- Faculty of Sciences, Department of Biochemistry, University of Geneva, Geneva, Switzerland
The soil-dwelling social amoeba Dictyostelium discoideum feeds on bacteria. Each meal is a potential infection because some bacteria have evolved mechanisms to resist predation. To survive such a hostile environment, D. discoideum has in turn evolved efficient antimicrobial responses that are intertwined with phagocytosis and autophagy, its nutrient acquisition pathways. The core machinery and antimicrobial functions of these pathways are conserved in the mononuclear phagocytes of mammals, which mediate the initial, innate-immune response to infection. In this review, we discuss the advantages and relevance of D. discoideum as a model phagocyte to study cell-autonomous defenses. We cover the antimicrobial functions of phagocytosis and autophagy and describe the processes that create a microbicidal phagosome: acidification and delivery of lytic enzymes, generation of reactive oxygen species, and the regulation of Zn2+, Cu2+, and Fe2+ availability. High concentrations of metals poison microbes while metal sequestration inhibits their metabolic activity. We also describe microbial interference with these defenses and highlight observations made first in D. discoideum. Finally, we discuss galectins, TNF receptor-associated factors, tripartite motif-containing proteins, and signal transducers and activators of transcription, microbial restriction factors initially characterized in mammalian phagocytes that have either homologs or functional analogs in D. discoideum.
Introduction
The mononuclear phagocyte system (MPS), comprising monocytes, macrophages, and dendritic cells, is the first line of defense against infection. MPS cells monitor blood and tissue for the presence of microbes and respond to conserved molecules produced by and/or to damage caused by potential pathogens. These signals trigger innate-immune pathways that activate cell-autonomous defense mechanisms and secretion of intercellular signals that orchestrate inflammatory and subsequent adaptive immune responses.
Cell-autonomous defense mechanisms, which exist in MPS cells and also non-immune cells, provide a rapid antimicrobial response and evolved from the predator–prey relationship among the ancestors of current eukaryotes and prokaryotes (1, 2). In MPS cells, cell-autonomous defenses include phagocytosis and autophagy, processes that originated in ancient eukaryotes for nutrient acquisition and reallocation. Indeed, the basic components of these pathways are conserved in immune cells of all metazoa and in bacterivorous and fungivorous single-celled eukaryotes such as amoebae. Microbial mechanisms to subvert cell-autonomous defenses predate metazoa and likely originated as resistance to predation by single-celled eukaryotes. Given this evolutionary context, amoebae are relevant surrogate phagocytes for the study of the conserved innate-immune responses of MPS cells and of microbial mechanisms to resist killing. Although the infection of amoebae cannot completely recapitulate the complexities of host–pathogen interactions in metazoa, the innate defenses of MPS cells establish the critical initial immune response to microbes, and MPS cells are often the physical interface of host and intracellular pathogens. Valuable insight can thus be gained by studying the interactions between microbes and amoebae that rely solely on cell-autonomous defenses for survival.
In this review, we focus on the use of the soil dwelling, social amoeba Dictyostelium discoideum as a model phagocyte to study the interactions between intracellular pathogens and cell-autonomous defense mechanisms of MPS cells. We cover phagocytosis with an emphasis on phagosome maturation and the mechanisms used to create an antimicrobial environment within the phagosome. These mechanisms include the delivery of lytic enzymes, the generation of reactive oxygen species (ROS), and the manipulation of the concentrations of divalent metals to either poison microbes or inhibit their metabolic activity. We describe autophagy as a defense pathway that responds when phagocytosis is insufficient to eliminate infection. We also highlight microbial interference with these defense responses first elucidated using D. discoideum. Finally, we discuss microbial restriction factors, initially characterized in MPS cells, that have either sequence homologs or potentially functional analogs in D. discoideum. Determining their contribution to cell-autonomous defense mechanisms in this amoeba will further strengthen its usefulness as a model for host–pathogen interactions.
Predation Resistance, Virulence, and Cell-Autonomous Defenses
Given the complex interactions between pathogenic microorganisms and their metazoan hosts, it is difficult to pinpoint the origins of microbial virulence. How would a naïve bacterium encountering an elaborate immune response for the first time persist long enough to evolve mechanisms to counteract antimicrobial defenses? On the host side, why are such extensive immune responses in place? When did it all begin? An emerging concept in host–pathogen interactions is that microbial virulence evolved from selective forces in the environment such as the pressure to resist predation by amoebae and other protozoa (3–6).
In addition to competing for nutrients and adapting to variations in environmental conditions such as temperature and moisture, successful microbes avoid being a meal for predatory protozoa. These resistance mechanisms include avoidance of phagocytosis, e.g., masking of the microbial surface and biofilm formation, avoidance of digestion, e.g., inhibition of phagosome maturation, escape from the phagosome, the killing of the predator by toxin secretion pre- or post-phagocytosis, and the use of specialized secretion systems (3). On the predator side, the advent of resistance in turn selected for more robust strategies to kill bacteria and/or counteract this resistance. This ancient fight for survival among single-celled species with short generation times and large population sizes provided the context for the evolution of virulence and cell-autonomous defenses. As metazoa evolved and gained complexity, these defenses were conserved and also expanded (7, 8). Concurrently, some microbes evolved mechanisms not just to avoid killing but to survive inside hosts and exploit them for resources.
The coevolution of host and microbes beginning at the single-celled stage provides a plausible explanation for the conservation of cell-autonomous defenses. It is important to remember that single-celled eukaryotic predators continued to evolve and that successful cell-autonomous defense strategies are present in extant species such as D. discoideum. Bacteria must therefore contend with similar defense mechanisms in macrophages and amoebae; consequently, predation selects for bacterial survival strategies that are likely to resist killing by MPS cells (9–12). Another consequence of this conservation is that amoebae can select for and act as reservoirs of microbes that can infect humans, including Mycobacterium species, Legionella pneumophila, and Vibrio cholera (13–15). This evolutionary history makes amoebae ideal, relevant model phagocytes to study host–pathogen interactions (16–19).
Dictyostelium discoideum
Dictyostelium discoideum belongs to the Amoebozoa phylum, which diverged from the Opisthokonts, the phylum to which the animals belong, after sharing a common ancestor with plants. During its growth phase, the amoeba replicates by binary fission and employs phagocytosis to kill and extract nutrients from bacteria in the soil. Upon nutrient depletion, starvation induces amoebae to undergo a developmental cycle in which approximately 100,000 cells aggregate by chemotaxing toward cyclic AMP, differentiate into multiple cell types, and transition through several multicellular stages to ultimately produce the fruiting body that comprises a spore-containing sorus resting upon a stalk of dead cells (Figure 1) (20–22). Depending on environmental conditions, the early multicellular stage continues to develop in place or transitions to a motile slug that migrates away from ammonia and toward heat, light, and oxygen before fruiting body culmination (20–25). When development is initiated underground these cues guide the slug to the soil surface, where spore dispersion is more likely.
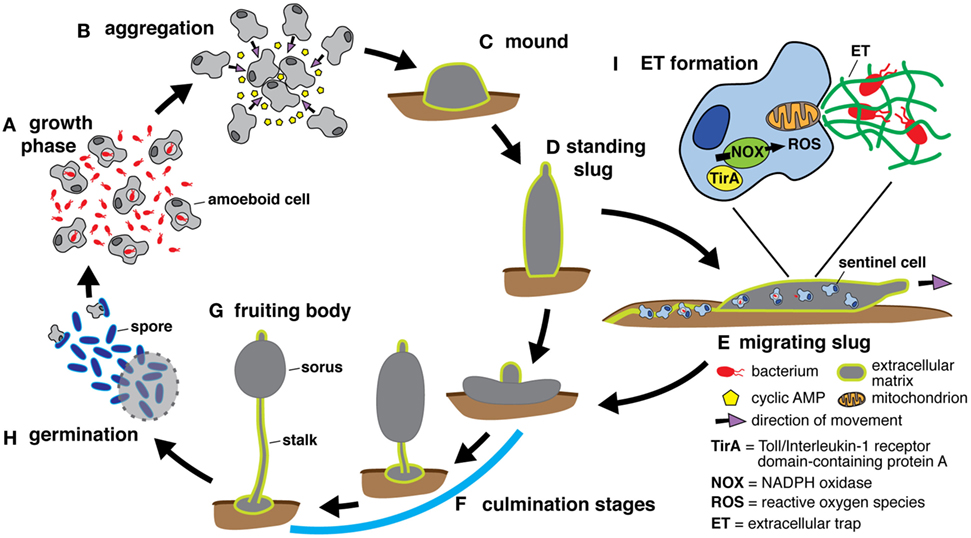
Figure 1. The Dictyostelium discoideum life cycle includes multicellular stages. (A) During the growth phase of development, amoeboid cells feed on bacteria and replicate by binary fission. The development cycle is initiated upon resource depletion, and aggregation occurs when starving cells secrete cyclic AMP to recruit additional cells (B). The aggregating cells organize to form the mound stage enclosed within an extracellular matrix composed of cellulose and mucopolysaccharide (26) (C) and continue to develop into the standing slug (D). Depending on its environment, the standing slug either falls over to become a migrating slug that moves toward heat and light (E) or proceeds directly to the culmination stages (F) that ultimately produce the fruiting body, which consists of a spore-containing structure, the sorus, held aloft by a stalk of dead cells (G). Spores are released from the sorus and germinate into growing cells (H). Under optimal conditions, the developmental cycle takes around 24 h. If the slug forms underground, it migrates toward the surface to maximize spore dissemination. To protect itself from infection during migration, the slug possesses a rudimentary immune system comprising phagocytic sentinel cells. These cells move throughout the slug, take up bacteria and toxins, and are shed along with extracellular matrix as the slug moves (E). In response to bacteria, sentinel cells release extracellular traps, derived from mitochondrial DNA, via an unknown mechanism involving NADPH oxidase (NOX)-generated reactive oxygen species (ROS) and TirA, a soluble protein containing a toll/interleukin 1 receptor domain (I).
Due to its multicellular cycle, D. discoideum, referred to as a social amoeba, is used as a model to study aspects of development including intercellular signaling (27) [reviewed in Ref. (22)], quorum sensing (28), cell–cell recognition (29), cell fate determination (30), tissue patterning (31), and even societal concepts such as cooperativity and altruism (32). It is also an established model for basic cell biological processes, including phagocytosis (33), macropinocytosis (34), chemotaxis (35), autophagy (36), and oxygen sensing (37), and the functions of proteins implicated in human diseases including Alzheimer’s and Parkinson’s (38–41). Indeed, this amoeba has proven to be a versatile model organism and is gaining traction as an attractive alternative to animal models (42, 43).
Dictyostelium discoideum has been used as a host cell for L. pneumophila (44–47), Mycobacterium species (48–51), V. cholera (17), Francisella noatunensis (52, 53), Pseudomonas aeruginosa (16), Salmonella enterica (54), and other intracellular pathogens (55). Moreover, its genome encodes numerous homologs of proteins and protein domains involved in sensing and responding to microbes by macrophages (5) (http://dictybase.org). The conservation of macropinocytosis, chemotaxis, phagocytosis, and autophagy pathways in D. discoideum make it a model MPS cell. Here we focus on phagocytosis and autophagy within the context of cell-autonomous defenses. Macropinocytosis and chemotaxis are beyond the scope of this review and have been covered extensively elsewhere [for reviews on chemotaxis (56, 57); for reviews on macropinocytosis (58, 59)].
From a practical standpoint, its amenability to experimentation also makes D. discoideum an ideal model organism. It is easily cultivatable and can be grown axenically in liquid media, which enables analysis of mutant strains defective for growth on bacteria. Cultures can be readily scaled up for biochemical and cell biological techniques (43) as well as high-throughput genetic and drug discovery screens (60–62). It is also well suited for microscopy including live-cell imaging (63). An extensive molecular genetic toolkit has been developed for the generation of mutants and ectopic gene expression (64). The haploid genome of multiple strains and closely related species have been sequenced (65–68), and numerous RNAseq and transcriptomic analyses have been performed (69, 70). The community’s online resource, dictyBase, provides a central location to access sequence data, techniques, and available mutants and plasmids (71) (http://dictybase.org). Relevant its use as a model phagocyte, there are established protocols for infecting D. discoideum with various bacterial pathogens (63, 72, 73) and for monitoring and quantifying autophagy (74).
Phagocytosis
Phagocytosis is the process that allows engulfment of particles larger than 200 nm and is used by MPS cells to ingest and kill pathogens as well as to activate the adaptive immune response through antigen presentation. The phagocytosis maturation pathway is highly conserved between MPS cells and D. discoideum, which uses the process for feeding (8). In a simplified view, the particle to ingest is recognized by surface receptors, and this interaction triggers a signaling cascade that stimulates polymerization of actin to deform the membrane around the particle. After closure of the phagocytic cup, the newly formed phagosome undergoes maturation, a series of steps necessary to render the phagosome a highly acidic, degradative and oxidative compartment (Figure 2). Many pathogens, such as L. pneumophila, S. enterica, and Mycobacterium spp., have evolved ways to escape the phagosome or subvert its maturation to replicate within the host cell. In this section, we will briefly summarize the phagocytosis maturation steps in D. discoideum and how this model phagocyte has been used to extend our knowledge of several infectious diseases. It should be noted that studies using D. discoideum as a model phagocyte have mainly been performed with axenic laboratory strains. These strains are able to grow in the absence of bacteria due to a null mutation in the gene encoding the Ras-regulating neurofibromin, which results in enlarged macropinosomes that facilitate uptake of sufficient nutrients from liquid media to support growth and also enables the mutants to phagocytose larger particles than wild-type strains (75). Effects of this mutation on phagosome maturation have not been reported.
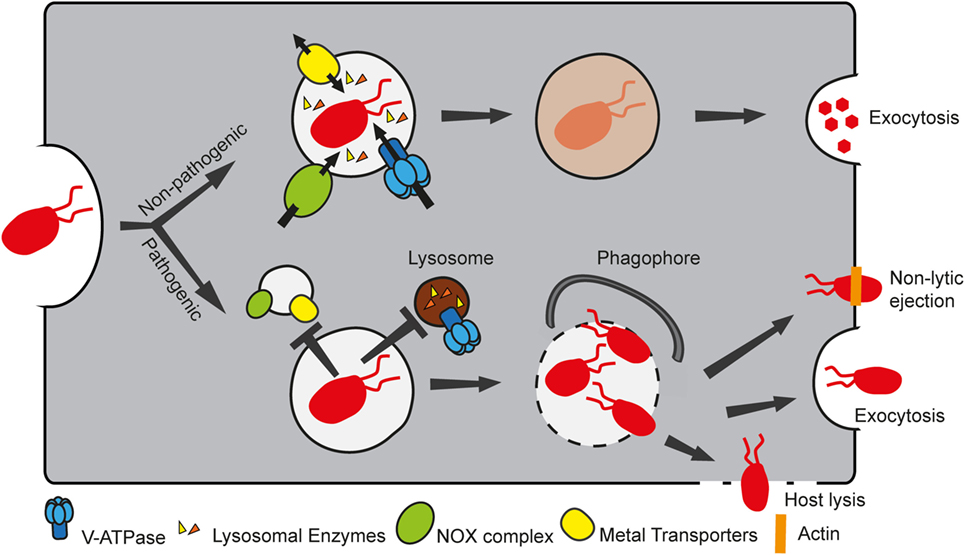
Figure 2. Non-pathogenic and pathogenic bacteria follow different fates in Dictyostelium discoideum. D. discoideum takes up bacteria by phagocytosis. Non-pathogenic food bacteria follow the normal phagosomal maturation pathway, whereby the phagosome acquires several components, including the vacuolar ATPase (V-ATPase), lysosomal enzymes, the NADPH oxidase (NOX) complex, and several metal transporters to create a microbicidal compartment that digests and kills bacteria. Intracellular pathogens, however, are able to manipulate the maturation program, by preventing the phagosome from becoming bactericidal, thus ensuring proliferation in a “friendly” compartment. In addition, certain pathogens can eventually escape the compartment. In this case, they can either be recaptured by autophagy, or exit the host cell by exocytosis, or by lytic or non-lytic processes.
Particle Recognition and Phagocytosis Initiation
Innate-immune cells can recognize several pathogen-associated molecular patterns (PAMPs) secreted or present at the bacterial cell wall via specific pathogen recognition receptors (PRRs). In mammals, these include toll-like receptors (TLRs), integrins, scavenger receptors, and C-type lectins (76). TLRs monitor the cell surface and endocytic compartments and activate cell-autonomous defenses upon detection of PAMPs, while lectins, scavenger receptors, and integrins function as phagocytic receptors that bind to particles and are able to trigger uptake even in non-phagocytic cells (76). TLRs contain ligand-binding leucine-rich repeats (LRRs) in the luminal/extracellular domains and a toll/interleukin 1 receptor (TIR) domain in the cytoplasmic tail that mediates protein–protein interactions. D. discoideum does not have TLRs, but two cytosolic proteins with TIR domains, TirA and TirB, have been identified. Depletion of TirA inhibits growth on a laboratory strain of Klebsiella used as a food bacterium, but not in media (77). How TirA is involved in sensing and/or induction of phagocytic uptake remains to be studied. The D. discoideum genome encodes >150 LRR-containing proteins, but whether they function as PRRs remains to be determined (5).
In D. discoideum, only a few phagocytic receptors have been molecularly identified so far (5). The most studied are the integrin-like Sib (similar to integrin-β) family of proteins, comprising five members (SibA-E). Like human integrins, they contain a von Willebrand factor type A and a glycine-rich transmembrane domain and can interact with the actin-binding protein talin (78). Two members of the family, SibA and SibC, are directly involved in adhesion to substrate and to particles (78). Phg1a, a member of the TM9 family, and SadA were previously identified as phagocytic receptors (79, 80); however, recent findings show that these two proteins are instead involved in regulating surface levels of SibA (81).
Lectins and scavenger receptors might also function as phagocytic receptors in D. discoideum. Three homologs of scavenger receptor class B proteins in mammals, LmpA, LmpB, and LmpC, are present in D. discoideum. LmpB is found on lipid rafts at the plasma membrane and in early phagocytic compartments and may function as a phagocytic receptor (82–84). It is thought that D. discoideum also possesses lectin-like receptors, as it was shown to be able to bind specifically to certain sugar derivatives (85, 86).
A well-studied chemoattractant and phagocytosis stimulator for D. discoideum is folate, a metabolite secreted by certain bacteria. Recently, Pan and colleagues identified fAR1, a G-protein coupled receptor for folic acid involved in signaling and initiation of uptake but not binding of bacteria (87). Presumably, the cytoskeletal rearrangements downstream of fAR1 that facilitate chemotaxis can also initiate phagocytosis when a burst of fAR1 activation occurs around a bacterium that is a concentrated source of folate. D. discoideum sensing of bacterial capsule independent of folate has also been described but not further characterized (88).
Actin Dynamics and Phosphatidylinositol Phosphates (PIPs)
After binding of ligands to their receptors, heterotrimeric G proteins are involved in downstream signaling to initiate phagocytosis through F-actin rearrangements. In D. discoideum, the G4αGβγ complex has been proposed to be activated by the fAR1 folate receptor, was shown to be implicated in particle uptake, and is therefore the most likely candidate involved in signaling from the phagocytic receptors to drive actin polymerization (87, 89). F-actin rearrangements occur at the uptake site to drive formation of pseudopods and the phagocytic cup. F-actin polymerization at the uptake site is driven by the Arp2/3 complex, an actin nucleation factor, and its activator, the SCAR/WAVE complex, in both macrophages and D. discoideum (90, 91). In addition, several other actin-binding proteins, such as profilins, cofilins, and Abp1, are present during the formation of the phagocytic cup [(92, 93), for a more detailed review on the phagocytic process in D. discoideum, see Ref. (94)]. Regulation of actin dynamics and subsequent trafficking events by Rho GTPases is also conserved in D. discoideum, with Rac1 homologs (RacA, B, C, and G) thought to be the main regulators of phagocytic uptake. Notably, Rac1 is involved in FcγR-mediated phagocytosis in macrophages [for a comprehensive review on Rho signaling, see Ref. (95)].
Phosphatidylinositol phosphates are important players during phagocytic uptake and maturation because they provide an identity to each compartment. PIP dynamics are well conserved between macrophages and D. discoideum. Briefly, phosphatidylinositol (4,5)-bisphosphate PI(4,5)P2 is the predominant PIP of the plasma membrane and is involved in recruiting and activating actin-binding proteins and nucleation-promoting factors. After receptor engagement, PI(4,5)P2 is phosphorylated into PI(3,4,5,)P3 by phosphatidylinositol 3-kinase and hydrolyzed into diacylglycerol and inositol (1,4,5)-trisphosphate, second messengers involved in calcium release and activation of further signaling cascades, by the phospholipase C kinase. Decrease of PI(4,5)P2 around the uptake site is necessary to then allow actin disassembly and closure of the phagocytic cup. In addition, closure of the phagocytic cup requires Dd5P4, the D. discoideum homolog of the phosphatase OCRL, which dephosphorylates PI(3,4,5)P3 into PI(3,4)P2 (96–98). Extensive recycling of plasma membrane components, including adhesion molecules, is a common feature shared by D. discoideum and macrophages in the early phases of phagosome formation, and in both organisms this step is regulated by the WASH complex, an Arp2/3 activator (99–101).
Phagosome Maturation
After closure of the phagocytic cup, the ingested particle is found in a closed compartment termed the phagosome. Extensive proteomic analyses as well as more recent live microscopy experiments have demonstrated the extraordinary plasticity of this organelle and the high degree of conservation of the phagosome maturation pathway between mammals and D. discoideum (8, 84). Phagosome maturation is a well-orchestrated series of events, which ensures killing and digestion of ingested bacteria (Figure 3). In a simplified view, Rab GTPases, notably Rab5 and Rab7, act as the masterminds of phagosome maturation by sequentially recruiting effectors involved in the various maturation steps [for an extensive review, see Ref. (102)]. In macrophages, Rab5 and its effectors are responsible for docking and fusion of endocytic compartments with the nascent phagosome and for acquisition of early phagosomal markers (103, 104). Subsequently, Rab7 ensures fusion with late-endosomal/lysosomal compartments and thus delivery of the lysosomal digestive content into the phagosome (104, 105). Rab GTPases are highly conserved at the protein sequence level between mammals and D. discoideum; indeed, this amoeba has homologs of most mammalian Rab GTPases that have been reported to be associated with phagosomes (102). D. discoideum Rab7 is recruited as early as 1–3 min after phagosome closure and regulates delivery of lysosomal proteins (106, 107). The localization and function of D. discoideum Rab5 have not been reported.
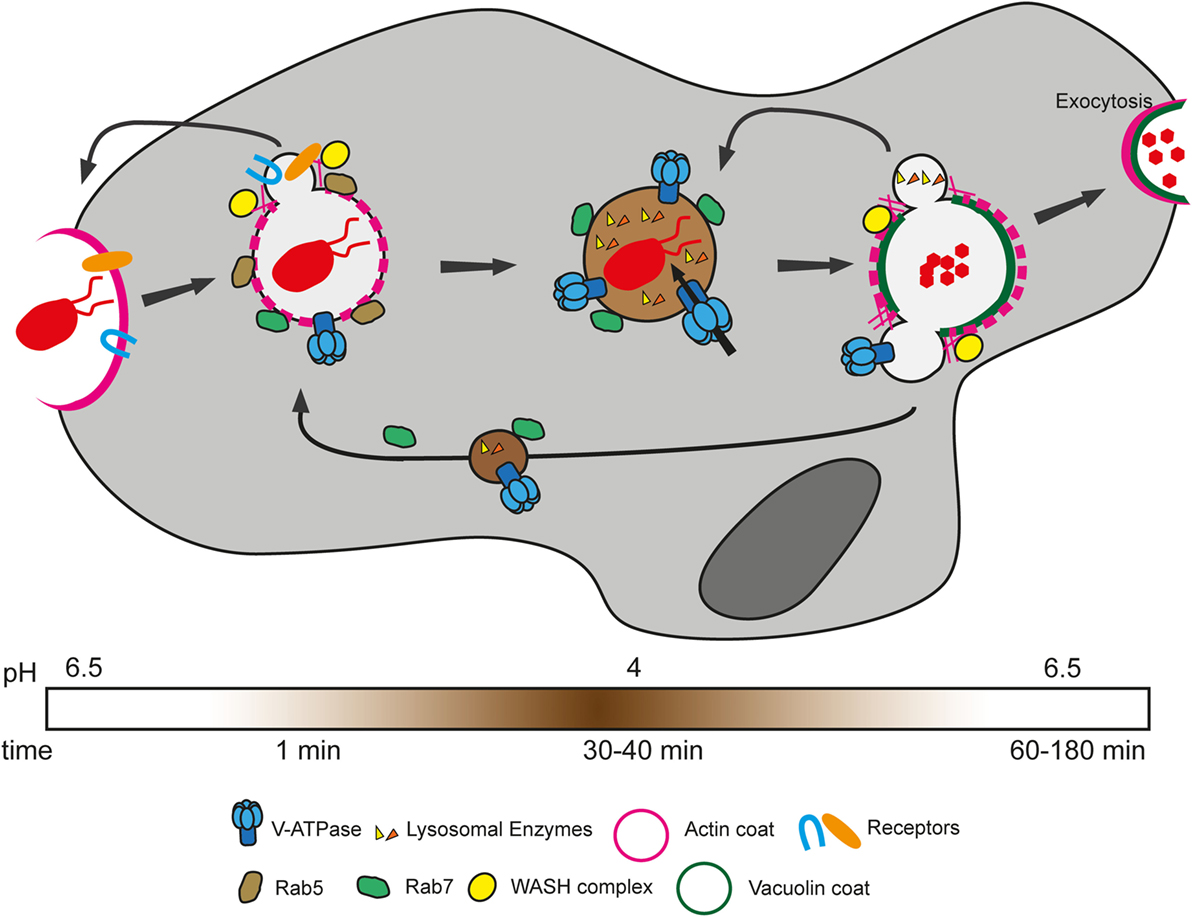
Figure 3. Phagosome maturation in Dictyostelium discoideum. Bacteria are recognized and sensed by various phagocytic and/or signaling receptors. This triggers signaling cascades that allow actin polymerization and deformation of the membrane to engulf the particle. After closure of the phagosome, bacteria are enclosed in an early phagosome, which gradually loses its actin coat and is characterized by the presence of Rab5. As early as 1 min after uptake, Rab7 is recruited to the phagosome, enabling fusion with lysosomes. Meanwhile, phagocytic receptors and plasma membrane proteins are recycled to the cell surface through actin polymerization induced by the WASH complex through Arp2/3 activation. The proton pump vacuolar ATPase (V-ATPase) is also acquired early in the maturation, ensuring rapid decrease of the luminal pH. Lysosomal enzymes, comprising proteases, are acquired in subsequent waves of delivery and function at low pH to degrade bacterial components. After about 40 min, the V-ATPase and lysosomal enzymes are recycled by the WASH complex in separate waves of recycling. Finally, non-digested bacterial remnants are expelled by exocytosis.
