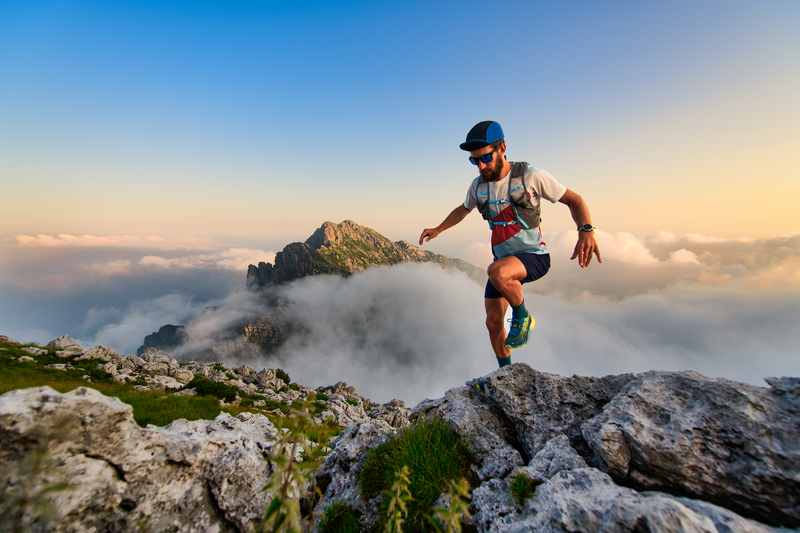
94% of researchers rate our articles as excellent or good
Learn more about the work of our research integrity team to safeguard the quality of each article we publish.
Find out more
REVIEW article
Front. Immunol. , 22 December 2017
Sec. Inflammation
Volume 8 - 2017 | https://doi.org/10.3389/fimmu.2017.01898
This article is part of the Research Topic Regulation of Inflammation in Chronic Disease View all 48 articles
Inflammation is typically induced in response to a microbial infection. The release of proinflammatory cytokines enhances the stimulatory capacity of antigen-presenting cells, as well as recruits adaptive and innate immune effectors to the site of infection. Once the microbe is cleared, inflammation is resolved by various mechanisms to avoid unnecessary tissue damage. Autoimmunity arises when aberrant immune responses target self-tissues causing inflammation. In type 1 diabetes (T1D), T cells attack the insulin producing β cells in the pancreatic islets. Genetic and environmental factors increase T1D risk by in part altering central and peripheral tolerance inducing events. This results in the development and expansion of β cell-specific effector T cells (Teff) which mediate islet inflammation. Unlike protective immunity where inflammation is terminated, autoimmunity is sustained by chronic inflammation. In this review, we will highlight the key events which initiate and sustain T cell-driven pancreatic islet inflammation in nonobese diabetic mice and in human T1D. Specifically, we will discuss: (i) dysregulation of thymic selection events, (ii) the role of intrinsic and extrinsic factors that enhance the expansion and pathogenicity of Teff, (iii) defects which impair homeostasis and suppressor activity of FoxP3-expressing regulatory T cells, and (iv) properties of β cells which contribute to islet inflammation.
Type 1 diabetes (T1D) is an autoimmune disease characterized by the chronic inflammation of the pancreatic islets of Langerhans (1–4). Islet inflammation is typically marked by infiltrating adaptive and innate immune effectors. Insulitis progresses over time and when a sufficient amount of β cell mass has been rendered nonfunctional and/or destroyed, hyperglycemic blood levels are achieved, and clinical diabetes established. The immune mechanisms mediating β cell autoimmunity are heterogeneous, as reflected by the nature of the islet infiltrate and the age of clinical onset. Nevertheless, T1D is generally viewed as a T cell-driven autoimmune disease, particularly for the more prevalent and aggressive type of T1D that develops in children and adolescents versus adults (5–17). A T cell-independent subtype of T1D, however, may also exist that is thought to be largely mediated by innate immune effectors (18, 19). The events leading to the loss of β cell-specific tolerance and chronic islet inflammation are complex, and influenced by both genetic and environmental factors (20–22).
Type 1 diabetes is polygenic with more than 20 insulin-dependent diabetes mellitus (IDDM) genetic loci identified that are associated with increased or decreased risk for T1D (23–28). The strongest genetic association is with the human leukocyte antigen locus (IDDM1), and particular class I and II haplotypes, consistent with a key role for T cells in T1D (29, 30). A number of genes regulating T, B, and innate cell immunobiology are also linked with T1D, as are genetic variants intrinsic to β cells, which deleteriously affect β cell function and/or responses to inflammation (31–37).
The identity and role of environmental factors in T1D are poorly understood. The most common hypothesis is that microbial infections initiate and/or exacerbate islet inflammation in genetically susceptible individuals (38, 39). For instance, T1D is associated with enteroviruses such as coxsackievirus B1 (40–44). Viral infection of β cells may result in direct cytolysis and/or elicit local inflammation that initiates and/or drives autoimmunity (45–47). The gut microbiota also has a profound regulatory effect on β cell autoimmunity (48, 49). In the nonobese diabetic (NOD) mouse, a spontaneous model of T1D, β cell destruction can be either promoted or prevented by changes in the composition of the gut microbiota (50, 51). Here bacterial components and metabolites are thought to impact the activation and/or differentiation status of innate and adaptive immune effectors. Longitudinal studies of at risk subjects also indicate a role for gut microbiota in human T1D (52–54).
The T cell-related events that drive chronic islet inflammation in T1D stem from dysregulation of central and peripheral tolerance, alterations in self-antigen processing, and modified β cell responses (Figure 1). Here, we discuss how events critical for initiating and amplifying the development of T cell-mediated islet inflammation are regulated in NOD mice and human T1D.
Figure 1. Dysregulated thymic and peripheral events culminate in chronic islet inflammation. In general, overt diabetes results from the gradual loss of functional insulin producing β cells due to the inflammatory environment driven by infiltrating self-reactive T cells and antigen-presenting cell (APC). Although β cell-specific T cell clones are detected in both healthy and type 1 diabetes (T1D) susceptible individuals, a number of factors promote T1D development in the latter population. Decreased efficiency of negative selection in the thymus, either due to altered tissue-specific antigen expression or due to T cell receptor (TCR) signaling, allows for the increased escape of β cell-specific T cell clones into the periphery. In addition, β cell-specific Foxp3+Treg development may also be suboptimal due to dysregulation of TCR signaling. In the periphery, β cell-specific T cells are stimulated in the pancreatic lymph nodes (pLN) by APC derived from the islets, leading to effector T cell (Teff) differentiation. These pathogenic Teff then infiltrate the islets and drive inflammation leading to reduced β cell function and/or survival. Not all islets are infiltrated potentially due to an immature phenotype and reduced autoantigen expression by β cells. Ongoing islet inflammation also leads to the generation of neoautoantigens either directly in β cells or during antigen processing by APC. The presentation of neoautoantigens within the pLN promotes the activation and expansion of additional Teff pools. These events amplify and drive a chronic state of islet inflammation leading to impaired functional β cell mass and clinical onset of T1D.
The generation of an autoreactive T cell receptor (TCR) repertoire in the periphery is established in part by inefficient negative selection of anti-self-single positive thymocytes (SP) in the thymus (55, 56). Early in ontogeny negative selection is lax, resulting in increased escape of anti-self-SP (57–59). This temporal decrease in negative selection and elevated survival of β cell-specific clonotypes may help explain the predominance of T1D onset in childhood. With time, changes in thymic structural organization and maturation of thymic antigen-presenting cells (APC) leads to more efficient negative selection and increased death of autoreactive SP (57).
Key mediators of negative selection are medullary thymic epithelial cells (mTEC) and dendritic cells (DC). Notably, mTEC express and present several tissue-specific antigens (TSA) (60–63). Recognition of MHC-self-peptide complexes with increasing avidity/affinity results in elevated TCR signaling and SP apoptosis. Dysregulation of negative selection generates a peripheral pool of anti-self-T cells displaying increased avidity/affinity, and likely an enhanced “pathogenic potential.”
