on the secondary aggregate size (34, 40) with smaller particles being more toxic. However, the molecular mechanism underlying size-dependent toxicity remains largely unknown.
Class A scavenger receptors such as SR-A1 and MARCO are known to bind to silica and titanium particles (Figure 2) (41); however, given that SR-A1- and MARCO-deficient mice and macrophages still show inflammatory responses to these particles (42–44), it seems likely that additional receptor(s) may be involved. Using unbiased functional screening, our laboratory recently identified the class B scavenger receptor member 1 (SR-B1) as a novel silica receptor (Figure 2) (26). In contrast to SR-A1 and MARCO, which bind to both silica and titanium particles (41), SR-B1 binds to silica but not to titanium particles (26). Moreover, SR-B1-deficient macrophages showed impaired internalization of silica and subsequent inflammasome activation (26). However, since SR-B1 binds to both crystalline and amorphous silica irrespective of particle size (26), the recognition by SR-B1 does not account for the size-dependent toxicity of silica particles. On the other hand, Nishijima et al. have recently shown that anti-SR-A1 mAb inhibits THP-1 cell-inflammatory responses to 50-nm silica particles, but not to other sizes of silica particles, suggesting that the recognition by SR-A1 may account for the size-dependent toxicity of silica (45).
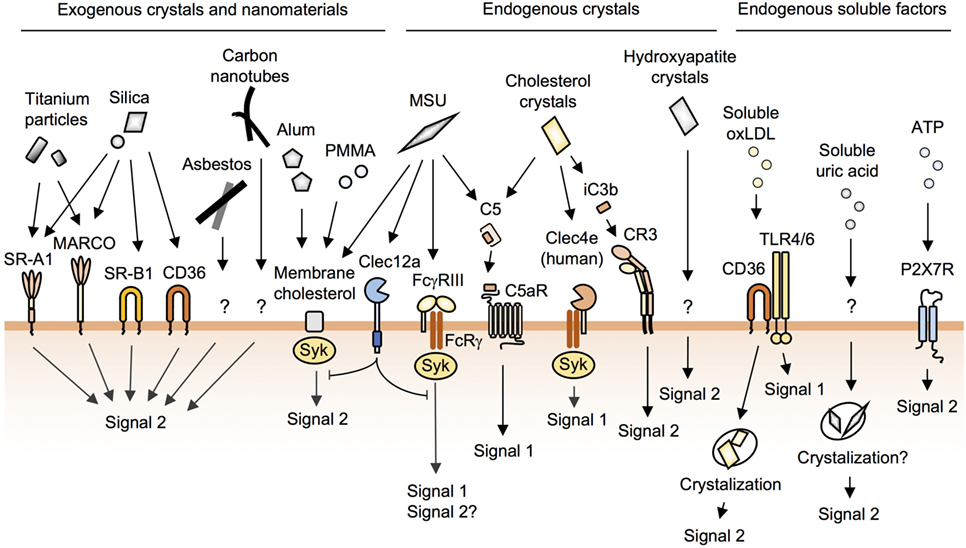
Figure 2. The recognition of crystals and nanoparticles on the macrophage surface. Macrophages recognize and internalize crystals and nanoparticles through cell-surface receptors and membrane cholesterol. Silica particles are recognized by SR-A1, MARCO, SR-B1, and CD36. Alum, poly(methyl methacrylate) (PMMA), and monosodium urate (MSU) crystals bind directly to membrane cholesterol to be internalized. MSU and cholesterol crystals activate complement pathways. Soluble oxidized low-density lipoprotein (oxLDL) is internalized by CD36 and then crystallized in phagosomes. P2X7R does not cause lysosomal damage. In addition to these, many unknown pathways of phagocytosis remain to be identified.
The class B scavenger receptor CD36 also binds to silica particles (Figure 2) (26); however, CD36 is not involved in silica-induced acute lung inflammation in mice (26, 46). This is probably due to the marginal expression of CD36 on resident alveolar macrophages (26, 47). Nevertheless, since CD36 is expressed on inflammatory macrophages infiltrating alveolar spaces (47), CD36 may contribute to the chronic lung inflammation.
Because scavenger receptors have only a short cytoplasmic tail (18), they probably work as tethering receptors rather than as signaling receptors following particle recognition. Therefore, co-receptors may also be required in order to internalize particles. Indeed, the ectopic expression of SR-A1 or SR-B1 on non-phagocytic cells enables these cells to bind, but not internalize, to silica particles (26). It has been reported that a chemical inhibitor of Mer receptor tyrosine kinase (MerTK) inhibits IL-1β secretion from THP-1 cells, which use SR-A1 to recognize silica particles. This suggests that MerTK works as a co-receptor of the scavenger receptor (45). Therefore, it would be intriguing to address whether the co-expression of MerTK and the scavenger receptor impart cells with the ability to internalize silica particles.
Asbestos and CNTs
It is well known that the prolonged inhalation of large amounts of asbestos causes mesothelioma and lung cancer (48). Like silica, asbestos is also efficiently internalized by macrophages, resulting in NLRP3 inflammasome activation and cell death (49). Since asbestos is a silicate mineral, asbestos may also bind to scavenger receptors. Murthy et al. reported that MARCO-deficient mice show less fibrosis following exposure to chrysotile asbestos (50). Although the authors did not show the direct binding of MARCO to asbestos, these data suggest that MARCO may contribute to asbestos-induced lung fibrosis.