Recruited within minutes to the phagosome, the H+-vacuolar ATPase (V-ATPase) is the main agent of acidification and pumps protons inside the phagosome thanks to ATP hydrolysis (108). By creating a proton gradient, the V-ATPase is a crucial complex not only for the killing and digestion of bacteria but also for the progression of the maturation program. Notably, the proton gradient is necessary for proper delivery and activity of lysosomal enzymes, as well as for the function of ion transporters, involved in poisoning by or deprivation of metals (detailed below). The V-ATPase has been shown to be delivered by fusion with lysosomal vesicles or tubules in D. discoideum and macrophages, respectively (108–110). Rapid acidification of the compartment ensues, with the lowest pH reached between 10 and 30 min after phagosome formation, depending on the measuring method (93, 111). In contrast to macrophages, whose phagosomes were reported to reach a pH of 4.5–5, D. discoideum phagosomes are more acidic, with a pH as low as 3.5–4 (111–113).
Although unprocessed antigens have been shown to be regurgitated from late-endosomal compartments in dendritic cells to allow antigen uptake by other MPS cells (114, 115), in general, the acidic phagosome of MPS cells is thought of as a dead-end for ingested bacteria. In contrast, the acidic D. discoideum phagosome matures into a postlysosome. The V-ATPase and lysosomal enzymes were shown to be retrieved in several subsequent waves of recycling mediated by the WASH complex, which drives local actin polymerization (116, 117). The WASH complex is a nucleation-promoting factor necessary for the activation of the Arp2/3 complex and actin polymerization in both mammals and D. discoideum (99, 100, 117). During retrieval of the V-ATPase, the phagosome reaches its neutral pH, and progressively acquires the postlysosomal markers vacuolin A and B, homologs of the mammalian lipid raft-associated flotillins (118–120). The postlysosome is also characterized by the presence of an actin coat and the actin-binding protein coronin (118). This compartment then fuses with the plasma membrane in a mechanism akin to exocytosis to expel its non-digested materials. Interestingly, this process is reminiscent of exocytosis of secretory lysosomes in mammalian cytotoxic cells of the immune system (121). In mammals, lysosomes were shown to fuse with the plasma membrane upon increase of cytosolic Ca2+ concentration. In D. discoideum, Ca2+ was also shown to be involved in exocytosis, with mucolipin, a Ca2+ transporter, involved in regulating this process. Mucolipin is thought to pump Ca2+ inside postlysosomes, thereby inhibiting fusion with the plasma membrane by decreasing local Ca2+ concentration (122).
Microbial Manipulation of D. discoideum Phagocytosis
Certain intracellular bacterial pathogens are known for subverting phagosome maturation to prevent the formation of an unfriendly bactericidal compartment and to enable replication within the host cell. L. pneumophila, a Gram-negative bacterium that causes Legionnaire’s disease, is able to arrest early phagosomal maturation. In fact, the V-ATPase and other early endocytic markers are not delivered to the Legionella-containing vacuole (LCV) in either D. discoideum or macrophages (123). The endoplasmic reticulum (ER) is recruited in proximity to and fuses with the LCV, which becomes enriched in ER markers such as calnexin and calreticulin. This enrichment of ER markers is a consequence of a major strategy used by L. pneumophila to proliferate inside the host cell, which is termed identity theft and consists of changing the identity of its compartment to resemble the ER by recruiting different GTPases and PIPs (124, 125). For example, thanks to several bacterial effectors secreted through its type 4 secretion system, this pathogen is able to change the PIP composition of the phagosome by notably acquiring PI(4)P, a PIP normally associated with the trans-Golgi, ER, and plasma membrane (47). Other proteins involved in PIP dynamics and phagosome maturation, such as phosphatidylinositol 3-kinase and Dd5P4 have been shown to restrict L. pneumophila growth in D. discoideum (45, 46). D. discoideum has been used successfully to isolate and characterize the proteome of LCVs (44, 126). These studies have highlighted the importance of ER/Golgi small GTPases, such as Arf1 and Rab1, in gradually modifying the identity of the LCV. Rab8, a trans-Golgi-associated Rab GTPase, has also been detected at the LCV membrane and been shown to play a role in the association of SidC, a bacterial effector that mediates ER recruitment (44). Interestingly, Hoffmann and colleagues compared the LCV proteomes purified from murine macrophages and D. discoideum and uncovered that, if only considering proteins with conserved roles in these two organisms, about 50% of LCV-associated proteins were found in both organisms (126). These include the aforementioned Arf1, Rab8, and Rab1, as well as proteins involved in lipid metabolism, suggesting that L. pneumophila uses similar mechanisms to replicate in D. discoideum and macrophages and further corroborating the case that D. discoideum is an excellent model to study L. pneumophila infection.
Like Legionella pneumophila, albeit with completely different strategies, Mycobacterium spp. manipulate the phagosome maturation pathway. In fact, Mycobacterium tuberculosis, the causative agent of tuberculosis, and Mycobacterium marinum, a closely related mycobacterium that infects frogs and fish, prevent acquisition of the V-ATPase in macrophages (127). This was confirmed in D. discoideum, where it was shown that M. marinum is able to prevent accumulation of the V-ATPase and of cathepsin D (48). Recently, it was proposed that the WASH complex plays a role in preventing association of the V-ATPase with the mycobacteria-containing vacuole (MCV) by inducing polymerization of actin around the MCV, which probably prevents fusion with acidic vesicles. This mechanism was first studied in D. discoideum, but further confirmed in human macrophages with both M. tuberculosis and M. marinum (128). Of note, the mechanism of escape of cytosolic M. marinum from the host cell, termed ejection, and of cell-to-cell spreading has been well characterized and studied using D. discoideum as a host model [(49); reviewed in Ref. (129, 130)]. Interestingly, D. discoideum has also been extensively used as a model phagocyte to screen for new mycobacterial virulence factors (131–134).
Microbicidal Phagosome
Dictyostelium discoideum and macrophages employ conserved strategies to kill bacteria. As discussed previously, the V-ATPase has a central role in phagosome acidification; however, a low pH is not sufficient per se to kill bacteria. In fact, the phagosome acquires a series of proteases, hydrolases, lysozymes, and antimicrobial peptides necessary to breakdown several bacterial components or disrupt membrane integrity. Moreover, microbes can be poisoned and killed by transport of certain metals or by the production of ROS inside the compartment. Furthermore, metals can be pumped out of the phagosome to prevent bacterial growth. These bacterial-control strategies will be described in this section.
Lysozymes and Lysosomal Enzymes
Lysozymes, glycosidases that digest the peptidoglycan layer present in the cell wall of bacteria, belong to the Aly family in D. discoideum (135). Upregulation of lysozyme expression differs depending on the bacteria used as food. D. discoideum grown on Gram-positive bacteria upregulate AlyA, AlyB, AlyC, and AlyD whereas growth on Gram-negative bacteria leads to an increase in AlyL expression (136).
Lysosomal enzymes comprise several classes of enzymes involved in hydrolysis of sugar groups, such as mannosidases, or peptide bonds, such as proteases, and have been involved extensively in resistance to certain pathogens as well as bacterial killing in MPS cells. For instance, cysteine and serine cathepsins have been implicated in resistance to and killing of Staphylococcus aureus in neutrophils and macrophages (137, 138). More recently, M. tuberculosis was shown to downregulate expression and inhibit activity of cysteine proteases in macrophages, thus ensuring its replication (139). Cathepsin D, an aspartic protease, was also involved in resistance to Listeria monocytogenes, an intracellular food-borne pathogen (140).
Two main classes of lysosomal enzymes, bearing different posttranslational modifications, have been characterized in D. discoideum. The first class includes α-mannosidase, β-glucosidase, and cathepsins and is modified with mannose-6-phosphomethyldiester and/or mannose-6-sulfate, also known as common antigen-1 (141, 142). The second class of enzymes contains an N-acetylglucosamine-1-phosphate and comprises cysteine proteases (143). These different classes of enzymes reside in different vesicles at the steady state level and are recruited to phagosomes in a sequential manner, with first a wave of cysteine proteases followed by enzymes bearing the mannose-6-sulfate modification (84, 144).
Although lysosomal enzymes appear to be involved in bacterial killing in D. discoideum as in macrophages, direct evidence is lacking. Deletion of the cathepsin D gene is not sufficient to abolish growth on the food bacterium Klebsiella (142). Several D. discoideum mutants impaired in lysosomal enzyme trafficking and/or activity have been characterized including strains lacking WshA, a subunit of the WASH complex involved in lysosomal enzyme recycling (117), LvsB, a protein involved in restricting heterotypic fusion of early endosomes with postlysosomal compartments (145), and TM9 protein A, which is involved in the sorting of glycosidases, cathepsins, and lysozymes (146). Interestingly, these mutants exhibit growth defects specific to certain subsets of bacterial species (117, 145–148). These data suggest that different classes of lysosomal enzymes might play redundant roles, that they are not the sole killing strategy employed by D. discoideum, and that specific mechanisms may be used depending on the encountered bacteria.
Reactive Oxygen Species
Reactive oxygen species are key components of cell-autonomous defenses of MPS cells and function as antimicrobial effectors (149) as well as signaling molecules that regulate NF-κB (150, 151), autophagy (152), cytokine secretion (153), inflammasome activation (154), and apoptosis (155). ROS are implicated in the regulation of pH within phagosomes and the production of antigenic peptides in dendritic cells (156–158). ROS have also been implicated in the regulation of cytoskeleton dynamics and chemotaxis (159, 160). The major source of ROS in MPS cells is the NADPH oxidase (NOX) 2. Depending on its localization, NOX2 generates superoxide by transferring an electron from cytosolic NADPH to O2 in either the extracellular space or the lumen of the phagosome. Subsequent reactions convert superoxide into additional ROS. Superoxide dismutase catalyzes its conversion to hydrogen peroxide, which in turn reacts with Fe2+ in the Fenton reaction to generate hydroxyl radicals or with Cl− to produce hypochlorous acid via myeloperoxidase (161–163).
NOX2 is a heterodimer comprising the transmembrane proteins gp91phox/Nox2, the catalytic subunit, and p22phox, the regulatory subunit. NOX2 activation occurs downstream of extracellular receptors including integrins and Fc receptors and is coupled with phagocytosis. Activation requires three additional subunits, p67phox/neutrophil cytosol factor (Ncf) 2, p40phox/Ncf4, and p47phox/Ncf1, which form a ternary complex in the cytosol that is recruited to membrane-localized NOX2 by the small GTPases Rac1 and 2 (161–163). Mutations in NOX2 subunits cause chronic granulomatous disease (CGD), a condition that makes patients susceptible to recurring bacterial and fungal infections and demonstrates the importance of the NOX2-generated oxidative burst in the immune response (164, 165). Mitochondrial ROS production activated downstream of TLR signaling also contributes to antimicrobial mechanisms (166, 167).
Because ROS can damage host and microbe alike, ROS production and localization are tightly regulated, and MPS cells express ROS detoxifying enzymes such as superoxide dismutases (SODs), catalases, and peroxiredoxins to prevent self-damage. Microbes that persist inside the phagosome have mechanisms to minimize oxidative stress. These include expression of robust systems to maintain internal redox homeostasis (168), secretion of SODs and catalases to detoxify their compartment (149, 169), and deployment of effectors that inhibit NOX2 activation and/or delivery to the phagosome (170–173).
The D. discoideum genome encodes three catalytic NOX subunits: NoxA and NoxB, which are homologs of gp91phox/Nox2, and NoxC, which is a homolog of Nox5 (174–176). It also encodes one homolog of p22phox, CybA, and NcfA, a homolog of the cytosolic activating factor p67phox/Ncf2 (174, 175). RNAseq data indicate that NoxA, CybA, and NcfA are expressed during growth while NoxB and NoxC are mainly expressed during development (69, 174). However, long-term growth on Klebsiella can cause upregulation of NoxB in growing cells (136). Interestingly, D. discoideum expresses multiple SOD and catalase homologs (177–179) and exhibits a high resistance to oxidative stress (180), which suggests that it encounters internally and/or externally generated ROS regularly.
Whether ROS contribute to cell-autonomous defenses during the growth phase of D. discoideum is not clear. Mutants lacking noxA or both noxA and noxB exhibit no growth or killing defects when grown on Klebsiella (148, 174). This lack of a defect might be a consequence of redundant killing mechanisms or of challenge with a bacterium that is easily killed. Intriguingly, a D. discoideum mutant lacking the Xpf nuclease, a component of DNA damage repair machinery, accumulates more mutations when grown on a range of bacteria including Klebsiella than when grown axenically in media (181). One possible explanation is that Xpf is required to repair DNA damaged by ROS generated in response to bacteria.
Excessive ROS production in growing cells due to a deletion of a surface-localized SOD causes defects in chemotaxis and cell motility via sustained Ras activation (182, 183). The authors hypothesize that the chemotaxis defect prevents the inclusion of the cell in the multicellular stage and thus prevents propagation of the potentially mutagenized genome. It is tempting to speculate that excessive ROS production in response to a resistant microbe causes the same effect and inhibits the inclusion of an infected cell in the multicellular stages. Although the context is different, this “self-sacrifice” might be analogous to ROS-induced apoptosis of infected macrophages (155, 184).
Reactive oxygen species have functions during development. Extracellular ROS scavengers inhibit aggregation (185), and mutations in the individual NOX subunit genes, noxA, noxB, noxC, or cybA, or in the development stage-specific catalase gene, catB, cause defects in fruiting body formation when developed under axenic conditions (174, 186). These results indicate a signaling role for ROS. When developed after feeding on bacteria, a noxABC− triple mutant exhibits increased bacterial contamination of fruiting bodies compared with wild type (187). Thus, ROS also have an immunity function.
One possible cause of the immunity defect in the noxABC− mutant is the abrogation of DNA extracellular trap (ET) formation (Figure 1). The slug stage can persist for multiple days, during which it migrates through a dangerous melange of infectious bacteria and fungi that could decrease and/or prevent spore production. Phagocytic flux is limited in non-feeding developed cells (188). Protection from infection and intoxication appears to be mediated in part by a subpopulation of cells (<1%) that retain the capacity for phagocytosis (77). These so-called sentinel cells (S cells) are motile within the slug and phagocytose bacteria and toxins until they are eventually shed. Compared with the other cell types in slugs, S cells are enriched for tirA mRNA, and S cells from tirA− mutants have a decreased capacity to kill bacteria (77). In response to bacteria or LPS, S cells secrete mitochondrially derived ETs via a mechanism that requires both TirA and NOX-generated ROS, and increased contamination of fruiting bodies correlates with decreased ET production (187). Binding of the TLR2 TIR domain with Nox2 during M. tuberculosis infection of macrophages has been reported (189). How NOX and TirA fit into the pathway and whether they interact awaits further examination, as do the questions of whether S cells kill intracellular bacteria and, if so, whether TirA and NOX are involved. ET formation during the growth phase of the D. discoideum life cycle has not been observed.
First discovered in neutrophils and named neutrophil extracellular traps (NETs), ETs have been observed in numerous immune cell types including macrophages (190–193). ETs comprise antimicrobial peptides, proteases, and signaling molecules bound to a meshwork of DNA released from the nucleus or mitochondria [(190); reviewed in Ref. (194)]. The mechanisms by which ETs kill extracellular bacteria and/or prevent their dissemination are not well understood. Neutrophils from CGD patients fail to generate NETs (195), although NOX-independent mechanisms have also been described (196–199), some of which utilize ROS from mitochondria (200). ET production by S cells further illustrates the conservation of cell-autonomous defense mechanisms in D. discoideum, a precursor to the specialized phagocytes of the vertebrate immune system (201).
Divalent Metals
Maintaining the concentration of divalent trace metals such as iron, manganese, zinc, and copper is essential for every living organism to preserve metabolism and cell growth. Metalloproteins with trace metals as cofactors play a role in many important cellular functions such as signaling, respiration, transcription, translation, and cell division. Tight regulation of divalent metals is necessary: low levels of iron and manganese have detrimental metabolic effects, whereas zinc and copper are toxic at high concentrations.
For intracellular bacterial pathogens, trace metals are an important micronutrient resource with a role in many metabolic processes and are, as a consequence, essential for intracellular growth. Host cells such as macrophages have developed strategies to control growth of intracellular bacteria by sequestering metals such as iron and manganese (i.e., metal deprivation or nutritional immunity) or by pumping toxic metals inside the pathogen-containing compartment [i.e., metal poisoning; reviewed in Ref. (202–204)]. Bacteria have established ways to counteract metal deprivation or poisoning by expressing siderophores (i.e., small molecules with high affinity for the relevant metal) and uptake systems or by upregulating efflux systems such as P-type ATPases (203).
Consequently, the phagosomal concentration of essential trace metals varies during phagocytosis and infection with bacterial pathogens. Wagner et al. elegantly measured the metal concentration in the phagosome upon macrophage activation with inflammatory cytokines and upon infection with M. tuberculosis or Mycobacterium avium [(205); reviewed in Ref. (206)]. At 1-h post infection (hpi), the early phagosome was shown to be enriched in sulfur and chloride and depleted of calcium and potassium. At a later stage (24 hpi), the MCV harbored zinc, iron, and calcium at high concentrations. In addition, activation of infected macrophages with cytokines leads to a large increase in zinc and copper and a depletion of iron and chloride (205). Importantly, the metal concentration in the phagosome is very likely coupled to the proton gradient. Acidification of the phagosome is achieved by the combined actions of the V-ATPase and the Hv1 H+-channel (207). To counter-balance the electrogenic H+-gradient across the phagosomal membrane, Cl− is imported into the phagosome by transporters of the CFTR (208) and CLC family [(209); reviewed in Ref. (210)], respectively [reviewed in Ref. (206)]. Lysosomal acidification is also facilitated by the efflux of cations (211).
Metal Poisoning
Zinc serves as a cofactor for more than 3,000 metalloproteins and is consequently the second most abundant trace element after iron. Zinc is redox neutral and has many roles in various biological processes as structural, catalytic and signaling component [reviewed in Ref. (212)]. It is essential for macrophage antimicrobial functions and controls among other processes monocyte chemotaxis, phagocytosis, and cytokine production [reviewed in Ref. (204)]. Intracellular zinc homeostasis is tightly regulated. 50% of zinc is present in the cytoplasm, whereas 30–40% can be found inside the nucleus and 10% is bound to membranes [reviewed in Ref. (212)]. To keep the cytosolic concentration of free zinc low (i.e., in the low nanomolar range), it is either bound to metalloproteins or metallothioneins or sequestered into membrane-bound organelles. Zinc is transported through biological membranes by various zinc transport proteins that are classified as zinc transporters (ZnTs, also cation diffusion facilitators) or Zrt-, Irt-related proteins (ZIPs) [reviewed in Ref. (212)]. Whereas ZnTs mediate the zinc transport from the cytosol to either organelles or the extracellular space, the ZIP family mediates transport into the cytosol from the extracellular space or organelles [reviewed in Ref. (212)]. Besides ZnT and ZIP transporters, various other proteins have been shown to mediate zinc transport, such as calcium channels (213), mucolipin-1 in interaction with TMEM163 (214), and members of the NRAMP family [reviewed in Ref. (215)].
Eleven putative zinc transporters have been previously identified in D. discoideum and categorized by functional analogy to mammalian zinc transporters into different subgroups (216). Three of the 11 were classified as members of a “ZIP subfamily” and 4 as members of an “LZT-like subfamily” (216). These two subfamilies correspond to the combined ZIP I and ZIP II subfamilies and the LIV-1 subfamily of mammalian ZIPs, respectively (212). Importantly, the members of the LZT-like family were named ZntA–ZntD even though they were classified as ZIP transporters, and four potential ZnT transporter homologs encoded in the D. discoideum genome were grouped by the authors as the “Cation efflux subfamily” (216).
To demonstrate that the proteins initially named ZntA–ZntD belong to the ZIP family of zinc transporters and are not ZnTs, we generated two simplified phylogenetic trees comparing the sequences of various D. discoideum zinc transport proteins with zinc transporters of other taxonomic groups such as amoebozoa, fungi, plantae, and metazoa. These taxonomic groups were chosen based on previously published phylogenetic studies (176, 217). According to our phylogenetic tree of ZIP transporters (Figure 4) an unequivocal classification of the seven D. discoideum ZIP-like proteins (Zpl) into ZIP I, ZIP II, and LIV-1 subgroups by analogy to the human classification, as was done previously (216), is not possible. Three of the seven ZIP transporters are more similar to each other and cluster in one group (ZplA–C) that is more related to the ZIP transporters of fungi and ZIP II transporters of mammals. The three proteins ZplD–ZplF are more similar to proteins of Amoebozoa, Stramenopiles, and Plantae. ZplG is more related to the human ZIP I subfamily.
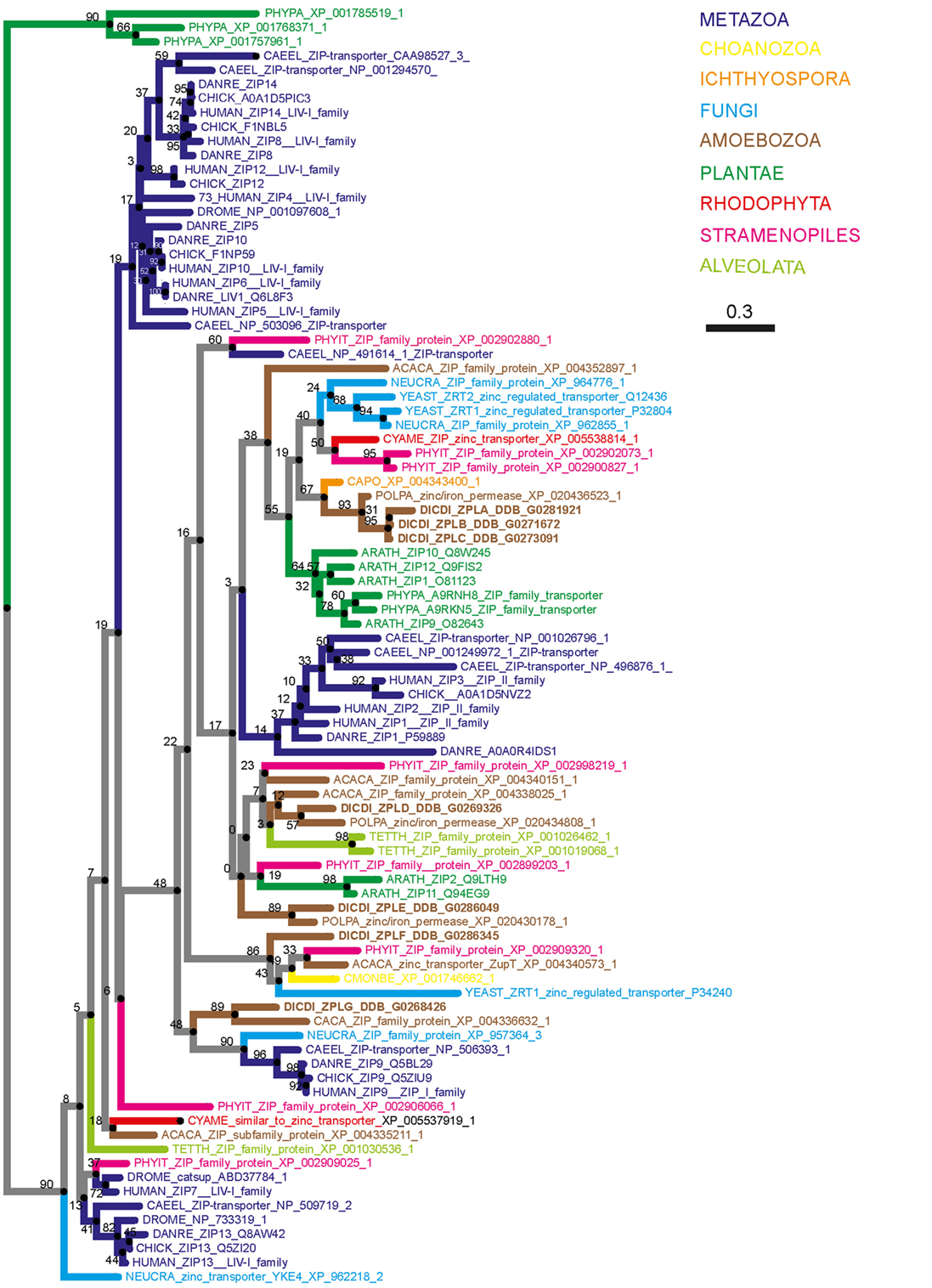
Figure 4. Phylogenetic tree of the Zrt-, Irt-related protein (ZIP) family. The sequence of human ZIP7 was used to search for zinc transporters of the ZIP family among non-redundant sequences in selected organisms using NCBI PSI-BLAST. A score of 2 × 10−5 was used as a threshold. Sequences were aligned with the software AliView [(218); http://ormbunkar.se/aliview/] and curated manually, to remove divergent N- and C-terminus. Trees were built using MAFFT and the E-INS-i strategy (219).
