- 1Technical University of Munich, Munich, Germany
- 2ZIEL—Institute for Food & Health, Technical University of Munich, Munich, Germany
The gastrointestinal (GI) tract provides a compartmentalized interface with an enormous repertoire of immune and metabolic activities, where the multicellular structure of the mucosa has acquired mechanisms to sense luminal factors, such as nutrients, microbes, and a variety of host-derived and microbial metabolites. The GI tract is colonized by a complex ecosystem of microorganisms, which have developed a highly coevolved relationship with the host’s cellular and immune system. Intestinal epithelial pattern recognition receptors (PRRs) substantially contribute to tissue homeostasis and immune surveillance. The role of bacteria-derived signals in intestinal epithelial homeostasis and repair has been addressed in mouse models deficient in PRRs and signaling adaptors. While critical for host physiology and the fortification of barrier function, the intestinal microbiota poses a considerable health challenge. Accumulating evidence indicates that dysbiosis is associated with the pathogenesis of numerous GI tract diseases, including inflammatory bowel diseases (IBD) and colorectal cancer (CRC). Aberrant signal integration at the epithelial cell level contributes to such diseases. An increased understanding of bacterial-specific structure recognition and signaling mechanisms at the intestinal epithelial interface is of great importance in the translation to future treatment strategies. In this review, we summarize the growing understanding of the regulation and function of the intestinal epithelial barrier, and discuss microbial signaling in the dynamic host–microbe mutualism in both health and disease.
Introduction
The human gastrointestinal (GI) tract represents the most densely colonized organ of the body, with the highest microbial load of 1011 bacteria/mL content in the colon (1, 2). Bacteria dominate the microbial ecosystem in the GI tract, with more than 90% belonging to the phyla Bacteroidetes and the Firmicutes (3–5). Despite considerable progress the functional complexity of the microbiome is still unresolved, and to date, mechanisms of microbe–host interactions involve a pleiotropic network of immune, metabolic, and trophic functions (1, 6). Studies in germ-free animals recognized the essential role played by the intestinal microbiome in the development and regulation of the mucosal immune system during early life (7–12). While many organisms have been shown to fulfill protective functions in the GI tract and are critical for host physiology, complex shifts in the community structure and abundance of certain microbes have been associated with the onset of inflammatory and tumorigenic diseases, such as inflammatory bowel diseases (IBD) and colorectal cancer (CRC) (6, 13–15).
Loss of epithelial barrier function and innate immunity are fundamental to the pathogenesis of inflammatory and infectious diseases. The intestinal immune system has the challenge of responding to pathogens, while remaining tolerant to food antigens and the commensal microbiota. The intestinal epithelium executes a compartmentalization between the lumen and the host, simultaneously acting as a selectively permeable first line of defense to fulfill its function of absorption, while maintaining an effective barrier against the intestinal microbiota, antigens and toxins. Intestinal epithelial cells (IECs) express pro-inflammatory cytokines in response to infectious invasive bacteria (16), but largely ignore non-pathogenic commensals (17). Certain intestinal pathogens (18, 19) and opportunistic commensals (20), however, can evade this first line of defense and enter IECs, suggesting that the existence of epithelial cell-intrinsic immune mechanisms for bacterial detection and limitation are essential. One key cell-autonomous mechanism of antibacterial defense is intestinal epithelial autophagy, shown to be activated following bacterial invasion through adaptor protein myeloid differentiation primary response gene-88 (MyD88) cell-intrinsic signaling, with autophagy-deficiency in mice causing increased dissemination of invasive bacteria (21), indicating that autophagy could have a broader role in inflammatory disease. IECs and innate immune cells of the lamina propria are able to differentiate self from non-self through a selective spatial and cellular expression of pattern-recognition receptors (PRRs) (22). Classically the detection of pathogen-associated molecular patterns (PAMPs) allows the intestinal epithelium to activate signaling pathways that induce the early host response to infection. The role of microbe-associated molecular patterns (MAMPs) in mediating innate recognition of the commensal “non-infectious” microbiota remains controversial. Paradoxically, recent progress in understanding IBD pathogenesis suggests that a defective innate immune system predisposes the host toward chronic inflammation (23, 24), supporting a protective role of PRR signaling in maintaining intestinal tissue homeostasis. Early work related to the activation of inflammation-related transcription factors, such as the nuclear factor kB (NF-kB), suggested a hormetic adaptation of the epithelium in response to commensal bacteria (25, 26), with elegant studies related to epithelial cell-specific inhibition of NF-kB activation validating the importance of this signaling pathway in maintaining tissue homeostasis (27). This paradigm shift was supported by Medzhitov and colleagues, demonstrating that microbiota-derived signals via the toll-like receptor (TLR)-related adaptor protein MyD88 protect mice from the development of colitis (28) and intestinal tumor formation (29). Thus, bacteria (dead or alive) and their metabolites form key mediators for the cross-talk between IECs and other mucosal cell types, through the interaction with host PRRs.
Although it is recognized that the intestinal microbiota has profound influences on health and disease, the understanding of the precise mechanism(s) by which this is exerted remains largely unknown (30). This review summarizes our knowledge of specific bacterial interactions and signaling mechanisms at the intestinal epithelial interface. We discuss bacterial signaling in inflammation and cancer, and reflect on how increasing knowledge of such mechanisms might be translated to the benefit of patient care.
The Intestinal Epithelium: Our Dynamic Protective Barrier
In spite of the symbiotic nature of the microbe–host relationship, the close proximity of bacteria to intestinal tissue poses a considerable health challenge. An effective and dynamic intestinal epithelial barrier is therefore crucial to conserve a compartmentalized microbe–host interaction and tissue homeostasis (Figure 1). In the healthy organ, the epithelium maintains a distinct anatomical barrier relevant for a constant state of homeostasis, while being exposed to a myriad of environmental stimuli that include, but are not limited to, microbes, dietary products and inorganic materials (31). A single-cell layer of IECs forms a continuous physical barrier, with tight junctions connecting adjacent IECs and associating with cytoplasmic actin and myosin networks that regulate intestinal permeability (32). Long-lived pluripotent stem cells located at the base of intestinal crypts continuously produce tissue-specific precursor cells that transit through a differentiation pathway that gives rise to absorptive lineage cells (enterocyte/colonocyte) or secretory lineage cells (goblet, Paneth, enteroendocrine and tuft) (33). IECs represent not only a physical barrier but also contribute to intestinal health through the production of mucus (goblet cells) and the secretion of antimicrobial peptides (AMPs) (Paneth cells).
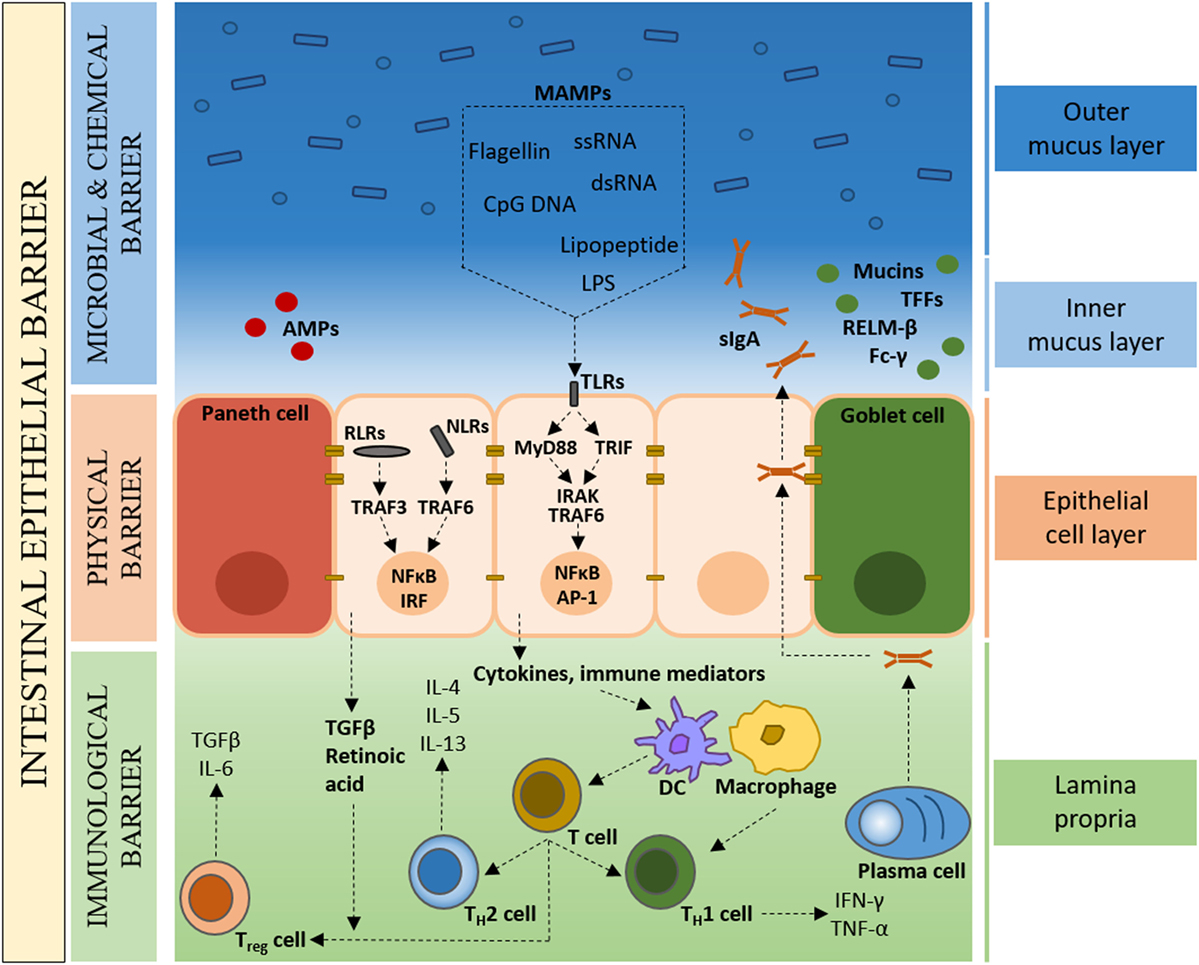
Figure 1. The colonic intestinal epithelium as a dynamic protective barrier. The single-cell layer (10 µm) of intestinal epithelial cells (IECs), which is comprised of distinct subpopulations, separates the luminal intestinal microbiota from the underlying tissue, forming a physical barrier. Overlying the IECs is the microbial and chemical barrier, mainly composed of the mucus layer(s). Goblet cells secrete mucins, which form a proteoglycan gel to create an inner mucus layer that is devoid of bacteria, and an outer mucus layer that forms a habitat for the intestinal microbiota. The largely sterile inner mucus layer has a high concentration of secretory immunoglobulin A (sIgA), antimicrobial peptides (AMPs), microbe-associated molecular patterns (MAMPs), as well as other bioactive molecules such as trefoil factor peptides (TFFs), resistin-like molecule β (RELMβ), and Fc-γ binding protein. Underlying the IECs, the Lipopeptide/lipoprotein (LP) contains mainly plasma cells, macrophages, and dendritic cells that, in the healthy state, are of a naïve nature with limited expression of inflammatory cytokines.