Parameters influencing the efficiency of negative selection both intrinsic and extrinsic to thymocytes have been linked to the development of β cell-specific T cells and T1D. Thymocyte intrinsic properties reported in NOD mice have included reduced SP sensitivity to apoptosis and altered double positive thymocyte differentiation to SP (64–67). In humans, TCR signaling needed to drive apoptosis of β cell-specific SP may be limited by a T1D-associated variant of the protein tyrosine phosphatase non-receptor 22 (PTPN22) gene (31, 32). PTPN22 is a negative regulator of TCR signaling, and elevated phosphatase activity by PTPN22 is predicted to reduce TCR signaling strength and diminish apoptosis induction in SP (68). An increase in PTPN22 activity may also limit thymic development of β cell-specific FoxP3-expressing regulatory CD4+ T cells (FOXP3+Treg), which is dependent on high(er) avidity/affinity recognition of self-peptide.
Thymocyte extrinsic factors that impact negative selection include aberrant expression of TSA in the medulla. The importance of thymic expression and presentation of TSA is readily evident in mice and humans deficient of the transcription factor autoimmune regulator (AIRE) (60, 69). Lack of AIRE, which drives expression of select TSA by mTEC, results in inefficient thymic negative selection and reduced development of tissue-specific Foxp3+Treg, leading to multiorgan autoimmunity in mice (70–74). Similarly, aberrant AIRE expression and function in humans results in the development of autoimmune polyendorinology candidiasis and ectodermal dysplasia (APECD) in which a variety of organs are targeted by T cells; notably a subset of APCED patients develop T1D (75, 76). Reduced AIRE expression has been reported in NOD mice, which reflects not only T1D development but also T cell-mediated inflammation of other tissues such as the thyroid, salivary, and lacrimal glands (77).
In human T1D, a strong genetic association is linked to the insulin encoding gene INS2 found in IDDM2 (78). Insulin is believed to be a key autoantigen driving human T1D, which is supported by studies in NOD mice (79–81). INS2 is preceded by a variable number of tandem repeats (VNTRs). Individuals that have 26–63 VNTRs, associated with decreased thymic INS2 expression, have an increased risk of developing T1D. In contrast, INS2 expression is increased with VNTRs ranging between 140 and 210, which in turn is associated with a protective phenotype (82, 83). Reduced thymic insulin expression is expected to both limit negative selection and development of insulin-specific SP and FOXP3+Treg, respectively. Future studies are needed to directly demonstrate that thymic selection is dysregulated, and contributes to an expanded β cell-specific peripheral T cell pool in human T1D. Whether defects in thymic selection and development of β cell-specific T cells are necessary only early on or required throughout the disease process is another issue that needs to be tackled.
It is noteworthy that β cell-specific T cells are detected in the blood of healthy individuals, likely reflecting in part the reduced efficiency of thymic negative selection early in ontogeny. However, the phenotype of circulating β cell-specific T cells is distinct in T1D patients versus healthy subjects (84–89). The former exhibit mostly an effector/memory phenotype and expression of proinflammatory cytokines consistent with ongoing β cell autoimmunity (84–88). These findings indicate that in addition to the TCR repertoire, other factors contribute to the differentiation and expansion of diabetogenic effector T cells (Teff). For instance, the extent of tissue destruction and lethality of AIRE deficiency in mice is influenced by genotype with AIRE-deficient NOD versus C57BL/6 mice exhibiting more severe systemic autoimmunity (90, 91). Additionally, distinct TCR repertoires have been found in NOD mice in contrast to MHC matched C57BL/6 mice (92). Overall, dysregulation of thymic selection events in NOD mice acts as a precursor for islet inflammation.
The initiation of islet inflammation in NOD mice and humans is ill-defined. In NOD mice pancreatic remodeling shortly after birth is thought to play a key role starting the diabetogenic response (93, 94). Remodeling of the pancreas results in a wave of β cell apoptosis and release of antigens which are endocytosed by resident macrophages and DC (95). These APC then traffick to the draining pancreatic lymph nodes (pLN) to prime β cell-specific T cells and promote Teff differentiation (96, 97). Once established Teff migrate into the islets and mediate inflammation (97–99).
As alluded to above, shifts in the composition of the gut microbiota early in ontogeny are also believed to play a key role in regulating Teff differentiation in both mice and humans. Systemic release of microbiota-derived products can activate APC that in turn prime β cell-specific T cells providing an “environmental trigger” to incite T1D development (48). NOD mice in which the response to the microbiome is limited due to a deficiency in the Toll-like receptor adaptor protein MyD88, exhibit reduced β cell-specific Teff reactivity and diabetes incidence (50, 100). Strikingly, diabetes is prevented in NOD mice housed under germ-free conditions and inoculated with microbiota derived from MyD88-deficient animals (50), demonstrating that the microbiota also has a protective role in T1D. A less diverse gut microbiota in young individuals at risk for T1D is associated with progression to clinical diabetes (54). Changes in the gut microbiome have also been linked to the female bias of T1D in NOD mice (100). Interestingly, studies show that the lymphopenic environment in neonatal mice induces naïve T cells to rapidly expand and transition into a memory-like phenotype, that in turn is influenced by gut microbiota (101–104). Expansion of memory-like T cells, also seen in newborn humans, may enhance the pathogenic potential of the peripheral T cell pool and favor the development of autoimmunity in susceptible individuals.
Both CD4+ and CD8+ T cells are required for efficient β cell destruction in NOD mice (105). Islet CD8+ T cells primarily mediate β cell destruction by a cognate interaction involving perforin and granzyme B-, and Fas-Fas ligand-mediated killing (106, 107). On the other hand, islet CD4+ T cells drive β cell destruction in a bystander manner via secretion of proinflammatory cytokines. CD4+ and CD8+ T cells are also detected in the islets of diabetic subjects, with CD8+ T cells often predominating (6, 106). Several β cell autoantigens are recognized by the islet infiltrating T cells, and a number of these are similarly targeted in both the NOD and human diabetogenic responses including glutamic acid decarboxylase 65, proinsulin, insulin B chain, islet antigen-2, and islet-specific glucose-6-phosphatase catalytic subunit-related protein (108).
The majority of CD4+ and CD8+ T cells infiltrating the islets of NOD mice and T1D subjects exhibit a T helper 1 (Th1) effector phenotype, marked by IFNγ secretion (109). Increased Th17 cells are seen in the islets of NOD mice and the pLN of T1D subjects (109–111). The role of Th17 cells in mediating islet inflammation, however, is ill-defined. Elevated local levels of IFNγ are believed to establish a feed-forward loop that drives islet pathology. Based on NOD mouse studies, IFNγ secreted by islet CD4+ (and CD8+) Teff results in local upregulation of chemotactic cues that induce additional T, B, and innate cells to migrate into the islets, as well as promote islet retention of these effectors (109, 112). IFNγ also activates islet resident APC and stromal cells to elevate production of additional inflammatory mediators, such reactive oxygen species, which impair function and mediate β cell necrosis (107, 113, 114). Furthermore, IFNγ in the context of IL-1β and TNFα induces β cell apoptosis (113, 114).
Another proinflammatory cytokine thought to contribute to islet inflammation is IL-21 which is elevated in T1D patient serum (115). Notably, the murine IL-21 gene is located in the Idd3 locus and IL-21 receptor (R) deficiency prevents T1D in NOD mice (116). CD4+ T follicular helper cells, which are increased in the pLN of NOD mice, are the primary source of IL-21 (112, 117–119). IL-21 has a critical role in supporting B cell development and antibody production. B cells, serving as APC, are required for efficient β cell destruction in NOD mice and likely in human T1D (117, 118, 120, 121). IL-21 also enhances maintenance of CD8+ Teff by preventing exhaustion during chronic inflammation (122, 123). Interestingly, the pathogenicity of β cell-specific CD8+ T cells is dependent on IL-21R expression (124, 125).