Carbon nanotubes are a highly representative product of nanotechnology (51), and although the worldwide production of CNTs is less than that of silica and titanium nanoparticles (35, 51), the production of CNTs has been increasing drastically year after year as they are applied to a wide variety of commercial products including rechargeable batteries and automotive parts (51). Electron microscopy reveals that some CNTs have a needle-like structure reminiscent of asbestos (52, 53). Indeed, recent animal studies have shown that these CNTs have asbestos-like pathogenic behavior (9, 54). For instance, a seminal study by Poland et al. showed that the intraperitoneal injection with multi-walled CNTs (MWCNTs) as well as asbestos causes massive granulomatous inflammation in the diaphragms of wild-type mice (55). Furthermore, Takagi et al. showed that the intraperitoneal injection of MWCNT caused mesothelioma in p53+/– mice (56), while Palomaki et al. found that CNTs and asbestos induce NLPR3 inflammasome activation in human macrophages (57). However, it remains unknown how macrophages recognize CNTs on their cell surface.
Alum Crystals
Alums (aluminum adjuvants) are widely used for vaccination in both humans and animals (58). It has been reported that upon being internalized, alum activates NLRP3 inflammasomes, which are essential for alum-induced acute inflammation (59, 60). However, recent studies have proposed that the adjuvant effect of alum is mediated via NLRP3-independent phagocyte cell death (Figure 1) (61, 62). This occurs when dying cells release their intracellular contents, some of which trigger innate immune responses. Specifically, alum-induced Th2 responses have been reported to be mediated via host DNA and uric acid (61, 62). On the other hand, it has been reported that NLRP3 is expressed in the nuclei of Th2 cells and works as a transcriptional regulator of Th2 differentiation (63). Thus, the requirement of NLRP3 for Th2 responses remains controversial.
Shi and colleagues have reported that the phagocytosis of alum as well as MSU crystals (discussed below) is not mediated by cell-surface receptors (64, 65). By using atomic force microscopy, they showed that alum directly binds to membrane lipids, and this lipid ligation activates Syk and PI3K (Figure 2) (64, 65).
In addition to the direct recognition of crystals and nanoparticles by macrophage receptors and membrane cholesterol, these particles may also be opsonized and recognized indirectly by macrophages. Indeed, nanoparticles are absorbed by various plasma proteins called the protein corona (66–68). For instance, it has been reported that albumin and complement bind to silica nanoparticles (69), although it remains unknown whether the protein corona contributes to phagocytosis. Some in vitro studies have shown that the protein corona does not enhance but rather suppresses the phagocytosis of nanoparticles by macrophages (70, 71). As discussed below, endogenous crystals such as MSU and cholesterol activate complement pathways.
Recognition of Endogenous Crystals by Macrophages
MSU Crystals
Dying cells release uric acid, and these crystals trigger inflammation (72). In addition, the saturation of uric acid in body fluids results in the formation of MSU crystals, which trigger macrophage NLRP3 inflammasome activation and are associated with the pathogenesis of gout (10, 73). Early studies reported that MSU crystals activate complement pathways (74, 75), and this has been confirmed by a recent study which showed that MSU and cholesterol crystals (discussed below), but not silica or alum, activate complement pathways (76). Although MSU-activated C5a binds to C5aR, a G protein-coupled receptor (Figure 2), to activate signal 1 (Figure 1) in human monocytes (76), C5aR was not found to contribute to the phagocytosis of MSU (76).
FcγRIII (CD16) has been reported to bind to MSU directly, resulting in the activation of the Syk pathway in human neutrophils (Figure 2) (77). FcγRIII associates with the FcRγ chain, and this receptor complex is a well-characterized phagocytic receptor for IgG-opsonized particles (22); however, the internalization of MSU by FcγRIII has not been demonstrated. Although we and others have observed that MSU crystals have negatively charged surfaces (26, 27), we failed to observe the binding of MSU crystals to scavenger receptors, which can bind to polyanionic particles (26). These results suggest that scavenger receptors may recognize not only surface charges but also shapes and/or substances of particles. Phagocytic receptors for MSU crystals remain to be identified.
Shi and colleagues proposed that MSU crystals bind directly to plasma membrane cholesterols, a driving force for their internalization (Figure 2) (65). This group also proposed that the receptor-independent model can be applied for alum (64) and biomaterial microspheres of poly(methyl methacrylate) (Figure 2) (78). While this is an attractive model for understanding the recognition of crystals and nanoparticles with uniform surfaces by phagocytes, it remains unknown why these particles preferentially bind to phagocytes when cholesterol is present in the membrane of all cell types.
Clec12a (also called MICL, DCAL2, and CLL-1), a C-type lectin receptor, has been recently reported to recognize MSU, but not other particles such as polystyrene, silica, or zymosan (Figure 2) (79). Clec12a has an ITIM in its cytoplasmic domain, and the activation of this receptor has been shown to inhibit Syk signaling. Moreover, Clec12a-deficient mice showed enhanced inflammation in response to MSU (79), although it remains unknown whether Clec12a suppresses phagocytosis of MSU.
A recent study has shown that soluble uric acid also triggers NLRP3 inflammasome activation, although the authors do not exclude the possibility that this activation could be caused by undetectable microcrystals of uric acid (80). It is also possible that internalized soluble uric acid is crystallized in phagocytes just like soluble oxidized LDL as described below (81) (Figure 2). Either way, this finding may propose that uric acid released from dying cells or hyperuricemia directly causes inflammation without crystal deposition.