Goblet cells secrete mucin glycoproteins, of which Muc2 is the main constituent of the approximately 150-µm thick (in the mouse) colonic mucus layer (34). While the mucus layer in the small intestine consist of a single layer, in the colon, two structurally distinct mucus layers are formed; an inner mucus layer that is devoid of bacteria, and an outer mucus layer that forms a habitat for a large number of bacteria (35, 36). In addition to mucins, goblet cells secrete a range of bioactive molecules such as trefoil factor peptides (TFFs), resistin-like molecule β (RELMβ), and Fc-γ binding protein (37). Intestinal Paneth cells are the main source of AMPs that function in host defense and in establishing and maintaining the intestinal microbiota (38, 39). Secretory immunoglobulin A (sIgA) directed against intestinal bacteria and produced by Lipopeptide/lipoprotein (LP) plasma cells, binds the polymeric immunoglobulin receptor (pIgR), and transcytoses across the epithelium to prevent microbial translocation across the epithelial barrier (40, 41). This concerted interplay between plasma cells and IECs provides an adaptive immune element to the intestinal epithelial barrier. Also found scattered throughout the LP are T cells, stromal cells, and antigen presenting cells such as dendritic cells (DCs) and macrophages. Specialized IECs, called microfold (M) cells, and goblet cells facilitate the transport of luminal antigens and bacteria across the intestinal epithelial barrier to DCs, with macrophages sampling through trans-epithelial dendrites (42–44). Under steady-state conditions, the intestinal immune system detects commensal bacteria and provides basal signals without the full activation of adaptive immune responses (7).
The intestinal microbiota forms part of the intestinal barrier by limiting bacterial colonization and stimulating epithelial turnover (45). For example, Bifidobacteria species produce high concentrations of the short-chain fatty acid (SCFA) acetate, and can thereby prevent enteropathogenic Escherichia coli (EHEC) infection and its Shiga toxin release (46). Similarly, butyrate-producing Fecalibacterium prausnitzii, Eubacterium rectale, and Roseburia species directly target virulence gene expression to prevent bacterial infection (47). Studies have demonstrated that bacteria-dependent signals regulate the intestinal epithelial barrier and contribute to its effective functioning. Experiments in germ-free mice have shown that mucus layer thickness is reduced compared with conventionally housed mice, and that stimulation with lipopolysaccharide (LPS) and peptidoglycan (PGN) can reverse this to SPF-like levels of mucus thickness (48). Similarly, AMP and antimicrobial protein production, transcriptional- and post-translational regulation can be dependent on and enhanced by the presence of intestinal microbial signals (49–51). TLR, NOD-like receptor (NLR), RIG-like receptor (RLR), and C-type lectin receptor (CLR) family members provide distinct microbial signaling pathways in the intestinal epithelium (52–57). Despite evidence from mouse models deficient in PRRs and signaling adaptors (27, 52–56, 58), there is further need for epithelial-specific PRR knock-out mice to fully comprehend the role of bacteria-derived signals in intestinal epithelial homeostasis and repair.
Bacterial Recognition at the Intestinal Epithelial Interface
Of the four signaling receptor families (TLR, NLR, RLR, and CLR), members of the TLR family of type I transmembrane proteins are the best-characterized receptors in the intestinal mucosa. NLRs are cytoplasmic receptors, of which nucleotide-binding oligomerization domain-containing protein 1 (NOD1) and NOD2 functions have been well characterized, that signal to elicit cytokine, chemokine, and defensing expression (59). RLRs recognize viral RNAs and induce innate antiviral responses (60). TLRs can be located at the cell surface or internal cell compartments, respond to specific ligands, and are associated with particular adaptors that activate downstream signaling cascades. Nearly all TLRs are expressed in the human colon, with the expression of TLR1, TLR2, TLR3, TLR4, TLR5, and TLR9 demonstrated in IECs of the human small intestine (61). Studies have identified four main adaptor molecules [MyD88, MyD88-adapter-like (Mal/TIRAP), TIR domain-containing adaptor-inducing interferon-β (TRIF), and TRIF-related adaptor molecule (TRAM)] that interact with TLRs in response to ligand stimulation (62, 63).
With the exception of TLR3, all TLRs signal via the adaptor protein MyD88, whose engagement triggers signaling cascades that ultimately lead to the activation of transcription factors such as NF-ĸB, interferon regulatory factor (IRF) and activator protein 1 (AP-1) (64). While the lack of MyD88 in certain mouse strains was shown to have a significant impact on the composition of the intestinal microbiota, linking TLR signaling to the structure of the microbial community (65), a study published the same year using MyD88- and TLR-deficient mice and wild-type littermates, demonstrates that colony and housing differences between laboratories make it difficult to clearly define the influence of innate immune signaling pathways on the microbiota (66). Here, Ubeda et al. found that MyD88 and TLR signaling does not detectably alter the composition of the intestinal microbiota, demonstrating the need for caution in the interpretation of microbiota analysis in mutant mice. It is important to bear in mind that observations in MyD88-deficiency do not imply a direct link to microbial signals, but may in fact be intrinsic. Besides TLR receptors, MyD88 associates with all receptors of the IL-1 cytokine family, and contributes to tissue homeostasis, including tissue repair and regeneration (28, 67, 68). Therefore, the inability of MyD88-deficient mice to respond to the IL-1 cytokine family is likely also involved. In the colon epithelium, for example, it was shown that the protective effect of MyD88 is, at least in part, mediated by the IL-1 cytokine family member IL-18 (69).
The monoassociation of germ-free mice with the prominent gut commensal Bacteroides fragilis revealed that this bacterium specifically signals through TLR2 on regulatory T cell via its polysaccharide A (PSA) symbiosis factor, to enable its niche-specific mucosal colonization (70). Similarly, the colonization of mice with B. fragilis protects against experimental colitis in a TLR2-dependent manner (70, 71). Monocolonization in germ-free rats with the commensal Bifidobacterium lactis was shown to cause TLR2-mediated MAPK and NF-ĸB pathway activation in IECs (72). Furthermore, The colonization of germ-free rodents with Enterococcus faecalis or Bacteroides vulgatus activate NF-ĸB signaling and induce chemokine expression in colonic IECs through TLR2 and TLR4 signaling, respectively (26, 73).
A study in TLR5-deficient mice showed that the cecal microbiota differed from wild-type littermates in >100 bacterial phylotypes (74), indicating that TLR signaling has implications in the regulation of the intestinal microbiota. This was also shown in MyD88-deficient mice that demonstrated higher levels cecal Rikenellaceae and Porphyromonadaceae families (75). In the healthy state, mice deficient in TLR signaling (MyD88-deficient, TLR4-deficient, MyD88/TRIF-knockouts) do not show any differences in proliferation and IEC barrier function compared with wild-type mice (76, 77). Under conditions of injury, however, MyD88-, TLR2-, and TLR4-deficient mice show increased susceptibility to dextran sodium sulfate (DSS)-induced colitis (28, 77, 78). Despite the importance of PRRs in the bidirectional crosstalk between the intestinal microbiota and the host, studies in PRR-deficient mice have shown that only those deficient in TLR5, NLRP6, or RIG-I develop spontaneous intestinal inflammation (79–81). This may suggest a major role of compensatory mechanisms, where PAMPs are recognized by multiple synergizing host PRRs that share key innate immune signaling pathways, resulting in a sufficient host response to commensal bacteria in PRR-deficient mice that do not show spontaneous phenotypes. It is important to consider that not all laboratories and animal colonies observe spontaneous basal inflammation in the above-mentioned PRR-deficient mice (82).
Bacterial Signaling Mechanisms in Intestinal Inflammation
Despite difficulties in assigning the intestinal microbiota to the role of cause or consequence, chronic mucosal and, in particular, GI inflammation is linked to an imbalance of commensal bacteria and their gene products in patient groups with IBD (83–87). IBD is the collective name for multifactorial chronic relapsing inflammatory infections of the intestinal tract, which primarily includes Crohn’s disease (CD) and ulcerative colitis (UC). IBD can be debilitating and may lead to life-threatening complications. The development of IBD is characterized by a change in the normal intestinal microbiota (dysbiosis), with a reduction in both bacterial quantity and bacterial diversity (83, 88–90). In the context of IBD, microbiota analyses have negatively associated Faecalibacterium prausnitzii and Akkermansia municiphila with the disease, whereas Escherichia coli, Fusobacterium nucleatum, Haemophilus parainfluenzae, Veillonella parvula, Eikenella corrodens, and Gemella moribillum were shown to be positively associated with the inflammatory disease (86, 91–94). Dysbiosis is associated with a breakdown of host–microbial mutualism, with accumulating evidence from numerous scientific disciplines firmly implicating such a breakdown in mutualism in the pathogenesis of IBD (95, 96).