Defects intrinsic to Teff are also thought to facilitate chronic islet inflammation. Variants of the CTLA4 gene are linked to T1D susceptibility in both NOD mice (Idd5.1) and human T1D (IDDM12) (33, 126). CTLA-4 which binds to the costimulatory molecules CD80 and CD86 expressed on APC, is a negative regulator of T cell activation and proliferation (34). Polymorphisms in the human CTLA4 gene region are associated with reduced mRNA levels and a decrease in expression of the soluble (s) CTLA-4 isoform (33, 34, 126). sCTLA-4 also negatively regulates TCR signaling (33, 34, 126). Reduced expression of CTLA-4 and sCTLA-4 is expected to facilitate expansion of β cell-specific T cells. This scenario is consistent with the exacerbated β cell autoimmunity seen in NOD mice expressing a diabetogenic TCR transgene and lacking CTLA-4 expression (127). Noteworthy is that both NOD-derived and human T1D Teff also exhibit reduced sensitivity to Foxp3+Treg-mediated suppression (128, 129). In sum, the culmination of a variety of extrinsic and intrinsic factors enables Teff to expand, persist, and in turn amplify islet inflammation.
In addition to Teff that are resistant to regulatory mechanisms that limit expansion and function, evidence indicates that the Foxp3+Treg pool is compromised in T1D (130, 131). Here, dysregulation of Foxp3+Treg homeostasis is thought to permit preferential differentiation and expansion of pathogenic β cell-specific Teff. Foxp3+Treg have an essential role in regulating immune homeostasis and reactivity to self (132–135). The lack of thymic development of Foxp3+Treg due to deficient expression or function of the FoxP3 transcription factor, results in systemic autoimmunity in both mice and humans. Foxp3+Treg mediate suppression of T cells and other immune effectors via multiple mechanisms including cell-contact dependent suppression, and secretion of anti-inflammatory cytokines and mediators such as IL-10, TGFβ1, and IL-35, and adenosine, respectively (136). Foxp3+Treg also function as an “IL-2 depot” to deprive Teff of IL-2 needed for expansion (136). The latter is mediated by constitutive expression of CD25, the α subunit of the IL-2R (136). Therefore, Foxp3+Treg, expressing the high affinity IL-2R, are able to out compete Teff for IL-2, which transiently express high affinity IL-2R.
IL-2 is essential for Foxp3+Treg homeostasis, expansion, and function (136). Unlike conventional T cells, Foxp3+Treg do not produce IL-2 due to FoxP3-mediated negative regulation of Il2 transcription. Therefore, Foxp3+Treg are dependent on T cells and DC as IL-2 sources (136). This dependency is thought to enable Foxp3+Treg to more readily sense and respond to ongoing inflammation. Accordingly, defects in the IL-2/IL-2R axis have been described in the NOD model and human T1D (137–142). In NOD mice, an Il2 variant located in Idd3 results in reduced levels of IL-2 expression by Teff, and impaired survival and function of islet resident Foxp3+Treg (130, 143). Increased levels of proinflammatory cytokines, such as IFNγ and IL-6 that downregulate FoxP3 expression may also promote dedifferentiation of islet Foxp3+Treg into a Teff-like subsets (144, 145). These events lead to a progressive loss of islet Foxp3+Treg suppression, thereby “releasing the brakes” and favoring pathogenic Teff expansion.
The frequency of FOXP3+Treg found in blood is largely unaffected in T1D subjects (140, 141, 146–148). However, FOXP3+Treg from T1D subjects exhibit reduced suppressor function measured in vitro (128, 129). This aberrant activity is linked to T1D risk variants of IL2RA (CD25) and PTPN2, a phosphatase involved in IL-2R signaling (149). Notably, FOXP3+Treg expressing these variants display reduced IL-2R signaling that in turn correlates with limited suppressor activity (149, 150). Defects in IL-2R signaling have led to clinical studies testing whether low-dose IL-2 therapy enhances the FOXP3+Treg pool in T1D subjects (149, 151). This approach has been effective in preventing and/or reversing diabetes in NOD mice by increasing the number and function of islet Foxp3+Treg (149, 152). One key question not addressed is the specificity of FOXP3+Treg in T1D subjects. Reduced thymic development of β cell-specific FOXP3+Treg, as discussed above, would be expected to limit the “anti-diabetogenic” effects of the peripheral FOXP3+Treg pool.
Recent findings have demonstrated that the proinflammatory milieu of the islets promotes processing of “neoautoantigens” (153). Importantly, these neoautoantigens are only found in the periphery so that corresponding T cell clonotypes, not deleted in the thymus and possibly expressing high affinity TCR, can be recruited into the inflammatory response. Neoautoantigens are generated via posttranslational modifications (PTM), such as deamidation by tissue transglutaminase (tTG) (153). PTM can occur during APC antigen processing or directly in β cells (154). tTG-dependent deamidation of a proinsulin C-peptide for instance is detected in both human DC and islets under inflammatory conditions (154). Notably the resulting modified peptide is recognized by CD4+ T cells derived from T1D subjects. The MHC binding and in turn T cell stimulatory properties of peptides can also be enhanced by deamidation (155).
Neoautoantigens consisting of hybrid peptides have recently been identified. In NOD mice hybrid insulin peptides are generated via covalent crosslinking of a proinsulin C peptide with peptides derived from naturally occurring cleavage products produced in the β cell secretory granules (156). In addition to ongoing inflammation, PTM occurs via endoplasmic reticulum stress, which can be induced in β cells by the normal physiological demands associated with high levels of insulin secretion (157). Therefore, it is possible that β cell neoautoantigens in addition to amplifying inflammation, play a role in initiating the diabetogenic response. Neoautoantigens are also generated at a transcriptional level. A mutation in the open reading frame of insulin mRNA generates a neoautoantigen that stimulates CD8+ T cells from T1D subjects causing β cells lysis in vitro (158). Alternative RNA splicing may be another mechanism leading to neoautoantigen expression, particularly since ~30% of genes in inflamed β cells undergo aberrant alternative splicing (159). In sum, β cell neoautoantigens serve as bona fide targets of pathogenic CD4+ and CD8+ T cells. The breadth of the peptidome of neoautoantigens produced and presented, and the properties of neoautoantigen-specific T cells, in terms of frequency, avidity/affinity, subset phenotype (e.g., pathogenic versus regulatory), and overall contribution to islet inflammation require further investigation.
Studies have demonstrated that intrinsic properties of β cells also influence islet inflammation. For instance, CXCL10 is produced by β cells although the role of this chemokine in disease is controversial (160). CXCL10 regulates migration of CXCR3 expressing Teff and Foxp3+Treg into the islets (97–99, 161). Overexpression of CXCL10 in β cells accelerates T1D progression, and antibody blockade of CXCL10 prevents Teff migration into the islets of NOD mice (97–99, 161). On the other hand, Cxcr3 deficiency accelerates T1D by reducing islet resident Foxp3+Treg (162, 163). Therefore, depending on the context, β cells may affect inflammatory and immunoregulatory events via CXCL10 production. The chemokine CCL2 is also secreted by β cells, and over-expression of ectopic CCL2 recruits tolerogenic CCR2-expressing DC and blocks T1D progression in NOD mice (164). Interestingly, NOD APC shows defective migration in response to CCL2, and human T1D patients have reduced serum levels of CCL2 (164–166). Additionally, β cells produce CXCL1 and CXCL2 that recruit CXCR2-expressing neutrophils to the islets, which contribute to stimulating β cell-specific T cell reactivity (167, 168). Overall, β cell produced chemotactic cues regulate the progression of the diabetogenic response.
Along with chemokines, β cells secrete the cytokine IL-1β, which at low levels promotes β cell proliferation, and enhances production of CCL2, CXCL1, CXCL2, and insulin (169, 170). However, IL-1β also primes leukocyte effector-mediated inflammation, and as noted above, IL-1β in the context of TNFα, and/or IFN-γ induces β cell apoptosis in vitro (113, 114). Notably, glucagon-secreting α cells also produce IL-1β, indicating that other islet resident endocrine cells may also contribute to local inflammation (171, 172). Islet inflammation also induces upregulation of MHC class I and II on β cells to further increase β cell immunogenicity (173). Interestingly, a subpopulation of β cells have been identified which under inflammatory conditions acquires resistance to immune-mediated destruction in NOD mice (174). The latter is associated with a more immature β cell phenotype coupled with reduced expression of autoantigens and upregulation of immunomodulatory molecules such as PD-L1, an inducer of T cell exhaustion. A similar phenotype is seen for human β cells (174). Therefore, β cells not only contribute to islet inflammation but also adapt under the inflammatory conditions in order to persist. A better understanding of the events regulating this dichotomy has important implications for the treatment of T1D patients via β cell replacement strategies for instance.