Cholesterol Crystals
Cholesterol accumulation leads to the formation of crystals, which have been shown to be engulfed by macrophages in atherosclerotic sinus lesions (12), leading to pro-inflammatory responses through NLRP3 inflammasome activation (10, 82). In addition, early studies have shown that cholesterol crystals as well as MSU crystals activate complement pathways (83, 84). Recently, Samstad et al. showed that cholesterol crystals activate the C5a and the C5aR pathways leading to the upregulation of CR3 (CD11b and CD18 complexes) (Figure 2) (85). Mechanistically, C5aR, a G-protein-coupled receptor, activates ERK and NF-κB pathways (signal 1) (86). These pathways lead to the induction of the expression of CR3, a phagocytic receptor for iC3b-tagged particles (19), which contributes to the phagocytosis of cholesterol crystals (signal 2) (85). Indeed, this group showed that the inhibition of C5 or C3 reduces the phagocytosis of cholesterol crystals by human monocytes (85, 87).
The human, but not mouse, C-type lectin Mincle (also called Clec4e) has been shown to have a cholesterol recognition amino acid consensus (CRAC) motif in its extracellular domain (88). Through this CRAC motif, human Mincle binds to cholesterol crystals, resulting in the activation of pro-inflammatory signals via the associated FcRγ chain (Figure 2) (88). Although the FcRγ chain is able to mediate a phagocytic signal (22), it remains unknown whether human Mincle is involved in the phagocytosis of cholesterol crystals.
In addition to cholesterol crystals, soluble oxidized low-density lipoprotein (oxLDL) is internalized by macrophages, and the crystals are then nucleated, resulting in the lysosomal disruption and activation of the NLRP3 inflammasome (81, 82). CD36 is a receptor for oxLDL and is essential for both signal 1 (NF-κB activation in conjunction with TLR4 and -6) and signal 2 (internalization of oxLDL), resulting in NLRP3 inflammasome activation (Figures 1 and 2) (81, 89).
Hydroxyapatite Crystals
Hydroxyapatite, a basic calcium phosphate crystal, is a major component of bones and teeth. The ectopic deposition of these crystals is predominantly observed in osteoarthritis (OA) joints and is implicated in the pathogenesis of OA (10, 90). In addition, synthetic hydroxyapatite crystals are the widely used biomaterials, although it has been shown that these crystals can trigger local inflammation upon being released from implanted prosthetics (91). Recent studies have reported that hydroxyapatite crystals are internalized by macrophages through unknown mechanisms where they trigger NLRP3 inflammasome activation (Figure 2) (92, 93). NLRP3 is essential for crystal-induced IL-1β secretion in vitro; however, the requirement for NLRP3 is only partial in mouse models of arthritis (92, 94) as shown in NLRP3-deficient mice where various crystals such as silica and alum still induce macrophage cell death and inflammation (discussed below). Thus, hydroxyapatite crystal-induced arthritis may be mediated via NLRP3-independent macrophage death.
Particle-Induced Cell Death and Diseases
Crystals cause lysosomal damages, resulting in the release of the lysosomal enzyme cathepsins to cytosol (95), which is the upstream of NLRP3 inflammasome activation (Figure 1). Rock and colleagues have recently shown that multiple cathepsins including cathepsins b, l, x, and s contribute to NLRP3- and caspase-1-independent cell death (Figure 1) (96). However, the downstream mechanisms of action of the cathepsins remain unknown. It would be intriguing to address whether cathepsins directly cause membrane damage or activate pore-forming proteins such as gasdermin D (97–99). It has also been reported that receptor-interacting serine/threonine kinase-3 and mixed-lineage kinase domain-like protein-mediated necroptosis pathways (100) are involved in crystal-induced cell death in epithelial cells (101) but not in macrophages (Figure 1) (96, 102).
Dying cells release DAMPs such as uric acid and ATP (72). As mentioned earlier, uric acid induces inflammation (80). ATP, which is released from pannexin-1, binds to P2X7 receptor to induce cell death and NLRP3 inflammasome activation without causing lysosomal damage (Figure 2) (103). Besides DAMPs, dying macrophages release internalized crystals, which could induce cell death of the neighboring macrophages. This sequential cell death may be more crucial than NLRP3 inflammasome activation in the pathogenesis of crystal-induced chronic inflammation and fibrosis such as arthritis (92, 94), silicosis (104), and asbestosis (105) as these diseases develop in NLRP3-deficient mice.
Conclusion
Crystals such as silica, asbestos, and MSU cause inflammatory diseases through macrophage activation and cell death. As discussed here, macrophages have been found to recognize crystals via cell-surface receptors and/or membrane cholesterol, although these pathways account for only a fraction of crystal phagocytosis. Therefore, many unknown pathways of phagocytosis remain to be identified. While phagocytosis and subsequent lysosomal damage appear to be essential for the pathogenesis of particle-induced-inflammatory diseases, it remains unknown how the physicochemical properties (element, size, etc.) of particles impact lysosomal damage. A better understanding of the molecular mechanisms underlying particle-induced inflammation will provide opportunities not only for the development of therapeutic approaches for incurable silicosis and asbestosis but also for the development of safer nanomaterials in the future.
Author Contributions
The author confirms being the sole contributor of this work and approved it for publication.