Abnormal PRR signaling is implicated in the development of chronic intestinal inflammation. The cytosolic NLR NOD2 (also known as CARD15) recognizes bacterial PGN-derived muramyl peptide (MDP) to elicit NF-ĸB-mediated proinflammatory responses and AMP synthesis (97–99). Nod2-deficient mice harbor an elevated load of commensal resident bacteria, display dysbiosis, and show a reduced ability to prevent intestinal pathogen colonization (100, 101). In turn, NOD2 expression is dependent on the intestinal microbiota, suggesting a feedback mechanism in the maintenance of intestinal homeostasis (101). In line with the above findings, Nod2 gene mutations were identified in patients with CD (102, 103), suggesting that Nod2 gene mutations may be associated with changes in the commensal microbiota that may facilitate disease progression.
The genetically engineered interleukin-10-deficient mouse (IL-10−/−) provides a model of spontaneous intestinal inflammation (104) and has been extensively used as an experimental tool to mirror the multifactorial nature of IBD. Evidence for the requirement of resident enteric bacteria for the development of colitis in IL10−/− mice stemmed from studies in germ-free animals, where colitis development was not observed (105). It has been shown that the gram-positive intestinal bacterium E. faecalis drives distal colonic inflammation in IL-10−/− mice following monoassociation (106, 107). Furthermore, increased mucosal growth of, and specific antibody-titers against, E. faecalis have been shown in patients with UC, also correlating with disease severity (108, 109). Findings from our own group identified that the virulence factor gelatinase E (GelE) partially impairs intestinal epithelial barrier integrity in IL-10−/− mice (110), and that the colitogenic activity of E. faecalis was partially and almost completely abrogated when deficient for the enterococcal polysaccharide antigen (ΔepaB) and lipoproteins (Δlgt) envelope structures, respectively (111). Monoassociation of IL-10−/− mice with the commensal bacteria E. faecalis, E. coli, or Pseudomonas fluorescens demonstrated that different commensal species selectively initiate distinct immune-mediated intestinal inflammation in the same host (107). Such results invite the hypothesis that particular microbial effectors, or a combination of effectors from different bacteria, are required to elicit pathogenesis or maintain the necessary barrier function for intestinal homeostasis. Additionally, not only the specific bacterium, but the susceptibility of the host plays a major role in disease progression, as shown by the induction of colitis by Bacteroides vulgatus in HLA/B27-β2m transgenic rats, but not in IL-2−/− mice (107, 112, 113).
Identifying bacterial gene products that drive protective rather than pathogenic inflammation in the intestine is crucial to rebalance homeostasis in inflammatory diseases and malignancies. Lactobacillus species, such as Lactobacillus acidophilus, are normal inhabitants of the intestinal microbiota and have received considerable attention as beneficial ecosystem members (114, 115). Several studies have shown that TLR2 regulates epithelial barrier function and enhances tight junction formation, as well as playing a crucial role in driving acute intestinal inflammation, but not chronic intestinal inflammation (116–118). L. acidophilus stimulates DCs through TLR2 via lipoteichoic acid (LTA) to trigger the production of inflammatory and regulatory cytokines (119–121). Deletion of the phosphoglycerol transferase gene (LBA0447) that synthesizes LTA generated an L. acidophilus derivative (NCK2025) that diminishes colitis when administered orally in a murine colitis model (122), confirming the role of LTA in inducing inflammation (123, 124). Of note here is that LTA, among others, may not present a true TLR2 ligand, as the large number of structurally diverse putative ligands may rather show their effects due to lipopeptide/lipoprotein (LP) contamination. In another example, L. paracasei, a single strain derived from the VSL#3 bacterial mixture clinically shown to reduce inflammation in IBD patients (125–127), was found to secrete the prtP-encoded protease lactocepin with anti-inflammatory effects via the degradation of proinflammatory chemokines (128, 129).
Collectively, the above findings support the notion that the colitogenic activity of opportunistic pathogens can be assigned to specific bacterial structures, and that such characterizations are indispensable in understanding host–microbe interactions relevant for the development of intestinal inflammation.
Bacterial Signaling Mechanisms in CRC
Colorectal cancer is one of the leading causes of death in the western society, being ranked third most lethal neoplasia in the United States in both men and women (130). Multiple lines of evidence show that the gut microbiota plays a major role in CRC development, both quantitatively and qualitatively. The significant role played by bacteria in inflammation-driven tumorigenesis is evident by the decreased tumor formation found in several CRC mouse models housed in germ-free conditions (131–133), or under antibiotic treatment (134). Accordingly, the inhibition of microbial recognition through the loss of PRR signaling or T-helper cell activation leads to a diminished neoplastic transformation (29, 131, 135, 136). Numerous bacterial species including, but not limited to, Streptococcus bovis, Bacteroides fragilis, and E. coli have been found in CRC samples. The best-known association is that of S. bovis bacteremia and CRC (137). It was demonstrated that S. bovis and its wall antigens induce IL-8 production, leading to the formation of nitric oxide (NO) and reactive oxygen species (ROS), which contribute to the neoplastic process (138). More recently, Peptostreptococcus anaerobius was identified as a candidate to be significantly enriched in the stool and mucosa of patients with CRC (139–141). A study assessed the association of P. anaerobius in stool and colonic tissue from patients with colorectal adenomas and adenocarcinomas, providing mechanistic insights that the actions of P. anaerobius are mediated via interaction with TLR2/4 on host cells to induce ROS production, increase cholesterol biosynthesis, and activate pro-oncogenic factors and pathways to promote CRC (142).
Approximately 80% of sporadic colorectal tumors are associated with mutations in the adenomatous polyposis coli (APC) gene (143); a central gatekeeper protein in CRC. Multiple intestinal neoplasia mice with a point mutation in Apc (ApcMin/+) mimic sporadic cancer and familial adenomatous polyposis, and have been used to study the role of TLR signaling in intestinal tumorigenesis through the crossing with MyD88-deficient mice (MYD88-deficient × ApcMin/+). While tumor incidence was similar in these mice compared with ApcMin/+ mice, a reduction in tumor number and size was observed, which was linked to a reduced expression of the tumor growth-promotor COX2 (29, 144). These data suggest that TLR signaling is involved in tumor growth, but not tumor initiation. Further evidence for the contribution of TLR signaling to the development of sporadic cancer, and colitis-associated cancer, stemmed from the use of TLR4-deficient mice that were protected against tumorigenesis following azoxymethane (AOM) and DSS treatment (145). Furthermore, TLR4 activation on tumor cells can prevent their lysis, thereby protecting cancer cells (146). This is of particular relevance with regard to cancer treatment strategies, as the immunosuppressant drug Rapamycin decreases TLR4 expression and its prostaglandin E2 production (147). Findings from animal models of CRC are corroborated by human studies; the TLR4/MyD88 co-receptor complex showed enhanced expression in approximately 20% of CRC patient samples, compared with normal mucosae and adenomas (148, 149).
Mechanistically, bacteria may promote tumorigenesis by numerous processes, including toxic metabolite production and genotoxic biosynthesis (150), thereby providing further CRC treatment tactics. One study aimed at inhibiting toxic effects of colibactin toxin-producing E. coli; frequent colonizers of CRC (151). Here, two identified boronic acid-based com pounds were shown to bind to the active site of the ClbP enzyme involved in the synthesis of colibactin, and shown to suppress DNA damage and tumorigenesis induced by pks-harboring [conserved genomic island coding for nonribosomal peptide synthetases (NRPS) and polyketide synthetases (PKS) bacteria (152)]. These findings not only confirm the role of colibactin toxin-producing E. coli in carcinogenesis but also provide a novel family of inhibitors to target pks-harboring bacteria in the treatment of CRC.
Injection of specific bacteria into tumor tissue may help eradicate tumors through the stimulation of inflammation and anti-tumor responses (153). In line with the above comment that a combination of multiple effectors may be necessary to maintain homeostasis or elicit pathogenesis: two bacteria can be better than one in cancer immunotherapy. A recent study applied an approach to cancer immunotherapy through the use of an attenuated Salmonella typhimurium strain engineered to secrete Vibrio vulnificus Flagellin B (FlaB) (154). Zheng et al. showed that FlaB-mediated tumor suppression is associated with TLR5-mediated host reactions and dependent on TLR4 and MyD88 signaling, as shown with TLR/MyD88 knockout mice and cell lines. Evidently it is feasible that non-virulent tumor-targeting bacteria can release multiple TLR ligands, and can be used as cancer immunotherapeutics.
In a latest study, Sahu et al. linked the dysbiotic behavior of a constitutively invasive variant of commensal non-pathogenic E. coli to CRC tumorigenesis (155). Aberrant host invasion leads to realignment of multiple host signal transduction cascades through reciprocal modulation of microbe sensing pathways Nod1/Rip2 and TLR/MyD88, leading to an expansion of the cancer stem cell population. This supports the notion that microbe-driven tumorigenesis may result from self-derived and contextual cues, which determine the role of such microbes in homeostasis and carcinogenesis, rather than strict correlations with commensal virulence.
Lactobacillus acidophilus NCK2025, discussed earlier with regards to the regulation of inflammation in a colitis model, was investigated in a mouse model of colonic polyposis (TS4Cre × APClox468) to assess its moderation of pathogenic inflammation within the tumor microenvironment (156). Khazaie et al. reported that oral treatment with the LTA-deficient L. acidophilus NCK2025 normalized innate and adaptive pathogenic immune responses, causing a regression of established precancerous colonic polyps. This work demonstrates the ability of the probiotic strain with anti-inflammatory properties to reverse preneoplasia, rendering this L. acidophilus strain as a potential candidate for regulating intestinal immunity in the protection against inflammation and CRC susceptibility. Additional investigations are key to further characterize bacterial gene products that can influence inflammation to restore intestinal homeostasis, to provide novel avenues for the treatment and prevention of inflammatory and cancer pathologies.