Type 1 diabetes is complex involving genetic, epigenetic and environmental factors that influence adaptive and innate effector cell populations, which ultimately culminate in pathological, chronic islet inflammation (Figure 1). The heterogeneity associated with human T1D and in turn the nature of islet inflammation is expected to reflect the genotype of the individual, and type of environmental insult(s) encountered (20–22). These factors dictate which immune effectors are the key drivers of pathology, the pace of disease progression, and the degree of β cell dysregulation and/or death. We propose that the rapid and aggressive T1D seen early in life is marked by a broad β cell-specific TCR repertoire with increased avidity/affinity due to insufficient negative selection (57–59). This is coupled with β cell-specific Teff that are insensitive to peripheral tolerance inducing events, β cell-specific FOXP3+Treg with impaired suppressor activity, and β cells which readily promote islet inflammation (128, 129, 147, 169, 170). Robust inflammation leads to increased β cell neoautoantigen production further amplifying the kinetics and overall inflammatory response (153, 154, 156, 158). Under these “ideal” conditions, early onset T1D develops. On the other hand, in individuals with only a partial complement of these key “disease components,” islet inflammation is less robust and the kinetics of T1D onset proportionately delayed. Defining the events driving early versus late(r) T1D onset is critical for a better understanding of how islet inflammation is regulated in humans. The latter is also important for developing rational and effective immunotherapies to prevent and/or treat T1D. Devising strategies to enhance thymic negative selection early in ontogeny for instance, would be expected to purge the diabetogenic TCR repertoire to prevent T1D. Indeed, approaches are currently being studied to manipulate thymic negative selection in the context of cancer treatment by expanding the T cell repertoire specific for self-tumor antigens (175–177). Altering the gut microbiome early in life may also prove to be an effective strategy to limit expansion of the anti-self-T cell repertoire and establish robust immunoregulation in the periphery. Administration of β cell neoautoantigens may augment the efficacy of antigen-based immunotherapies to block disease progression at later stages of T1D (157). Depending on the mode of administration, β cell neoautoantigens can be used to target the corresponding clonotypes by tolerizing pathogenic Teff and/or inducing/expanding FOXP3+Treg. In view of the heterogeneity in the immunopathology of T1D, it is very likely these approaches and others currently being studied will need to be combined to effectively suppress the chronic islet inflammation and β cell autoimmunity long term.
MC, CJK, and RMT contributed to the preparation of the review article.
The authors have no personal, professional, or financial relationships that are considered to be a conflict of interest.
This work was supported by National Institutes of Health grants R01DK100256 and R01DK1035486 (RMT) and T32AI007273 (MC).
1. Bach JF. Insulin-dependent diabetes mellitus as an autoimmune disease. Endocr Rev (1994) 15(4):516–42. doi:10.1210/edrv-15-4-516
2. Tisch R, McDevitt H. Insulin-dependent diabetes mellitus. Cell (1996) 85(3):291–7. doi:10.1016/S0092-8674(00)81106-X
3. Eisenbarth GS. Type 1 diabetes: molecular, cellular and clinical immunology. Adv Exp Med Biol (2004) 552:306–10.
4. Anderson MS, Bluestone JA. The NOD mouse: a model of immune dysregulation. Annu Rev Immunol (2005) 23:447–85. doi:10.1146/annurev.immunol.23.021704.115643
5. Pugliese A. Autoreactive T cells in type 1 diabetes. J Clin Invest (2017) 127(8):2881–91. doi:10.1172/JCI94549
6. Coppieters KT, Dotta F, Amirian N, Campbell PD, Kay TW, Atkinson MA, et al. Demonstration of islet-autoreactive CD8 T cells in insulitic lesions from recent onset and long-term type 1 diabetes patients. J Exp Med (2012) 209(1):51–60. doi:10.1084/jem.20111187
7. Atkinson MA, von Herrath M, Powers AC, Clare-Salzler M. Current concepts on the pathogenesis of type 1 diabetes – considerations for attempts to prevent and reverse the disease. Diabetes Care (2015) 38(6):979–88. doi:10.2337/dc15-0144
8. Arif S, Gibson VB, Nguyen V, Bingley PJ, Todd JA, Guy C, et al. Beta-cell specific T-lymphocyte response has a distinct inflammatory phenotype in children with Type 1 diabetes compared with adults. Diabet Med (2017) 34(3):419–25. doi:10.1111/dme.13153
9. Heninger AK, Eugster A, Kuehn D, Buettner F, Kuhn M, Lindner A, et al. A divergent population of autoantigen-responsive CD4+ T cells in infants prior to beta cell autoimmunity. Sci Transl Med (2017) 9(378):eaaf8848. doi:10.1126/scitranslmed.aaf8848
10. Willcox A, Richardson SJ, Bone AJ, Foulis AK, Morgan NG. Analysis of islet inflammation in human type 1 diabetes. Clin Exp Immunol (2009) 155(2):173–81. doi:10.1111/j.1365-2249.2008.03860.x
11. Atkinson MA, Gianani R. The pancreas in human type 1 diabetes: providing new answers to age-old questions. Curr Opin Endocrinol Diabetes Obes (2009) 16(4):279–85. doi:10.1097/MED.0b013e32832e06ba
12. Coppieters KT, Roep BO, von Herrath MG. Beta cells under attack: toward a better understanding of type 1 diabetes immunopathology. Semin Immunopathol (2011) 33(1):1–7. doi:10.1007/s00281-010-0236-6
13. Richardson SJ, Willcox A, Bone AJ, Morgan NG, Foulis AK. Immunopathology of the human pancreas in type-I diabetes. Semin Immunopathol (2011) 33(1):9–21. doi:10.1007/s00281-010-0205-0
14. Campbell-Thompson M. Organ donor specimens: what can they tell us about type 1 diabetes? Pediatr Diabetes (2015) 16(5):320–30. doi:10.1111/pedi.12286
15. Campbell-Thompson M, Fu A, Kaddis JS, Wasserfall C, Schatz DA, Pugliese A, et al. Insulitis and beta-cell mass in the natural history of type 1 diabetes. Diabetes (2016) 65(3):719–31. doi:10.2337/db15-0779
16. Babon JA, DeNicola ME, Blodgett DM, Crevecoeur I, Buttrick TS, Maehr R, et al. Analysis of self-antigen specificity of islet-infiltrating T cells from human donors with type 1 diabetes. Nat Med (2016) 22(12):1482–7. doi:10.1038/nm.4203
17. Michels AW, Landry LG, McDaniel KA, Yu L, Campbell-Thompson M, Kwok WW, et al. Islet-derived CD4 T cells targeting proinsulin in human autoimmune diabetes. Diabetes (2017) 66(3):722–34. doi:10.2337/db16-1025
18. In’t Veld P. Insulitis in human type 1 diabetes: the quest for an elusive lesion. Islets (2011) 3(4):131–8. doi:10.4161/isl.3.4.15728