Similarly, our phylogenetic tree of ZnT transporters clearly shows that the four proposed D. discoideum zinc transporters identified based on their homology to ZnTs indeed belong to this family (Figure 5). Consequently, we propose renaming the various transporters as outlined in Table 1 according to their respective family names (Zpl or ZnT). The D. discoideum proteins ZntC and ZntD are likely homologs of the human proteins ZNT6 and ZNT7, which are located in the early secretory pathway and contribute to the activation of zinc-containing enzymes (220). Whereas ZntA does not have a close mammalian homolog, the closest human relatives of ZntB are the early endosomal protein ZNT10 and the plasma membrane protein ZNT1 (212).
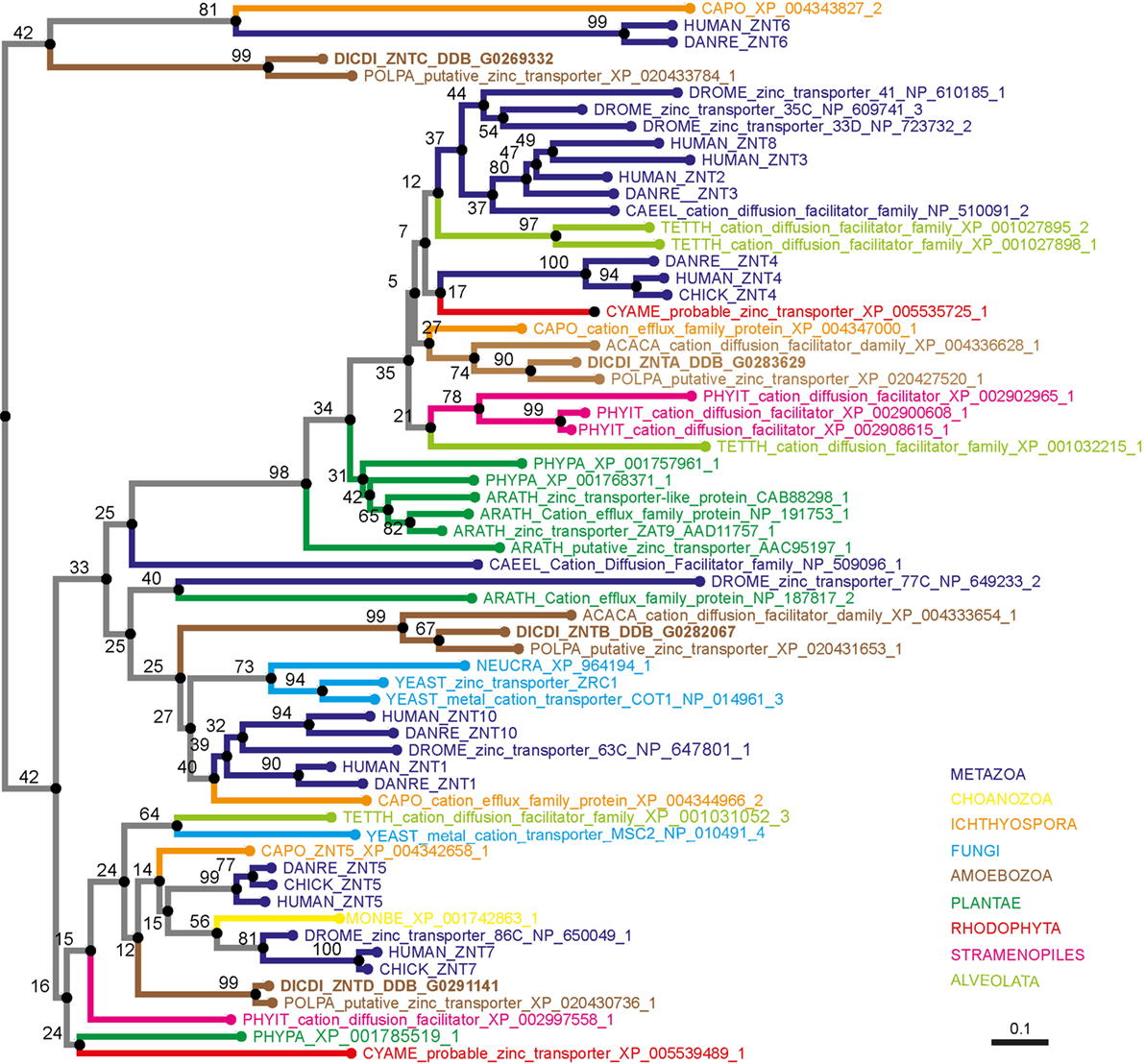
Figure 5. Phylogenetic tree of the ZnT family. The sequence of human ZNT4 was used to search for zinc transporters of the ZnT family among non-redundant sequences in selected organisms using NCBI PSI-BLAST. A score of 2 × 10−5 was used as a threshold. Sequences were aligned with the software AliView [(218); http://ormbunkar.se/aliview/] and curated manually, to remove divergent N- and C-terminus. Trees were built using MAFFT and the E-INS-i strategy (219).
At the host–pathogen interface, zinc deprivation (221) or zinc poisoning are strategies of mammalian professional phagocytes to restrict intracellular bacteria growth. Both processes are probably highly dependent on zinc transporter proteins (222, 223) During M. tuberculosis infection, it was proposed that free zinc is released from metallothioneins via the oxidative burst that is induced upon infection and, consequently, zinc poisoning was proposed as “a new chapter in the host–microbe arms race” (224). The contributions of zinc transporters to cell-autonomous defenses of macrophages and D. discoideum await elucidation.
In contrast to zinc, copper is redox active and cycles under physiological conditions between the two oxidative states Cu+ (i.e., cuprous) and Cu2+ (i.e., cupric). Consequently, copper serves as an ideal cofactor for electron transfer and redox reactions such as respiration and detoxification of free radicals [reviewed in Ref. (225)]. Proteins involved in copper uptake, sequestration, and trafficking regulate copper homeostasis in eukaryotic cells [reviewed in Ref. (226)]. Copper uptake into the cytosol is mediated by the copper permease CTR1 [reviewed in Ref. (227)]. Similar to zinc, copper belongs to the so-called “death metals” and is toxic at high concentrations. Therefore, by analogy to zinc, low copper concentrations in the cytosol are maintained by metallothioneins. Directly after its uptake, chaperones such as ATOX1, CCS, and COX17 are involved in copper trafficking inside the cytoplasm (228).
Copper is imported into the trans-Golgi network by the action of two P-type ATPases: ATP7A and ATP7B. ATP7A is also located at the plasma membrane, where it mediates copper efflux from the cytosol to the extracellular space, and at the phagosomal membrane, where it imports copper from the cytosol into the phagosomal lumen [reviewed in Ref. (226, 228)].
Copper has many antimicrobial properties. Its ability to switch between two oxidation states supports the production of hydroxyl radicals via the Fenton- and the Haber–Weiss reactions [reviewed in Ref. (229)]. In addition, copper is able to disrupt the structure of proteins, and Cu2+ might be able to disrupt Fe–S clusters. In macrophages, copper transport proteins such as ATP7A that mediate Cu2+ import into the phagosome are induced upon infection and stimulation with inflammatory cytokines (230). Bacteria have evolved strategies to overcome high copper concentrations. For instance, a multi-copper oxidase is required for copper resistance of M. tuberculosis, probably by oxidizing toxic Cu2+ in the periplasm (231).
The D. discoideum genome encodes one Ctr-type copper permease (i.e., p80) that, by analogy to mammalian cells, should mediate copper uptake into the cytosol, and three putative copper-translocating P-type ATPases that were annotated as atp1 (DDB_G0273675), atp2, the ortholog of ATP7A (DDB_G0284141), and atp3 (DDB_G0269590) (232, 233). ATP1 was induced upon incubation of D. discoideum with copper salts, leading to the conclusion that ATP1 is responsible for copper tolerance in D. discoideum (233). Expression of ATP7A and ATP3 was increased upon bacteria ingestion and decreased when bacteria and copper salts were added together, arguing for a possible role in copper trafficking during phagocytosis and killing of bacteria. The increased expression of p80 only upon incubation with bacteria suggests that copper is needed for bacterial killing (233).
Nutritional Immunity
In contrary to metal poisoning, nutritional immunity does not kill the bacteria but restricts its intracellular growth. During infection, pathogens need to acquire essential nutrients from the host such as amino acids, lipids, sugars, and, importantly, transition metals. Thus, to restrict their availability to the pathogen, these metals are depleted from the phagosome. The best studied metals that are sequestered by the host are iron and manganese (206, 234–236).
Nutritional immunity of transition metals is controlled in part by the Natural Resistance-Associated Macrophage Protein (i.e., NRAMP) family of divalent-metal transmembrane transporters (237–239). The NRAMP family is widely represented from bacteria to mammals (240, 241), as well as in plants (242) and yeast (243). The family members play an important role in intracellular metal-ion homeostasis and are able to transport a broad range of transition metals (215, 244).
In mammals, two NRAMP members have been identified: NRAMP1 [(SLC11A1) (237)] and NRAMP2 [(SLC11A2, DMT1, or DCT1) (241)]. Between these proteins, 63% of residues are identical and 15% are highly conservative substitutions, and they share very similar secondary structures with hydrophobic cores of 10 transmembrane segments (245). Regarding divalent-metal affinity, NRAMP1 has a clear preference for Mn2+, Fe2+, and Co2+ (246), whereas NRAMP2 transports those three metals and also Zn2+, Cd2+, Cu2+, Ni2+, and Pb2+ (241). NRAMP1 expression is restricted to late endocytic compartments (i.e., endo-lysosomes) of professional phagocytes such as macrophages and neutrophils (247). NRAMP2 is ubiquitously expressed in all mammalian cells and is located at the plasma membrane (248). In addition, NRAMP2 was observed at the apical membrane of enterocytes as well in recycling endosomes (249). Mutations in nramp2 lead to severe microcytic anemia related to an iron absorption deficiency (250, 251). Both NRAMP1 and NRAMP2 might be involved in neurodegenerative diseases such as Parkinson’s (252, 253). NRAMP1 contributes to the resistance to intracellular bacterial infection. Indeed, depletion of NRAMP1 leads to an increased susceptibility of mice to several intracellular pathogens such as Mycobacterium species, Leishmania donovani, and Salmonella species (237, 254–259) by impairing phagosomal acidification and reducing fusion with the lysosomes (260). In human, nramp1 polymorphic variants are associated with susceptibility to tuberculosis (261, 262) or leprosy (263).
Although it is accepted that NRAMPs depend on a V-ATPase-generated proton gradient to drive metal transport (264), the direction of metal transport at the phagosomal membrane remains controversial. Whether NRAMP1 is an antiporter or a symporter and whether NRAMP1 imports or depletes metals from the phagosome are unclear. On the one hand, some authors suggest that NRAMP1 acts as an antiporter of protons and delivers cation metals into the phagosome, contributing to the generation and accumulation of toxic free radicals involved in bacteria killing (265–268). On the other hand, the hypothesis that NRAMP1 operates as a symporter of protons to efflux metals from the phagosome to the cytosol is better supported by the literature and is consistent with the function of its paralog NRAMP2. This is in line with the current hypothesis of nutritional immunity in which pathogen access to metals is restricted (246, 269). These two different scenarios are nicely described in previous reviews (215, 244, 270).
The D. discoideum genome encodes two NRAMP proteins called NRAMP1 (DDB_G0276973) and NRAMPB (DDB_G0275815, formerly NRAMP2). NRAMP1 is an archetypical NRAMP protein, orthologous to NRAMP1 in mammals, whereas NRAMPB is not the ortholog of NRAMP2 in mammals but rather is more closely related to the prototypical NRAMP from bacteria (271). Both transporters are in different subcellular compartments; however, they both co-localize with the V-ATPase. NRAMP1 localizes to macropinosomes and phagosomes with the V-ATPase, and NRAMP1 also localizes to the Golgi region (272). NRAMPB is exclusively found in the membrane of the contractile vacuole (CV) (273), which is enriched for the V-ATPase (108, 274) but has a neutral pH. Single nramp1 or B null mutants exhibit slower growth than wild type under conditions of iron depletion while a double nramp1 and B mutant, but not single mutants, is more resistant than wild type to iron overload (273). These results suggest that NRAMPB and NRAMP1 act non-redundantly to regulate iron homeostasis and that the CV serves as a transient storage compartment for metal cations (Figure 6). During infection, an nramp1 mutant strain is more permissive for intracellular growth of Mycobacterium species and L. pneumophila (272), and nrampB null or nramp1 and B double null mutants are more permissive for L. pneumophila growth (effects of nrampB deletion on the growth of Mycobacterium species have not been reported) (273). Moreover, L. pneumophila inhibits the recruitment of the V-ATPase, which attenuates the antimicrobial effects of NRAMP1 by preventing its proton-driven iron transport activity (46). A recent study demonstrates that, in addition to having an impact on phagosomal iron concentration, both NRAMP1 and NRAMPB influence the translocation efficiency of F. noatunensis from the bacteria-containing compartment into the cytosol, possibly due to alterations in phagosome maturation (53). This new insight into NRAMP function is in line with the results obtained for M. tuberculosis infection in macrophages, in which nramp1 deletion induced a higher level of escape from its vacuole (275).
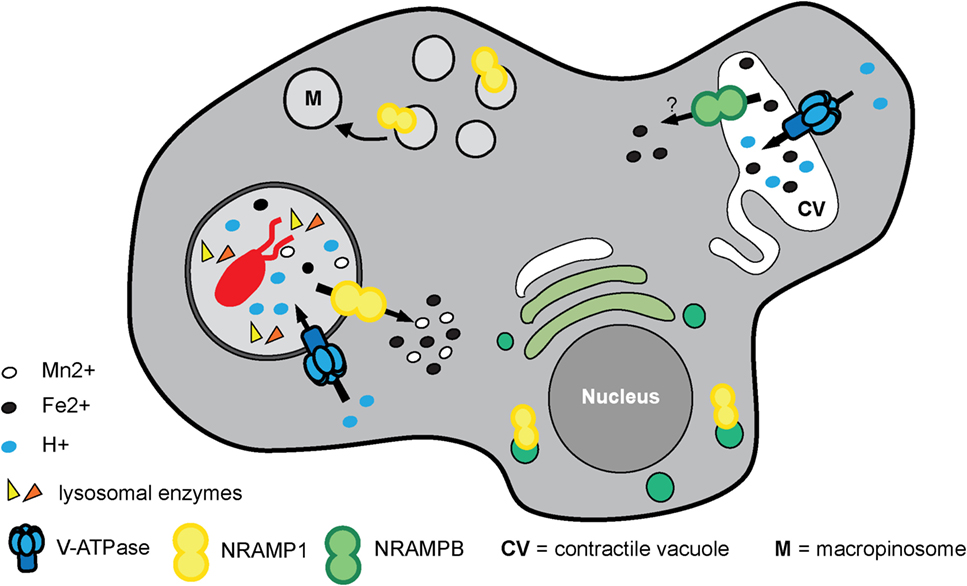
Figure 6. “Nutritional immunity” and homeostasis of transitional metals orchestrated by NRAMP transporters in Dictyostelium discoideum. NRAMP1 is localized at macropinosomes, phagosomes, and the Golgi network whereas NRAMPB is exclusively found in the membrane of the contractile vacuole. Both co-localize with the vacuolar ATPase (V-ATPase), but only NRAMP1 is dependent on the H+-gradient to efflux metals from the phagosome to the cytosol to restrict metal availability to the pathogen in the process referred to as “nutritional immunity.” NRAMPB, together with NRAMP1, contributes to iron homeostasis and regulates osmolarity inside the cell independent of the H+-gradient. Although the literature supports it as similar to the symporter NRAMP1, its role as symporter or antiporter still remains to be clearly defined.
As described earlier, the directionality of the metal transport mediated by NRAMP1 is still poorly understood. Studies in D. discoideum suggest transport into the cytosol. In assays with purified phagosomes, iron export was NRAMP1- and ATP dependent (272). This observation is consistent with NRAMP1 acting as a symporter that uses a V-ATPase-generated proton gradient to transport iron out of the phagosome. Using the iron-chelating fluorochrome calcein, it was shown that NRAMP1 mediates iron efflux from macropinosomes in vivo (271). In addition, to obtain better insight into the ion selectivity of NRAMP1, the authors used Xenopus oocytes expressing NRAMP1, NRAMPB, or rat DMT1/NRAMP2 (used as an internal control). Interestingly, it was shown that NRAMP1 and DMT1 are able to transport Fe2+ and Mn2+ but not Fe3+ or Cu2+ in an electrogenic and proton-dependent manner, whereas NRAMPB transports only Fe2+, and this in a non-electrogenic manner independently from protons (271) (Figure 6).
Autophagy
As described earlier, phagocytosis is the major mechanism by which D. discoideum digests intracellular bacteria with the purpose of nutrient acquisition. However, pathogenic microbes have evolved mechanisms to escape degradation within phagosomes (276). In D. discoideum, as in other eukaryotic phagocytes, bacterial escape from the phagosome triggers a more stringent catabolic pathway named autophagy, which serves as an additional defense mechanism for the infected amoeba [a comprehensive review on autophagy in D. discoideum can be found in Ref. (277)]. The autophagic process by which intracellular pathogens and/or their damaged phagosomes are specifically recognized and digested is termed xenophagy.
The autophagy pathway consists of the formation, upon induction by various stresses such as oxidation, nutrient starvation, or microbial infection, of a double-membrane cisterna, the phagophore, at multiple sites on the ER (36). During xenophagy, the membranes of the phagophore expand around the cytosolic bacterium and/or its damaged compartment to finally engulf them in a closed vacuole called the autophagosome, which, upon fusion with lysosomes, forms an acidic and degradative compartment, the autolysosome, where bacterium and membranes are digested (Figure 7). Many of the proteins involved in the process of autophagosome formation (for instance, proteins forming part of the TORC1, the ULK/Atg1 and the phosphatidylinositol 3-kinase complexes) are conserved between mammalian cells and D. discoideum (278). Interestingly, certain autophagy proteins conserved in both humans and D. discoideum are actually absent in Saccharomyces cerevisiae, making their study in this amoeba a perfect complement to those already performed in yeasts (279).
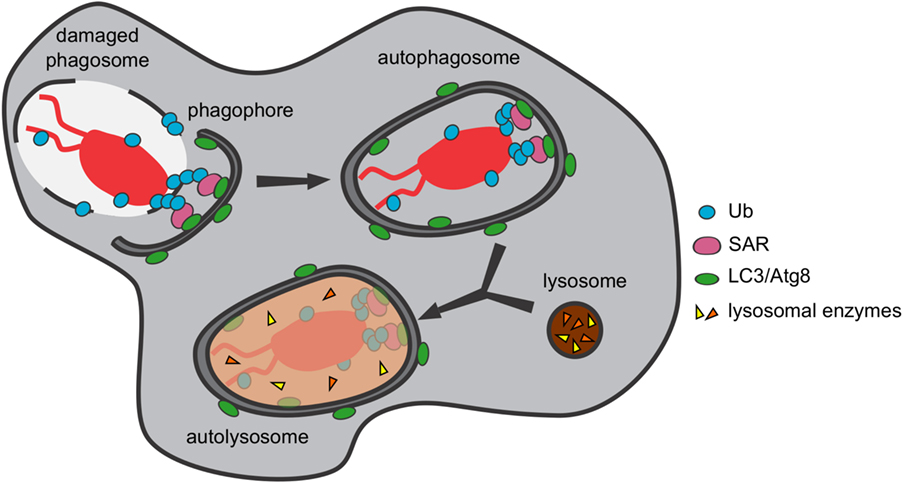
Figure 7. Pathogenic bacteria are captured and digested by xenophagy. The autophagic machinery of Dictyostelium discoideum recognizes and captures cytosolic bacteria and/or their damaged phagosomes in autophagosomes, where they are digested upon fusion with lysosomes.
Specific receptor proteins are in charge of recruiting the phagophore membranes to the bacterial cargos or the remnants of the bacteria-containing compartment, which are tagged with ubiquitin for degradation. These receptors contain a ubiquitin-binding domain, which recognizes the ubiquitinated material, and an LC3-interacting region, which binds the phagophore membrane through interaction with LC3/Atg8, the main autophagosomal marker (280). In D. discoideum, the only selective autophagy receptor identified so far is p62/SQSTM1 (278), which has been shown to recognize the intracellular pathogens F. noatunensis and M. marinum (51, 52, 130). In addition, the mRNA levels of p62/sqstm1 increase upon infection of D. discoideum with both bacterial pathogens, which, in the case of M. marinum, has been demonstrated to be dependent on the membrane damage caused by the bacterium. However, M. marinum avoids its xenophagic killing by presumably blocking lysosomal fusion (51), a mechanism of intracellular mycobacterial survival that was previously proposed to occur in human dendritic cells during infection with M. tuberculosis (281). Other bacteria known to be captured and digested by xenophagy in this amoeba are S. enterica and S. aureus (54, 282).
In addition to pathogenic bacteria, D. discoideum xenophagy also fights against various S. cerevisiae strains (283). Mutant amoebae lacking the autophagy proteins Atg5, Atg6, Atg7, or Atg8 have a decreased capability of preying on this fungus. However, in atg1- amoebae, which cannot produce autophagosomes, S. cerevisiae, Candida albicans, and Candida glabrata are surprisingly killed more efficiently. Koller and collaborators suggest, among other hypotheses, that the autophagic machinery might be used by these yeasts to escape D. discoideum in a non-lytic manner, as already shown for M. marinum. During ejection from the D. discoideum cytosol, the wound generated by the egress of this bacterium through the plasma membrane is sealed with phagophores (130). One might speculate that the yeasts could egress from D. discoideum by autophagosome exocytosis, a process already shown to occur in this amoeba during secretion of the spore differentiation factor 2 precursor AcbA (284). During this unconventional exocytosis, yeasts would be engulfed by autophagic membranes, which would then fuse with multivesicular endosomes before fusing with the plasma membrane to release the yeast. Amoebae lacking Atg1 might be unable to exocytose the yeast efficiently, thus trapping them and facilitating their death. Further investigations are required to validate this hypothesis.
Conserved Microbial Restriction Factors: Lessons from MPS Cells
Studies conducted in MPS cells have identified numerous factors that are involved in the successful restriction of intracellular pathogens. Among these proteins are the glycan-binding galectins, TNF receptor-associated factors (TRAFs), and tripartite motif-containing proteins (TRIMs), which are E3 ubiquitin ligases, the guanylate-binding proteins (GBPs), a family of cytokine-induced large GTPases, and finally the signal transducers and activators of transcription (STAT) proteins. D. discoideum expresses a family of lectins, the discoidins, that might function analogously to galectins. Its genome also encodes homologs of TRAFs, TRIMs, GBPs, and STATs. Based on their counterparts in MPS cells, the D. discoideum versions of these restriction factors are likely to have immune functions (Figure 8).