Conflict of Interest Statement
The author declares that the research was conducted in the absence of any commercial or financial relationships that could be construed as a potential conflict of interest.
Funding
This work was supported by Grants in Aid for Scientific Research (B) #16H02960 from the Japan Society for the Promotion of Science (JSPS) and by PRESTO #JPMJPR17H9 from the Japan Science and Technology Agency (JST).
References
1. Maurer KH, Schumacher HR. Hydroxyapatite phagocytosis by human polymorphonuclear leucocytes. Ann Rheum Dis (1979) 38:84–8. doi:10.1136/ard.38.1.84
2. Policard A, Collet A, Giltaireralyte L. [Electron microscopy study of the phagocytosis of silica particles]. Rev Hematol (1955) 10:674–88.
3. Schumacher HR, Phelps P. Sequential changes in human polymorphonuclear leukocytes after urate crystal phagocytosis. An electron microscopic study. Arthritis Rheum (1971) 14:513–26. doi:10.1002/art.1780140411
5. Archer VE. Carcinogenicity of fibers and films: a theory. Med Hypotheses (1979) 5:1257–62. doi:10.1016/0306-9877(79)90008-2
6. Broz P, Dixit VM. Inflammasomes: mechanism of assembly, regulation and signalling. Nat Rev Immunol (2016) 16:407–20. doi:10.1038/nri.2016.58
7. Franklin BS, Mangan MS, Latz E. Crystal formation in inflammation. Annu Rev Immunol (2016) 34:173–202. doi:10.1146/annurev-immunol-041015-055539
9. Donaldson K, Murphy FA, Duffin R, Poland CA. Asbestos, carbon nanotubes and the pleural mesothelium: a review of the hypothesis regarding the role of long fibre retention in the parietal pleura, inflammation and mesothelioma. Part Fibre Toxicol (2010) 7:5. doi:10.1186/1743-8977-7-5
10. Mulay SR, Anders HJ. Crystallopathies. N Engl J Med (2016) 374:2465–76. doi:10.1056/NEJMra1601611
11. Rock KL, Kataoka H, Lai JJ. Uric acid as a danger signal in gout and its comorbidities. Nat Rev Rheumatol (2013) 9:13–23. doi:10.1038/nrrheum.2012.143
12. Tall AR, Yvan-Charvet L. Cholesterol, inflammation and innate immunity. Nat Rev Immunol (2015) 15:104–16. doi:10.1038/nri3793
13. Gross O, Thomas CJ, Guarda G, Tschopp J. The inflammasome: an integrated view. Immunol Rev (2011) 243:136–51. doi:10.1111/j.1600-065X.2011.01046.x
14. Latz E, Xiao TS, Stutz A. Activation and regulation of the inflammasomes. Nat Rev Immunol (2013) 13:397–411. doi:10.1038/nri3452
15. Rathinam VA, Fitzgerald KA. Inflammasome complexes: emerging mechanisms and effector functions. Cell (2016) 165:792–800. doi:10.1016/j.cell.2016.03.046
16. Bournazos S, Ravetch JV. Fcgamma receptor function and the design of vaccination strategies. Immunity (2017) 47:224–33. doi:10.1016/j.immuni.2017.07.009
17. Ishikawa E, Mori D, Yamasaki S. Recognition of mycobacterial lipids by immune receptors. Trends Immunol (2017) 38:66–76. doi:10.1016/j.it.2016.10.009
18. PrabhuDas MR, Baldwin CL, Bollyky PL, Bowdish DME, Drickamer K, Febbraio M, et al. A consensus definitive classification of scavenger receptors and their roles in health and disease. J Immunol (2017) 198:3775–89. doi:10.4049/jimmunol.1700373
19. Taylor PR, Martinez-Pomares L, Stacey M, Lin HH, Brown GD, Gordon S. Macrophage receptors and immune recognition. Annu Rev Immunol (2005) 23:901–44. doi:10.1146/annurev.immunol.23.021704.115816
20. Underhill DM, Goodridge HS. Information processing during phagocytosis. Nat Rev Immunol (2012) 12:492–502. doi:10.1038/nri3244
21. Canton J, Neculai D, Grinstein S. Scavenger receptors in homeostasis and immunity. Nat Rev Immunol (2013) 13:621–34. doi:10.1038/nri3515
22. Bournazos S, DiLillo DJ, Ravetch JV. The role of Fc-FcgammaR interactions in IgG-mediated microbial neutralization. J Exp Med (2015) 212:1361–9. doi:10.1084/jem.20151267
23. Ricklin D, Reis ES, Lambris JD. Complement in disease: a defence system turning offensive. Nat Rev Nephrol (2016) 12:383–401. doi:10.1038/nrneph.2016.70
24. Dambuza IM, Brown GD. C-type lectins in immunity: recent developments. Curr Opin Immunol (2015) 32:21–7. doi:10.1016/j.coi.2014.12.002
25. Cho WS, Duffin R, Thielbeer F, Bradley M, Megson IL, Macnee W, et al. Zeta potential and solubility to toxic ions as mechanisms of lung inflammation caused by metal/metal oxide nanoparticles. Toxicol Sci (2012) 126:469–77. doi:10.1093/toxsci/kfs006
26. Tsugita M, Morimoto N, Tashiro M, Kinoshita K, Nakayama M. SR-B1 is a silica receptor that mediates canonical inflammasome activation. Cell Rep (2017) 18:1298–311. doi:10.1016/j.celrep.2017.01.004