In light of high-sensitivity-detection of pre-cancerous lesions still posing a great challenge, the potential of fecal microbiota for the early-stage detection of CRC was recently investigated (157). In a metagenomic sequencing study to identify taxonomic markers in CRC patients, Zeller et al. found functional and taxonomic associations with CRC from noninvasive fecal sample readouts. Furthermore, general dysbiosis common to inflammation was addressed by including published metagenomes from IBD patients in the marker species classifier, showing that stronger associations were observed with CRC, with only modest influences by inflammation-related microbiota changes. This study demonstrates the possibility of CRC detection from fecal microbial markers, and the potential for further identification of cancer-associated differences in gene function, gene content and genomic variation through additional metagenomic data.
Concluding Remarks
Over the years, it has become evident that the intestinal microbiota is not merely a bystander in the complex events that regulate intestinal homeostasis, but that it plays a fundamental role in eliciting both beneficial and detrimental effects in the host. Collectively, the studies outlined in this review highlight the diverse and multifaceted roles of IECs and the intestinal microbiota in the maintenance of intestinal homeostasis, and the complexity of the relationship between the two. The diverse barrier functions of the intestinal epithelium play a crucial role in microbe–host mutualism. Cells of the intestinal epithelium express a range of PRRs that sense and respond to a variety of microbial signals to maintain an effective barrier and respond to pathogens (Figure 2). Evidence of the importance of PRR signaling stems from studies in mice with specific defects in such signaling pathways, which show increased susceptibility to developing disease (28). Regarding the host side of the mutualism, future studies to increase our understanding of how mucus, AMPs, and sIgA dynamics can be regulated to maintain barrier function will provide avenues to develop therapeutic interventions for preserving intestinal homeostasis. Probiotic and prebiotic treatment options available to consumers are currently drawn from a narrow range of organisms. Increasing knowledge of the intestinal microbiota with its constituents is changing this paradigm; however, due to the complex and dynamic nature of the intestinal ecosystem, the mechanistic understanding of the integration of bacterial signals remains a great challenge to this field. Antibiotics selectively targeting bacterial pathogens have been extensively used in the prevention and treatment of numerous diseases (158, 159). In light of antibiotics disrupting the composition of the enteric intestinal microbiota and promoting antibiotic resistance, future mechanistic experimental efforts to elucidate (yet) unidentified mechanisms of bacterial effector proteins to enable the development of novel drugs aimed at targeting rather than killing bacterial pathogens, seems like the logical step forward. To this end, animal models of inflammation and cancer provide useful approaches to demonstrate functionality, given the high interindividual variation and nature of studies using human cohorts.
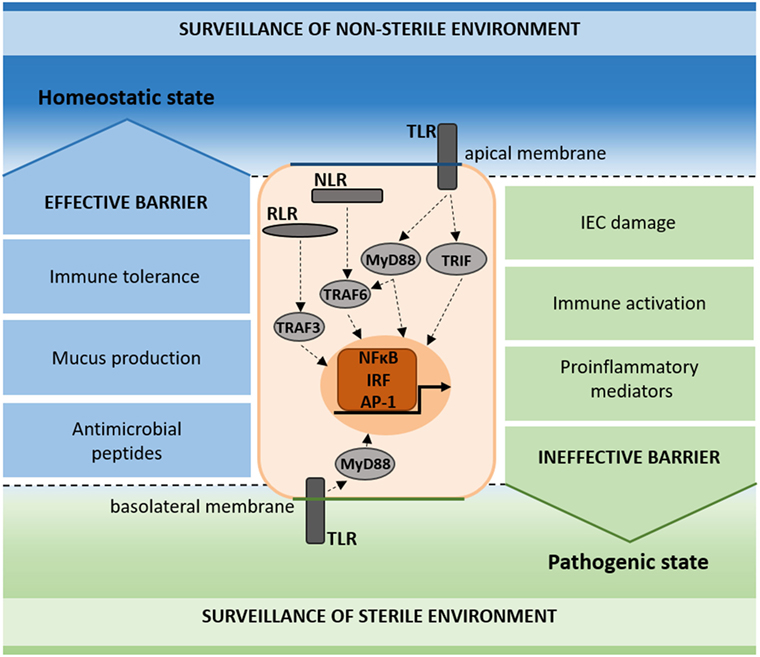
Figure 2. Schematic representation of pattern recognition receptor (PRR) surveillance in the homeostatic and pathogenic state. PRRs (TLR, NLR, and RLR) signal on the apical and basolateral membrane of intestinal epithelial cells (IECs), contributing to the surveillance of the non-sterile (apical) and sterile (basal) environments. In the homeostatic state, immune tolerance, mucus production, and antimicrobial peptides add to the maintenance of an effective barrier (blue). In the pathogenic state, IEC damage, immune activation, and proinflammatory mediators result in an ineffective barrier (green).
Author Contributions
All authors listed have made a substantial, direct, and intellectual contribution to the work and approved it for publication.
Conflict of Interest Statement
The authors declare that the research was conducted in the absence of any commercial or financial relationships that could be construed as a potential conflict of interest.
The reviewer MH declared a past coauthorship with one of the authors, DH, to the handling editor.
Funding
This work was supported by the German Research Foundation (DFG) and the Technical University of Munich (TUM) in the framework of the Open Access Publishing Program.
References
1. Berg RD. The indigenous gastrointestinal microflora. Trends Microbiol (1996) 4(11):430–5. doi:10.1016/0966-842X(96)10057-3
2. Ablikim M, Achasov MN, Alberto D, An Q, An ZH, Bai JZ, et al. etapi+ pi- resonant structure around 1.8 GeV/c(2) and eta(1405) in J/psi – > omegaetapi+ pi. Phys Rev Lett (2011) 107(18):182001. doi:10.1103/PhysRevLett.107.182001
3. Turroni F, Ribbera A, Foroni E, van Sinderen D, Ventura M. Human gut microbiota and bifidobacteria: from composition to functionality. Antonie Van Leeuwenhoek (2008) 94(1):35–50. doi:10.1007/s10482-008-9232-4
4. Zoetendal EG, Vaughan EE, de Vos WM. A microbial world within us. Mol Microbiol (2006) 59(6):1639–50. doi:10.1111/j.1365-2958.2006.05056.x
5. Bäckhed F, Ley RE, Sonnenburg JL, Peterson DA, Gordon JI. Host-bacterial mutualism in the human intestine. Science (2005) 307(5717):1915–20. doi:10.1126/science.1104816
6. Guarner F, Malagelada JR. Gut flora in health and disease. Lancet (2003) 361(9356):512–9. doi:10.1016/S0140-6736(03)12489-0
7. Macpherson AJ, Harris NL. Interactions between commensal intestinal bacteria and the immune system. Nat Rev Immunol (2004) 4(6):478–85. doi:10.1038/nri1373
8. Falk PG, Hooper LV, Midtvedt T, Gordon JI. Creating and maintaining the gastrointestinal ecosystem: what we know and need to know from gnotobiology. Microbiol Mol Biol Rev (1998) 62(4):1157–70.
9. Bouskra D, Brézillon C, Bérard M, Werts C, Varona R, Boneca IG, et al. Lymphoid tissue genesis induced by commensals through NOD1 regulates intestinal homeostasis. Nature (2008) 456(7221):507–10. doi:10.1038/nature07450
10. Abrams GD, Bauer H, Sprinz H. Influence of the normal flora on mucosal morphology and cellular renewal in the ileum. A comparison of germ-free and conventional mice. Lab Invest (1963) 12:355–64.
11. Bry L, Falk PG, Midtvedt T, Gordon JI. A model of host-microbial interactions in an open mammalian ecosystem. Science (1996) 273(5280):1380–3. doi:10.1126/science.273.5280.1380
12. Hooper LV, Gordon JI. Commensal host-bacterial relationships in the gut. Science (2001) 292(5519):1115–8. doi:10.1126/science.1058709
13. Coleman OI, Nunes T. Role of the microbiota in colorectal cancer: updates on microbial associations and therapeutic implications. Biores Open Access (2016) 5(1):279–88. doi:10.1089/biores.2016.0028
14. Zhang YJ, Li S, Gan RY, Zhou T, Xu DP, Li HB. Impacts of gut bacteria on human health and diseases. Int J Mol Sci (2015) 16(4):7493–519. doi:10.3390/ijms16047493
15. Mueller C, Macpherson AJ. Layers of mutualism with commensal bacteria protect us from intestinal inflammation. Gut (2006) 55(2):276–84. doi:10.1136/gut.2004.054098
16. Eckmann L, Jung HC, Schürer-Maly C, Panja A, Morzycka-Wroblewska E, Kagnoff MF, et al. Differential cytokine expression by human intestinal epithelial cell lines: regulated expression of interleukin 8. Gastroenterology (1993) 105(6):1689–97. doi:10.1016/0016-5085(93)91064-O
17. Melmed G, Thomas LS, Lee N, Tesfay SY, Lukasek K, Michelsen KS, et al. Human intestinal epithelial cells are broadly unresponsive to toll-like receptor 2-dependent bacterial ligands: implications for host-microbial interactions in the gut. J Immunol (2003) 170(3):1406–15. doi:10.4049/jimmunol.170.3.1406
18. Eichelberg K, Galan JE. Differential regulation of Salmonella typhimurium type III secreted proteins by pathogenicity island 1 (SPI-1)-encoded transcriptional activators InvF and hilA. Infect Immun (1999) 67(8):4099–105.