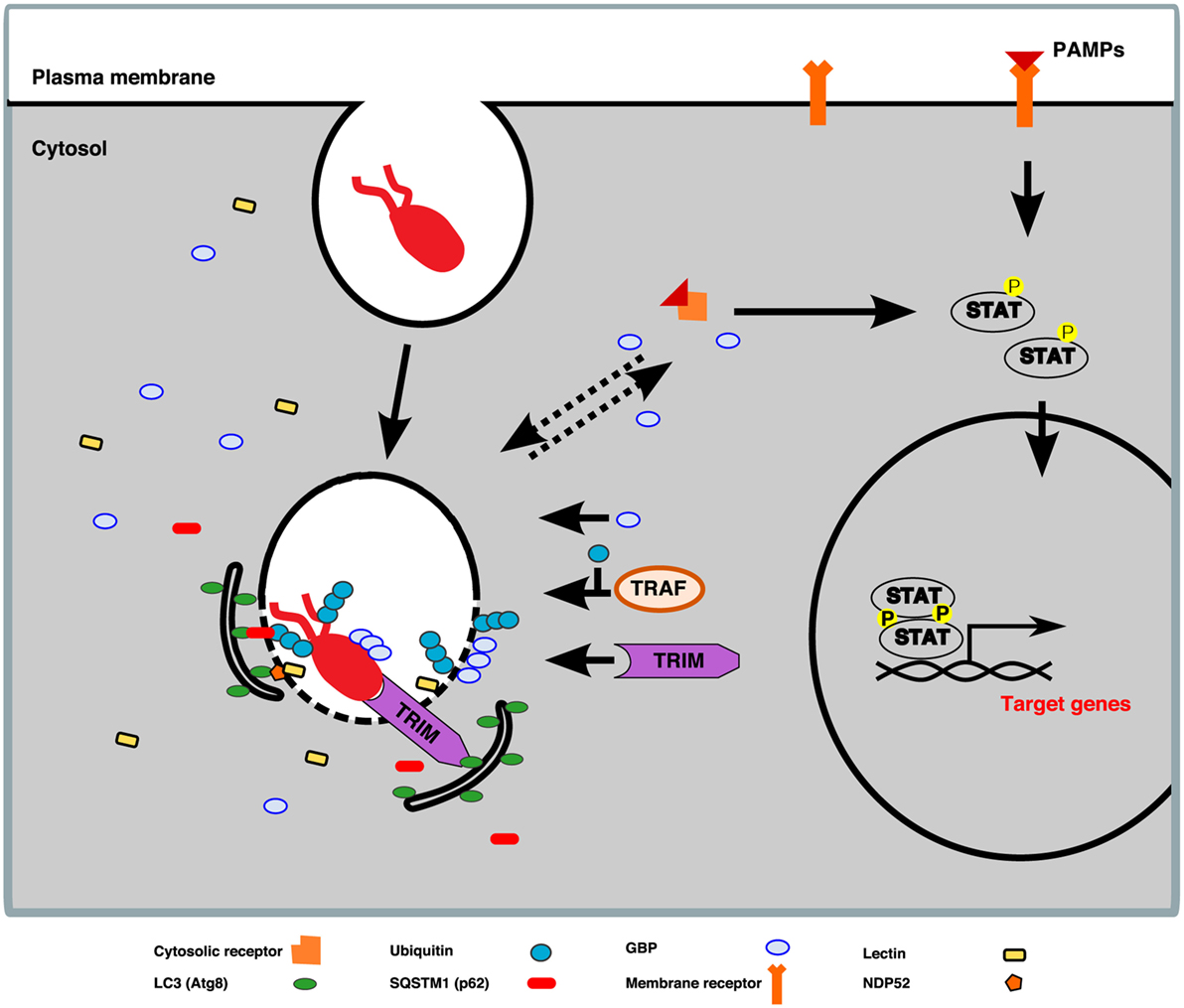
Figure 8. Working model of TNF receptor-associated factor (TRAF), tripartite motif-containing protein (TRIM), guanylate-binding protein (GBP), and signal transducers and activators of transcription (STAT) proteins in Dictyostelium discoideum. Lessons learned from studies in macrophages allow us to envision a model according to which, upon bacterium uptake by D. discoideum, pathogen-associated molecular patterns (PAMPs), or danger-associated molecular patterns, shared by a broad range of microbes, are detected by membrane or cytosolic receptors. The perception of pathogens leads to the activation of transcription factors from the STAT family, which are translocated to the nucleus, where they bind the promoters of innate-immunity-related target genes encoding galectins, GBPs, NADPH oxidases, SQSTM1, TRIMs, and NDP52. In addition, membrane damage of the pathogen-containing vacuole (PCV) exposes pathogens to the cytosol and permits their decoration with K63-linked polyubiquitin chains deposited by members of the TRAF E3-ligase family. The K63-linked polyubiquitination chains serve as a cue for recruitment of the autophagy machinery via autophagy cargo receptors, for instance, SQSTM1 (p62 in D. discoideum). Moreover, K63 ubiquitin-tagged membranes also promote the recruitment of GBP oligomers to the pathogen surface and/or PCV membrane, which facilitates bacteria killing and clearance. Furthermore, members of the TRIM E3-ligase family are able to detect and bind directly to the invading pathogen and mediate its degradation by autophagy. The aforementioned factors are likely to function in an interrelated manner in human macrophages, and it remains to be explored whether the D. discoideum versions are involved in its cell-autonomous defenses.
Discoidins
The galectins compose a family of 15 mammalian lectins with affinity for β-galactoside sugars that share a characteristic carbohydrate recognition domain (285). They have been classified into three groups according to their overall structure: the “prototype,” the “tandem-repeat,” and the “chimera-type.” Galectins are present in the cytosol (286) and nucleus (287) of cells but are also secreted extracellularly via an unconventional secretion mechanism that has remained elusive for decades (288). Galectins play a role in multiple biological processes, including angiogenesis (289), tumor growth (289), and inflammation (290), due to their ubiquitous localization as well as the high diversity of self and non-self glycoconjugates that they recognize [for a review, see Ref. (291)]. Importantly, extracellular lectins bind to the complex glycocalyx coat at the cell surface, which enables the formation of specific microdomains known as the galectin lattice (292). The galectin lattice restricts the mobility of glycoconjugates in the plasma membrane and has important functions in signaling and endocytosis (293). Some galectins recognize the surface of various pathogens (294–297) and have been proposed to have a direct antibacterial effect (298, 299). These interactions involve galectin binding to specific bacterial species or clades according to the carbohydrates displayed on their cell wall or capsules. In addition to their roles in the extracellular milieu, galectins have recently emerged as general innate-immune factors against a wide range of intracellular infections. This newly described function appears not to involve the binding of cytosolic galectins directly to the surface of intracellular pathogens but rather to result from the recognition of self glycans present on the luminal leaflet of pathogen-containing vacuoles. These glycans become accessible to the cytosolic lectins when membrane damage occurs, as shown during infection with S. enterica, L. pneumophila, and Yersinia pseudotuberculosis (300, 301). This process leads to the recruitment of the autophagy machinery (300) or of GBPs (301) to the compartment.
Although D. discoideum lacks galectin homologs, discoidins share molecular and biological characteristics with galectins. They form a family of four β-galactoside-binding and β-N-acetylgalactosamine-binding lectins with two carbohydrate binding domains (302, 303), and recent genome analyses identified three potential discoidin-like proteins in D. discoideum (http://dictybase.org). Discoidins are expressed throughout the D. discoideum life cycle (69, 70). They are highly abundant in the cytosol and are also secreted despite their lack of a signal peptide (304). Early reports suggested a possible role in self-recognition during the multicellular cycle of D. discoideum (305), but it was later observed that mutants with much reduced expression of discoidins (1–2% of wild type) were able to form apparently normal fruiting bodies (306). In addition, subsequent studies were unable to confirm surface localization of discoidins (307, 308). Consequently, the role of discoidins in self-recognition and adhesion during D. discoideum development has remained controversial for several decades. Whether discoidins bind bacteria and/or have a role in cell-autonomous defenses similar to that of galectins is currently under investigation.
Ubiquitination
The significance of ubiquitination in the regulation of various aspects of mammalian immunity has been increasingly recognized in recent years. Ubiquitination is an omnipresent posttranslational modification in which the 76-amino acid ubiquitin is covalently linked to lysine (K) residues of substrate proteins. The stepwise enzymatic cascade of ubiquitination involves following three proteins: ubiquitin-activating enzyme (E1), ubiquitin-conjugating enzyme (E2), and ubiquitin ligase (E3). Their activity results in the attachment of one ubiquitin to the substrate protein linked by an isopeptide bond between the ubiquitin C-terminus and the NH2 group of the substrate K residue. This is referred to as monoubiquitination. Repeated ubiquitination leads to the generation of a polyubiquitin chain, known also as polyubiquitination. Ubiquitin contains seven K residues (K6, K11, K27, K29, K33, K48, and K63). Typically, the attachment of K48-linked polyubiquitin chains to substrate proteins serves as a signal for their degradation by the proteasome. However, the other linkages and the C- or N-terminal linear linkage of ubiquitin moieties play roles in almost all aspects of plant and animal biology, such as growth, development, stress responses, and immunity. In mammals, K63-linked polyubiquitination has been associated with a broad range of immunity-related processes and particularly the activation of the NF-κB pathway, xenophagy and apoptosis (309). D. discoideum has 13 genes encoding ubiquitin, and the TRAFs and TRIMs responsible for K63-linked polyubiquitination are conserved from D. discoideum to mammals.
TNF Receptor-Associated Factors
TNF receptor-associated factors are a family of proteins primarily involved in the regulation of inflammation, antiviral responses, and apoptosis (309, 310). Currently, seven TRAF proteins have been characterized in humans: TRAF1–7. Typically, the TRAF proteins comprise an N-terminal RING domain that mediates the interaction between an E2 ligase and the substrate, followed by a zinc-finger domain, which may play a role in DNA, RNA, protein, and/or lipid binding, and a C-terminal TRAF homology (MATH) domain. The TRAF/MATH domain has an N-terminal TRAF region that mediates homo- and hetero-oligomerization between TRAF members and a C-terminal region that is important for interactions with receptors and adaptor proteins (310).
TNF receptor-associated factor 6, perhaps the most ancient mammalian TRAF, is a RING-type E3-ligase that ubiquitinates via the K63-linkage (311–313). It is required for mTORC1 translocation to the lysosome, and TRAF6-catalyzed K63 polyubiquitination modulates mTORC1 amino acid sensing capacity (314). Moreover, in macrophages, TRAF6 is responsible for the decoration of pathogens and pathogen-containing vacuoles with polyubiquitin chains, which serve as a cue for GBP recruitment and as a recognition signal for the autophagy cargo receptor p62 (313, 315).
BLAST analyses predict that TRAF-like proteins are also present in several social amoeba species. More than 40 TRAF-like proteins in D. discoideum are predicted, of which 16 contain the RING, zinc-finger and TRAF domains present in mammalian TRAF2, TRAF3, TRAF5, and TRAF6 (Figure 9; Table 2). Despite the fact that human and D. discoideum TRAF proteins show significant similarities with respect to their major domains, their evolutionary divergence precludes ortholog assignment. Determining whether D. discoideum TRAFs regulate nutrient sensing and/or ubiquitinate pathogens will expand our understanding of their roles in infection.
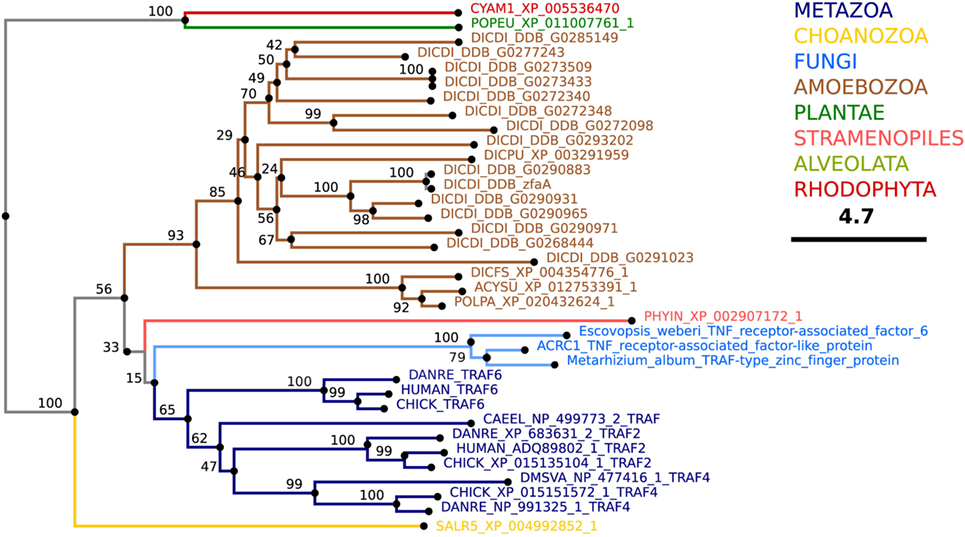
Figure 9. Theoretical phylogenetic relations of TNF receptor-associated factor (TRAF) proteins. The sequence of human TRAF6 was used to search for TRAF homologs among the non-redundant sequences in selected organisms using NCBI PSI-BLAST. A score of 2 × 10−5 was used as a threshold. Similarities of the selected sequences were determined using BLOSUM62 matrix and E-INS-i strategy (219). Sequences were manually curated using AliView software [(218); http://ormbunkar.se/aliview/], and the resulting final alignment was used to generate a neighbor joining phylogenetic tree (NJ, bootstrap 1,000×).
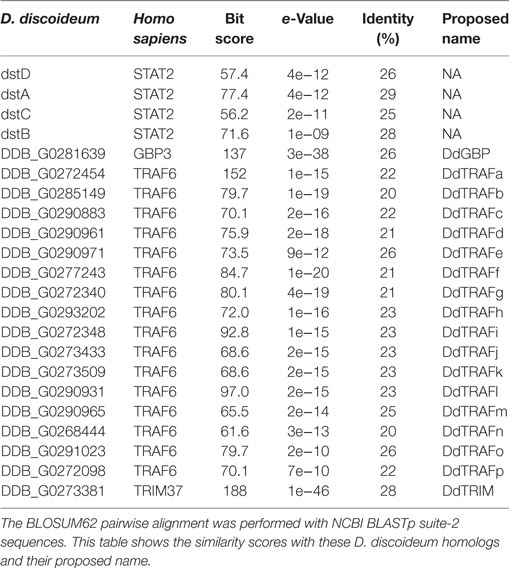
Table 2. Pairwise comparisons of the human TNF receptor-associated factor (TRAF) 6, tripartite motif-containing protein (TRIM) 37, guanylate-binding protein (GBP) 3, and signal transducers and activators of transcription (STAT) 2 with their putative Dictyostelium discoideum homologs.
Tripartite Motif-Containing Proteins
The TRIM superfamily is remarkably conserved among metazoans and, perhaps as a result of an expansion during vertebrate evolution, is represented by more than 80 members in humans. TRIMs typically comprise an N-terminal RING domain, a B-box domain containing several zinc-binding motifs, a coiled-coil domain, and a considerably diverse C-terminal domain important for substrate binding (316).
Tripartite motif-containing proteins are important for many aspects of immunity resistance to pathogens. Recent studies in mouse macrophages demonstrate that various TRIM proteins are induced upon infection with influenza virus or activation of TLRs in a type-I-interferon (IFN)-dependent manner (317, 318). TRIMs are involved in restriction of HIV replication and activation of NF-κB downstream of TLRs (318). Moreover, they play a dual role as receptors and regulators of autophagy. As regulators, TRIMs serve as platforms for the assembly of the core autophagy initiators ULK1 (Atg1 in yeast and D. discoideum) and Beclin1 (Atg6 in yeast and D. discoideum) (319). In macrophages, autophagy cargo receptors recognize and bind K63-linked polyubiquitin chains and galectins, which serve as “eat-me” signals and mediate the binding of the cargo to phagophore-conjugated LC3. As receptors, TRIMs are able to recognize endogenous and exogenous (e.g., bacteria) cargo intended for autoloysosomes via binding of their diverse C-terminal domains to the cargo in a ubiquitin-independent manner and mediate delivery to the phagophore by also binding LC3 (320, 321).
Tripartite motif-containing protein homologs are found in multiple social amoeba species, and a single TRIM has been identified in D. discoideum, DdTRIM, which is an ortholog of human TRIM37 (Figure 10; Table 2) (http://dictybase.org). According to an accumulating amount of evidence, human TRIMs are emerging as critical regulators of cell-autonomous defenses. Particularly, human TRIM37 has been shown to restrict HIV-1 replication (322). At present, the role of DdTRIM remains unknown, and the presence of a single member of the TRIM superfamily early in evolution makes D. discoideum an interesting model to explore the primordial role of TRIM proteins before their expansion.
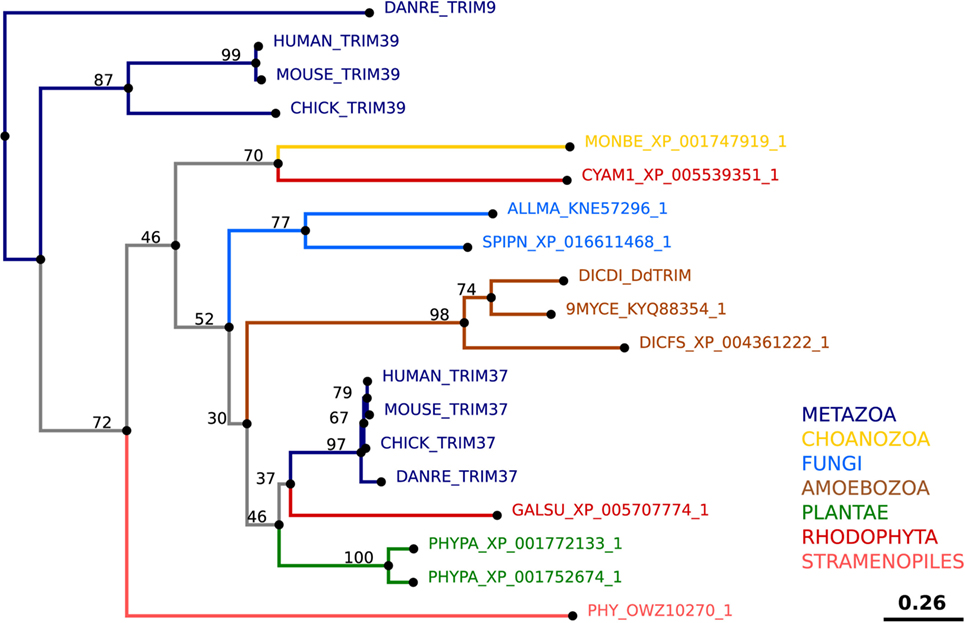
Figure 10. Theoretical phylogenetic relations of tripartite motif-containing protein (TRIM) proteins. The sequence of human TRIM37 was used to search for TRIM homologs among the non-redundant sequences in selected organisms using NCBI PSI-BLAST. A score of 2 × 10−5 was used as a threshold. Similarities of the selected sequences were determined using BLOSUM62 matrix and E-INS-i strategy (219). Sequences were manually curated using AliView software [(218); http://ormbunkar.se/aliview/], and the resulting final alignment was used to generate a neighbor joining phylogenetic tree (NJ, bootstrap 1,000×). TRIM39 was used as an outgroup.
Guanylate-Binding Proteins
The GBP proteins are IFN-gamma-inducible, immunity-related GTPases. Generally, the GBP proteins comprise a globular N-terminal domain and a C-terminal alpha-helical baculovirus inhibitor of apoptosis repeat (BIR) domain. Both the globular N-terminal domain, which confers GTPase activity, and the C-terminal BIR domain mediate protein–protein and protein–lipid interactions and contribute to nucleotide-dependent homotypic and heterotypic GBP protein assembly (323). In addition, BIR domains have been reported to act as caspase regulators and mediators of homotypic interactions (324). GTP binding to the GTPase activity domain allows dimer formation, and its hydrolysis enables conformational changes resulting in GBP tetramer formation. In vertebrates, the GBPs proteins have been linked to a multitude of innate immunity-related responses such as inflammasome activation and xenophagy (325). Essential for their function is their ability to oligomerize and to bind target endomembranes (323, 326, 327). In mouse macrophages, GBP2 recruitment to Chlamydia trachomatis- and Toxoplasma gondii-containing vacuoles correlates with their host-mediated lysis, underlying the importance of these large GTPases in the successful immune response against intracellular invaders (313, 328).
Multiple social amoeba species have homologs of GBPs, and a single GBP homolog has been identified in D. discoideum, DdGBP (Figure 11; Table 2). Its role remains to be elucidated. As a single GBP representative, it may allow a better understanding of the primordial and conserved role that GBPs play in MPS cells.
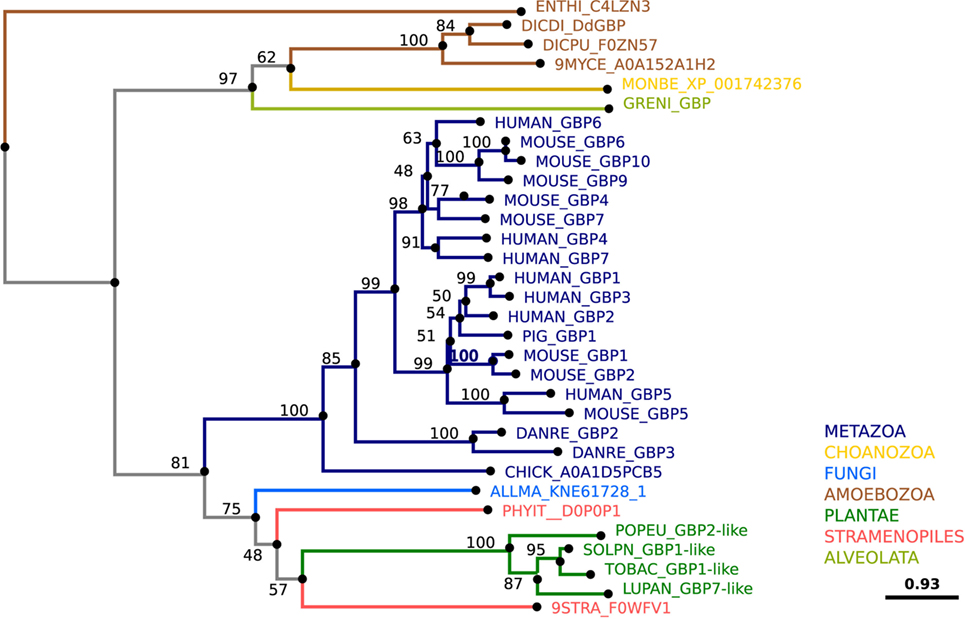
Figure 11. Theoretical phylogenetic relations of guanylate-binding proteins (GBPs). The sequences of human GBPs were used to search for GBP homologs among the non-redundant sequences in selected organisms using NCBI PSI-BLAST. A score of 2 × 10−5 was used as a threshold. Similarities of the selected sequences were determined using BLOSUM62 matrix and E-INS-i strategy (219). Sequences were manually curated using AliView software [(218); http://ormbunkar.se/aliview/], and the resulting final alignment was used to generate a neighbor joining phylogenetic tree (NJ, bootstrap 1,000×).
Signal Transducers and Activators of Transcription
In humans, there are seven STATs, which have a unique N-terminus important for nuclear translocation and protein–protein interactions. This region is followed by a coiled-coil domain involved in nuclear export and regulation of tyrosine phosphorylation, a DNA-binding domain that mediates the recognition of sequences related to TTCN3–4GAA in the promoters of responsive genes, and an Src-homology (SH2) domain, which allows for specific recognition and docking to phosphotyrosines on cytokine receptors, Janus kinases (JAKs), and other STAT molecules. The C-terminus contains a divergent transactivator domain, which mediates STAT transcription factor transactivation via various cofactors (329).
Signal transducers and activators of transcription proteins are activated mainly by cytokines and growth factors. Binding of these signaling molecules to their receptors triggers receptor dimerization, allowing transphosphorylation and activation of receptor-associated JAKs. The JAKs also phosphorylate the cytoplasmic tails of the receptors, which promotes recruitment of the STAT proteins through their SH2 domain. The subsequent tyrosine phosphorylation of STATs results in the formation of homodimers and/or heterodimers and nuclear translocation, whereupon they bind to the promoter regions and initiate transcription of various immunity-related genes such as galectins, GBPs, NOXs, SQSTM1, TRIMs, and NDP52 (330). As part of their functional cycle, STATs shuttle between the cytosol and the nucleus (329). Mammalian STAT proteins act in a tissue-specific manner to regulate growth and development, various immunity-related processes, and cellular stress responses (331, 332). Gain- or loss-of-function mutations in components of the JAK–STAT signaling pathway have been associated with a broad range of innate-immune deficiencies and autoimmune diseases (333).
Multiple social amoeba species have STAT homologs, and four D. discoideum homologs of STATs have been identified, DstA, DstB, DstC, and DstD [reviewed in Ref. (334)] (Figure 12; Table 2). These proteins have a predicted SH2 domain (335), and their activity is regulated in part by tyrosine kinases (336, 337) and phosphatases (338, 339). Like mammalian STATs, D. discoideum STATs regulate growth and development in response to extracellular signaling molecules (335, 340–342) and are activated by cellular stresses (339, 343). Whether they also contribute to antimicrobial responses in D. discoideum is unknown, and addressing this question will provide insight into their possible roles in cell-autonomous defenses.