19. Skog O, Korsgren S, Melhus A, Korsgren O. Revisiting the notion of type 1 diabetes being a T-cell-mediated autoimmune disease. Curr Opin Endocrinol Diabetes Obes (2013) 20(2):118–23. doi:10.1097/MED.0b013e32835edb89
20. van Belle TL, Coppieters KT, von Herrath MG. Type 1 diabetes: etiology, immunology, and therapeutic strategies. Physiol Rev (2011) 91(1):79–118. doi:10.1152/physrev.00003.2010
21. Atkinson MA. The pathogenesis and natural history of type 1 diabetes. Cold Spring Harb Perspect Med (2012) 2(11):a007641. doi:10.1101/cshperspect.a007641
22. Katsarou A, Gudbjornsdottir S, Rawshani A, Dabelea D, Bonifacio E, Anderson BJ, et al. Type 1 diabetes mellitus. Nat Rev Dis Primers (2017) 3:17016. doi:10.1038/nrdp.2017.16
23. Barrett JC, Clayton DG, Concannon P, Akolkar B, Cooper JD, Erlich HA, et al. Genome-wide association study and meta-analysis find that over 40 loci affect risk of type 1 diabetes. Nat Genet (2009) 41(6):703–7. doi:10.1038/ng.381
24. Concannon P, Erlich HA, Julier C, Morahan G, Nerup J, Pociot F, et al. Type 1 diabetes: evidence for susceptibility loci from four genome-wide linkage scans in 1,435 multiplex families. Diabetes (2005) 54(10):2995–3001. doi:10.2337/diabetes.54.10.2995
25. Pociot F, Akolkar B, Concannon P, Erlich HA, Julier C, Morahan G, et al. Genetics of type 1 diabetes: what’s next? Diabetes (2010) 59(7):1561–71. doi:10.2337/db10-0076
26. Pociot F, McDermott MF. Genetics of type 1 diabetes mellitus. Genes Immun (2002) 3(5):235–49. doi:10.1038/sj.gene.6363875
27. Noble JA, Erlich HA. Genetics of type 1 diabetes. Cold Spring Harb Perspect Med (2012) 2(1):a007732. doi:10.1101/cshperspect.a007732
28. Onengut-Gumuscu S, Chen WM, Burren O, Cooper NJ, Quinlan AR, Mychaleckyj JC, et al. Fine mapping of type 1 diabetes susceptibility loci and evidence for colocalization of causal variants with lymphoid gene enhancers. Nat Genet (2015) 47(4):381–6. doi:10.1038/ng.3245
29. Singal DP, Blajchman MA. Histocompatibility (HL-A) antigens, lymphocytotoxic antibodies and tissue antibodies in patients with diabetes mellitus. Diabetes (1973) 22(6):429–32. doi:10.2337/diab.22.6.429
30. Nerup J, Platz P, Andersen OO, Christy M, Lyngsoe J, Poulsen JE, et al. HL-A antigens and diabetes mellitus. Lancet (1974) 2(7885):864–6. doi:10.1016/S0140-6736(74)91201-X
31. Bottini N, Musumeci L, Alonso A, Rahmouni S, Nika K, Rostamkhani M, et al. A functional variant of lymphoid tyrosine phosphatase is associated with type I diabetes. Nat Genet (2004) 36(4):337–8. doi:10.1038/ng1323
32. Smyth D, Cooper JD, Collins JE, Heward JM, Franklyn JA, Howson JM, et al. Replication of an association between the lymphoid tyrosine phosphatase locus (LYP/PTPN22) with type 1 diabetes, and evidence for its role as a general autoimmunity locus. Diabetes (2004) 53(11):3020–3. doi:10.2337/diabetes.53.11.3020
33. Nistico L, Buzzetti R, Pritchard LE, Van der Auwera B, Giovannini C, Bosi E, et al. The CTLA-4 gene region of chromosome 2q33 is linked to, and associated with, type 1 diabetes. Belgian Diabetes Registry. Hum Mol Genet (1996) 5(7):1075–80. doi:10.1093/hmg/5.7.1075
34. Ueda H, Howson JM, Esposito L, Heward J, Snook H, Chamberlain G, et al. Association of the T-cell regulatory gene CTLA4 with susceptibility to autoimmune disease. Nature (2003) 423(6939):506–11. doi:10.1038/nature01621
35. Field LL, Larsen Z, Pociot F, Nerup J, Tobias R, Bonnevie-Nielsen V. Evidence for a locus (IDDM16) in the immunoglobulin heavy chain region on chromosome 14q32.3 producing susceptibility to type 1 diabetes. Genes Immun (2002) 3(6):338–44. doi:10.1038/sj.gene.6363857
36. Morahan G, Huang D, Ymer SI, Cancilla MR, Stephen K, Dabadghao P, et al. Linkage disequilibrium of a type 1 diabetes susceptibility locus with a regulatory IL12B allele. Nat Genet (2001) 27(2):218–21. doi:10.1038/84872
37. Dooley J, Tian L, Schonefeldt S, Delghingaro-Augusto V, Garcia-Perez JE, Pasciuto E, et al. Genetic predisposition for beta cell fragility underlies type 1 and type 2 diabetes. Nat Genet (2016) 48(5):519–27. doi:10.1038/ng.3531
38. Filippi CM, von Herrath MG. Viral trigger for type 1 diabetes: pros and cons. Diabetes (2008) 57(11):2863–71. doi:10.2337/db07-1023
39. Op de Beeck A, Eizirik DL. Viral infections in type 1 diabetes mellitus – why the beta cells? Nat Rev Endocrinol (2016) 12(5):263–73. doi:10.1038/nrendo.2016.30
40. Schulte BM, Bakkers J, Lanke KH, Melchers WJ, Westerlaken C, Allebes W, et al. Detection of enterovirus RNA in peripheral blood mononuclear cells of type 1 diabetic patients beyond the stage of acute infection. Viral Immunol (2010) 23(1):99–104. doi:10.1089/vim.2009.0072
41. Laitinen OH, Honkanen H, Pakkanen O, Oikarinen S, Hankaniemi MM, Huhtala H, et al. Coxsackievirus B1 is associated with induction of beta-cell autoimmunity that portends type 1 diabetes. Diabetes (2014) 63(2):446–55. doi:10.2337/db13-0619
42. Oikarinen S, Tauriainen S, Hober D, Lucas B, Vazeou A, Sioofy-Khojine A, et al. Virus antibody survey in different European populations indicates risk association between coxsackievirus B1 and type 1 diabetes. Diabetes (2014) 63(2):655–62. doi:10.2337/db13-0620
43. Richardson SJ, Leete P, Dhayal S, Russell MA, Oikarinen M, Laiho JE, et al. Detection of enterovirus in the islet cells of patients with type 1 diabetes: what do we learn from immunohistochemistry? Reply to Hansson SF, Korsgren S, Ponten F et al [letter]. Diabetologia (2014) 57(3):647–9. doi:10.1007/s00125-014-3167-2
44. Lin HC, Wang CH, Tsai FJ, Hwang KP, Chen W, Lin CC, et al. Enterovirus infection is associated with an increased risk of childhood type 1 diabetes in Taiwan: a nationwide population-based cohort study. Diabetologia (2015) 58(1):79–86. doi:10.1007/s00125-014-3400-z
45. Ylipaasto P, Klingel K, Lindberg AM, Otonkoski T, Kandolf R, Hovi T, et al. Enterovirus infection in human pancreatic islet cells, islet tropism in vivo and receptor involvement in cultured islet beta cells. Diabetologia (2004) 47(2):225–39. doi:10.1007/s00125-003-1297-z
46. Dotta F, Censini S, van Halteren AG, Marselli L, Masini M, Dionisi S, et al. Coxsackie B4 virus infection of beta cells and natural killer cell insulitis in recent-onset type 1 diabetic patients. Proc Natl Acad Sci U S A (2007) 104(12):5115–20. doi:10.1073/pnas.0700442104
47. Krogvold L, Edwin B, Buanes T, Frisk G, Skog O, Anagandula M, et al. Detection of a low-grade enteroviral infection in the islets of Langerhans of living patients newly diagnosed with type 1 diabetes. Diabetes (2015) 64(5):1682–7. doi:10.2337/db14-1370
48. Knip M, Siljander H. The role of the intestinal microbiota in type 1 diabetes mellitus. Nat Rev Endocrinol (2016) 12(3):154–67. doi:10.1038/nrendo.2015.218