27. Shi Y, Mucsi AD, Ng G. Monosodium urate crystals in inflammation and immunity. Immunol Rev (2010) 233:203–17. doi:10.1111/j.0105-2896.2009.00851.x
28. Moreno T, Reche C, Rivas I, Cruz Minguillon M, Martins V, Vargas C, et al. Urban air quality comparison for bus, tram, subway and pedestrian commutes in Barcelona. Environ Res (2015) 142:495–510. doi:10.1016/j.envres.2015.07.022
29. Pozzi R, De Berardis B, Paoletti L, Guastadisegni C. Inflammatory mediators induced by coarse (PM2.5-10) and fine (PM2.5) urban air particles in raw 264.7 cells. Toxicology (2003) 183:243–54. doi:10.1016/S0300-483X(02)00545-0
30. Brauer M, Avila-Casado C, Fortoul TI, Vedal S, Stevens B, Churg A. Air pollution and retained particles in the lung. Environ Health Perspect (2001) 109:1039–43. doi:10.1289/ehp.011091039
31. WHO. Elimination of Silicosis GOHNET News Letter. (2007). p. 1–20. Availablefrom: http://www.who.int/occupational_health/publications/newsletter/gohnet12e.pdf
32. Merget R, Bauer T, Kupper HU, Philippou S, Bauer HD, Breitstadt R, et al. Health hazards due to the inhalation of amorphous silica. Arch Toxicol (2002) 75:625–34. doi:10.1007/s002040100266
33. Huh D, Matthews BD, Mammoto A, Montoya-Zavala M, Hsin HY, Ingber DE. Reconstituting organ-level lung functions on a chip. Science (2010) 328:1662–8. doi:10.1126/science.1188302
34. Kusaka T, Nakayama M, Nakamura K, Ishimiya M, Furusawa E, Ogasawara K. Effect of silica particle size on macrophage inflammatory responses. PLoS One (2014) 9:e92634. doi:10.1371/journal.pone.0092634
35. Mitrano DM, Motellier S, Clavaguera S, Nowack B. Review of nanomaterial aging and transformations through the life cycle of nano-enhanced products. Environ Int (2015) 77:132–47. doi:10.1016/j.envint.2015.01.013
36. Shi H, Magaye R, Castranova V, Zhao J. Titanium dioxide nanoparticles: a review of current toxicological data. Part Fibre Toxicol (2013) 10:15. doi:10.1186/1743-8977-10-15
37. Yazdi AS, Guarda G, Riteau N, Drexler SK, Tardivel A, Couillin I, et al. Nanoparticles activate the NLR pyrin domain containing 3 (Nlrp3) inflammasome and cause pulmonary inflammation through release of IL-1alpha and IL-1beta. Proc Natl Acad Sci U S A (2010) 107:19449–54. doi:10.1073/pnas.1008155107
38. Lison D, Vietti G, van den Brule S. Paracelsus in nanotoxicology. Part Fibre Toxicol (2014) 11:35. doi:10.1186/s12989-014-0035-7
39. Tsugita M, Morimoto N, Nakayama M. SiO2 and TiO2 nanoparticles synergistically trigger macrophage inflammatory responses. Part Fibre Toxicol (2017) 14:11. doi:10.1186/s12989-017-0192-6
40. Rabolli V, Lison D, Huaux F. The complex cascade of cellular events governing inflammasome activation and IL-1beta processing in response to inhaled particles. Part Fibre Toxicol (2016) 13:40. doi:10.1186/s12989-016-0150-8
41. Thakur SA, Hamilton RF Jr, Holian A. Role of scavenger receptor a family in lung inflammation from exposure to environmental particles. J Immunotoxicol (2008) 5:151–7. doi:10.1080/15476910802085863
42. Arredouani M, Yang Z, Ning Y, Qin G, Soininen R, Tryggvason K, et al. The scavenger receptor MARCO is required for lung defense against pneumococcal pneumonia and inhaled particles. J Exp Med (2004) 200:267–72. doi:10.1084/jem.20040731
43. Beamer CA, Holian A. Scavenger receptor class A type I/II (CD204) null mice fail to develop fibrosis following silica exposure. Am J Physiol Lung Cell Mol Physiol (2005) 289:L186–95. doi:10.1152/ajplung.00474.2004
44. Thakur SA, Beamer CA, Migliaccio CT, Holian A. Critical role of MARCO in crystalline silica-induced pulmonary inflammation. Toxicol Sci (2009) 108:462–71. doi:10.1093/toxsci/kfp011
45. Nishijima N, Hirai T, Misato K, Aoyama M, Kuroda E, Ishii KJ, et al. Human scavenger receptor A1-mediated inflammatory response to silica particle exposure is size specific. Front Immunol (2017) 8:379. doi:10.3389/fimmu.2017.00379
46. Hamilton RF Jr, Thakur SA, Mayfair JK, Holian A. MARCO mediates silica uptake and toxicity in alveolar macrophages from C57BL/6 mice. J Biol Chem (2006) 281:34218–26. doi:10.1074/jbc.M605229200
47. Lagasse HA, Anidi IU, Craig JM, Limjunyawong N, Poupore AK, Mitzner W, et al. Recruited monocytes modulate malaria-induced lung injury through CD36-mediated clearance of sequestered infected erythrocytes. J Leukoc Biol (2016) 99:659–71. doi:10.1189/jlb.4HI0315-130RRR