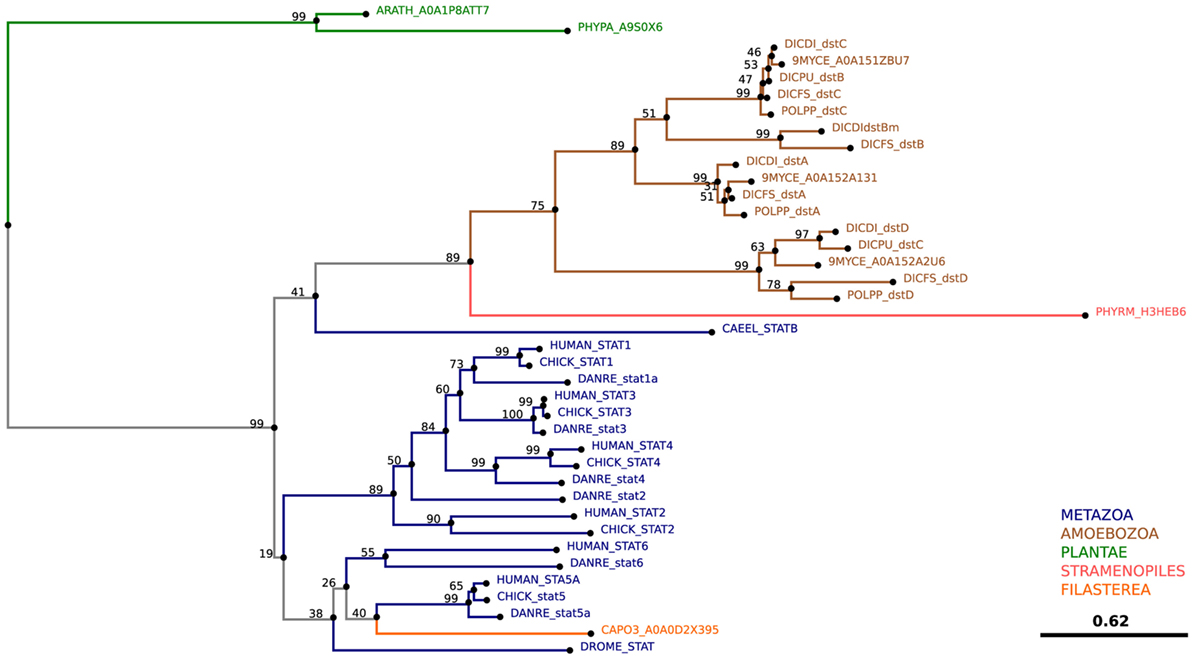
Figure 12. Theoretical phylogenetic relations of signal transducers and activators of transcription (STAT) family proteins. The sequence of the human STAT3 was used to search for STAT homologs among the non-redundant sequences in selected organisms using NCBI PSI-BLAST. A score of 2 × 10−5 was used as a threshold. Similarities of the selected sequences were determined using BLOSUM62 matrix and E-INS-i strategy (219). Sequences were manually curated using AliView software [(218); http://ormbunkar.se/aliview/], and the resulting final alignment was used to generate a neighbor joining phylogenetic tree (NJ, bootstrap 1,000×).
Conclusion and Perspectives
Dictyostelium discoideum is a natural predator of bacteria and must contend with the fact that every meal is a potential infection. To survive this situation, it has evolved multiple mechanisms to generate a microbicidal environment within phagosomes and thus, phagocytosis, its means of nutrient acquisition, is simultaneously a major component of its defenses against infection. Autophagy, a pathway of nutrient reallocation, has also been incorporated into its defenses and is activated when microbes disrupt the phagosome and/or escape into the cytosol. Importantly, core components of these pathways are conserved in the specialized phagocytes of metazoans such as MPS cells. Consequently, D. discoideum is a relevant model to study the role of cell-autonomous defenses in the response of MPS cells to infection. Indeed, it has been used successfully to identify bacterial virulence factors and mechanisms by which bacteria interfere with phagosome maturation and autophagy.
Although one might question the need for model organisms in the CRISPR era of genomic editing, the use of D. discoideum has many advantages and is far from over. The generation of unbiased D. discoideum mutant libraries requires fewer financial and technical resources than creating a library of CRISPR-edited macrophages, and cultivation of this amoeba requires no special growth factors or cytokines. Moreover, growing D. discoideum cells respond to and phagocytose microbes without the need for prior activation. High-throughput mutant screens are thus more feasible and more accessible to laboratories with fewer financial resources. Similarly, the functions of individual bacterial or host factors in infection can be more readily and thoroughly assessed in D. discoideum due to its amenability to numerous research techniques. The knowledge gained can then be used to pose specific questions that can be answered by targeted studies in MPS cells. Primary macrophages or dendritic cells are often used to avoid the potential artifacts of immortalized cell lines. The use of D. discoideum, an organism compliant with the 3R initiative to reduce the use of animals (344), minimizes the need to sacrifice mice to procure these cells. Finally, given its lack of adaptive immunity, D. discoideum provides a context to study cell-autonomous defenses independent of other immune responses.
In addition to its importance as a model phagocyte, D. discoideum is also important from an ecological perspective. It is a key component of a complex network of bacteria, fungi, protists, and insects in the soil. The chemical language of this network is a potential source of natural products (345–348). Interactions between D. discoideum and bacteria can be symbiotic (349–351), and, as a reservoir and training ground for amoeba-resistant microbes, it can provide clues to understand and identify emerging pathogens. The structure of fruiting bodies appears to be evolutionarily optimized to be spread by insects (352), and insect-mediated transmission of fruiting bodies containing infectious Bordetella bronchiseptica, a respiratory pathogen of small mammals, has been demonstrated (353).
Many questions regarding interactions between intracellular pathogens and MPS cells remain unanswered, and D. discoideum still has much to teach us. For example, other than detecting disruption of the phagosome membrane, how D. discoideum “knows” it is infected is unknown. It might sense bacteria with PRRs, but, unless the bacteria are in the cytosol, this would not necessarily distinguish food bacteria that are readily killed from pathogenic bacteria. Given that its defense and nutrient pathways are intertwined, one possibility is that D. discoideum senses dysregulation of metabolism due to nutrient deprivation or imbalance. Another possibility is the recognition of DNA damage caused by bacterial toxins or excessive ROS production. Understanding how D. discoideum senses infection will open new avenues to explore in MPS cells. Other studies will continue to reveal the strategies used by microbes to resist being killed in the phagosome or by autophagy, to delineate the contributions of ROS to antimicrobial responses, and to elucidate the complexities of metal poisoning and nutritional immunity. Characterization of the D. discoideum versions of microbial restriction factors discovered in MPS cells will provide insight into their function and further establish the model. The future is bright for this fascinating and versatile amoeba.
Author Contributions
JDD contributed ideas, wrote sections, created figures, edited, and compiled the manuscript. CBo, CBa, LR, LHL, EC-M, and ATL-J contributed ideas, wrote sections, and created figures. TS contributed ideas and edited.
Conflict of Interest Statement
The authors declare that the research was conducted in the absence of any commercial or financial relationships that could be construed as a potential conflict of interest.
Acknowledgments
The authors gratefully acknowledge the collaboration of Dr. Petra Fey at http://dictybase.org and Dr. Takefumi Kawata for accepting and implementing the new nomenclature of Zinc transporters proposed here.
Funding
The TS laboratory is supported by multiple grants from the Swiss National Science Foundation, and TS is a member of iGE3 (www.ige3.unige.ch) as well as of the COST Actions BM1203 EU-ROS and CA15138 TRANSAUTOPHAGY.
Supplementary Material
The Supplementary Material for this article can be found online at http://www.frontiersin.org/articles/10.3389/fimmu.2017.01906/full#supplementary-material.
References
1. Randow F, MacMicking JD, James LC. Cellular self-defense: how cell-autonomous immunity protects against pathogens. Science (2013) 340(6133):701–6. doi:10.1126/science.1233028
2. Gaudet RG, Bradfield CJ, MacMicking JD. Evolution of cell-autonomous effector mechanisms in macrophages versus non-immune cells. Microbiol Spectr (2016) 4(6). doi:10.1128/microbiolspec.MCHD-0050-2016
3. Matz C, Kjelleberg S. Off the hook – how bacteria survive protozoan grazing. Trends Microbiol (2005) 13(7):302–7. doi:10.1016/j.tim.2005.05.009
4. Casadevall A, Pirofski L-A. Accidental virulence, cryptic pathogenesis, Martians, lost hosts, and the pathogenicity of environmental microbes. Eukaryot Cell (2007) 6(12):2169–74. doi:10.1128/EC.00308-07
5. Cosson P, Soldati T. Eat, kill or die: when amoeba meets bacteria. Curr Opin Microbiol (2008) 11(3):271–6. doi:10.1016/j.mib.2008.05.005
6. Erken M, Lutz C, McDougald D. The rise of pathogens: predation as a factor driving the evolution of human pathogens in the environment. Microb Ecol (2013) 65(4):860–8. doi:10.1007/s00248-013-0189-0
7. Desjardins M, Houde M, Gagnon E. Phagocytosis: the convoluted way from nutrition to adaptive immunity. Immunol Rev (2005) 207:158–65. doi:10.1111/j.0105-2896.2005.00319.x
8. Boulais J, Trost M, Landry CR, Dieckmann R, Levy ED, Soldati T, et al. Molecular characterization of the evolution of phagosomes. Mol Syst Biol (2010) 6:423. doi:10.1038/msb.2010.80
9. Matz C, Bergfeld T, Rice SA, Kjelleberg S. Microcolonies, quorum sensing and cytotoxicity determine the survival of Pseudomonas aeruginosa biofilms exposed to protozoan grazing. Environ Microbiol (2004) 6(3):218–26. doi:10.1038/nrmicro876
10. Matz C, McDougald D, Moreno AM, Yung PY, Yildiz FH, Kjelleberg S. Biofilm formation and phenotypic variation enhance predation-driven persistence of Vibrio cholerae. Proc Natl Acad Sci U S A (2005) 102(46):16819–24. doi:10.1073/pnas.0505350102
11. Adiba S, Nizak C, van Baalen M, Denamur E, Depaulis F. From grazing resistance to pathogenesis: the coincidental evolution of virulence factors. PLoS One (2010) 5(8):e11882. doi:10.1371/journal.pone.0011882
12. Amaro F, Wang W, Gilbert JA, Anderson OR, Shuman HA. Diverse protist grazers select for virulence-related traits in Legionella. ISME J (2015) 9(7):1607–18. doi:10.1038/ismej.2014.248
13. Salah IB, Ghigo E, Drancourt M. Free-living amoebae, a training field for macrophage resistance of mycobacteria. Clin Microbiol Infect (2009) 15(10):894–905. doi:10.1111/j.1469-0691.2009.03011.x
14. Drancourt M. Looking in amoebae as a source of mycobacteria. Microb Pathog (2014) 77:119–24. doi:10.1016/j.micpath.2014.07.001
15. Van der Henst C, Scrignari T, Maclachlan C, Blokesch M. An intracellular replication niche for Vibrio cholerae in the amoeba Acanthamoeba castellanii. ISME J (2016) 10(4):897–910. doi:10.1038/ismej.2015.165
16. Pukatzki S, Kessin RH, Mekalanos JJ. The human pathogen Pseudomonas aeruginosa utilizes conserved virulence pathways to infect the social amoeba Dictyostelium discoideum. Proc Natl Acad Sci U S A (2002) 99(5):3159–64. doi:10.1073/pnas.052704399
17. Pukatzki S, Ma AT, Sturtevant D, Krastins B, Sarracino D, Nelson WC, et al. Identification of a conserved bacterial protein secretion system in Vibrio cholerae using the Dictyostelium host model system. Proc Natl Acad Sci U S A (2006) 103(5):1528–33. doi:10.1073/pnas.0510322103
18. Hilbi H, Weber SS, Ragaz C, Nyfeler Y, Urwyler S. Environmental predators as models for bacterial pathogenesis. Environ Microbiol (2007) 9(3):563–75. doi:10.1111/j.1462-2920.2007.01238.x
19. Pan Y-J, Lin T-L, Hsu C-R, Wang J-T. Use of a Dictyostelium model for isolation of genetic loci associated with phagocytosis and virulence in Klebsiella pneumoniae. Infect Immun (2011) 79(3):1006. doi:10.1128/IAI.00906-10
20. Kessin RH. Dictyostelium: Evolution, Cell Biology, and the Development of Multicellularity. Cambridge, UK; New York: Cambridge University Press (2001).
21. Schaap P. Evolutionary crossroads in developmental biology: Dictyostelium discoideum. Development (2011) 138(3):387–96. doi:10.1242/dev.048934
22. Loomis WF. Cell signaling during development of Dictyostelium. Dev Biol (2014) 391(1):1–16. doi:10.1016/j.ydbio.2014.04.001
23. Sternfeld J, David CN. Oxygen gradients cause pattern orientation in Dictyostelium cell clumps. J Cell Sci (1981) 50:9–17.
24. Feit IN, Medynski EJ, Rothrock MJ. Ammonia differentially suppresses the cAMP chemotaxis of anterior-like cells and prestalk cells in Dictyostelium discoideum. J Biosci (2001) 26(2):157–66. doi:10.1007/BF02703640
25. Bonner JT, Lamont DS. Behavior of cellular slime molds in the soil. Mycologia (2005) 97(1):178–84. doi:10.3852/mycologia.97.1.178
26. Huber RJ, O’Day DH. Extracellular matrix dynamics and functions in the social amoeba Dictyostelium: a critical review. Biochim Biophys Acta (2017) 1861(1 Pt A):2971–80. doi:10.1016/j.bbagen.2016.09.026
27. Suess PM, Watson J, Chen W, Gomer RH. Extracellular polyphosphate signals through Ras and Akt to prime Dictyostelium discoideum cells for development. J Cell Sci (2017) 130(14):2394–404. doi:10.1242/jcs.203372
28. Singh S, Mohamed W, Aguessy A, Dyett E, Shah S, Khan M, et al. Functional interaction of PkcA and PldB regulate aggregation and development in Dictyostelium discoideum. Cell Signal (2017) 34:47–54. doi:10.1016/j.cellsig.2017.02.022
29. Hirose S, Benabentos R, Ho HI, Kuspa A, Shaulsky G. Self-recognition in social amoebae is mediated by allelic pairs of tiger genes. Science (2011) 333(6041):467–70. doi:10.1126/science.1203903
30. Chattwood A, Nagayama K, Bolourani P, Harkin L, Kamjoo M, Weeks G, et al. Developmental lineage priming in Dictyostelium by heterogeneous Ras activation. Elife (2013) 2:e01067. doi:10.7554/eLife.01067
31. Hirose S, Santhanam B, Katoh-Kurosawa M, Shaulsky G, Kuspa A. Allorecognition, via TgrB1 and TgrC1, mediates the transition from unicellularity to multicellularity in the social amoeba Dictyostelium discoideum. Development (2015) 142(20):3561–70. doi:10.1242/dev.123281
32. Levin SR, Brock DA, Queller DC, Strassmann JE. Concurrent coevolution of intra-organismal cheaters and resisters. J Evol Biol (2015) 28(4):756–65. doi:10.1111/jeb.12618
33. Junemann A, Filic V, Winterhoff M, Nordholz B, Litschko C, Schwellenbach H, et al. A Diaphanous-related formin links Ras signaling directly to actin assembly in macropinocytosis and phagocytosis. Proc Natl Acad Sci U S A (2016) 113(47):E7464–73. doi:10.1073/pnas.1611024113
34. Veltman DM, Williams TD, Bloomfield G, Chen BC, Betzig E, Insall RH, et al. A plasma membrane template for macropinocytic cups. Elife (2016) 5:e20085. doi:10.7554/eLife.20085
35. Scavello M, Petlick AR, Ramesh R, Thompson VF, Lotfi P, Charest PG. Protein kinase A regulates the Ras, Rap1 and TORC2 pathways in response to the chemoattractant cAMP in Dictyostelium. J Cell Sci (2017) 130(9):1545–58. doi:10.1242/jcs.177170
36. Tabara LC, Escalante R. VMP1 establishes ER-microdomains that regulate membrane contact sites and autophagy. PLoS One (2016) 11(11):e0166499. doi:10.1371/journal.pone.0166499
37. West CM, Blader IJ. Oxygen sensing by protozoans: how they catch their breath. Curr Opin Microbiol (2015) 26:41–7. doi:10.1016/j.mib.2015.04.006
38. Gilsbach BK, Ho FY, Vetter IR, van Haastert PJ, Wittinghofer A, Kortholt A. Roco kinase structures give insights into the mechanism of Parkinson disease-related leucine-rich-repeat kinase 2 mutations. Proc Natl Acad Sci U S A (2012) 109(26):10322–7. doi:10.1073/pnas.1203223109
39. Ludtmann MH, Otto GP, Schilde C, Chen ZH, Allan CY, Brace S, et al. An ancestral non-proteolytic role for presenilin proteins in multicellular development of the social amoeba Dictyostelium discoideum. J Cell Sci (2014) 127(Pt 7):1576–84. doi:10.1242/jcs.140939
40. Chen S, Annesley SJ, Jasim RAF, Musco VJ, Sanislav O, Fisher PR. The Parkinson’s disease-associated protein DJ-1 plays a positive nonmitochondrial role in endocytosis in Dictyostelium cells. Dis Model Mech (2017) 10(10):1261–71. doi:10.1242/dmm.028084
41. Frej AD, Otto GP, Williams RS. Tipping the scales: lessons from simple model systems on inositol imbalance in neurological disorders. Eur J Cell Biol (2017) 96(2):154–63. doi:10.1016/j.ejcb.2017.01.007
42. Bozzaro S. The model organism Dictyostelium discoideum. Methods Mol Biol (2013) 983:17–37. doi:10.1007/978-1-62703-302-2_2
43. Muller-Taubenberger A, Kortholt A, Eichinger L. Simple system – substantial share: the use of Dictyostelium in cell biology and molecular medicine. Eur J Cell Biol (2013) 92(2):45–53. doi:10.1016/j.ejcb.2012.10.003
44. Urwyler S, Nyfeler Y, Ragaz C, Lee H, Mueller LN, Aebersold R, et al. Proteome analysis of Legionella vacuoles purified by magnetic immunoseparation reveals secretory and endosomal GTPases. Traffic (2009) 10:76–87. doi:10.1111/j.1600-0854.2008.00851.x
45. Weber SS, Ragaz C, Hilbi H. The inositol polyphosphate 5-phosphatase OCRL1 restricts intracellular growth of Legionella, localizes to the replicative vacuole and binds to the bacterial effector LpnE. Cell Microbiol (2009) 11:442–60. doi:10.1111/j.1462-5822.2008.01266.x
46. Peracino B, Balest A, Bozzaro S. Phosphoinositides differentially regulate bacterial uptake and Nramp1-induced resistance to Legionella infection in Dictyostelium. J Cell Sci (2010) 123(Pt 23):4039–51. doi:10.1242/jcs.072124
47. Weber S, Wagner M, Hilbi H. Live-cell imaging of phosphoinositide dynamics and membrane architecture during Legionella infection. MBio (2014) 5: e00839–e813. doi:10.1128/mBio.00839-13
48. Hagedorn M, Soldati T. Flotillin and RacH modulate the intracellular immunity of Dictyostelium to Mycobacterium marinum infection. Cell Microbiol (2007) 9(11):2716–33. doi:10.1111/j.1462-5822.2007.00993.x
49. Hagedorn M, Rohde KH, Russell DG, Soldati T. Infection by tubercular mycobacteria is spread by nonlytic ejection from their amoeba hosts. Science (2009) 323(5922):1729–33. doi:10.1126/science.1169381
50. Barisch C, Soldati T. Mycobacterium marinum degrades both triacylglycerols and phospholipids from its Dictyostelium host to synthesise its own triacylglycerols and generate lipid inclusions. PLoS Pathog (2017) 13(1):e1006095. doi:10.1371/journal.ppat.1006095
51. Cardenal-Munoz E, Arafah S, Lopez-Jimenez AT, Kicka S, Falaise A, Bach F, et al. Mycobacterium marinum antagonistically induces an autophagic response while repressing the autophagic flux in a TORC1- and ESX-1-dependent manner. PLoS Pathog (2017) 13(4):e1006344. doi:10.1371/journal.ppat.1006344
52. Lampe EO, Brenz Y, Herrmann L, Repnik U, Griffiths G, Zingmark C, et al. Dissection of Francisella-host cell interactions in Dictyostelium discoideum. Appl Environ Microbiol (2016) 82(5):1586–98. doi:10.1128/AEM.02950-15
53. Brenz Y, Ohnezeit D, Winther-Larsen HC, Hagedorn M. Nramp1 and NrampB contribute to resistance against Francisella in Dictyostelium. Front Cell Infect Microbiol (2017) 7:282. doi:10.3389/fcimb.2017.00282
54. Jia K, Thomas C, Akbar M, Sun Q, Adams-Huet B, Gilpin C, et al. Autophagy genes protect against Salmonella typhimurium infection and mediate insulin signaling-regulated pathogen resistance. Proc Natl Acad Sci U S A (2009) 106(34):14564–9. doi:10.1073/pnas.0813319106
55. Bozzaro S, Eichinger L. The professional phagocyte Dictyostelium discoideum as a model host for bacterial pathogens. Curr Drug Targets (2011) 12:942–54. doi:10.2174/138945011795677782
56. Artemenko Y, Lampert TJ, Devreotes PN. Moving towards a paradigm: common mechanisms of chemotactic signaling in Dictyostelium and mammalian leukocytes. Cell Mol Life Sci (2014) 71(19):3711–47. doi:10.1007/s00018-014-1638-8
57. Nichols JM, Veltman D, Kay RR. Chemotaxis of a model organism: progress with Dictyostelium. Curr Opin Cell Biol (2015) 36:7–12. doi:10.1016/j.ceb.2015.06.005
58. Bloomfield G, Kay RR. Uses and abuses of macropinocytosis. J Cell Sci (2016) 129(14):2697–705. doi:10.1242/jcs.176149
59. Buckley CM, King JS. Drinking problems: mechanisms of macropinosome formation and maturation. FEBS J (2017) 284(22):3778–90. doi:10.1111/febs.14115
60. Kicka S, Trofimov V, Harrison C, Ouertatani-Sakouhi H, McKinney J, Scapozza L, et al. Establishment and validation of whole-cell based fluorescence assays to identify anti-mycobacterial compounds using the Acanthamoeba castellanii-Mycobacterium marinum host-pathogen system. PLoS One (2014) 9(1):e87834. doi:10.1371/journal.pone.0087834
61. Liao XH, Meena NP, Southall N, Liu L, Swaroop M, Zhang AL, et al. A high-throughput, multi-cell phenotype assay for the identification of novel inhibitors of chemotaxis/migration. Sci Rep (2016) 6:22273. doi:10.1038/srep22273
62. Ouertatani-Sakouhi H, Kicka S, Chiriano G, Harrison CF, Hilbi H, Scapozza L, et al. Inhibitors of Mycobacterium marinum virulence identified in a Dictyostelium discoideum host model. PLoS One (2017) 12(7):e0181121. doi:10.1371/journal.pone.0181121
63. Barisch C, Lopez-Jimenez AT, Soldati T. Live imaging of Mycobacterium marinum infection in Dictyostelium discoideum. Methods Mol Biol (2015) 1285:369–85. doi:10.1007/978-1-4939-2450-9_23
64. Eichinger L, Rivero-Crespo F, editors. Dictyostelium discoideum Protocols. Totowa, NJ: Humana Press (2013).
65. Eichinger L, Pachebat JA, Glockner G, Rajandream MA, Sucgang R, Berriman M, et al. The genome of the social amoeba Dictyostelium discoideum. Nature (2005) 435(7038):43–57. doi:10.1038/nature03481
66. Sucgang R, Kuo A, Tian X, Salerno W, Parikh A, Feasley CL, et al. Comparative genomics of the social amoebae Dictyostelium discoideum and Dictyostelium purpureum. Genome Biol (2011) 12(2):R20. doi:10.1186/gb-2011-12-2-r20
67. Basu S, Fey P, Pandit Y, Dodson R, Kibbe WA, Chisholm RL. DictyBase 2013: integrating multiple dictyostelid species. Nucleic Acids Res (2013) 41(Database issue):D676–83. doi:10.1093/nar/gks1064
68. Glockner G, Lawal HM, Felder M, Singh R, Singer G, Weijer CJ, et al. The multicellularity genes of dictyostelid social amoebas. Nat Commun (2016) 7:12085. doi:10.1038/ncomms12085
69. Rosengarten RD, Santhanam B, Fuller D, Katoh-Kurasawa M, Loomis WF, Zupan B, et al. Leaps and lulls in the developmental transcriptome of Dictyostelium discoideum. BMC Genomics (2015) 16:294. doi:10.1186/s12864-015-1491-7
70. Stajdohar M, Rosengarten RD, Kokosar J, Jeran L, Blenkus D, Shaulsky G, et al. dictyExpress: a web-based platform for sequence data management and analytics in Dictyostelium and beyond. BMC Bioinformatics (2017) 18(1):291. doi:10.1186/s12859-017-1706-9
71. Basu S, Fey P, Jimenez-Morales D, Dodson RJ, Chisholm RL. dictyBase 2015: expanding data and annotations in a new software environment. Genesis (2015) 53(8):523–34. doi:10.1002/dvg.22867
72. Arafah S, Kicka S, Trofimov V, Hagedorn M, Andreu N, Wiles S, et al. Setting up and monitoring an infection of Dictyostelium discoideum with mycobacteria. In: Eichinger L, Rivero F, editors. Dictyostelium discoideum Protocols. Totowa, NJ: Humana Press (2013). p. 403–17.