49. Vaarala O, Atkinson MA, Neu J. The “perfect storm” for type 1 diabetes: the complex interplay between intestinal microbiota, gut permeability, and mucosal immunity. Diabetes (2008) 57(10):2555–62. doi:10.2337/db08-0331
50. Wen L, Ley RE, Volchkov PY, Stranges PB, Avanesyan L, Stonebraker AC, et al. Innate immunity and intestinal microbiota in the development of type 1 diabetes. Nature (2008) 455(7216):1109–13. doi:10.1038/nature07336
51. Markle JG, Frank DN, Mortin-Toth S, Robertson CE, Feazel LM, Rolle-Kampczyk U, et al. Sex differences in the gut microbiome drive hormone-dependent regulation of autoimmunity. Science (2013) 339(6123):1084–8. doi:10.1126/science.1233521
52. de Goffau MC, Luopajarvi K, Knip M, Ilonen J, Ruohtula T, Harkonen T, et al. Fecal microbiota composition differs between children with beta-cell autoimmunity and those without. Diabetes (2013) 62(4):1238–44. doi:10.2337/db12-0526
53. Endesfelder D, Zu Castell W, Ardissone A, Davis-Richardson AG, Achenbach P, Hagen M, et al. Compromised gut microbiota networks in children with anti-islet cell autoimmunity. Diabetes (2014) 63(6):2006–14. doi:10.2337/db13-1676
54. Kostic AD, Gevers D, Siljander H, Vatanen T, Hyotylainen T, Hamalainen AM, et al. The dynamics of the human infant gut microbiome in development and in progression toward type 1 diabetes. Cell Host Microbe (2015) 17(2):260–73. doi:10.1016/j.chom.2015.01.001
55. Takaba H, Takayanagi H. The mechanisms of T cell selection in the thymus. Trends Immunol (2017) 38(11):805–16. doi:10.1016/j.it.2017.07.010
56. Klein L, Kyewski B, Allen PM, Hogquist KA. Positive and negative selection of the T cell repertoire: what thymocytes see (and don’t see). Nat Rev Immunol (2014) 14(6):377–91. doi:10.1038/nri3667
57. He Q, Morillon YM II, Spidale NA, Kroger CJ, Liu B, Sartor RB, et al. Thymic development of autoreactive T cells in NOD mice is regulated in an age-dependent manner. J Immunol (2013) 191(12):5858–66. doi:10.4049/jimmunol.1302273
58. Kroger CJ, Wang B, Tisch R. Temporal increase in thymocyte negative selection parallels enhanced thymic SIRPalpha+ DC function. Eur J Immunol (2016) 46(10):2352–62. doi:10.1002/eji.201646354
59. Guerau-de-Arellano M, Martinic M, Benoist C, Mathis D. Neonatal tolerance revisited: a perinatal window for Aire control of autoimmunity. J Exp Med (2009) 206(6):1245–52. doi:10.1084/jem.20090300
60. Anderson MS, Su MA. AIRE expands: new roles in immune tolerance and beyond. Nat Rev Immunol (2016) 16(4):247–58. doi:10.1038/nri.2016.9
61. Anderson MS, Venanzi ES, Klein L, Chen Z, Berzins SP, Turley SJ, et al. Projection of an immunological self shadow within the thymus by the aire protein. Science (2002) 298(5597):1395–401. doi:10.1126/science.1075958
62. Derbinski J, Schulte A, Kyewski B, Klein L. Promiscuous gene expression in medullary thymic epithelial cells mirrors the peripheral self. Nat Immunol (2001) 2(11):1032–9. doi:10.1038/ni723
63. Liston A, Lesage S, Wilson J, Peltonen L, Goodnow CC. Aire regulates negative selection of organ-specific T cells. Nat Immunol (2003) 4(4):350–4. doi:10.1038/ni906
64. Liston A, Lesage S, Gray DH, O’Reilly LA, Strasser A, Fahrer AM, et al. Generalized resistance to thymic deletion in the NOD mouse; a polygenic trait characterized by defective induction of Bim. Immunity (2004) 21(6):817–30. doi:10.1016/j.immuni.2004.10.014
65. Kishimoto H, Sprent J. A defect in central tolerance in NOD mice. Nat Immunol (2001) 2(11):1025–31. doi:10.1038/ni726
66. Mingueneau M, Jiang W, Feuerer M, Mathis D, Benoist C. Thymic negative selection is functional in NOD mice. J Exp Med (2012) 209(3):623–37. doi:10.1084/jem.20112593
67. Zucchelli S, Holler P, Yamagata T, Roy M, Benoist C, Mathis D. Defective central tolerance induction in NOD mice: genomics and genetics. Immunity (2005) 22(3):385–96. doi:10.1016/j.immuni.2005.01.015
68. Vang T, Congia M, Macis MD, Musumeci L, Orru V, Zavattari P, et al. Autoimmune-associated lymphoid tyrosine phosphatase is a gain-of-function variant. Nat Genet (2005) 37(12):1317–9. doi:10.1038/ng1673
69. Abramson J, Husebye ES. Autoimmune regulator and self-tolerance – molecular and clinical aspects. Immunol Rev (2016) 271(1):127–40. doi:10.1111/imr.12419
70. Ramsey C, Winqvist O, Puhakka L, Halonen M, Moro A, Kampe O, et al. Aire deficient mice develop multiple features of APECED phenotype and show altered immune response. Hum Mol Genet (2002) 11(4):397–409. doi:10.1093/hmg/11.4.397
71. Zuklys S, Balciunaite G, Agarwal A, Fasler-Kan E, Palmer E, Hollander GA. Normal thymic architecture and negative selection are associated with Aire expression, the gene defective in the autoimmune-polyendocrinopathy-candidiasis-ectodermal dystrophy (APECED). J Immunol (2000) 165(4):1976–83. doi:10.4049/jimmunol.165.4.1976
72. Yang S, Fujikado N, Kolodin D, Benoist C, Mathis D. Immune tolerance. Regulatory T cells generated early in life play a distinct role in maintaining self-tolerance. Science (2015) 348(6234):589–94. doi:10.1126/science.aaa7017
73. Aricha R, Feferman T, Scott HS, Souroujon MC, Berrih-Aknin S, Fuchs S. The susceptibility of Aire(-/-) mice to experimental myasthenia gravis involves alterations in regulatory T cells. J Autoimmun (2011) 36(1):16–24. doi:10.1016/j.jaut.2010.09.007
74. Lei Y, Ripen AM, Ishimaru N, Ohigashi I, Nagasawa T, Jeker LT, et al. Aire-dependent production of XCL1 mediates medullary accumulation of thymic dendritic cells and contributes to regulatory T cell development. J Exp Med (2011) 208(2):383–94. doi:10.1084/jem.20102327
75. Nagamine K, Peterson P, Scott HS, Kudoh J, Minoshima S, Heino M, et al. Positional cloning of the APECED gene. Nat Genet (1997) 17(4):393–8. doi:10.1038/ng1297-393
76. Finnish-German AC. An autoimmune disease, APECED, caused by mutations in a novel gene featuring two PHD-type zinc-finger domains. Nat Genet (1997) 17(4):399–403. doi:10.1038/ng1297-399
77. Fornari TA, Donate PB, Macedo C, Marques MM, Magalhaes DA, Passos GA. Age-related deregulation of Aire and peripheral tissue antigen genes in the thymic stroma of non-obese diabetic (NOD) mice is associated with autoimmune type 1 diabetes mellitus (DM-1). Mol Cell Biochem (2010) 342(1–2):21–8. doi:10.1007/s11010-010-0464-z
78. Bell GI, Horita S, Karam JH. A polymorphic locus near the human insulin gene is associated with insulin-dependent diabetes mellitus. Diabetes (1984) 33(2):176–83. doi:10.2337/diabetes.33.2.176
79. Unanue ER, Ferris ST, Carrero JA. The role of islet antigen presenting cells and the presentation of insulin in the initiation of autoimmune diabetes in the NOD mouse. Immunol Rev (2016) 272(1):183–201. doi:10.1111/imr.12430