19. Müller AJ, Kaiser P, Dittmar KE, Weber TC, Haueter S, Endt K, et al. Salmonella gut invasion involves TTSS-2-dependent epithelial traversal, basolateral exit, and uptake by epithelium-sampling lamina propria phagocytes. Cell Host Microbe (2012) 11(1):19–32. doi:10.1016/j.chom.2011.11.013
20. Klare I, Werner G, Witte W. Enterococci. Habitats, infections, virulence factors, resistances to antibiotics, transfer of resistance determinants. Contrib Microbiol (2001) 8:108–22. doi:10.1159/000060406
21. Benjamin JL, Sumpter R Jr, Levine B, Hooper LV. Intestinal epithelial autophagy is essential for host defense against invasive bacteria. Cell Host Microbe (2013) 13(6):723–34. doi:10.1016/j.chom.2013.05.004
22. Medzhitov R, Janeway CA Jr. Decoding the patterns of self and nonself by the innate immune system. Science (2002) 296(5566):298–300. doi:10.1126/science.1068883
23. Liu JZ, van Sommeren S, Huang H, Ng SC, Alberts R, Takahashi A, et al. Association analyses identify 38 susceptibility loci for inflammatory bowel disease and highlight shared genetic risk across populations. Nat Genet (2015) 47(9):979–86. doi:10.1038/ng.3359
24. Rivas MA, Beaudoin M, Gardet A, Stevens C, Sharma Y, Zhang CK, et al. Deep resequencing of GWAS loci identifies independent rare variants associated with inflammatory bowel disease. Nat Genet (2011) 43(11):1066–73. doi:10.1038/ng.952
25. Haller D, Russo MP, Sartor RB, Jobin C. IKK beta and phosphatidylinositol 3-kinase/Akt participate in non-pathogenic Gram-negative enteric bacteria-induced RelA phosphorylation and NF-kappa B activation in both primary and intestinal epithelial cell lines. J Biol Chem (2002) 277(41):38168–78. doi:10.1074/jbc.M205737200
26. Haller D, Holt L, Kim SC, Schwabe RF, Sartor RB, Jobin C. Transforming growth factor-beta 1 inhibits non-pathogenic Gram negative bacteria-induced NF-kappa B recruitment to the interleukin-6 gene promoter in intestinal epithelial cells through modulation of histone acetylation. J Biol Chem (2003) 278(26):23851–60. doi:10.1074/jbc.M300075200
27. Nenci A, Becker C, Wullaert A, Gareus R, van Loo G, Danese S, et al. Epithelial NEMO links innate immunity to chronic intestinal inflammation. Nature (2007) 446(7135):557–61. doi:10.1038/nature05698
28. Rakoff-Nahoum S, Paglino J, Eslami-Varzaneh F, Edberg S, Medzhitov R. Recognition of commensal microflora by toll-like receptors is required for intestinal homeostasis. Cell (2004) 118(2):229–41. doi:10.1016/j.cell.2004.07.002
29. Rakoff-Nahoum S, Medzhitov R. Regulation of spontaneous intestinal tumorigenesis through the adaptor protein MyD88. Science (2007) 317(5834):124–7. doi:10.1126/science.1140488
30. Butto LF, Haller D. Functional relevance of microbiome signatures: the correlation era requires tools for consolidation. J Allergy ClinImmunol (2017) 139(4):1092–8. doi:10.1016/j.jaci.2017.02.010
31. Hooper LV, Littman DR, Macpherson AJ. Interactions between the microbiota and the immune system. Science (2012) 336(6086):1268–73. doi:10.1126/science.1223490
32. Van Itallie CM, Anderson JM. Architecture of tight junctions and principles of molecular composition. Semin Cell Dev Biol (2014) 36:157–65. doi:10.1016/j.semcdb.2014.08.011
33. van der Flier LG, Clevers H. Stem cells, self-renewal, and differentiation in the intestinal epithelium. Annu Rev Physiol (2009) 71:241–60. doi:10.1146/annurev.physiol.010908.163145
34. Malmberg EK, Noaksson KA, Phillipson M, Johansson ME, Hinojosa-Kurtzberg M, Holm L, et al. Increased levels of mucins in the cystic fibrosis mouse small intestine, and modulator effects of the Muc1 mucin expression. Am J Physiol Gastrointest Liver Physiol (2006) 291(2):G203–10. doi:10.1152/ajpgi.00491.2005
35. Johansson MEV, Larsson JMH, Hansson GC. The two mucus layers of colon are organized by the MUC2 mucin, whereas the outer layer is a legislator of host-microbial interactions. Proc Natl Acad Sci U S A (2011) 108:4659–65. doi:10.1073/pnas.1006451107
36. Johansson ME, Phillipson M, Petersson J, Velcich A, Holm L, Hansson GC. The inner of the two Muc2 mucin-dependent mucus layers in colon is devoid of bacteria. Proc Natl Acad Sci U S A (2008) 105(39):15064–9. doi:10.1073/pnas.0803124105
37. Dharmani P, Srivastava V, Kissoon-Singh V, Chadee K. Role of intestinal mucins in innate host defense mechanisms against pathogens. J Innate Immun (2009) 1(2):123–35. doi:10.1159/000163037
38. Porter EM, Bevins CL, Ghosh D, Ganz T. The multifaceted Paneth cell. Cell Mol Life Sci (2002) 59(1):156–70. doi:10.1007/s00018-002-8412-z
39. Ouellette AJ. Paneth cells and innate mucosal immunity. Curr Opin Gastroenterol (2010) 26(6):547–53. doi:10.1097/MOG.0b013e32833dccde
40. Johansen FE, Kaetzel CS. Regulation of the polymeric immunoglobulin receptor and IgA transport: new advances in environmental factors that stimulate pIgR expression and its role in mucosal immunity. Mucosal Immunol (2011) 4(6):598–602. doi:10.1038/mi.2011.37
41. Macpherson AJ, Uhr T. Induction of protective IgA by intestinal dendritic cells carrying commensal bacteria. Science (2004) 303(5664):1662–5. doi:10.1126/science.1091334
42. Mabbott NA, Donaldson DS, Ohno H, Williams IR, Mahajan A. Microfold (M) cells: important immunosurveillance posts in the intestinal epithelium. Mucosal Immunol (2013) 6(4):666–77. doi:10.1038/mi.2013.30
43. McDole JR, Wheeler LW, McDonald KG, Wang B, Konjufca V, Knoop KA, et al. Goblet cells deliver luminal antigen to CD103+ dendritic cells in the small intestine. Nature (2012) 483(7389):345–9. doi:10.1038/nature10863
44. Rescigno M, Urbano M, Valzasina B, Francolini M, Rotta G, Bonasio R, et al. Dendritic cells express tight junction proteins and penetrate gut epithelial monolayers to sample bacteria. Nat Immunol (2001) 2(4):361–7. doi:10.1038/86373
45. Savage DC, Siegel JE, Snellen JE, Whitt DD. Transit time of epithelial cells in the small intestines of germfree mice and ex-germfree mice associated with indigenous microorganisms. Appl Environ Microbiol (1981) 42(6):996–1001.
46. Fukuda S, Toh H, Hase K, Oshima K, Nakanishi Y, Yoshimura K, et al. Bifidobacteria can protect from enteropathogenic infection through production of acetate. Nature (2011) 469(7331):543–7. doi:10.1038/nature09646
47. Guilloteau P, Martin L, Eeckhaut V, Ducatelle R, Zabielski R, Van Immerseel F. From the gut to the peripheral tissues: the multiple effects of butyrate. Nutr Res Rev (2010) 23(2):366–84. doi:10.1017/S0954422410000247
48. Petersson J, Schreiber O, Hansson GC, Gendler SJ, Velcich A, Lundberg JO, et al. Importance and regulation of the colonic mucus barrier in a mouse model of colitis. Am J Physiol Gastrointest Liver Physiol (2011) 300(2):G327–33. doi:10.1152/ajpgi.00422.2010
49. Vaishnava S, Behrendt CL, Ismail AS, Eckmann L, Hooper LV. Paneth cells directly sense gut commensals and maintain homeostasis at the intestinal host-microbial interface. Proc Natl Acad Sci U S A (2008) 105(52):20858–63. doi:10.1073/pnas.0808723105
50. Vaishnava S, Yamamoto M, Severson KM, Ruhn KA, Yu X, Koren O, et al. The antibacterial lectin RegIIIgamma promotes the spatial segregation of microbiota and host in the intestine. Science (2011) 334(6053):255–8. doi:10.1126/science.1209791
51. Gallo RL, Hooper LV. Epithelial antimicrobial defence of the skin and intestine. Nat Rev Immunol (2012) 12(7):503–16. doi:10.1038/nri3228
52. Abreu MT. Toll-like receptor signalling in the intestinal epithelium: how bacterial recognition shapes intestinal function. Nat Rev Immunol (2010) 10(2):131–44. doi:10.1038/nri2707