73. Weber S, Hilbi H. Live cell imaging of phosphoinositide dynamics during Legionella infection. Methods Mol Biol (2014) 1197:153–67. doi:10.1007/978-1-4939-1261-2_9
74. Dominguez-Martin E, Cardenal-Munoz E, King JS, Soldati T, Coria R, Escalante R. Methods to monitor and quantify autophagy in the social amoeba Dictyostelium discoideum. Cells (2017) 6(3):E18. doi:10.3390/cells6030018
75. Bloomfield G, Traynor D, Sander SP, Veltman DM, Pachebat JA, Kay RR. Neurofibromin controls macropinocytosis and phagocytosis in Dictyostelium. Elife (2015) 4. doi:10.7554/eLife.04940
76. Freeman SA, Grinstein S. Phagocytosis: receptors, signal integration, and the cytoskeleton. Immunol Rev (2014) 262:193–215. doi:10.1111/imr.12212
77. Chen G, Zhuchenko O, Kuspa A. Immune-like phagocyte activity in the social amoeba. Science (2007) 317(5838):678–81. doi:10.1126/science.1143991
78. Cornillon S, Gebbie L, Benghezal M, Nair P, Keller S, Wehrle-Haller B, et al. An adhesion molecule in free-living Dictyostelium amoebae with integrin β features. EMBO Rep (2006) 7:617–21. doi:10.1038/sj.embor.7400701
79. Cornillon S, Pech E, Benghezal M, Ravanel K, Gaynor E, Letourneur F, et al. Phg1p Is a nine-transmembrane protein superfamily member involved in Dictyostelium adhesion and phagocytosis. J Biol Chem (2000) 275:34287–92. doi:10.1074/jbc.M006725200
80. Fey P, Stephens S, Titus MA, Chisholm RL. SadA, a novel adhesion receptor in Dictyostelium. J Cell Biol (2002) 159:1109–19. doi:10.1083/jcb.200206067
81. Froquet R, Coadic M.l, Perrin J, Cherix N, Cornillon S, Cosson P. TM9/Phg1 and SadA proteins control surface expression and stability of SibA adhesion molecules in Dictyostelium. Mol Biol Cell (2012) 23:679–86. doi:10.1091/mbc.E11-04-0338
82. Harris TJ, Ravandi A, Siu CH. Assembly of glycoprotein-80 adhesion complexes in Dictyostelium. Receptor compartmentalization and oligomerization in membrane rafts. J Biol Chem (2001) 276(52):48764–74. doi:10.1074/jbc.M108030200
83. Janssen K-P, Rost R, Eichinger L, Schleicher M. Characterization of CD36/LIMPII homologues in Dictyostelium discoideum. J Biol Chem (2001) 276:38899–910. doi:10.1074/jbc.M103384200
84. Gotthardt D, Warnatz HJ, Henschel O, Brückert F, Schleicher M, Soldati T. High-resolution dissection of phagosome maturation reveals distinct membrane trafficking phases. Mol Biol Cell (2002) 13:3508–20. doi:10.1091/mbc.E02-04-0206
85. Vogel G, Thilo L, Schwarz H, Steinhart R. Mechanism of phagocytosis in Dictyostelium discoideum: phagocytosis is mediated by different recognition sites as disclosed by mutants with altered phagocytotic properties. J Cell Biol (1980) 86:456–65. doi:10.1083/jcb.86.2.456
86. Bozzaro S, Roseman S. Adhesion of Dictyostelium discoideum cells to carbohydrates immobilized in polyacrylamide gels. I. Evidence for three sugar-specific cell surface receptors. J Biol Chem (1983) 258:13882–9.
87. Pan M, Xu X, Chen Y, Jin T. Identification of a chemoattractant G-protein-coupled receptor for folic acid that controls both chemotaxis and phagocytosis. Dev Cell (2016) 36:428–39. doi:10.1016/j.devcel.2016.01.012
88. Lima WC, Balestrino D, Forestier C, Cosson P. Two distinct sensing pathways allow recognition of Klebsiella pneumoniae by Dictyostelium amoebae. Cell Microbiol (2014) 16(3):311–23. doi:10.1111/cmi.12226
89. Gotthardt D, Blancheteau V, Bosserhoff A, Ruppert T, Delorenzi M, Soldati T. Proteomics fingerprinting of phagosome maturation and evidence for the role of a Gα during uptake. Mol Cell Proteom (2006) 5:2228–43. doi:10.1074/mcp.M600113-MCP200
90. Insall R, Müller-Taubenberger A, Machesky L, Köhler J, Simmeth E, Atkinson SJ, et al. Dynamics of the Dictyostelium Arp2/3 complex in endocytosis, cytokinesis, and chemotaxis. Cell Motil Cytoskeleton (2001) 50:115–28. doi:10.1002/cm.10005
91. Seastone DJ, Harris E, Temesvari LA, Bear JE, Saxe CL, Cardelli J. The WASp-like protein Scar regulates macropinocytosis, phagocytosis and endosomal membrane flow in Dictyostelium. J Cell Sci (2001) 114:2673–83.
92. Dieckmann R, von Heyden Y, Kistler C, Gopaldass N, Hausherr S, Crawley SW, et al. A myosin IK-Abp1-PakB circuit acts as a switch to regulate phagocytosis efficiency. Mol Biol Cell (2010) 21(9):1505–18. doi:10.1091/mbc.E09-06-0485
93. Gopaldass N, Patel D, Kratzke R, Dieckmann R, Hausherr S, Hagedorn M, et al. Dynamin A, myosin IB and Abp1 couple phagosome maturation to F-actin binding. Traffic (2012) 13:120–30. doi:10.1111/j.1600-0854.2011.01296.x
94. Bozzaro S, Bucci C, Steinert M. Chapter 6 phagocytosis and host-pathogen interactions in Dictyostelium with a look at macrophages. In: Jeon KW, editor. International Review of Cell and Molecular Biology. Burlington: Academic Press (2008). p. 253–300.
95. Rivero F, Xiong H. Rho signaling in Dictyostelium discoideum. Int Rev Cell Mol Biol (2016) 322:61–181. doi:10.1016/bs.ircmb.2015.10.004
96. Dormann D, Weijer G, Dowler S, Weijer CJ. In vivo analysis of 3-phosphoinositide dynamics during Dictyostelium phagocytosis and chemotaxis. J Cell Sci (2004) 117(Pt 26):6497–509. doi:10.1242/jcs.01579
97. Blanc C, Charette S, Cherix N, Lefkir Y, Cosson P, Letourneur F. A novel phosphatidylinositol 4,5-bisphosphate-binding domain targeting the Phg2 kinase to the membrane in Dictyostelium cells. Eur J Cell Biol (2005) 84(12):951–60. doi:10.1016/j.ejcb.2005.09.014
98. Loovers HM, Kortholt A, de Groote H, Whitty L, Nussbaum RL, van Haastert PJM. Regulation of phagocytosis in Dictyostelium by the inositol 5-phosphatase OCRL homolog Dd5P4. Traffic (2007) 8:618–28. doi:10.1111/j.1600-0854.2007.00546.x
99. Derivery E, Sousa C, Gautier JJ, Lombard B, Loew D, Gautreau A. The Arp2/3 activator WASH controls the fission of endosomes through a large multiprotein complex. Dev Cell (2009) 17:712–23. doi:10.1016/j.devcel.2009.09.010
100. Gomez TS, Billadeau DD. A FAM21-containing WASH complex regulates retromer-dependent sorting. Dev Cell (2009) 17:699–711. doi:10.1016/j.devcel.2009.09.009
101. Buckley CM, Gopaldass N, Bosmani C, Johnston SA, Soldati T, Insall RH, et al. WASH drives early recycling from macropinosomes and phagosomes to maintain surface phagocytic receptors. Proc Natl Acad Sci U S A (2016) 113(40):E5906–15. doi:10.1073/pnas.1524532113
102. Gutierrez MG. Functional role(s) of phagosomal Rab GTPases. Small GTPases (2013) 4:148–58. doi:10.4161/sgtp.25604
103. Gorvel JP, Chavrier P, Zerial M, Gruenberg J. rab5 controls early endosome fusion in vitro. Cell (1991) 64(5):915–25. doi:10.1016/0092-8674(91)90316-Q
104. Vieira OV, Bucci C, Harrison RE, Trimble WS, Lanzetti L, Gruenberg J, et al. Modulation of Rab5 and Rab7 recruitment to phagosomes by phosphatidylinositol 3-kinase. Mol Cell Biol (2003) 23(7):2501–14. doi:10.1128/MCB.23.7.2501-2514.2003
105. Harrison RE, Bucci C, Vieira OV, Schroer TA, Grinstein S. Phagosomes fuse with late endosomes and/or lysosomes by extension of membrane protrusions along microtubules: role of Rab7 and RILP. Mol Cell Biol (2003) 23(18):6494–506. doi:10.1128/MCB.23.18.6494-6506.2003
106. Buczynski G, Bush J, Zhang L, Rodriguez-Paris J, Cardelli J. Evidence for a recycling role for Rab7 in regulating a late step in endocytosis and in retention of lysosomal enzymes in Dictyostelium discoideum. Mol Biol Cell (1997) 8(7):1343–60. doi:10.1091/mbc.8.7.1343
107. Rupper A, Grove B, Cardelli J. Rab7 regulates phagosome maturation in Dictyostelium. J Cell Sci (2001) 114:2449–60.
108. Clarke M, Köhler J, Arana Q, Liu T, Heuser J, Gerisch G. Dynamics of the vacuolar H+-ATPase in the contractile vacuole complex and the endosomal pathway of Dictyostelium cells. J Cell Sci (2002) 115:2893–905.
109. Neuhaus EM, Almers W, Soldati T. Morphology and dynamics of the endocytic pathway in Dictyostelium discoideum. Mol Biol Cell (2002) 13(4):1390–407. doi:10.1091/mbc.01-08-0392
110. Sun-Wada G-H, Tabata H, Kawamura N, Aoyama M, Wada Y. Direct recruitment of H+-ATPase from lysosomes for phagosomal acidification. J Cell Sci (2009) 122:2504–13. doi:10.1242/jcs.050443
111. Sattler N, Monroy R, Soldati T. Quantitative analysis of phagocytosis and phagosome maturation. Methods Mol Biol (2013) 983:383–402. doi:10.1007/978-1-62703-302-2_21
112. Yates RM, Hermetter A, Russell DG. The kinetics of phagosome maturation as a function of phagosome/lysosome fusion and acquisition of hydrolytic activity. Traffic (2005) 6:413–20. doi:10.1111/j.1600-0854.2005.00284.x
113. Marchetti A, Lelong E, Cosson P. A measure of endosomal pH by flow cytometry in Dictyostelium. BMC Res Notes (2009) 2:7. doi:10.1186/1756-0500-2-7
114. Le Roux D, Le Bon A, Dumas A, Taleb K, Sachse M, Sikora R, et al. Antigen stored in dendritic cells after macropinocytosis is released unprocessed from late endosomes to target B cells. Blood (2012) 119(1):95–105. doi:10.1182/blood-2011-02-336123
115. Le Roux D, Niedergang F. New insights into antigen encounter by B cells. Immunobiology (2012) 217(12):1285–91. doi:10.1016/j.imbio.2012.07.011
116. Carnell M, Zech T, Calaminus SD, Ura S, Hagedorn M, Johnston SA, et al. Actin polymerization driven by WASH causes V-ATPase retrieval and vesicle neutralization before exocytosis. J Cell Biol (2011) 193:831–9. doi:10.1083/jcb.201009119
117. King JS, Gueho A, Hagedorn M, Gopaldass N, Leuba F, Soldati T, et al. WASH is required for lysosomal recycling and efficient autophagic and phagocytic digestion. Mol Biol Cell (2013) 24(17):2714–26. doi:10.1091/mbc.E13-02-0092
118. Rauchenberger R, Hacker U, Murphy J, Niewöhner J, Maniak M. Coronin and vacuolin identify consecutive stages of a late, actin-coated endocytic compartment in Dictyostelium. Curr Biol (1997) 7:215–8. doi:10.1016/S0960-9822(97)70093-9
119. Jenne N, Rauchenberger R, Hacker U, Kast T, Maniak M. Targeted gene disruption reveals a role for vacuolin B in the late endocytic pathway and exocytosis. J Cell Sci (1998) 111:61–70.
120. Wienke D, Drengk A, Schmauch C, Jenne N, Maniak M. Vacuolin, a flotillin/reggie-related protein from Dictyostelium oligomerizes for endosome association. Eur J Cell Biol (2006) 85:991–1000. doi:10.1016/j.ejcb.2006.04.010
121. Holt OJ, Gallo F, Griffiths GM. Regulating secretory lysosomes. J Biochem (2006) 140:7–12. doi:10.1093/jb/mvj126
122. Lima WC, Leuba F, Soldati T, Cosson P. Mucolipin controls lysosome exocytosis in Dictyostelium. J Cell Sci (2012) 125(Pt 9):2315–22. doi:10.1242/jcs.100362
123. Lu H, Clarke M. Dynamic properties of Legionella-containing phagosomes in Dictyostelium amoebae. Cell Microbiol (2005) 7:995–1007. doi:10.1111/j.1462-5822.2005.00528.x
124. Hilbi H, Weber S, Finsel I. Anchors for effectors: subversion of phosphoinositide lipids by Legionella. Front Microbiol (2011) 2:91. doi:10.3389/fmicb.2011.00091
125. Simon S, Hilbi H. Subversion of cell-autonomous immunity and cell migration by Legionella pneumophila effectors. Front Immunol (2015) 6:447. doi:10.3389/fimmu.2015.00447
126. Hoffmann C, Finsel I, Otto A, Pfaffinger G, Rothmeier E, Hecker M, et al. Functional analysis of novel Rab GTPases identified in the proteome of purified Legionella-containing vacuoles from macrophages. Cell Microbiol (2014) 16:1034–52. doi:10.1111/cmi.12256
127. Vergne I, Chua J, Singh SB, Deretic V. Cell biology of Mycobacterium tuberculosis phagosome. Annu Rev Cell Dev Biol (2004) 20:367–94. doi:10.1146/annurev.cellbio.20.010403.114015
128. Kolonko M, Geffken AC, Blumer T, Hagens K, Schaible UE, Hagedorn M. WASH-driven actin polymerization is required for efficient mycobacterial phagosome maturation arrest. Cell Microbiol (2014) 16:232–46. doi:10.1111/cmi.12217
129. Friedrich N, Hagedorn M, Soldati-Favre D, Soldati T. Prison break: pathogens’ strategies to egress from host cells. Microbiol Mol Biol Rev (2012) 76(4):707–20. doi:10.1128/MMBR.00024-12
130. Gerstenmaier L, Pilla R, Herrmann L, Herrmann H, Prado M, Villafano GJ, et al. The autophagic machinery ensures nonlytic transmission of mycobacteria. Proc Natl Acad Sci U S A (2015) 112(7):E687–92. doi:10.1073/pnas.1423318112
131. Alibaud L, Rombouts Y, Trivelli X, Burguière A, Cirillo SLG, Cirillo JD, et al. A Mycobacterium marinum TesA mutant defective for major cell wall-associated lipids is highly attenuated in Dictyostelium discoideum and zebrafish embryos. Mol Microbiol (2011) 80:919–34. doi:10.1111/j.1365-2958.2011.07618.x
132. Weerdenburg EM, Abdallah AM, Rangkuti F, Ghany MAE, Otto TD, Adroub SA, et al. Genome-wide transposon mutagenesis indicates that Mycobacterium marinum customizes its virulence mechanisms for survival and replication in different hosts. Infect Immun (2015) 83:1778–88. doi:10.1128/IAI.03050-14
133. Chen Y-Y, Yang F-L, Wu S-H, Lin T-L, Wang J-T. Mycobacterium marinum mmar_2318 and mmar_2319 are responsible for lipooligosaccharide biosynthesis and virulence toward Dictyostelium. Front Microbiol (2016) 6:1458. doi:10.3389/fmicb.2015.01458
134. Mohandas P, Budell WC, Mueller E, Au A, Bythrow GV, Quadri LEN. Pleiotropic consequences of gene knockouts in the phthiocerol dimycocerosate and phenolic glycolipid biosynthetic gene cluster of the opportunistic human pathogen Mycobacterium marinum. FEMS Microbiol Lett (2016) 363:fnw016. doi:10.1093/femsle/fnw016
135. Muller I, Subert N, Otto H, Herbst R, Ruhling H, Maniak M, et al. A Dictyostelium mutant with reduced lysozyme levels compensates by increased phagocytic activity. J Biol Chem (2005) 280(11):10435–43. doi:10.1074/jbc.M411445200
136. Nasser W, Santhanam B, Miranda ER, Parikh A, Juneja K, Rot G, et al. Bacterial discrimination by dictyostelid amoebae reveals the complexity of ancient interspecies interactions. Curr Biol (2013) 23:862–72. doi:10.1016/j.cub.2013.04.034
137. Reeves EP, Lu H, Jacobs HL, Messina CGM, Bolsover S, Gabella G, et al. Killing activity of neutrophils is mediated through activation of proteases by K+ flux. Nature (2002) 416:291–7. doi:10.1038/416291a
138. Müller S, Faulhaber A, Sieber C, Pfeifer D, Hochberg T, Gansz M, et al. The endolysosomal cysteine cathepsins L and K are involved in macrophage-mediated clearance of Staphylococcus aureus and the concomitant cytokine induction. FASEB J (2014) 28:162–75. doi:10.1096/fj.13-232272
139. Pires D, Marques J, Pombo JP, Carmo N, Bettencourt P, Neyrolles O, et al. Role of cathepsins in Mycobacterium tuberculosis survival in human macrophages. Sci Rep (2016) 6:sre32247. doi:10.1038/srep32247
140. del Cerro-Vadillo E, Madrazo-Toca F, Carrasco-Marín E, Fernandez-Prieto L, Beck C, Leyva-Cobián F, et al. Cutting edge: a novel nonoxidative phagosomal mechanism exerted by cathepsin-D controls Listeria monocytogenes intracellular growth. J Immunol (2006) 176:1321–5. doi:10.4049/jimmunol.176.3.1321
141. Freeze HH, Bush JM, Cardelli J. Biochemical and genetic analysis of an antigenic determinant found on N-linked oligosaccharides in Dictyostelium. Dev Genet (1990) 11:463–72. doi:10.1002/dvg.1020110523
142. Journet A, Chapel A, Jehan S, Adessi C, Freeze H, Klein G, et al. Characterization of Dictyostelium discoideum cathepsin D. J Cell Sci (1999) 112:3833–43.
143. Souza GM, Hirai J, Mehta DP, Freeze HH. Identification of two novel Dictyostelium discoideum cysteine proteinases that carry N-acetylglucosamine-1-P-modification. J Biol Chem (1995) 270:28938–45. doi:10.1074/jbc.270.48.28938
144. Souza GM, Mehta DP, Lammertz M, Rodriguez-Paris J, Wu R, Cardelli JA, et al. Dictyostelium lysosomal proteins with different sugar modifications sort to functionally distinct compartments. J Cell Sci (1997) 110:2239–48.