80. Zhang L, Nakayama M, Eisenbarth GS. Insulin as an autoantigen in NOD/human diabetes. Curr Opin Immunol (2008) 20(1):111–8. doi:10.1016/j.coi.2007.11.005
81. Krishnamurthy B, Dudek NL, McKenzie MD, Purcell AW, Brooks AG, Gellert S, et al. Responses against islet antigens in NOD mice are prevented by tolerance to proinsulin but not IGRP. J Clin Invest (2006) 116(12):3258–65. doi:10.1172/JCI29602
82. Pugliese A, Zeller M, Fernandez A Jr, Zalcberg LJ, Bartlett RJ, Ricordi C, et al. The insulin gene is transcribed in the human thymus and transcription levels correlated with allelic variation at the INS VNTR-IDDM2 susceptibility locus for type 1 diabetes. Nat Genet (1997) 15(3):293–7. doi:10.1038/ng0397-293
83. Vafiadis P, Bennett ST, Todd JA, Nadeau J, Grabs R, Goodyer CG, et al. Insulin expression in human thymus is modulated by INS VNTR alleles at the IDDM2 locus. Nat Genet (1997) 15(3):289–92. doi:10.1038/ng0397-289
84. Monti P, Scirpoli M, Rigamonti A, Mayr A, Jaeger A, Bonfanti R, et al. Evidence for in vivo primed and expanded autoreactive T cells as a specific feature of patients with type 1 diabetes. J Immunol (2007) 179(9):5785–92. doi:10.4049/jimmunol.179.9.5785
85. Skowera A, Ladell K, McLaren JE, Dolton G, Matthews KK, Gostick E, et al. beta-cell-specific CD8 T cell phenotype in type 1 diabetes reflects chronic autoantigen exposure. Diabetes (2015) 64(3):916–25. doi:10.2337/db14-0332
86. Viglietta V, Kent SC, Orban T, Hafler DA. GAD65-reactive T cells are activated in patients with autoimmune type 1a diabetes. J Clin Invest (2002) 109(7):895–903. doi:10.1172/JCI14114
87. Arif S, Tree TI, Astill TP, Tremble JM, Bishop AJ, Dayan CM, et al. Autoreactive T cell responses show proinflammatory polarization in diabetes but a regulatory phenotype in health. J Clin Invest (2004) 113(3):451–63. doi:10.1172/JCI19585
88. Luce S, Lemonnier F, Briand JP, Coste J, Lahlou N, Muller S, et al. Single insulin-specific CD8+ T cells show characteristic gene expression profiles in human type 1 diabetes. Diabetes (2011) 60(12):3289–99. doi:10.2337/db11-0270
89. Velthuis JH, Unger WW, Abreu JR, Duinkerken G, Franken K, Peakman M, et al. Simultaneous detection of circulating autoreactive CD8+ T-cells specific for different islet cell-associated epitopes using combinatorial MHC multimers. Diabetes (2010) 59(7):1721–30. doi:10.2337/db09-1486
90. Chen Z, Benoist C, Mathis D. How defects in central tolerance impinge on a deficiency in regulatory T cells. Proc Natl Acad Sci U S A (2005) 102(41):14735–40. doi:10.1073/pnas.0507014102
91. Jiang W, Anderson MS, Bronson R, Mathis D, Benoist C. Modifier loci condition autoimmunity provoked by Aire deficiency. J Exp Med (2005) 202(6):805–15. doi:10.1084/jem.20050693
92. Ferreira C, Singh Y, Furmanski AL, Wong FS, Garden OA, Dyson J. Non-obese diabetic mice select a low-diversity repertoire of natural regulatory T cells. Proc Natl Acad Sci U S A (2009) 106(20):8320–5. doi:10.1073/pnas.0808493106
93. St-Onge L, Wehr R, Gruss P. Pancreas development and diabetes. Curr Opin Genet Dev (1999) 9(3):295–300. doi:10.1016/S0959-437X(99)80044-6
94. Finegood DT, Scaglia L, Bonner-Weir S. Dynamics of beta-cell mass in the growing rat pancreas. Estimation with a simple mathematical model. Diabetes (1995) 44(3):249–56. doi:10.2337/diabetes.44.3.249
95. Gagnerault MC, Luan JJ, Lotton C, Lepault F. Pancreatic lymph nodes are required for priming of beta cell reactive T cells in NOD mice. J Exp Med (2002) 196(3):369–77. doi:10.1084/jem.20011353
96. Turley S, Poirot L, Hattori M, Benoist C, Mathis D. Physiological beta cell death triggers priming of self-reactive T cells by dendritic cells in a type-1 diabetes model. J Exp Med (2003) 198(10):1527–37. doi:10.1084/jem.20030966
97. Rotondi M, Chiovato L, Romagnani S, Serio M, Romagnani P. Role of chemokines in endocrine autoimmune diseases. Endocr Rev (2007) 28(5):492–520. doi:10.1210/er.2006-0044
98. Sarkar SA, Lee CE, Victorino F, Nguyen TT, Walters JA, Burrack A, et al. Expression and regulation of chemokines in murine and human type 1 diabetes. Diabetes (2012) 61(2):436–46. doi:10.2337/db11-0853
99. Rhode A, Pauza ME, Barral AM, Rodrigo E, Oldstone MB, von Herrath MG, et al. Islet-specific expression of CXCL10 causes spontaneous islet infiltration and accelerates diabetes development. J Immunol (2005) 175(6):3516–24. doi:10.4049/jimmunol.175.6.3516
100. Yurkovetskiy L, Burrows M, Khan AA, Graham L, Volchkov P, Becker L, et al. Gender bias in autoimmunity is influenced by microbiota. Immunity (2013) 39(2):400–12. doi:10.1016/j.immuni.2013.08.013
101. Kawabe T, Jankovic D, Kawabe S, Huang Y, Lee PH, Yamane H, et al. Memory-phenotype CD4+ T cells spontaneously generated under steady-state conditions exert innate TH1-like effector function. Sci Immunol (2017) 2(12):eaam9304. doi:10.1126/sciimmunol.aam9304
102. Zhang X, Zhivaki D, Lo-Man R. Unique aspects of the perinatal immune system. Nat Rev Immunol (2017) 17(8):495–507. doi:10.1038/nri.2017.54
103. Jameson SC, Lee YJ, Hogquist KA. Innate memory T cells. Adv Immunol (2015) 126:173–213. doi:10.1016/bs.ai.2014.12.001
104. Kieper WC, Troy A, Burghardt JT, Ramsey C, Lee JY, Jiang HQ, et al. Recent immune status determines the source of antigens that drive homeostatic T cell expansion. J Immunol (2005) 174(6):3158–63. doi:10.4049/jimmunol.174.6.3158
105. Christianson SW, Shultz LD, Leiter EH. Adoptive transfer of diabetes into immunodeficient NOD-scid/scid mice. Relative contributions of CD4+ and CD8+ T-cells from diabetic versus prediabetic NOD.NON-Thy-1a donors. Diabetes (1993) 42(1):44–55. doi:10.2337/diab.42.1.44
106. Roep BO. The role of T-cells in the pathogenesis of Type 1 diabetes: from cause to cure. Diabetologia (2003) 46(3):305–21. doi:10.1007/s00125-003-1089-5
107. Pang S, Zhang L, Wang H, Yi Z, Li L, Gao L, et al. CD8(+) T cells specific for beta cells encounter their cognate antigens in the islets of NOD mice. Eur J Immunol (2009) 39(10):2716–24. doi:10.1002/eji.200939408
108. Mallone R, Brezar V, Boitard C. T cell recognition of autoantigens in human type 1 diabetes: clinical perspectives. Clin Dev Immunol (2011) 2011:513210. doi:10.1155/2011/513210
109. Walker LS, von Herrath M. CD4 T cell differentiation in type 1 diabetes. Clin Exp Immunol (2016) 183(1):16–29. doi:10.1111/cei.12672
110. Kuriya G, Uchida T, Akazawa S, Kobayashi M, Nakamura K, Satoh T, et al. Double deficiency in IL-17 and IFN-gamma signalling significantly suppresses the development of diabetes in the NOD mouse. Diabetologia (2013) 56(8):1773–80. doi:10.1007/s00125-013-2935-8