53. Chen GY, Nunez G. Inflammasomes in intestinal inflammation and cancer. Gastroenterology (2011) 141(6):1986–99. doi:10.1053/j.gastro.2011.10.002
54. Elinav E, Henao-Mejia J, Flavell RA. Integrative inflammasome activity in the regulation of intestinal mucosal immune responses. Mucosal Immunol (2013) 6(1):4–13. doi:10.1038/mi.2012.115
55. Li XD, Chiu YH, Ismail AS, Behrendt CL, Wight-Carter M, Hooper LV, et al. Mitochondrial antiviral signaling protein (MAVS) monitors commensal bacteria and induces an immune response that prevents experimental colitis. Proc Natl Acad Sci U S A (2011) 108(42):17390–5. doi:10.1073/pnas.1107114108
56. Broquet AH, Hirata Y, McAllister CS, Kagnoff MF. RIG-I/MDA5/MAVS are required to signal a protective IFN response in rotavirus-infected intestinal epithelium. J Immunol (2011) 186(3):1618–26. doi:10.4049/jimmunol.1002862
57. Geijtenbeek TB, van Vliet SJ, Engering A, ’t Hart BA, van Kooyk Y. Self- and nonself-recognition by C-type lectins on dendritic cells. Annu Rev Immunol (2004) 22:33–54. doi:10.1146/annurev.immunol.22.012703.104558
58. Greten FR, Eckmann L, Greten TF, Park JM, Li ZW, Egan LJ, et al. IKKbeta links inflammation and tumorigenesis in a mouse model of colitis-associated cancer. Cell (2004) 118(3):285–96. doi:10.1016/j.cell.2004.07.013
59. Williams A, Flavell RA, Eisenbarth SC. The role of NOD-like receptors in shaping adaptive immunity. Curr Opin Immunol (2010) 22(1):34–40. doi:10.1016/j.coi.2010.01.004
60. Rehwinkel J, Reis e Sousa C. RIGorous detection: exposing virus through RNA sensing. Science (2010) 327(5963):284–6. doi:10.1126/science.1185068
61. Otte JM, Cario E, Podolsky DK. Mechanisms of cross hyporesponsiveness to toll-like receptor bacterial ligands in intestinal epithelial cells. Gastroenterology (2004) 126(4):1054–70. doi:10.1053/j.gastro.2004.01.007
62. Akashi-Takamura S, Miyake K. TLR accessory molecules. Curr Opin Immunol (2008) 20(4):420–5. doi:10.1016/j.coi.2008.07.001
63. Kenny EF, O’Neill LA. Signalling adaptors used by toll-like receptors: an update. Cytokine (2008) 43(3):342–9. doi:10.1016/j.cyto.2008.07.010
64. Kumar H, Kawai T, Akira S. Toll-like receptors and innate immunity. Biochem Biophys Res Commun (2009) 388(4):621–5. doi:10.1016/j.bbrc.2009.08.062
65. Larsson E, Tremaroli V, Lee YS, Koren O, Nookaew I, Fricker A, et al. Analysis of gut microbial regulation of host gene expression along the length of the gut and regulation of gut microbial ecology through MyD88. Gut (2012) 61(8):1124–31. doi:10.1136/gutjnl-2011-301104
66. Ubeda C, Lipuma L, Gobourne A, Viale A, Leiner I, Equinda M, et al. Familial transmission rather than defective innate immunity shapes the distinct intestinal microbiota of TLR-deficient mice. J Exp Med (2012) 209(8):1445–56. doi:10.1084/jem.20120504
67. Macedo L, Pinhal-Enfield G, Alshits V, Elson G, Cronstein BN, Leibovich SJ. Wound healing is impaired in MyD88-deficient mice: a role for MyD88 in the regulation of wound healing by adenosine A2A receptors. Am J Pathol (2007) 171(6):1774–88. doi:10.2353/ajpath.2007.061048
68. Iimuro Y, Seki E, Son G, Tsutsui H, Nakanishi K, Fujimoto J. Role of innate immune response in liver regeneration. J Gastroenterol Hepatol (2007) 22(Suppl 1):S57–8. doi:10.1111/j.1440-1746.2006.04651.x
69. Salcedo R, Worschech A, Cardone M, Jones Y, Gyulai Z, Dai RM, et al. MyD88-mediated signaling prevents development of adenocarcinomas of the colon: role of interleukin 18. J Exp Med (2010) 207(8):1625–36. doi:10.1084/jem.20100199
70. Round JL, Lee SM, Li J, Tran G, Jabri B, Chatila TA, et al. The toll-like receptor 2 pathway establishes colonization by a commensal of the human microbiota. Science (2011) 332(6032):974–7. doi:10.1126/science.1206095
71. Mazmanian SK, Round JL, Kasper DL. A microbial symbiosis factor prevents intestinal inflammatory disease. Nature (2008) 453(7195):620–5. doi:10.1038/nature07008
72. Ruiz PA, Hoffmann M, Szcesny S, Blaut M, Haller D. Innate mechanisms for Bifidobacterium lactis to activate transient pro-inflammatory host responses in intestinal epithelial cells after the colonization of germ-free rats. Immunology (2005) 115(4):441–50. doi:10.1111/j.1365-2567.2005.02176.x
73. Ruiz PA, Shkoda A, Kim SC, Sartor RB, Haller D. IL-10 gene-deficient mice lack TGF-beta/Smad-mediated TLR2 degradation and fail to inhibit proinflammatory gene expression in intestinal epithelial cells under conditions of chronic inflammation. Ann N Y Acad Sci (2006) 1072:389–94. doi:10.1196/annals.1326.023
74. Vijay-Kumar M, Aitken JD, Carvalho FA, Cullender TC, Mwangi S, Srinivasan S, et al. Metabolic syndrome and altered gut microbiota in mice lacking toll-like receptor 5. Science (2010) 328(5975):228–31. doi:10.1126/science.1179721
75. Wen L, Ley RE, Volchkov PY, Stranges PB, Avanesyan L, Stonebraker AC, et al. Innate immunity and intestinal microbiota in the development of type 1 diabetes. Nature (2008) 455(7216):1109–13. doi:10.1038/nature07336
76. Slack E, Hapfelmeier S, Stecher B, Velykoredko Y, Stoel M, Lawson MA, et al. Innate and adaptive immunity cooperate flexibly to maintain host-microbiota mutualism. Science (2009) 325(5940):617–20. doi:10.1126/science.1172747
77. Fukata M, Michelsen KS, Eri R, Thomas LS, Hu B, Lukasek K, et al. Toll-like receptor-4 is required for intestinal response to epithelial injury and limiting bacterial translocation in a murine model of acute colitis. Am J Physiol Gastrointest Liver Physiol (2005) 288(5):G1055–65. doi:10.1152/ajpgi.00328.2004
78. Araki A, Kanai T, Ishikura T, Makita S, Uraushihara K, Iiyama R, et al. MyD88-deficient mice develop severe intestinal inflammation in dextran sodium sulfate colitis. J Gastroenterol (2005) 40(1):16–23. doi:10.1007/s00535-004-1492-9
79. Vijay-Kumar M, Sanders CJ, Taylor RT, Kumar A, Aitken JD, Sitaraman SV, et al. Deletion of TLR5 results in spontaneous colitis in mice. J Clin Invest (2007) 117(12):3909–21. doi:10.1172/JCI33084
80. Wang Y, Zhang HX, Sun YP, Liu ZX, Liu XS, Wang L, et al. Rig-I-/- mice develop colitis associated with downregulation of G alpha i2. Cell Res (2007) 17(10):858–68. doi:10.1038/cr.2007.81
81. Elinav E, Strowig T, Kau AL, Henao-Mejia J, Thaiss CA, Booth CJ, et al. NLRP6 inflammasome regulates colonic microbial ecology and risk for colitis. Cell (2011) 145(5):745–57. doi:10.1016/j.cell.2011.04.022
82. Letran SE, Lee SJ, Atif SM, Flores-Langarica A, Uematsu S, Akira S, et al. TLR5-deficient mice lack basal inflammatory and metabolic defects but exhibit impaired CD4 T cell responses to a flagellated pathogen. J Immunol (2011) 186(9):5406–12. doi:10.4049/jimmunol.1003576
83. Frank DN, St Amand AL, Feldman RA, Boedeker EC, Harpaz N, Pace NR. Molecular-phylogenetic characterization of microbial community imbalances in human inflammatory bowel diseases. Proc Natl Acad Sci U S A (2007) 104(34):13780–5. doi:10.1073/pnas.0706625104
84. Willing BP, Dicksved J, Halfvarson J, Andersson AF, Lucio M, Zheng Z, et al. A pyrosequencing study in twins shows that gastrointestinal microbial profiles vary with inflammatory bowel disease phenotypes. Gastroenterology (2010) 139(6):1844–U105. doi:10.1053/j.gastro.2010.08.049
85. Joossens M, Huys G, Cnockaert M, De Preter V, Verbeke K, Rutgeerts P, et al. Dysbiosis of the faecal microbiota in patients with Crohn’s disease and their unaffected relatives. Gut (2011) 60(5):631–7. doi:10.1136/gut.2010.223263
86. Gevers D, Kugathasan S, Denson LA, Vázquez-Baeza Y, Van Treuren W, Ren B, et al. The treatment-naive microbiome in new-onset Crohn’s disease. Cell Host Microbe (2014) 15(3):382–92. doi:10.1016/j.chom.2014.02.005