145. Kypri E, Schmauch C, Maniak M, Lozanne AD. The BEACH protein LvsB is localized on lysosomes and postlysosomes and limits their fusion with early endosomes. Traffic (2007) 8:774–83. doi:10.1111/j.1600-0854.2007.00567.x
146. Le Coadic M, Froquet R, Lima WC, Dias M, Marchetti A, Cosson P. Phg1/TM9 proteins control intracellular killing of bacteria by determining cellular levels of the Kil1 sulfotransferase in Dictyostelium. PLoS One (2013) 8(1):e53259. doi:10.1371/journal.pone.0053259
147. Harris E, Wang N, Wu W-L, Weatherford A, De Lozanne A, Cardelli J. Dictyostelium LvsB mutants model the lysosomal defects associated with Chediak-Higashi syndrome. Mol Biol Cell (2002) 13:656–69. doi:10.1091/mbc.01-09-0454
148. Benghezal M, Fauvarque MO, Tournebize R, Froquet R, Marchetti A, Bergeret E, et al. Specific host genes required for the killing of Klebsiella bacteria by phagocytes. Cell Microbiol (2006) 8(1):139–48. doi:10.1111/j.1462-5822.2005.00607.x
149. Fang FC. Antimicrobial actions of reactive oxygen species. MBio (2011) 2(5):e00141–111. doi:10.1128/mBio.00141-11
150. Li Q, Harraz MM, Zhou W, Zhang LN, Ding W, Zhang Y, et al. Nox2 and Rac1 regulate H2O2-dependent recruitment of TRAF6 to endosomal interleukin-1 receptor complexes. Mol Cell Biol (2006) 26(1):140–54. doi:10.1128/MCB.26.1.140-154.2006
151. Yang C-S, Kim J-J, Lee SJ, Hwang JH, Lee C-H, Lee M-S, et al. TLR3-triggered reactive oxygen species contribute to inflammatory responses by activating signal transducer and activator of transcription-1. J Immunol (2013) 190(12):6368–77. doi:10.4049/jimmunol.1202574
152. Huang J, Canadien V, Lam GY, Steinberg BE, Dinauer MC, Magalhaes MAO, et al. Activation of antibacterial autophagy by NADPH oxidases. Proc Natl Acad Sci U S A (2009) 106(15):6226–31. doi:10.1073/pnas.0811045106
153. Liu W, Peng Y, Yin Y, Zhou Z, Zhou W, Dai Y. The involvement of NADPH oxidase-mediated ROS in cytokine secretion from macrophages induced by Mycobacterium tuberculosis ESAT-6. Inflammation (2014) 37(3):880–92. doi:10.1007/s10753-013-9808-7
154. Cruz CM, Rinna A, Forman HJ, Ventura ALM, Persechini PM, Ojcius DM. ATP activates a reactive oxygen species-dependent oxidative stress response and secretion of proinflammatory cytokines in macrophages. J Biol Chem (2007) 282(5):2871–9. doi:10.1074/jbc.M608083200
155. Miller JL, Velmurugan K, Cowan MJ, Briken V. The type I NADH dehydrogenase of Mycobacterium tuberculosis counters phagosomal NOX2 activity to inhibit TNF-alpha-mediated host cell apoptosis. PLoS Pathog (2010) 6(4):e1000864. doi:10.1371/journal.ppat.1000864
156. Savina A, Jancic C, Hugues S, Guermonprez P, Vargas P, Moura IC, et al. NOX2 controls phagosomal pH to regulate antigen processing during crosspresentation by dendritic cells. Cell (2006) 126(1):205–18. doi:10.1016/j.cell.2006.05.035
157. Jancic C, Savina A, Wasmeier C, Tolmachova T, El-Benna J, Dang PM-C, et al. Rab27a regulates phagosomal pH and NADPH oxidase recruitment to dendritic cell phagosomes. Nat Cell Biol (2007) 9(4):367–78. doi:10.1038/ncb1552
158. Mantegazza AR, Savina A, Vermeulen M, Pérez L, Geffner J, Hermine O, et al. NADPH oxidase controls phagosomal pH and antigen cross-presentation in human dendritic cells. Blood (2008) 112(12):4712–22. doi:10.1182/blood-2008-01-134791
159. Kuiper JW, Sun C, Magalhaes MA, Glogauer M. Rac regulates PtdInsP(3) signaling and the chemotactic compass through a redox-mediated feedback loop. Blood (2011) 118(23):6164–71. doi:10.1182/blood-2010-09-310383
160. Stanley A, Thompson K, Hynes A, Brakebusch C, Quondamatteo F. NADPH oxidase complex-derived reactive oxygen species, the actin cytoskeleton, and Rho GTPases in cell migration. Antioxid Redox Signal (2014) 20(13):2026–42. doi:10.1089/ars.2013.5713
161. Minakami R, Sumimotoa H. Phagocytosis-coupled activation of the superoxide-producing phagocyte oxidase, a member of the NADPH oxidase (nox) family. Int J Hematol (2006) 84(3):193–8. doi:10.1532/IJH97.06133
162. Bedard K, Krause KH. The NOX family of ROS-generating NADPH oxidases: physiology and pathophysiology. Physiol Rev (2007) 87(1):245–313. doi:10.1152/physrev.00044.2005
163. Nguyen GT, Green ER, Mecsas J. Neutrophils to the ROScue: mechanisms of NADPH oxidase activation and bacterial resistance. Front Cell Infect Microbiol (2017) 7:373. doi:10.3389/fcimb.2017.00373
164. Goldblatt D, Thrasher AJ. Chronic granulomatous disease. Clin Exp Immunol (2000) 122(1):1–9. doi:10.1046/j.1365-2249.2000.01314.x
165. Ben-Ari J, Wolach O, Gavrieli R, Wolach B. Infections associated with chronic granulomatous disease: linking genetics to phenotypic expression. Expert Rev Anti Infect Ther (2012) 10(8):881–94. doi:10.1586/eri.12.77
166. West AP, Brodsky IE, Rahner C, Woo DK, Erdjument-Bromage H, Tempst P, et al. TLR signalling augments macrophage bactericidal activity through mitochondrial ROS. Nature (2011) 472(7344):476–80. doi:10.1038/nature09973
167. Hall CJ, Boyle RH, Astin JW, Flores MV, Oehlers SH, Sanderson LE, et al. Immunoresponsive gene 1 augments bactericidal activity of macrophage-lineage cells by regulating beta-oxidation-dependent mitochondrial ROS production. Cell Metab (2013) 18(2):265–78. doi:10.1016/j.cmet.2013.06.018
168. Nambi S, Long JE, Mishra BB, Baker R, Murphy KC, Olive AJ, et al. The oxidative stress network of Mycobacterium tuberculosis reveals coordination between radical detoxification systems. Cell Host Microbe (2015) 17(6):829–37. doi:10.1016/j.chom.2015.05.008
169. Broxton CN, Culotta VC. SOD enzymes and microbial pathogens: surviving the oxidative storm of infection. PLoS Pathog (2016) 12(1):e1005295. doi:10.1371/journal.ppat.1005295
170. Lodge R, Diallo TO, Descoteaux A. Leishmania donovani lipophosphoglycan blocks NADPH oxidase assembly at the phagosome membrane. Cell Microbiol (2006) 8(12):1922–31. doi:10.1111/j.1462-5822.2006.00758.x
171. Sun J, Singh V, Lau A, Stokes RW, Obregon-Henao A, Orme IM, et al. Mycobacterium tuberculosis nucleoside diphosphate kinase inactivates small GTPases leading to evasion of innate immunity. PLoS Pathog (2013) 9(7):e1003499. doi:10.1371/journal.ppat.1003499
172. de Souza Santos M, Salomon D, Orth K. T3SS effector VopL inhibits the host ROS response, promoting the intracellular survival of vibrio parahaemolyticus. PLoS Pathog (2017) 13(6):e1006438. doi:10.1371/journal.ppat.1006438
173. Vareechon C, Zmina SE, Karmakar M, Pearlman E, Rietsch A. Pseudomonas aeruginosa effector ExoS inhibits ROS production in human neutrophils. Cell Host Microbe (2017) 21(5): 611–8.e615. doi:10.1016/j.chom.2017.04.001
174. Lardy B, Bof M, Aubry L, Paclet MH, Morel F, Satre M, et al. NADPH oxidase homologs are required for normal cell differentiation and morphogenesis in Dictyostelium discoideum. Biochim Biophys Acta (2005) 1744(2):199–212. doi:10.1016/j.bbamcr.2005.02.004
175. Bedard K, Lardy B, Krause KH. NOX family NADPH oxidases: not just in mammals. Biochimie (2007) 89(9):1107–12. doi:10.1016/j.biochi.2007.01.012
176. Zhang X, Krause KH, Xenarios I, Soldati T, Boeckmann B. Evolution of the ferric reductase domain (FRD) superfamily: modularity, functional diversification, and signature motifs. PLoS One (2013) 8(3):e58126. doi:10.1371/journal.pone.0058126
177. Akaza Y, Tsuji A, Yasukawa H. Analysis of the gene encoding copper/zinc superoxide dismutase homolog in Dictyostelium discoideum. Biol Pharm Bull (2002) 25(12):1528–32. doi:10.1248/bpb.25.1528
178. Tsuji A, Akaza Y, Kodaira K, Yasukawa H. Copper/zinc superoxide dismutases in Dictyostelium discoideum: amino acid sequences and expression kinetics. J Biochem Mol Biol Biophys (2002) 6(3):215–20. doi:10.1080/10258140290018711
179. Tsuji A, Akaza Y, Nakamura S, Kodaira K, Yasukawa H. Multinucleation of the sodC-deficient Dictyostelium discoideum. Biol Pharm Bull (2003) 26(8):1174–7. doi:10.1248/bpb.26.1174
180. Katoch B, Begum R. Biochemical basis of the high resistance to oxidative stress in Dictyostelium discoideum. J Biosci (2003) 28(5):581–8. doi:10.1007/BF02703333
181. Pontel LB, Langenick J, Rosado IV, Zhang XY, Traynor D, Kay RR, et al. Xpf suppresses the mutagenic consequences of phagocytosis in Dictyostelium. J Cell Sci (2016) 129(24):4449–54. doi:10.1242/jcs.196337
182. Veeranki S, Kim B, Kim L. The GPI-anchored superoxide dismutase SodC is essential for regulating basal Ras activity and for chemotaxis of Dictyostelium discoideum. J Cell Sci (2008) 121(Pt 18):3099–108. doi:10.1242/jcs.030056
183. Castillo B, Kim SH, Sharief M, Sun T, Kim LW. SodC modulates ras and PKB signaling in Dictyostelium. Eur J Cell Biol (2017) 96(1):1–12. doi:10.1016/j.ejcb.2016.11.001
184. Velmurugan K, Chen B, Miller JL, Azogue S, Gurses S, Hsu T, et al. Mycobacterium tuberculosis nuoG is a virulence gene that inhibits apoptosis of infected host cells. PLoS Pathog (2007) 3(7):e110. doi:10.1371/journal.ppat.0030110
185. Bloomfield G, Pears C. Superoxide signalling required for multicellular development of Dictyostelium. J Cell Sci (2003) 116(Pt 16):3387–97. doi:10.1242/jcs.00649
186. Garcia MX, Alexander H, Mahadeo D, Cotter DA, Alexander S. The Dictyostelium discoideum prespore-specific catalase B functions to control late development and to protect spore viability. Biochim Biophys Acta (2003) 1641(1):55–64. doi:10.1016/S0167-4889(03)00064-8
187. Zhang X, Zhuchenko O, Kuspa A, Soldati T. Social amoebae trap and kill bacteria by casting DNA nets. Nat Commun (2016) 7:10938. doi:10.1038/ncomms10938
188. Smith EW, Lima WC, Charette SJ, Cosson P. Effect of starvation on the endocytic pathway in Dictyostelium cells. Eukaryot Cell (2010) 9(3):387–92. doi:10.1128/EC.00285-09
189. Yang CS, Shin DM, Kim KH, Lee ZW, Lee CH, Park SG, et al. NADPH oxidase 2 interaction with TLR2 is required for efficient innate immune responses to mycobacteria via cathelicidin expression. J Immunol (2009) 182(6):3696–705. doi:10.4049/jimmunol.0802217
190. Brinkmann V, Reichard U, Goosmann C, Fauler B, Uhlemann Y, Weiss DS, et al. Neutrophil extracellular traps kill bacteria. Science (2004) 303(5663):1532–5. doi:10.1126/science.1092385
191. Chow OA, von Köckritz-Blickwede M, Bright AT, Hensler ME, Zinkernagel AS, Cogen AL, et al. Statins enhance formation of phagocyte extracellular traps. Cell Host Microbe (2010) 8(5):445–54. doi:10.1016/j.chom.2010.10.005
192. Boe DM, Curtis BJ, Chen MM, Ippolito JA, Kovacs EJ. Extracellular traps and macrophages: new roles for the versatile phagocyte. J Leukoc Biol (2015) 97(6):1023–35. doi:10.1189/jlb.4RI1014-521R
193. Kalsum S, Braian C, Koeken VACM, Raffetseder J, Lindroth M, van Crevel R, et al. The cording phenotype of Mycobacterium tuberculosis induces the formation of extracellular traps in human macrophages. Front Cell Infect Microbiol (2017) 7:278. doi:10.3389/fcimb.2017.00278
194. Papayannopoulos V. Neutrophil extracellular traps in immunity and disease. Nat Rev Immunol (2017). doi:10.1038/nri.2017.105
195. Fuchs TA, Abed U, Goosmann C, Hurwitz R, Schulze I, Wahn V, et al. Novel cell death program leads to neutrophil extracellular traps. J Cell Biol (2007) 176(2):231–41. doi:10.1083/jcb.200606027
196. Parker H, Dragunow M, Hampton MB, Kettle AJ, Winterbourn CC. Requirements for NADPH oxidase and myeloperoxidase in neutrophil extracellular trap formation differ depending on the stimulus. J Leukoc Biol (2012) 92(4):841–9. doi:10.1189/jlb.1211601
197. Parker H, Winterbourn CC. Reactive oxidants and myeloperoxidase and their involvement in neutrophil extracellular traps. Front Immunol (2012) 3:424. doi:10.3389/fimmu.2012.00424
198. Arai Y, Nishinaka Y, Arai T, Morita M, Mizugishi K, Adachi S, et al. Uric acid induces NADPH oxidase-independent neutrophil extracellular trap formation. Biochem Biophys Res Commun (2014) 443(2):556–61. doi:10.1016/j.bbrc.2013.12.007
199. Bjornsdottir H, Dahlstrand Rudin A, Klose FP, Elmwall J, Welin A, Stylianou M, et al. Phenol-soluble modulin alpha peptide toxins from aggressive Staphylococcus aureus induce rapid formation of neutrophil extracellular traps through a reactive oxygen species-independent pathway. Front Immunol (2017) 8:257. doi:10.3389/fimmu.2017.00257
200. Douda DN, Khan MA, Grasemann H, Palaniyar N. SK3 channel and mitochondrial ROS mediate NADPH oxidase-independent NETosis induced by calcium influx. Proc Natl Acad Sci U S A (2015) 112(9):2817–22. doi:10.1073/pnas.1414055112
201. Zhang X, Soldati T. Of amoebae and men: extracellular DNA traps as an ancient cell-intrinsic defense mechanism. Front Immunol (2016) 7:269. doi:10.3389/fimmu.2016.00269
202. Botella H, Stadthagen G, Lugo-Villarino G, de Chastellier C, Neyrolles O. Metallobiology of host-pathogen interactions: an intoxicating new insight. Trends Microbiol (2012) 20(3):106–12. doi:10.1016/j.tim.2012.01.005
203. German N, Luthje F, Hao X, Ronn R, Rensing C. Microbial virulence and interactions with metals. Prog Mol Biol Transl Sci (2016) 142:27–49. doi:10.1016/bs.pmbts.2016.05.010
204. Subramanian Vignesh K, Deepe GS Jr. Immunological orchestration of zinc homeostasis: the battle between host mechanisms and pathogen defenses. Arch Biochem Biophys (2016) 611:66–78. doi:10.1016/j.abb.2016.02.020
205. Wagner D, Maser J, Lai B, Cai Z, Barry CE III, Honer Zu Bentrup K, et al. Elemental analysis of Mycobacterium avium-, Mycobacterium tuberculosis-, and Mycobacterium smegmatis-containing phagosomes indicates pathogen-induced microenvironments within the host cell’s endosomal system. J Immunol (2005) 174(3):1491–500. doi:10.4049/jimmunol.174.3.1491
206. Soldati T, Neyrolles O. Mycobacteria and the intraphagosomal environment: take it with a pinch of salt(s)! Traffic (2012) 13(8):1042–52. doi:10.1111/j.1600-0854.2012.01358.x
207. El Chemaly A, Nunes P, Jimaja W, Castelbou C, Demaurex N. Hv1 proton channels differentially regulate the pH of neutrophil and macrophage phagosomes by sustaining the production of phagosomal ROS that inhibit the delivery of vacuolar ATPases. J Leukoc Biol (2014) 95(5):827–39. doi:10.1189/jlb.0513251
208. Di A, Brown ME, Deriy LV, Li C, Szeto FL, Chen Y, et al. CFTR regulates phagosome acidification in macrophages and alters bactericidal activity. Nat Cell Biol (2006) 8(9):933–44. doi:10.1038/ncb1456
209. Hara-Chikuma M, Yang B, Sonawane ND, Sasaki S, Uchida S, Verkman AS. ClC-3 chloride channels facilitate endosomal acidification and chloride accumulation. J Biol Chem (2005) 280(2):1241–7. doi:10.1074/jbc.M407030200
210. Jentsch TJ. Chloride and the endosomal-lysosomal pathway: emerging roles of CLC chloride transporters. J Physiol (2007) 578(Pt 3):633–40. doi:10.1113/jphysiol.2006.124719
211. Steinberg BE, Huynh KK, Brodovitch A, Jabs S, Stauber T, Jentsch TJ, et al. A cation counterflux supports lysosomal acidification. J Cell Biol (2010) 189(7):1171–86. doi:10.1083/jcb.200911083
212. Kambe T, Tsuji T, Hashimoto A, Itsumura N. The Physiological, biochemical, and molecular roles of zinc transporters in zinc homeostasis and metabolism. Physiol Rev (2015) 95(3):749–84. doi:10.1152/physrev.00035.2014
213. Bouron A, Oberwinkler J. Contribution of calcium-conducting channels to the transport of zinc ions. Pflugers Arch (2014) 466(3):381–7. doi:10.1007/s00424-013-1295-z
214. Cuajungco MP, Basilio LC, Silva J, Hart T, Tringali J, Chen CC, et al. Cellular zinc levels are modulated by TRPML1-TMEM163 interaction. Traffic (2014) 15(11):1247–65. doi:10.1111/tra.12205
215. Nevo Y, Nelson N. The NRAMP family of metal-ion transporters. Biochim Biophys Acta (2006) 1763(7):609–20. doi:10.1016/j.bbamcr.2006.05.007
216. Sunaga N, Monna M, Shimada N, Tsukamoto M, Kawata T. Expression of zinc transporter family genes in Dictyostelium. Int J Dev Biol (2008) 52(4):377–81. doi:10.1387/ijdb.072389ns
217. Sebe-Pedros A, Degnan BM, Ruiz-Trillo I. The origin of metazoa: a unicellular perspective. Nat Rev Genet (2017) 18(8):498–512. doi:10.1038/nrg.2017.21
218. Larsson A. AliView: a fast and lightweight alignment viewer and editor for large datasets. Bioinformatics (2014) 30(22):3276–8. doi:10.1093/bioinformatics/btu531
219. Katoh K, Kuma K, Toh H, Miyata T. MAFFT version 5: improvement in accuracy of multiple sequence alignment. Nucleic Acids Res (2005) 33(2):511–8. doi:10.1093/nar/gki198
220. Kambe T, Matsunaga M, Takeda TA. Understanding the contribution of zinc transporters in the function of the early secretory pathway. Int J Mol Sci (2017) 18(10):E2179. doi:10.3390/ijms18102179
221. Cassat JE, Skaar EP. Metal ion acquisition in Staphylococcus aureus: overcoming nutritional immunity. Semin Immunopathol (2012) 34(2):215–35. doi:10.1007/s00281-011-0294-4
222. Stafford SL, Bokil NJ, Achard ME, Kapetanovic R, Schembri MA, McEwan AG, et al. Metal ions in macrophage antimicrobial pathways: emerging roles for zinc and copper. Biosci Rep (2013) 33(4):e00049. doi:10.1042/BSR20130014
223. Flannagan RS, Heit B, Heinrichs DE. Antimicrobial mechanisms of macrophages and the immune evasion strategies of Staphylococcus aureus. Pathogens (2015) 4(4):826–68. doi:10.3390/pathogens4040826
224. Botella H, Peyron P, Levillain F, Poincloux R, Poquet Y, Brandli I, et al. Mycobacterial p(1)-type ATPases mediate resistance to zinc poisoning in human macrophages. Cell Host Microbe (2011) 10(3):248–59. doi:10.1016/j.chom.2011.08.006
225. Besold AN, Culbertson EM, Culotta VC. The Yin and Yang of copper during infection. J Biol Inorg Chem (2016) 21(2):137–44. doi:10.1007/s00775-016-1335-1
226. Neyrolles O, Wolschendorf F, Mitra A, Niederweis M. Mycobacteria, metals, and the macrophage. Immunol Rev (2015) 264(1):249–63. doi:10.1111/imr.12265
227. Inesi G. Molecular features of copper binding proteins involved in copper homeostasis. IUBMB Life (2017) 69(4):211–7. doi:10.1002/iub.1590
228. Wang Y, Hodgkinson V, Zhu S, Weisman GA, Petris MJ. Advances in the understanding of mammalian copper transporters. Adv Nutr (2011) 2(2):129–37. doi:10.3945/an.110.000273
229. Hodgkinson V, Petris MJ. Copper homeostasis at the host-pathogen interface. J Biol Chem (2012) 287(17):13549–55. doi:10.1074/jbc.R111.316406
230. White PJ, Broadley MR. Biofortification of crops with seven mineral elements often lacking in human diets – iron, zinc, copper, calcium, magnesium, selenium and iodine. New Phytol (2009) 182(1):49–84. doi:10.1111/j.1469-8137.2008.02738.x
231. Rowland JL, Niederweis M. A multicopper oxidase is required for copper resistance in Mycobacterium tuberculosis. J Bacteriol (2013) 195(16):3724–33. doi:10.1128/JB.00546-13
232. Burlando B, Evangelisti V, Dondero F, Pons G, Camakaris J, Viarengo A. Occurrence of Cu-ATPase in Dictyostelium: possible role in resistance to copper. Biochem Biophys Res Commun (2002) 291(3):476–83. doi:10.1006/bbrc.2002.6463
233. Hao X, Luthje F, Ronn R, German NA, Li X, Huang F, et al. A role for copper in protozoan grazing – two billion years selecting for bacterial copper resistance. Mol Microbiol (2016) 102(4):628–41. doi:10.1111/mmi.13483
234. Kehl-Fie TE, Skaar EP. Nutritional immunity beyond iron: a role for manganese and zinc. Curr Opin Chem Biol (2010) 14(2):218–24. doi:10.1016/j.cbpa.2009.11.008
235. Hood MI, Skaar EP. Nutritional immunity: transition metals at the pathogen-host interface. Nat Rev Microbiol (2012) 10(8):525–37. doi:10.1038/nrmicro2836
236. Morey JR, McDevitt CA, Kehl-Fie TE. Host-imposed manganese starvation of invading pathogens: two routes to the same destination. Biometals (2015) 28(3):509–19. doi:10.1007/s10534-015-9850-z
237. Vidal SM, Malo D, Vogan K, Skamene E, Gros P. Natural resistance to infection with intracellular parasites: isolation of a candidate for Bcg. Cell (1993) 73(3):469–85. doi:10.1016/0092-8674(93)90135-D
238. Cellier MF. Nramp: from sequence to structure and mechanism of divalent metal import. Curr Top Membr (2012) 69:249–93. doi:10.1016/B978-0-12-394390-3.00010-0
239. Wessling-Resnick M. Nramp1 and other transporters involved in metal withholding during infection. J Biol Chem (2015) 290(31):18984–90. doi:10.1074/jbc.R115.643973
240. Cellier M, Belouchi A, Gros P. Resistance to intracellular infections: comparative genomic analysis of Nramp. Trends Genet (1996) 12(6):201–4. doi:10.1016/0168-9525(96)30042-5
241. Gunshin H, Mackenzie B, Berger UV, Gunshin Y, Romero MF, Boron WF, et al. Cloning and characterization of a mammalian proton-coupled metal-ion transporter. Nature (1997) 388(6641):482–8. doi:10.1038/41343
242. Belouchi A, Kwan T, Gros P. Cloning and characterization of the OsNramp family from Oryza sativa, a new family of membrane proteins possibly implicated in the transport of metal ions. Plant Mol Biol (1997) 33(6):1085–92. doi:10.1023/A:1005723304911
243. Portnoy ME, Liu XF, Culotta VC. Saccharomyces cerevisiae expresses three functionally distinct homologues of the nramp family of metal transporters. Mol Cell Biol (2000) 20(21):7893–902. doi:10.1128/MCB.20.21.7893-7902.2000
244. Forbes JR, Gros P. Divalent-metal transport by NRAMP proteins at the interface of host-pathogen interactions. Trends Microbiol (2001) 9(8):397–403. doi:10.1016/S0966-842X(01)02098-4
245. Gruenheid S, Cellier M, Vidal S, Gros P. Identification and characterization of a second mouse Nramp gene. Genomics (1995) 25(2):514–25. doi:10.1016/0888-7543(95)80053-O
246. Forbes JR, Gros P. Iron, manganese, and cobalt transport by Nramp1 (Slc11a1) and Nramp2 (Slc11a2) expressed at the plasma membrane. Blood (2003) 102(5):1884–92. doi:10.1182/blood-2003-02-0425
247. Gruenheid S, Pinner E, Desjardins M, Gros P. Natural resistance to infection with intracellular pathogens: the Nramp1 protein is recruited to the membrane of the phagosome. J Exp Med (1997) 185(4):717–30. doi:10.1084/jem.185.4.717
248. Picard V, Govoni G, Jabado N, Gros P. Nramp 2 (DCT1/DMT1) expressed at the plasma membrane transports iron and other divalent cations into a calcein-accessible cytoplasmic pool. J Biol Chem (2000) 275(46):35738–45. doi:10.1074/jbc.M005387200
249. Gruenheid S, Canonne-Hergaux F, Gauthier S, Hackam DJ, Grinstein S, Gros P. The iron transport protein NRAMP2 is an integral membrane glycoprotein that colocalizes with transferrin in recycling endosomes. J Exp Med (1999) 189(5):831–41. doi:10.1084/jem.189.5.831
250. Fleming MD, Trenor CC III, Su MA, Foernzler D, Beier DR, Dietrich WF, et al. Microcytic anaemia mice have a mutation in Nramp2, a candidate iron transporter gene. Nat Genet (1997) 16(4):383–6. doi:10.1038/ng0897-383
251. Canonne-Hergaux F, Fleming MD, Levy JE, Gauthier S, Ralph T, Picard V, et al. The Nramp2/DMT1 iron transporter is induced in the duodenum of microcytic anemia MK mice but is not properly targeted to the intestinal brush border. Blood (2000) 96(12):3964–70.
252. Salazar J, Mena N, Hunot S, Prigent A, Alvarez-Fischer D, Arredondo M, et al. Divalent metal transporter 1 (DMT1) contributes to neurodegeneration in animal models of Parkinson’s disease. Proc Natl Acad Sci U S A (2008) 105(47):18578–83. doi:10.1073/pnas.0804373105
253. Wu KC, Liou HH, Kao YH, Lee CY, Lin CJ. The critical role of Nramp1 in degrading alpha-synuclein oligomers in microglia under iron overload condition. Neurobiol Dis (2017) 104:61–72. doi:10.1016/j.nbd.2017.05.001
254. Gros P, Skamene E, Forget A. Genetic control of natural resistance to Mycobacterium bovis (BCG) in mice. J Immunol (1981) 127(6):2417–21.
255. Skamene E, Gros P, Forget A, Kongshavn PA, St Charles C, Taylor BA. Genetic regulation of resistance to intracellular pathogens. Nature (1982) 297(5866):506–9. doi:10.1038/297506a0
256. Lissner CR, Swanson RN, O’Brien AD. Genetic control of the innate resistance of mice to Salmonella typhimurium: expression of the Ity gene in peritoneal and splenic macrophages isolated in vitro. J Immunol (1983) 131(6):3006–13.
257. Vidal S, Gros P, Skamene E. Natural resistance to infection with intracellular parasites: molecular genetics identifies Nramp1 as the Bcg/Ity/Lsh locus. J Leukoc Biol (1995) 58(4):382–90.
258. Vidal S, Tremblay ML, Govoni G, Gauthier S, Sebastiani G, Malo D, et al. The Ity/Lsh/Bcg locus: natural resistance to infection with intracellular parasites is abrogated by disruption of the Nramp1 gene. J Exp Med (1995) 182(3):655–66. doi:10.1084/jem.182.3.655
259. Vidal SM, Pinner E, Lepage P, Gauthier S, Gros P. Natural resistance to intracellular infections: Nramp1 encodes a membrane phosphoglycoprotein absent in macrophages from susceptible (Nramp1 D169) mouse strains. J Immunol (1996) 157(8):3559–68.