48. Mossman BT, Gee JB. Asbestos-related diseases. N Engl J Med (1989) 320:1721–30. doi:10.1056/NEJM198906293202604
49. Dostert C, Petrilli V, Van Bruggen R, Steele C, Mossman BT, Tschopp J. Innate immune activation through Nalp3 inflammasome sensing of asbestos and silica. Science (2008) 320:674–7. doi:10.1126/science.1156995
50. Murthy S, Larson-Casey JL, Ryan AJ, He C, Kobzik L, Carter AB. Alternative activation of macrophages and pulmonary fibrosis are modulated by scavenger receptor, macrophage receptor with collagenous structure. FASEB J (2015) 29:3527–36. doi:10.1096/fj.15-271304
51. De Volder MF, Tawfick SH, Baughman RH, Hart AJ. Carbon nanotubes: present and future commercial applications. Science (2013) 339:535–9. doi:10.1126/science.1222453
52. Murr LE, Soto KF. TEM comparison of chrysotile (asbestos) nanotubes and carbon nanotubes. J Sci Mater (2004) 39:4941–7. doi:10.1023/B:Jmsc.0000035342.99587.96
53. Service RF. Nanotoxicology. Nanotechnology grows up. Science (2004) 304:1732–4. doi:10.1126/science.304.5678.1732
54. Vietti G, Lison D, van den Brule S. Mechanisms of lung fibrosis induced by carbon nanotubes: towards an adverse outcome pathway (AOP). Part Fibre Toxicol (2016) 13:11. doi:10.1186/s12989-016-0123-y
55. Poland CA, Duffin R, Kinloch I, Maynard A, Wallace WA, Seaton A, et al. Carbon nanotubes introduced into the abdominal cavity of mice show asbestos-like pathogenicity in a pilot study. Nat Nanotechnol (2008) 3:423–8. doi:10.1038/nnano.2008.111
56. Takagi A, Hirose A, Nishimura T, Fukumori N, Ogata A, Ohashi N, et al. Induction of mesothelioma in p53± mouse by intraperitoneal application of multi-wall carbon nanotube. J Toxicol Sci (2008) 33:105–16. doi:10.2131/jts.33.105
57. Palomaki J, Valimaki E, Sund J, Vippola M, Clausen PA, Jensen KA, et al. Long, needle-like carbon nanotubes and asbestos activate the NLRP3 inflammasome through a similar mechanism. ACS Nano (2011) 5:6861–70. doi:10.1021/nn200595c
58. Lindblad EB. Aluminium adjuvants—in retrospect and prospect. Vaccine (2004) 22:3658–68. doi:10.1016/j.vaccine.2004.03.032
59. Eisenbarth SC, Colegio OR, O’Connor W, Sutterwala FS, Flavell RA. Crucial role for the Nalp3 inflammasome in the immunostimulatory properties of aluminium adjuvants. Nature (2008) 453:1122–6. doi:10.1038/nature06939
60. Hornung V, Bauernfeind F, Halle A, Samstad EO, Kono H, Rock KL, et al. Silica crystals and aluminum salts activate the NALP3 inflammasome through phagosomal destabilization. Nat Immunol (2008) 9:847–56. doi:10.1038/ni.1631
61. Kool M, Willart MA, van Nimwegen M, Bergen I, Pouliot P, Virchow JC, et al. An unexpected role for uric acid as an inducer of T helper 2 cell immunity to inhaled antigens and inflammatory mediator of allergic asthma. Immunity (2011) 34:527–40. doi:10.1016/j.immuni.2011.03.015
62. Marichal T, Ohata K, Bedoret D, Mesnil C, Sabatel C, Kobiyama K, et al. DNA released from dying host cells mediates aluminum adjuvant activity. Nat Med (2011) 17:996–1002. doi:10.1038/nm.2403
63. Bruchard M, Rebe C, Derangere V, Togbe D, Ryffel B, Boidot R, et al. The receptor NLRP3 is a transcriptional regulator of TH2 differentiation. Nat Immunol (2015) 16:859–70. doi:10.1038/ni.3202
64. Flach TL, Ng G, Hari A, Desrosiers MD, Zhang P, Ward SM, et al. Alum interaction with dendritic cell membrane lipids is essential for its adjuvanticity. Nat Med (2011) 17:479–87. doi:10.1038/nm.2306
65. Ng G, Sharma K, Ward SM, Desrosiers MD, Stephens LA, Schoel WM, et al. Receptor-independent, direct membrane binding leads to cell-surface lipid sorting and Syk kinase activation in dendritic cells. Immunity (2008) 29:807–18. doi:10.1016/j.immuni.2008.09.013
66. Caracciolo G, Farokhzad OC, Mahmoudi M. Biological identity of nanoparticles in vivo: clinical implications of the protein corona. Trends Biotechnol (2017) 35:257–64. doi:10.1016/j.tibtech.2016.08.011
67. Hadjidemetriou M, Kostarelos K. Nanomedicine: evolution of the nanoparticle corona. Nat Nanotechnol (2017) 12:288–90. doi:10.1038/nnano.2017.61
68. Lundqvist M, Stigler J, Elia G, Lynch I, Cedervall T, Dawson KA. Nanoparticle size and surface properties determine the protein corona with possible implications for biological impacts. Proc Natl Acad Sci U S A (2008) 105:14265–70. doi:10.1073/pnas.0805135105