87. Lee T, Clavel T, Smirnov K, Schmidt A, Lagkouvardos I, Walker A, et al. Oral versus intravenous iron replacement therapy distinctly alters the gut microbiota and metabolome in patients with IBD. Gut (2016) 66(5):863–71. doi:10.1136/gutjnl-2015-309940
88. Lepage P, Häsler R, Spehlmann ME, Rehman A, Zvirbliene A, Begun A, et al. Twin study indicates loss of interaction between microbiota and mucosa of patients with ulcerative colitis. Gastroenterology (2011) 141(1):227–36. doi:10.1053/j.gastro.2011.04.011
89. Willing B, Halfvarson J, Dicksved J, Rosenquist M, Järnerot G, Engstrand L, et al. Twin studies reveal specific imbalances in the mucosa-associated microbiota of patients with ileal Crohn’s disease. Inflamm Bowel Dis (2009) 15(5):653–60. doi:10.1002/ibd.20783
90. Gevers D, Kugathasan S, Denson LA, Vázquez-Baeza Y, Van Treuren W, Ren B, et al. The treatment-naive microbiome in early-onset Crohn’s disease. Gastroenterology (2014) 146(5):S144–144. doi:10.1016/S0016-5085(14)60512-6
91. Machiels K, Joossens M, Sabino J, De Preter V, Arijs I, Eeckhaut V, et al. A decrease of the butyrate-producing species Roseburia hominis and Faecalibacterium prausnitzii defines dysbiosis in patients with ulcerative colitis. Gut (2014) 63(8):1275–83. doi:10.1136/gutjnl-2013-304833
92. Sokol H, Pigneur B, Watterlot L, Lakhdari O, Bermúdez-Humarán LG, Gratadoux JJ, et al. Faecalibacterium prausnitzii is an anti-inflammatory commensal bacterium identified by gut microbiota analysis of Crohn disease patients. Proc Natl Acad Sci U S A (2008) 105(43):16731–6. doi:10.1073/pnas.0804812105
93. James SL, Christophersen CT, Bird AR, Conlon MA, Rosella O, Gibson PR, et al. Abnormal fibre usage in UC in remission. Gut (2015) 64(4):562–70. doi:10.1136/gutjnl-2014-307198
94. Rajilić-Stojanović M, Shanahan F, Guarner F, de Vos WM. Phylogenetic analysis of dysbiosis in ulcerative colitis during remission. Inflamm Bowel Dis (2013) 19(3):481–8. doi:10.1097/MIB.0b013e31827fec6d
95. Sartor RB, Blumberg RS, Braun J, Elson CO, Mayer LF. CCFA microbial-host interactions workshop: highlights and key observations. Inflamm Bowel Dis (2007) 13(5):600–19. doi:10.1002/ibd.20114
96. Butto LF, Schaubeck M, Haller D. Mechanisms of microbe-host interaction in Crohn’s disease: dysbiosis vs. pathobiont selection. Front Immunol (2015) 6:555. doi:10.3389/fimmu.2015.00555
97. Lecat A, Piette J, Legrand-Poels S. The protein Nod2: an innate receptor more complex than previously assumed. Biochem Pharmacol (2010) 80(12):2021–31. doi:10.1016/j.bcp.2010.07.016
98. Kobayashi KS, Chamaillard M, Ogura Y, Henegariu O, Inohara N, Nuñez G, et al. Nod2-dependent regulation of innate and adaptive immunity in the intestinal tract. Science (2005) 307(5710):731–4. doi:10.1126/science.1104911
99. Inohara N, Ogura Y, Fontalba A, Gutierrez O, Pons F, Crespo J, et al. Host recognition of bacterial muramyl dipeptide mediated through NOD2. J Biol Chem (2003) 278(8):5509–12. doi:10.1074/jbc.C200673200
100. Rehman A, Sina C, Gavrilova O, Häsler R, Ott S, Baines JF, et al. Nod2 is essential for temporal development of intestinal microbial communities. Gut (2011) 60(10):1354–62. doi:10.1136/gut.2010.216259
101. Petnicki-Ocwieja T, Hrncir T, Liu YJ, Biswas A, Hudcovic T, Tlaskalova-Hogenova H, et al. Nod2 is required for the regulation of commensal microbiota in the intestine. Proc Natl Acad Sci U S A (2009) 106(37):15813–8. doi:10.1073/pnas.0907722106
102. Lacher M, Helmbrecht J, Schroepf S, Koletzko S, Ballauff A, Classen M, et al. NOD2 mutations predict the risk for surgery in pediatric-onset Crohn’s disease. J Pediatr Surg (2010) 45(8):1591–7. doi:10.1016/j.jpedsurg.2009.10.046
103. Gutiérrez A, Holler E, Zapater P, Sempere L, Jover R, Pérez-Mateo M, et al. Antimicrobial peptide response to blood translocation of bacterial DNA in Crohn’s disease is affected by NOD2/CARD15 genotype. Inflamm Bowel Dis (2011) 17(8):1641–50. doi:10.1002/ibd.21537
104. Kühn R, Löhler J, Rennick D, Rajewsky K, Müller W. Interleukin-10-deficient mice develop chronic enterocolitis. Cell (1993) 75(2):263–74. doi:10.1016/0092-8674(93)80068-P
105. Sellon RK, Tonkonogy S, Schultz M, Dieleman LA, Grenther W, Balish E, et al. Resident enteric bacteria are necessary for development of spontaneous colitis and immune system activation in interleukin-10-deficient mice. Infect Immun (1998) 66(11):5224–31.
106. Balish E, Warner T. Enterococcus faecalis induces inflammatory bowel disease in interleukin-10 knockout mice. Am J Pathol (2002) 160(6):2253–7. doi:10.1016/S0002-9440(10)61172-8
107. Kim SC, Tonkonogy SL, Albright CA, Tsang J, Balish EJ, Braun J, et al. Variable phenotypes of enterocolitis in interleukin 10-deficient mice monoassociated with two different commensal bacteria. Gastroenterology (2005) 128(4):891–906. doi:10.1053/j.gastro.2005.02.009
108. Fite A, Macfarlane S, Furrie E, Bahrami B, Cummings JH, Steinke DT, et al. Longitudinal analyses of gut mucosal microbiotas in ulcerative colitis in relation to patient age and disease severity and duration. J Clin Microbiol (2013) 51(3):849–56. doi:10.1128/JCM.02574-12
109. Furrie E, Macfarlane S, Cummings JH, Macfarlane GT. Systemic antibodies towards mucosal bacteria in ulcerative colitis and Crohn’s disease differentially activate the innate immune response. Gut (2004) 53(1):91–8. doi:10.1136/gut.53.1.91
110. Steck N, Hoffmann M, Sava IG, Kim SC, Hahne H, Tonkonogy SL, et al. Enterococcus faecalis metalloprotease compromises epithelial barrier and contributes to intestinal inflammation. Gastroenterology (2011) 141(3):959–71. doi:10.1053/j.gastro.2011.05.035
111. Ocvirk S, Sava IG, Lengfelder I, Lagkouvardos I, Steck N, Roh JH, et al. Surface-associated lipoproteins link Enterococcus faecalis virulence to colitogenic activity in IL-10-deficient mice independent of their expression levels. PLoS Pathog (2015) 11(6):e1004911. doi:10.1371/journal.ppat.1004911
112. Waidmann M, Bechtold O, Frick JS, Lehr HA, Schubert S, Dobrindt U, et al. Bacteroides vulgatus protects against Escherichia coli-induced colitis in gnotobiotic interleukin-2-deficient mice. Gastroenterology (2003) 125(1):162–77. doi:10.1016/S0016-5085(03)00672-3
113. Rath HC, Wilson KH, Sartor RB. Differential induction of colitis and gastritis in HLA-B27 transgenic rats selectively colonized with Bacteroides vulgatus or Escherichia coli. Infect Immun (1999) 67(6):2969–74.
114. Mohamadzadeh M, Klaenhammer TR. Specific Lactobacillus species differentially activate toll-like receptors and downstream signals in dendritic cells. Expert Rev Vaccines (2008) 7(8):1155–64. doi:10.1586/14760584.7.8.1155
115. Ouwehand AC, Salminen S, Isolauri E. Probiotics: an overview of beneficial effects. Antonie Van Leeuwenhoek (2002) 82(1–4):279–89. doi:10.1023/A:1020620607611
116. Cario E, Gerken G, Podolsky DK. Toll-like receptor 2 controls mucosal inflammation by regulating epithelial barrier function. Gastroenterology (2007) 132(4):1359–74. doi:10.1053/j.gastro.2007.02.056
117. Cario E, Gerken G, Podolsky DK. Toll-like receptor 2 enhances ZO-1-associated intestinal epithelial barrier integrity via protein kinase C. Gastroenterology (2004) 127(1):224–38. doi:10.1053/j.gastro.2004.04.015
118. Boulard O, Asquith MJ, Powrie F, Maloy KJ. TLR2-independent induction and regulation of chronic intestinal inflammation. Eur J Immunol (2010) 40(2):516–24. doi:10.1002/eji.200939669
119. Kalina WV, Mohamadzadeh M. Lactobacilli as natural enhancer of cellular immune response. Discov Med (2005) 5(26):199–203.