260. Hackam DJ, Rotstein OD, Zhang W, Gruenheid S, Gros P, Grinstein S. Host resistance to intracellular infection: mutation of natural resistance-associated macrophage protein 1 (Nramp1) impairs phagosomal acidification. J Exp Med (1998) 188(2):351–64. doi:10.1084/jem.188.2.351
261. Bellamy R, Ruwende C, Corrah T, McAdam KP, Whittle HC, Hill AV. Variations in the NRAMP1 gene and susceptibility to tuberculosis in West Africans. N Engl J Med (1998) 338(10):640–4. doi:10.1056/NEJM199803053381002
262. Medapati RV, Suvvari S, Godi S, Gangisetti P. NRAMP1 and VDR gene polymorphisms in susceptibility to pulmonary tuberculosis among Andhra Pradesh population in India: a case-control study. BMC Pulm Med (2017) 17(1):89. doi:10.1186/s12890-017-0431-5
263. Abel L, Sanchez FO, Oberti J, Thuc NV, Hoa LV, Lap VD, et al. Susceptibility to leprosy is linked to the human NRAMP1 gene. J Infect Dis (1998) 177(1):133–45. doi:10.1086/513830
264. Mackenzie B, Hediger MA. SLC11 family of H+-coupled metal-ion transporters NRAMP1 and DMT1. Pflugers Arch (2004) 447(5):571–9. doi:10.1007/s00424-003-1141-9
265. Kuhn DE, Baker BD, Lafuse WP, Zwilling BS. Differential iron transport into phagosomes isolated from the RAW264.7 macrophage cell lines transfected with Nramp1Gly169 or Nramp1Asp169. J Leukoc Biol (1999) 66(1):113–9.
266. Zwilling BS, Kuhn DE, Wikoff L, Brown D, Lafuse W. Role of iron in Nramp1-mediated inhibition of mycobacterial growth. Infect Immun (1999) 67(3):1386–92.
267. Goswami T, Bhattacharjee A, Babal P, Searle S, Moore E, Li M, et al. Natural-resistance-associated macrophage protein 1 is an H+/bivalent cation antiporter. Biochem J (2001) 354(Pt 3):511–9. doi:10.1042/bj3540511
268. Kuhn DE, Lafuse WP, Zwilling BS. Iron transport into Mycobacterium avium-containing phagosomes from an Nramp1(Gly169)-transfected RAW264.7 macrophage cell line. J Leukoc Biol (2001) 69(1):43–9.
269. Jabado N, Jankowski A, Dougaparsad S, Picard V, Grinstein S, Gros P. Natural resistance to intracellular infections: natural resistance-associated macrophage protein 1 (Nramp1) functions as a pH-dependent manganese transporter at the phagosomal membrane. J Exp Med (2000) 192(9):1237–48. doi:10.1084/jem.192.9.1237
270. Courville P, Chaloupka R, Cellier MF. Recent progress in structure-function analyses of Nramp proton-dependent metal-ion transporters. Biochem Cell Biol (2006) 84(6):960–78. doi:10.1139/o06-193
271. Buracco S, Peracino B, Cinquetti R, Signoretto E, Vollero A, Imperiali F, et al. Dictyostelium Nramp1, which is structurally and functionally similar to mammalian DMT1 transporter, mediates phagosomal iron efflux. J Cell Sci (2015) 128(17):3304–16. doi:10.1242/jcs.173153
272. Peracino B, Wagner C, Balest A, Balbo A, Pergolizzi B, Noegel AA, et al. Function and mechanism of action of Dictyostelium Nramp1 (Slc11a1) in bacterial infection. Traffic (2006) 7(1):22–38. doi:10.1111/j.1600-0854.2005.00356.x
273. Peracino B, Buracco S, Bozzaro S. The Nramp (Slc11) proteins regulate development, resistance to pathogenic bacteria and iron homeostasis in Dictyostelium discoideum. J Cell Sci (2013) 126(Pt 1):301–11. doi:10.1242/jcs.116210
274. Heuser J, Zhu Q, Clarke M. Proton pumps populate the contractile vacuoles of Dictyostelium amoebae. J Cell Biol (1993) 121(6):1311–27. doi:10.1083/jcb.121.6.1311
275. Simeone R, Sayes F, Song O, Groschel MI, Brodin P, Brosch R, et al. Cytosolic access of Mycobacterium tuberculosis: critical impact of phagosomal acidification control and demonstration of occurrence in vivo. PLoS Pathog (2015) 11(2):e1004650. doi:10.1371/journal.ppat.1004650
276. Hybiske K, Stephens RS. Exit strategies of intracellular pathogens. Nat Rev Microbiol (2008) 6(2):99–110. doi:10.1038/nrmicro1821
277. Mesquita A, Cardenal-Munoz E, Dominguez E, Munoz-Braceras S, Nunez-Corcuera B, Phillips BA, et al. Autophagy in Dictyostelium: mechanisms, regulation and disease in a simple biomedical model. Autophagy (2017) 13(1):24–40. doi:10.1080/15548627.2016.1226737
278. Calvo-Garrido J, Carilla-Latorre S, Kubohara Y, Santos-Rodrigo N, Mesquita A, Soldati T, et al. Autophagy in Dictyostelium: genes and pathways, cell death and infection. Autophagy (2010) 6(6):686–701. doi:10.4161/auto.6.6.12513
279. Reggiori F, Klionsky DJ. Autophagic processes in yeast: mechanism, machinery and regulation. Genetics (2013) 194(2):341–61. doi:10.1534/genetics.112.149013
280. Stolz A, Ernst A, Dikic I. Cargo recognition and trafficking in selective autophagy. Nat Cell Biol (2014) 16(6):495–501. doi:10.1038/ncb2979
281. Romagnoli A, Etna MP, Giacomini E, Pardini M, Remoli ME, Corazzari M, et al. ESX-1 dependent impairment of autophagic flux by Mycobacterium tuberculosis in human dendritic cells. Autophagy (2012) 8(9):1357–70. doi:10.4161/auto.20881
282. Pflaum K, Gerdes K, Yovo K, Callahan J, Snyder ML. Lipopolysaccharide induction of autophagy is associated with enhanced bactericidal activity in Dictyostelium discoideum. Biochem Biophys Res Commun (2012) 422(3):417–22. doi:10.1016/j.bbrc.2012.05.006
283. Koller B, Schramm C, Siebert S, Triebel J, Deland E, Pfefferkorn AM, et al. Dictyostelium discoideum as a novel host system to study the interaction between phagocytes and yeasts. Front Microbiol (2016) 7:1665. doi:10.3389/fmicb.2016.01665
284. Cabral M, Anjard C, Malhotra V, Loomis WF, Kuspa A. Unconventional secretion of AcbA in Dictyostelium discoideum through a vesicular intermediate. Eukaryot Cell (2010) 9(7):1009–17. doi:10.1128/ec.00337-09
285. Barondes SH, Castronovo V, Cooper DN, Cummings RD, Drickamer K, Feizi T, et al. Galectins: a family of animal beta-galactoside-binding lectins. Cell (1994) 76(4):597–8. doi:10.1016/0092-8674(94)90498-7
286. Briles EB, Gregory W, Fletcher P, Kornfeld S. Vertebrate lectins, comparison of properties of beta-galactoside-binding lectins from tissues of calf and chicken. J Cell Biol (1979) 81(3):528–37. doi:10.1083/jcb.81.3.528
287. Arnoys EJ, Ackerman CM, Wang JL. Nucleocytoplasmic shuttling of galectin-3. Methods Mol Biol (2015) 1207:465–83. doi:10.1007/978-1-4939-1396-1_30
288. Seelenmeyer C, Stegmayer C, Nickel W. Unconventional secretion of fibroblast growth factor 2 and galectin-1 does not require shedding of plasma membrane-derived vesicles. FEBS Lett (2008) 582(9):1362–8. doi:10.1016/j.febslet.2008.03.024
289. Griffioen AW, Thijssen VL. Galectins in tumor angiogenesis. Ann Transl Med (2014) 2(9):90. doi:10.3978/j.issn.2305-5839.2014.09.01
290. Liu FT, Yang RY, Hsu DK. Galectins in acute and chronic inflammation. Ann N Y Acad Sci (2012) 1253:80–91. doi:10.1111/j.1749-6632.2011.06386.x
291. Arthur CM, Baruffi MD, Cummings RD, Stowell SR. Evolving mechanistic insights into galectin functions. Methods Mol Biol (2015) 1207:1–35. doi:10.1007/978-1-4939-1396-1_1
292. Nabi IR, Shankar J, Dennis JW. The galectin lattice at a glance. J Cell Sci (2015) 128(13):2213–9. doi:10.1242/jcs.151159
293. Lakshminarayan R, Wunder C, Becken U, Howes MT, Benzing C, Arumugam S, et al. Galectin-3 drives glycosphingolipid-dependent biogenesis of clathrin-independent carriers. Nat Cell Biol (2014) 16(6):595–606. doi:10.1038/ncb2970
294. Mey A, Leffler H, Hmama Z, Normier G, Revillard JP. The animal lectin galectin-3 interacts with bacterial lipopolysaccharides via two independent sites. J Immunol (1996) 156(4):1572–7.
295. Kamhawi S. Phlebotomine sand flies and Leishmania parasites: friends or foes? Trends Parasitol (2006) 22(9):439–45. doi:10.1016/j.pt.2006.06.012
296. Lee B. Envelope-receptor interactions in Nipah virus pathobiology. Ann N Y Acad Sci (2007) 1102:51–65. doi:10.1196/annals.1408.004
297. Sato S, St-Pierre C, Bhaumik P, Nieminen J. Galectins in innate immunity: dual functions of host soluble beta-galactoside-binding lectins as damage-associated molecular patterns (DAMPs) and as receptors for pathogen-associated molecular patterns (PAMPs). Immunol Rev (2009) 230(1):172–87. doi:10.1111/j.1600-065X.2009.00790.x
298. Stowell SR, Arthur CM, Dias-Baruffi M, Rodrigues LC, Gourdine JP, Heimburg-Molinaro J, et al. Innate immune lectins kill bacteria expressing blood group antigen. Nat Med (2010) 16(3):295–301. doi:10.1038/nm.2103
299. Stowell SR, Arthur CM, McBride R, Berger O, Razi N, Heimburg-Molinaro J, et al. Microbial glycan microarrays define key features of host-microbial interactions. Nat Chem Biol (2014) 10(6):470–6. doi:10.1038/nchembio.1525
300. Thurston TL, Wandel MP, von Muhlinen N, Foeglein A, Randow F. Galectin 8 targets damaged vesicles for autophagy to defend cells against bacterial invasion. Nature (2012) 482(7385):414–8. doi:10.1038/nature10744
301. Feeley EM, Pilla-Moffett DM, Zwack EE, Piro AS, Finethy R, Kolb JP, et al. Galectin-3 directs antimicrobial guanylate binding proteins to vacuoles furnished with bacterial secretion systems. Proc Natl Acad Sci U S A (2017) 114(9):E1698–706. doi:10.1073/pnas.1615771114
302. Aragao KS, Satre M, Imberty A, Varrot A. Structure determination of discoidin II from Dictyostelium discoideum and carbohydrate binding properties of the lectin domain. Proteins (2008) 73(1):43–52. doi:10.1002/prot.22038
303. Mathieu SV, Aragao KS, Imberty A, Varrot A. Discoidin I from Dictyostelium discoideum and interactions with oligosaccharides: specificity, affinity, crystal structures, and comparison with discoidin II. J Mol Biol (2010) 400(3):540–54. doi:10.1016/j.jmb.2010.05.042
304. Barondes SH, Cooper DN, Haywood-Reid PL. Discoidin I and discoidin II are localized differently in developing Dictyostelium discoideum. J Cell Biol (1983) 96(1):291–6. doi:10.1083/jcb.96.1.291
305. Ray J, Shinnick T, Lerner R. A mutation altering the function of a carbohydrate binding protein blocks cell-cell cohesion in developing Dictyostelium discoideum. Nature (1979) 279(5710):215–21. doi:10.1038/279215a0
306. Alexander S, Shinnick TM, Lerner RA. Mutants of Dictyostelium discoideum blocked in expression of all members of the developmentally regulated discoidin multigene family. Cell (1983) 34(2):467–75. doi:10.1016/0092-8674(83)90380-X
307. Erdos GW, Whitaker D. Failure to detect immunocytochemically reactive endogenous lectin on the cell surface of Dictyostelium discoideum. J Cell Biol (1983) 97(4):993–1000. doi:10.1083/jcb.97.4.993
308. Stadler J, Bauer G, Westphal M, Gerisch G. Monoclonal antibody against cytoplasmic lectins of Dictyostelium discoideum: cross-reactivity with a membrane glycoprotein, contact site A, and with E. coli beta-galactosidase and lac repressor. Hoppe Seylers Z Physiol Chem (1984) 365(3):283–8. doi:10.1515/bchm2.1984.365.1.283
309. Xie P. TRAF molecules in cell signaling and in human diseases. J Mol Signal (2013) 8:7–7. doi:10.1186/1750-2187-8-7
310. Bradley JR, Pober JS. Tumor necrosis factor receptor-associated factors (TRAFs). Oncogene (2001) 20(44):6482–91. doi:10.1038/sj.onc.1204788
311. Grech A, Quinn R, Srinivasan D, Badoux X, Brink R. Complete structural characterisation of the mammalian and Drosophila TRAF genes: implications for TRAF evolution and the role of RING finger splice variants. Mol Immunol (2000) 37(12–13):721–34. doi:10.1016/S0161-5890(00)00098-5
312. Yin Q, Lin S-C, Lo Y-C, Damo SM, Wu H. Chapter 49 – tumor necrosis factor receptor-associated factors in immune receptor signal transduction A2 – Bradshaw, Ralph A. 2nd ed. In: Dennis EA, editor. Handbook of Cell Signaling. San Diego: Academic Press (2010). p. 339–45.
313. Haldar AK, Foltz C, Finethy R, Piro AS, Feeley EM, Pilla-Moffett DM, et al. Ubiquitin systems mark pathogen-containing vacuoles as targets for host defense by guanylate binding proteins. Proc Natl Acad Sci U S A (2015) 112(41):E5628–37. doi:10.1073/pnas.1515966112
314. Linares JF, Duran A, Yajima T, Pasparakis M, Moscat J, Diaz-Meco MT. K63 polyubiquitination and activation of mTOR by the p62-TRAF6 complex in nutrient-activated cells. Mol Cell (2013) 51(3):283–96. doi:10.1016/j.molcel.2013.06.020
315. Deretic V, Saitoh T, Akira S. Autophagy in infection, inflammation and immunity. Nat Rev Immunol (2013) 13(10):722–37. doi:10.1038/nri3532
316. Hatakeyama S. TRIM Family Proteins: Roles in Autophagy, Immunity, and Carcinogenesis. Trends Biochem Sci (2017) 42(4):297–311. doi:10.1016/j.tibs.2017.01.002
317. Reymond A, Meroni G, Fantozzi A, Merla G, Cairo S, Luzi L, et al. The tripartite motif family identifies cell compartments. EMBO J (2001) 20(9):2140–51. doi:10.1093/emboj/20.9.2140
318. Ozato K, Shin DM, Chang TH, Morse HC III. TRIM family proteins and their emerging roles in innate immunity. Nat Rev Immunol (2008) 8(11):849–60. doi:10.1038/nri2413
319. Kimura T, Jain A, Choi SW, Mandell MA, Schroder K, Johansen T, et al. TRIM-mediated precision autophagy targets cytoplasmic regulators of innate immunity. J Cell Biol (2015) 210(6):973–89. doi:10.1083/jcb.201503023
320. Mandell MA, Jain A, Arko-Mensah J, Chauhan S, Kimura T, Dinkins C, et al. TRIM proteins regulate autophagy and can target autophagic substrates by direct recognition. Dev Cell (2014) 30(4):394–409. doi:10.1016/j.devcel.2014.06.013
321. Kimura T, Mandell M, Deretic V. Precision autophagy directed by receptor regulators – emerging examples within the TRIM family. J Cell Sci (2016) 129(5):881–91. doi:10.1242/jcs.163758
322. Tabah AA, Tardif K, Mansky LM. Anti-HIV-1 activity of Trim 37. J Gen Virol (2014) 95(Pt 4):960–7. doi:10.1099/vir.0.057653-0
323. Kim BH, Shenoy AR, Kumar P, Bradfield CJ, MacMicking JD. IFN-inducible GTPases in host cell defense. Cell Host Microbe (2012) 12(4):432–44. doi:10.1016/j.chom.2012.09.007
324. Wu G, Chai J, Suber TL, Wu J-W, Du C, Wang X, et al. Structural basis of IAP recognition by Smac/DIABLO. Nature (2000) 408(6815):1008–12. doi:10.1038/35050012
325. Coers J, Haldar AK. Ubiquitination of pathogen-containing vacuoles promotes host defense to Chlamydia trachomatis and Toxoplasma gondii. Commun Integr Biol (2015) 8(6):e1115163. doi:10.1080/19420889.2015.1115163
326. MacMicking JD. Interferon-inducible effector mechanisms in cell-autonomous immunity. Nat Rev Immunol (2012) 12(5):367–82. doi:10.1038/nri3210
327. Meunier E, Broz P. Interferon-inducible GTPases in cell autonomous and innate immunity. Cell Microbiol (2016) 18(2):168–80. doi:10.1111/cmi.12546
328. Finethy R, Jorgensen I, Haldar AK, de Zoete MR, Strowig T, Flavell RA, et al. Guanylate binding proteins enable rapid activation of canonical and noncanonical inflammasomes in Chlamydia-infected macrophages. Infect Immun (2015) 83(12):4740–9. doi:10.1128/IAI.00856-15
329. Reich NC, Liu L. Tracking STAT nuclear traffic. Nat Rev Immunol (2006) 6(8):602–12. doi:10.1038/nri1885
330. Stark GR, Darnell JE Jr. The JAK-STAT pathway at twenty. Immunity (2012) 36(4):503–14. doi:10.1016/j.immuni.2012.03.013
331. Gatsios P, Terstegen L, Schliess F, Haussinger D, Kerr IM, Heinrich PC, et al. Activation of the Janus kinase/signal transducer and activator of transcription pathway by osmotic shock. J Biol Chem (1998) 273(36):22962–8. doi:10.1074/jbc.273.36.22962
332. Levy DE, Darnell JE Jr. Stats: transcriptional control and biological impact. Nat Rev Mol Cell Biol (2002) 3(9):651–62. doi:10.1038/nrm909
333. Casanova JL, Holland SM, Notarangelo LD. Inborn errors of human JAKs and STATs. Immunity (2012) 36(4):515–28. doi:10.1016/j.immuni.2012.03.016
334. Kawata T. STAT signaling in Dictyostelium development. Dev Growth Differ (2011) 53(4):548–57. doi:10.1111/j.1440-169X.2010.01243.x
335. Kawata T, Shevchenko A, Fukuzawa M, Jermyn KA, Totty NF, Zhukovskaya NV, et al. SH2 signaling in a lower eukaryote: a STAT protein that regulates stalk cell differentiation in Dictyostelium. Cell (1997) 89(6):909–16. doi:10.1016/S0092-8674(00)80276-7
336. Araki T, Kawata T, Williams JG. Identification of the kinase that activates a nonmetazoan STAT gives insights into the evolution of phosphotyrosine-SH2 domain signaling. Proc Natl Acad Sci U S A (2012) 109(28):E1931–7. doi:10.1073/pnas.1202715109
337. Araki T, Vu LH, Sasaki N, Kawata T, Eichinger L, Williams JG. Two Dictyostelium tyrosine kinase-like kinases function in parallel, stress-induced STAT activation pathways. Mol Biol Cell (2014) 25(20):3222–33. doi:10.1091/mbc.E14-07-1182
338. Early A, Gamper M, Moniakis J, Kim E, Hunter T, Williams JG, et al. Protein tyrosine phosphatase PTP1 negatively regulates Dictyostelium STATa and is required for proper cell-type proportioning. Dev Biol (2001) 232(1):233–45. doi:10.1006/dbio.2001.0171
339. Araki T, Langenick J, Gamper M, Firtel RA, Williams JG. Evidence that DIF-1 and hyper-osmotic stress activate a Dictyostelium STAT by inhibiting a specific protein tyrosine phosphatase. Development (2008) 135(7):1347–53. doi:10.1242/dev.009936
340. Araki T, Gamper M, Early A, Fukuzawa M, Abe T, Kawata T, et al. Developmentally and spatially regulated activation of a Dictyostelium STAT protein by a serpentine receptor. EMBO J (1998) 17(14):4018–28. doi:10.1093/emboj/17.14.4018
341. Fukuzawa M, Araki T, Adrian I, Williams JG. Tyrosine phosphorylation-independent nuclear translocation of a Dictyostelium STAT in response to DIF signaling. Mol Cell (2001) 7(4):779–88. doi:10.1016/S1097-2765(01)00222-2
342. Zhukovskaya NV, Fukuzawa M, Tsujioka M, Jermyn KA, Kawata T, Abe T, et al. Dd-STATb, a Dictyostelium STAT protein with a highly aberrant SH2 domain, functions as a regulator of gene expression during growth and early development. Development (2004) 131(2):447–58. doi:10.1242/dev.00927
343. Araki T, Tsujioka M, Abe T, Fukuzawa M, Meima M, Schaap P, et al. A STAT-regulated, stress-induced signalling pathway in Dictyostelium. J Cell Sci (2003) 116(Pt 14):2907–15. doi:10.1242/jcs.00501
344. Otto GP, Cocorocchio M, Munoz L, Tyson RA, Bretschneider T, Williams RS. Employing Dictyostelium as an advantageous 3Rs model for pharmacogenetic research. Methods Mol Biol (2016) 1407:123–30. doi:10.1007/978-1-4939-3480-5_9
345. Stallforth P, Brock DA, Cantley AM, Tian X, Queller DC, Strassmann JE, et al. A bacterial symbiont is converted from an inedible producer of beneficial molecules into food by a single mutation in the gacA gene. Proc Natl Acad Sci U S A (2013) 110(36):14528–33. doi:10.1073/pnas.1308199110
346. Chen X, Kollner TG, Jia Q, Norris A, Santhanam B, Rabe P, et al. Terpene synthase genes in eukaryotes beyond plants and fungi: occurrence in social amoebae. Proc Natl Acad Sci U S A (2016) 113(43):12132–7. doi:10.1073/pnas.1610379113
347. Klapper M, Gotze S, Barnett R, Willing K, Stallforth P. Bacterial alkaloids prevent amoebal predation. Angew Chem Int Ed Engl (2016) 55(31):8944–7. doi:10.1002/anie.201603312
348. Gotze S, Herbst-Irmer R, Klapper M, Gorls H, Schneider KRA, Barnett R, et al. Structure, biosynthesis, and biological activity of the cyclic lipopeptide anikasin. ACS Chem Biol (2017) 12(10):2498–502. doi:10.1021/acschembio.7b00589
349. Brock DA, Read S, Bozhchenko A, Queller DC, Strassmann JE. Social amoeba farmers carry defensive symbionts to protect and privatize their crops. Nat Commun (2013) 4:2385. doi:10.1038/ncomms3385
350. DiSalvo S, Haselkorn TS, Bashir U, Jimenez D, Brock DA, Queller DC, et al. Burkholderia bacteria infectiously induce the proto-farming symbiosis of Dictyostelium amoebae and food bacteria. Proc Natl Acad Sci U S A (2015) 112(36):E5029–37. doi:10.1073/pnas.1511878112
351. Brock DA, Callison WE, Strassmann JE, Queller DC. Sentinel cells, symbiotic bacteria and toxin resistance in the social amoeba Dictyostelium discoideum. Proc Biol Sci (2016) 283:1829. doi:10.1098/rspb.2015.2727
352. Smith J, Queller DC, Strassmann JE. Fruiting bodies of the social amoeba Dictyostelium discoideum increase spore transport by Drosophila. BMC Evol Biol (2014) 14:105. doi:10.1186/1471-2148-14-105
Keywords: Dictyostelium discoideum, cell-autonomous defense, phagocytosis, autophagy, metal poisoning, nutritional immunity, mononuclear phagocyte system, host–pathogen interactions
Citation: Dunn JD, Bosmani C, Barisch C, Raykov L, Lefrançois LH, Cardenal-Muñoz E, López-Jiménez AT and Soldati T (2018) Eat Prey, Live: Dictyostelium discoideum As a Model for Cell-Autonomous Defenses. Front. Immunol. 8:1906. doi: 10.3389/fimmu.2017.01906
Received: 26 September 2017; Accepted: 13 December 2017;
Published: 04 January 2018
Edited by:
Geanncarlo Lugo-Villarino, UMR5089 Institut de Pharmacologie et de Biologie Structurale (IPBS), FranceReviewed by:
Pierre Stallforth, Leibniz-Institut für Naturstoff-Forschung und Infektionsbiologie, Hans Knöll Institut, GermanyChristopher West, University of Georgia, United States
Salvatore Bozzaro, Università degli Studi di Torino, Italy
Copyright: © 2018 Dunn, Bosmani, Barisch, Raykov, Lefrançois, Cardenal-Muñoz, López-Jiménez and Soldati. This is an open-access article distributed under the terms of the Creative Commons Attribution License (CC BY). The use, distribution or reproduction in other forums is permitted, provided the original author(s) or licensor are credited and that the original publication in this journal is cited, in accordance with accepted academic practice. No use, distribution or reproduction is permitted which does not comply with these terms.
*Correspondence: Joe Dan Dunn, am9lZGFuLmR1bm4mI3gwMDA0MDt1bmlnZS5jaA==