69. Tenzer S, Docter D, Kuharev J, Musyanovych A, Fetz V, Hecht R, et al. Rapid formation of plasma protein corona critically affects nanoparticle pathophysiology. Nat Nanotechnol (2013) 8:772–81. doi:10.1038/nnano.2013.181
70. Mirshafiee V, Kim R, Park S, Mahmoudi M, Kraft ML. Impact of protein pre-coating on the protein corona composition and nanoparticle cellular uptake. Biomaterials (2016) 75:295–304. doi:10.1016/j.biomaterials.2015.10.019
71. Yan Y, Gause KT, Kamphuis MM, Ang CS, O’Brien-Simpson NM, Lenzo JC, et al. Differential roles of the protein corona in the cellular uptake of nanoporous polymer particles by monocyte and macrophage cell lines. ACS Nano (2013) 7:10960–70. doi:10.1021/nn404481f
72. Kono H, Rock KL. How dying cells alert the immune system to danger. Nat Rev Immunol (2008) 8:279–89. doi:10.1038/nri2215
73. So AK, Martinon F. Inflammation in gout: mechanisms and therapeutic targets. Nat Rev Rheumatol (2017) 13:639–47. doi:10.1038/nrrheum.2017.155
74. Giclas PC, Ginsberg MH, Cooper NR. Immunoglobulin G independent activation of the classical complement pathway by monosodium urate crystals. J Clin Invest (1979) 63:759–64. doi:10.1172/JCI109360
75. Hasselbacher P. C3 activation by monosodium urate monohydrate and other crystalline material. Arthritis Rheum (1979) 22:571–8. doi:10.1002/art.1780220603
76. An LL, Mehta P, Xu L, Turman S, Reimer T, Naiman B, et al. Complement C5a potentiates uric acid crystal-induced IL-1beta production. Eur J Immunol (2014) 44:3669–79. doi:10.1002/eji.201444560
77. Barabe F, Gilbert C, Liao N, Bourgoin SG, Naccache PH. Crystal-induced neutrophil activation VI. Involvement of FcgammaRIIIB (CD16) and CD11b in response to inflammatory microcrystals. FASEB J (1998) 12:209–20.
78. Malik AF, Hoque R, Ouyang X, Ghani A, Hong E, Khan K, et al. Inflammasome components Asc and caspase-1 mediate biomaterial-induced inflammation and foreign body response. Proc Natl Acad Sci U S A (2011) 108:20095–100. doi:10.1073/pnas.1105152108
79. Neumann K, Castineiras-Vilarino M, Hockendorf U, Hannesschlager N, Lemeer S, Kupka D, et al. Clec12a is an inhibitory receptor for uric acid crystals that regulates inflammation in response to cell death. Immunity (2014) 40:389–99. doi:10.1016/j.immuni.2013.12.015
80. Braga TT, Forni MF, Correa-Costa M, Ramos RN, Barbuto JA, Branco P, et al. Soluble uric acid activates the NLRP3 inflammasome. Sci Rep (2017) 7:39884. doi:10.1038/srep39884
81. Sheedy FJ, Grebe A, Rayner KJ, Kalantari P, Ramkhelawon B, Carpenter SB, et al. CD36 coordinates NLRP3 inflammasome activation by facilitating intracellular nucleation of soluble ligands into particulate ligands in sterile inflammation. Nat Immunol (2013) 14:812–20. doi:10.1038/ni.2639
82. Duewell P, Kono H, Rayner KJ, Sirois CM, Vladimer G, Bauernfeind FG, et al. NLRP3 inflammasomes are required for atherogenesis and activated by cholesterol crystals. Nature (2010) 464:1357–61. doi:10.1038/nature08938
83. Hasselbacher P, Hahn JL. Activation of the alternative pathway of complement by microcrystalline cholesterol. Atherosclerosis (1980) 37:239–45. doi:10.1016/0021-9150(80)90009-X
84. Vogt W, von Zabern I, Damerau B, Hesse D, Luhmann B, Nolte R. Mechanisms of complement activation by crystalline cholesterol. Mol Immunol (1985) 22:101–6. doi:10.1016/S0161-5890(85)80003-1
85. Samstad EO, Niyonzima N, Nymo S, Aune MH, Ryan L, Bakke SS, et al. Cholesterol crystals induce complement-dependent inflammasome activation and cytokine release. J Immunol (2014) 192:2837–45. doi:10.4049/jimmunol.1302484
86. Kolev M, Le Friec G, Kemper C. Complement—tapping into new sites and effector systems. Nat Rev Immunol (2014) 14:811–20. doi:10.1038/nri3761
87. Pilely K, Rosbjerg A, Genster N, Gal P, Pal G, Halvorsen B, et al. Cholesterol crystals activate the lectin complement pathway via ficolin-2 and mannose-binding lectin: implications for the progression of atherosclerosis. J Immunol (2016) 196:5064–74. doi:10.4049/jimmunol.1502595
88. Kiyotake R, Oh-Hora M, Ishikawa E, Miyamoto T, Ishibashi T, Yamasaki S. Human Mincle binds to cholesterol crystals and triggers innate immune responses. J Biol Chem (2015) 290:25322–32. doi:10.1074/jbc.M115.645234
89. Stewart CR, Stuart LM, Wilkinson K, van Gils JM, Deng J, Halle A, et al. CD36 ligands promote sterile inflammation through assembly of a toll-like receptor 4 and 6 heterodimer. Nat Immunol (2010) 11:155–61. doi:10.1038/ni.1836