120. Mohamadzadeh M, Duong T, Sandwick SJ, Hoover T, Klaenhammer TR. Dendritic cell targeting of Bacillus anthracis protective antigen expressed by Lactobacillus acidophilus protects mice from lethal challenge. Proc Natl Acad Sci U S A (2009) 106(11):4331–6. doi:10.1073/pnas.0900029106
121. Konstantinov SR, Smidt H, de Vos WM, Bruijns SC, Singh SK, Valence F, et al. S layer protein A of Lactobacillus acidophilus NCFM regulates immature dendritic cell and T cell functions. Proc Natl Acad Sci U S A (2008) 105(49):19474–9. doi:10.1073/pnas.0810305105
122. Mohamadzadeh M, Pfeiler EA, Brown JB, Zadeh M, Gramarossa M, Managlia E, et al. Regulation of induced colonic inflammation by Lactobacillus acidophilus deficient in lipoteichoic acid. Proc Natl Acad Sci U S A (2011) 108(Suppl 1):4623–30. doi:10.1073/pnas.1005066107
123. Grangette C, Nutten S, Palumbo E, Morath S, Hermann C, Dewulf J, et al. Enhanced anti inflammatory capacity of a Lactobacillus plantarum mutant synthesizing modified teichoic acids. Proc Natl Acad Sci U S A (2005) 102(29):10321–6. doi:10.1073/pnas.0504084102
124. Claes IJ, Lebeer S, Shen C, Verhoeven TL, Dilissen E, De Hertogh G, et al. Impact of lipoteichoic acid modification on the performance of the probiotic Lactobacillus rhamnosus GG in experimental colitis. Clin Exp Immunol (2010) 162(2):306–14. doi:10.1111/j.1365-2249.2010.04228.x
125. Miele E, Pascarella F, Giannetti E, Quaglietta L, Baldassano RN, Staiano A, et al. Effect of a probiotic preparation (VSL# 3) on induction and maintenance of remission in children with ulcerative colitis. Am J Gastroenterol (2009) 104(2):437–43. doi:10.1038/ajg.2008.118
126. Sood A, Midha V, Makharia GK, Ahuja V, Singal D, Goswami P, et al. The probiotic preparation, VSL#3 induces remission in patients with mild-to-moderately active ulcerative colitis. Clin Gastroenterol Hepatol (2009) 7(11):1202–9. doi:10.1016/j.cgh.2009.07.016
127. Tursi A, Brandimarte G, Papa A, Giglio A, Elisei W, Giorgetti GM, et al. Treatment of relapsing mild-to-moderate ulcerative colitis with the probiotic VSL#3 as adjunctive to a standard pharmaceutical treatment: a double-blind, randomized, placebo-controlled study. Am J Gastroenterol (2010) 105(10):2218–27. doi:10.1038/ajg.2010.218
128. Hoermannsperger G, Clavel T, Hoffmann M, Reiff C, Kelly D, Loh G, et al. Post-translational inhibition of IP-10 secretion in IEC by probiotic bacteria: impact on chronic inflammation. PLoS One (2009) 4(2):e4365. doi:10.1371/journal.pone.0004365
129. von Schillde MA, Hörmannsperger G, Weiher M, Alpert CA, Hahne H, Bäuerl C, et al. Lactocepin secreted by Lactobacillus exerts anti-inflammatory effects by selectively degrading proinflammatory chemokines. Cell Host Microbe (2012) 11(4):387–96. doi:10.1016/j.chom.2012.02.006
130. Siegel R, Desantis C, Jemal A. Colorectal cancer statistics, 2014. CA Cancer J Clin (2014) 64(2):104–17. doi:10.3322/caac.21220
131. Uronis JM, Mühlbauer M, Herfarth HH, Rubinas TC, Jones GS, Jobin C. Modulation of the intestinal microbiota alters colitis-associated colorectal cancer susceptibility. PLoS One (2009) 4(6):e6026. doi:10.1371/journal.pone.0006026
132. Chu FF, Esworthy RS, Chu PG, Longmate JA, Huycke MM, Wilczynski S, et al. Bacteria-induced intestinal cancer in mice with disrupted Gpx1 and Gpx2 genes. Cancer Res (2004) 64(3):962–8. doi:10.1158/0008-5472.CAN-03-2272
133. Li Y, Kundu P, Seow SW, de Matos CT, Aronsson L, Chin KC, et al. Gut microbiota accelerate tumor growth via c-jun and STAT3 phosphorylation in APCMin/+ mice. Carcinogenesis (2012) 33(6):1231–8. doi:10.1093/carcin/bgs137
134. Zackular JP, Baxter NT, Iverson KD, Sadler WD, Petrosino JF, Chen GY, et al. The gut microbiome modulates colon tumorigenesis. MBio (2013) 4(6):e00692–13. doi:10.1128/mBio.00692-13
135. Wu S, Rhee KJ, Albesiano E, Rabizadeh S, Wu X, Yen HR, et al. A human colonic commensal promotes colon tumorigenesis via activation of T helper type 17 T cell responses. Nat Med (2009) 15(9):1016–22. doi:10.1038/nm.2015
136. Su LK, Kinzler KW, Vogelstein B, Preisinger AC, Moser AR, Luongo C, et al. Multiple intestinal neoplasia caused by a mutation in the murine homolog of the APC gene. Science (1992) 256(5057):668–70. doi:10.1126/science.256.5060.1114-c
137. Lebeer S, Claes IJ, Verhoeven TL, Vanderleyden J, De Keersmaecker SC. Exopolysaccharides of Lactobacillus rhamnosus GG form a protective shield against innate immune factors in the intestine. Microb Biotechnol (2011) 4(3):368–74. doi:10.1111/j.1751-7915.2010.00199.x
138. Ellmerich S, Djouder N, Schöller M, Klein JP. Production of cytokines by monocytes, epithelial and endothelial cells activated by Streptococcus bovis. Cytokine (2000) 12(1):26–31. doi:10.1006/cyto.1999.0521
139. Yu J, Feng Q, Wong SH, Zhang D, Liang QY, Qin Y, et al. Metagenomic analysis of faecal microbiome as a tool towards targeted non-invasive biomarkers for colorectal cancer. Gut (2017) 66(1):70–8. doi:10.1136/gutjnl-2015-309800
140. Nakatsu G, Li X, Zhou H, Sheng J, Wong SH, Wu WK, et al. Gut mucosal microbiome across stages of colorectal carcinogenesis. Nat Commun (2015) 6:8727. doi:10.1038/ncomms9727
141. Arthur JC, Perez-Chanona E, Mühlbauer M, Tomkovich S, Uronis JM, Fan TJ, et al. Intestinal inflammation targets cancer-inducing activity of the microbiota. Science (2012) 338(6103):120–3. doi:10.1126/science.1224820
142. Tsoi H, Chu ESH, Zhang X, Sheng J, Nakatsu G, Ng SC, et al. Peptostreptococcus anaerobius induces intracellular cholesterol biosynthesis in colon cells to induce proliferation and causes dysplasia in mice. Gastroenterology (2017) 152(6):1419–33.e5. doi:10.1053/j.gastro.2017.01.009
143. Fearnhead NS, Britton MP, Bodmer WF. The ABC of APC. Hum Mol Genet (2001) 10(7):721–33. doi:10.1093/hmg/10.7.721
144. Phelps RA, Chidester S, Dehghanizadeh S, Phelps J, Sandoval IT, Rai K, et al. A two-step model for colon adenoma initiation and progression caused by APC loss. Cell (2009) 137(4):623–34. doi:10.1016/j.cell.2009.02.037
145. Fukata M, Chen A, Vamadevan AS, Cohen J, Breglio K, Krishnareddy S, et al. Toll-like receptor-4 promotes the development of colitis-associated colorectal tumors. Gastroenterology (2007) 133(6):1869–81. doi:10.1053/j.gastro.2007.09.008
146. Huang B, Zhao J, Li H, He KL, Chen Y, Chen SH, et al. Toll-like receptors on tumor cells facilitate evasion of immune surveillance. Cancer Res (2005) 65(12):5009–14. doi:10.1158/0008-5472.CAN-05-0784
147. Sun Q, Liu Q, Zheng Y, Cao X. Rapamycin suppresses TLR4-triggered IL-6 and PGE(2) production of colon cancer cells by inhibiting TLR4 expression and NF-kappaB activation. Mol Immunol (2008) 45(10):2929–36. doi:10.1016/j.molimm.2008.01.025
148. Wang Y, Devkota S, Musch MW, Jabri B, Nagler C, Antonopoulos DA, et al. Regional mucosa-associated microbiota determine physiological expression of TLR2 and TLR4 in murine colon. PLoS One (2010) 5(10):e13607. doi:10.1371/journal.pone.0013607
149. Wang EL, Qian ZR, Nakasono M, Tanahashi T, Yoshimoto K, Bando Y, et al. High expression of toll-like receptor 4/myeloid differentiation factor 88 signals correlates with poor prognosis in colorectal cancer. Br J Cancer (2010) 102(5):908–15. doi:10.1038/sj.bjc.6605558
150. Candela M, Turroni S, Biagi E, Carbonero F, Rampelli S, Fiorentini C, et al. Inflammation and colorectal cancer, when microbiota-host mutualism breaks. World J Gastroenterol (2014) 20(4):908–22. doi:10.3748/wjg.v20.i4.908
151. Buc E, Dubois D, Sauvanet P, Raisch J, Delmas J, Darfeuille-Michaud A, et al. High prevalence of mucosa-associated E. coli producing cyclomodulin and genotoxin in colon cancer. PLoS One (2013) 8(2):e56964. doi:10.1371/journal.pone.0056964
152. Cougnoux A, Delmas J, Gibold L, Faïs T, Romagnoli C, Robin F, et al. Small-molecule inhibitors prevent the genotoxic and protumoural effects induced by colibactin-producing bacteria. Gut (2016) 65(2):278–85. doi:10.1136/gutjnl-2014-307241
153. Forbes NS. Engineering the perfect (bacterial) cancer therapy. Nat Rev Cancer (2010) 10(11):785–94. doi:10.1038/nrc2934
154. Zheng JH, Nguyen VH, Jiang SN, Park SH, Tan W, Hong SH, et al. Two-step enhanced cancer immunotherapy with engineered Salmonella typhimurium secreting heterologous flagellin. Sci Transl Med (2017) 9(376):eaak9537. doi:10.1126/scitranslmed.aak9537
155. Sahu U, Choudhury A, Parvez S, Biswas S, Kar S. Induction of intestinal stemness and tumorigenicity by aberrant internalization of commensal non-pathogenic E. coli. Cell Death Dis (2017) 8:e2667. doi:10.1038/cddis.2017.27
156. Khazaie K, Zadeh M, Khan MW, Bere P, Gounari F, Dennis K, et al. Abating colon cancer polyposis by Lactobacillus acidophilus deficient in lipoteichoic acid. Proc Natl Acad Sci U S A (2012) 109(26):10462–7. doi:10.1073/pnas.1207230109
157. Zeller G, Tap J, Voigt AY, Sunagawa S, Kultima JR, Costea PI, et al. Potential of fecal microbiota for early-stage detection of colorectal cancer. Mol Syst Biol (2014) 10:766. doi:10.15252/msb.20145645
158. Levy SB, Marshall B. Antibacterial resistance worldwide: causes, challenges and responses. Nat Med (2004) 10(12 Suppl):S122–9. doi:10.1038/nm1145
Keywords: intestinal epithelium, intestinal microbiota, bacterial signaling, inflammation, colorectal cancer
Citation: Coleman OI and Haller D (2018) Bacterial Signaling at the Intestinal Epithelial Interface in Inflammation and Cancer. Front. Immunol. 8:1927. doi: 10.3389/fimmu.2017.01927
Received: 11 July 2017; Accepted: 15 December 2017;
Published: 05 January 2018
Edited by:
Christoph Becker, University of Erlangen-Nuremberg, GermanyReviewed by:
Luca Pastorelli, Università degli Studi di Milano, ItalyMathias W. Hornef, Hannover Medical School, Germany
Copyright: © 2018 Coleman and Haller. This is an open-access article distributed under the terms of the Creative Commons Attribution License (CC BY). The use, distribution or reproduction in other forums is permitted, provided the original author(s) or licensor are credited and that the original publication in this journal is cited, in accordance with accepted academic practice. No use, distribution or reproduction is permitted which does not comply with these terms.
*Correspondence: Dirk Haller, ZGlyay5oYWxsZXJAdHVtLmRl