90. McCarthy GM, Cheung HS. Point: hydroxyapatite crystal deposition is intimately involved in the pathogenesis and progression of human osteoarthritis. Curr Rheumatol Rep (2009) 11:141–7. doi:10.1007/s11926-009-0020-6
91. Laquerriere P, Grandjean-Laquerriere A, Jallot E, Balossier G, Frayssinet P, Guenounou M. Importance of hydroxyapatite particles characteristics on cytokines production by human monocytes in vitro. Biomaterials (2003) 24:2739–47. doi:10.1016/S0142-9612(03)00089-9
92. Jin C, Frayssinet P, Pelker R, Cwirka D, Hu B, Vignery A, et al. NLRP3 inflammasome plays a critical role in the pathogenesis of hydroxyapatite-associated arthropathy. Proc Natl Acad Sci U S A (2011) 108:14867–72. doi:10.1073/pnas.1111101108
93. Pazar B, Ea HK, Narayan S, Kolly L, Bagnoud N, Chobaz V, et al. Basic calcium phosphate crystals induce monocyte/macrophage IL-1beta secretion through the NLRP3 inflammasome in vitro. J Immunol (2011) 186:2495–502. doi:10.4049/jimmunol.1001284
94. Joosten LA, Ea HK, Netea MG, Busso N. Interleukin-1beta activation during acute joint inflammation: a limited role for the NLRP3 inflammasome in vivo. Joint Bone Spine (2011) 78:107–10. doi:10.1016/j.jbspin.2010.11.004
95. Kroemer G, Jaattela M. Lysosomes and autophagy in cell death control. Nat Rev Cancer (2005) 5:886–97. doi:10.1038/nrc1738
96. Orlowski GM, Sharma S, Colbert JD, Bogyo M, Robertson SA, Kataoka H, et al. Frontline science: multiple cathepsins promote inflammasome-independent, particle-induced cell death during NLRP3-dependent IL-1beta activation. J Leukoc Biol (2017) 102:7–17. doi:10.1189/jlb.3HI0316-152R
97. Ding J, Wang K, Liu W, She Y, Sun Q, Shi J, et al. Pore-forming activity and structural autoinhibition of the gasdermin family. Nature (2016) 535:111–6. doi:10.1038/nature18590
98. Kayagaki N, Stowe IB, Lee BL, O’Rourke K, Anderson K, Warming S, et al. Caspase-11 cleaves gasdermin D for non-canonical inflammasome signalling. Nature (2015) 526:666–71. doi:10.1038/nature15541
99. Shi J, Zhao Y, Wang K, Shi X, Wang Y, Huang H, et al. Cleavage of GSDMD by inflammatory caspases determines pyroptotic cell death. Nature (2015) 526:660–5. doi:10.1038/nature15514
100. Weinlich R, Oberst A, Beere HM, Green DR. Necroptosis in development, inflammation and disease. Nat Rev Mol Cell Biol (2017) 18:127–36. doi:10.1038/nrm.2016.149
101. Mulay SR, Desai J, Kumar SV, Eberhard JN, Thomasova D, Romoli S, et al. Cytotoxicity of crystals involves RIPK3-MLKL-mediated necroptosis. Nat Commun (2016) 7:10274. doi:10.1038/ncomms10274
102. Jacobson LS, Lima H Jr, Goldberg MF, Gocheva V, Tsiperson V, Sutterwala FS, et al. Cathepsin-mediated necrosis controls the adaptive immune response by Th2 (T helper type 2)-associated adjuvants. J Biol Chem (2013) 288:7481–91. doi:10.1074/jbc.M112.400655
103. Di Virgilio F, Dal Ben D, Sarti AC, Giuliani AL, Falzoni S. The P2X7 receptor in infection and inflammation. Immunity (2017) 47:15–31. doi:10.1016/j.immuni.2017.06.020
104. Chow MT, Tschopp J, Moller A, Smyth MJ. NLRP3 promotes inflammation-induced skin cancer but is dispensable for asbestos-induced mesothelioma. Immunol Cell Biol (2012) 90:983–6. doi:10.1038/icb.2012.46
Keywords: macrophages, phagocytosis, crystals, nanoparticles, inflammation, scavenger receptors
Citation: Nakayama M (2018) Macrophage Recognition of Crystals and Nanoparticles. Front. Immunol. 9:103. doi: 10.3389/fimmu.2018.00103
Received: 22 November 2017; Accepted: 12 January 2018;
Published: 29 January 2018
Edited by:
Hans-Joachim Anders, Ludwig-Maximilians-Universität München, GermanyReviewed by:
Nicolas Riteau, National Institutes of Health (NIH), United StatesNiels Olsen Saraiva Camara, University of São Paulo, Brazil
Orestes Foresto-Neto, University of São Paulo, Brazil
Copyright: © 2018 Nakayama. This is an open-access article distributed under the terms of the Creative Commons Attribution License (CC BY). The use, distribution or reproduction in other forums is permitted, provided the original author(s) and the copyright owner are credited and that the original publication in this journal is cited, in accordance with accepted academic practice. No use, distribution or reproduction is permitted which does not comply with these terms.
*Correspondence: Masafumi Nakayama, bW5ha2F5YW1hQGZyaXMudG9ob2t1LmFjLmpw