111. Ferraro A, Socci C, Stabilini A, Valle A, Monti P, Piemonti L, et al. Expansion of Th17 cells and functional defects in T regulatory cells are key features of the pancreatic lymph nodes in patients with type 1 diabetes. Diabetes (2011) 60(11):2903–13. doi:10.2337/db11-0090
112. Martin AJ, Clark M, Gojanovich G, Manzoor F, Miller K, Kline DE, et al. Anti-coreceptor therapy drives selective T cell egress by suppressing inflammation-dependent chemotactic cues. JCI Insight (2016) 1(17):e87636. doi:10.1172/jci.insight.87636
113. Cnop M, Welsh N, Jonas JC, Jorns A, Lenzen S, Eizirik DL. Mechanisms of pancreatic beta-cell death in type 1 and type 2 diabetes: many differences, few similarities. Diabetes (2005) 54(Suppl 2):S97–107. doi:10.2337/diabetes.54.suppl_2.S97
114. Yoon JW, Jun HS. Autoimmune destruction of pancreatic beta cells. Am J Ther (2005) 12(6):580–91. doi:10.1097/01.mjt.0000178767.67857.63
115. Ferreira RC, Simons HZ, Thompson WS, Cutler AJ, Dopico XC, Smyth DJ, et al. IL-21 production by CD4+ effector T cells and frequency of circulating follicular helper T cells are increased in type 1 diabetes patients. Diabetologia (2015) 58(4):781–90. doi:10.1007/s00125-015-3509-8
116. Sutherland AP, Van Belle T, Wurster AL, Suto A, Michaud M, Zhang D, et al. Interleukin-21 is required for the development of type 1 diabetes in NOD mice. Diabetes (2009) 58(5):1144–55. doi:10.2337/db08-0882
117. Vinuesa CG, Tangye SG, Moser B, Mackay CR. Follicular B helper T cells in antibody responses and autoimmunity. Nat Rev Immunol (2005) 5(11):853–65. doi:10.1038/nri1714
118. Crotty S. Follicular helper CD4 T cells (TFH). Annu Rev Immunol (2011) 29:621–63. doi:10.1146/annurev-immunol-031210-101400
119. Kenefeck R, Wang CJ, Kapadi T, Wardzinski L, Attridge K, Clough LE, et al. Follicular helper T cell signature in type 1 diabetes. J Clin Invest (2015) 125(1):292–303. doi:10.1172/JCI76238
120. Serreze DV, Chapman HD, Varnum DS, Hanson MS, Reifsnyder PC, Richard SD, et al. B lymphocytes are essential for the initiation of T cell-mediated autoimmune diabetes: analysis of a new “speed congenic” stock of NOD.Ig mu null mice. J Exp Med (1996) 184(5):2049–53. doi:10.1084/jem.184.5.2049
121. Noorchashm H, Lieu YK, Noorchashm N, Rostami SY, Greeley SA, Schlachterman A, et al. I-Ag7-mediated antigen presentation by B lymphocytes is critical in overcoming a checkpoint in T cell tolerance to islet beta cells of nonobese diabetic mice. J Immunol (1999) 163(2):743–50.
122. Frohlich A, Kisielow J, Schmitz I, Freigang S, Shamshiev AT, Weber J, et al. IL-21R on T cells is critical for sustained functionality and control of chronic viral infection. Science (2009) 324(5934):1576–80. doi:10.1126/science.1172815
123. Yi JS, Du M, Zajac AJ. A vital role for interleukin-21 in the control of a chronic viral infection. Science (2009) 324(5934):1572–6. doi:10.1126/science.1175194
124. McGuire HM, Vogelzang A, Ma CS, Hughes WE, Silveira PA, Tangye SG, et al. A subset of interleukin-21+ chemokine receptor CCR9+ T helper cells target accessory organs of the digestive system in autoimmunity. Immunity (2011) 34(4):602–15. doi:10.1016/j.immuni.2011.01.021
125. Sutherland AP, Joller N, Michaud M, Liu SM, Kuchroo VK, Grusby MJ. IL-21 promotes CD8+ CTL activity via the transcription factor T-bet. J Immunol (2013) 190(8):3977–84. doi:10.4049/jimmunol.1201730
126. Anjos S, Nguyen A, Ounissi-Benkalha H, Tessier MC, Polychronakos C. A common autoimmunity predisposing signal peptide variant of the cytotoxic T-lymphocyte antigen 4 results in inefficient glycosylation of the susceptibility allele. J Biol Chem (2002) 277(48):46478–86. doi:10.1074/jbc.M206894200
127. Luhder F, Chambers C, Allison JP, Benoist C, Mathis D. Pinpointing when T cell costimulatory receptor CTLA-4 must be engaged to dampen diabetogenic T cells. Proc Natl Acad Sci U S A (2000) 97(22):12204–9. doi:10.1073/pnas.200348397
128. Schneider A, Rieck M, Sanda S, Pihoker C, Greenbaum C, Buckner JH. The effector T cells of diabetic subjects are resistant to regulation via CD4+ FOXP3+ regulatory T cells. J Immunol (2008) 181(10):7350–5. doi:10.4049/jimmunol.181.10.7350
129. D’Alise AM, Auyeung V, Feuerer M, Nishio J, Fontenot J, Benoist C, et al. The defect in T-cell regulation in NOD mice is an effect on the T-cell effectors. Proc Natl Acad Sci U S A (2008) 105(50):19857–62. doi:10.1073/pnas.0810713105
130. Tang Q, Adams JY, Penaranda C, Melli K, Piaggio E, Sgouroudis E, et al. Central role of defective interleukin-2 production in the triggering of islet autoimmune destruction. Immunity (2008) 28(5):687–97. doi:10.1016/j.immuni.2008.03.016
131. Xufre C, Costa M, Roura-Mir C, Codina-Busqueta E, Usero L, Pizarro E, et al. Low frequency of GITR+ T cells in ex vivo and in vitro expanded Treg cells from type 1 diabetic patients. Int Immunol (2013) 25(10):563–74. doi:10.1093/intimm/dxt020
132. Brunkow ME, Jeffery EW, Hjerrild KA, Paeper B, Clark LB, Yasayko SA, et al. Disruption of a new forkhead/winged-helix protein, scurfin, results in the fatal lymphoproliferative disorder of the scurfy mouse. Nat Genet (2001) 27(1):68–73. doi:10.1038/83784
133. Wildin RS, Smyk-Pearson S, Filipovich AH. Clinical and molecular features of the immunodysregulation, polyendocrinopathy, enteropathy, X linked (IPEX) syndrome. J Med Genet (2002) 39(8):537–45. doi:10.1136/jmg.39.8.537
134. Fontenot JD, Gavin MA, Rudensky AY. Foxp3 programs the development and function of CD4+CD25+ regulatory T cells. Nat Immunol (2003) 4(4):330–6. doi:10.1038/ni904
135. Collison LW, Chaturvedi V, Henderson AL, Giacomin PR, Guy C, Bankoti J, et al. IL-35-mediated induction of a potent regulatory T cell population. Nat Immunol (2010) 11(12):1093–101. doi:10.1038/ni.1952
136. Vignali DA, Collison LW, Workman CJ. How regulatory T cells work. Nat Rev Immunol (2008) 8(7):523–32. doi:10.1038/nri2343
137. Lowe CE, Cooper JD, Brusko T, Walker NM, Smyth DJ, Bailey R, et al. Large-scale genetic fine mapping and genotype-phenotype associations implicate polymorphism in the IL2RA region in type 1 diabetes. Nat Genet (2007) 39(9):1074–82. doi:10.1038/ng2102
138. Maier LM, Anderson DE, Severson CA, Baecher-Allan C, Healy B, Liu DV, et al. Soluble IL-2RA levels in multiple sclerosis subjects and the effect of soluble IL-2RA on immune responses. J Immunol (2009) 182(3):1541–7. doi:10.4049/jimmunol.182.3.1541
139. Giordano C, Panto F, Caruso C, Modica MA, Zambito AM, Sapienza N, et al. Interleukin 2 and soluble interleukin 2-receptor secretion defect in vitro in newly diagnosed type I diabetic patients. Diabetes (1989) 38(3):310–5. doi:10.2337/diab.38.3.310
140. Brusko T, Wasserfall C, McGrail K, Schatz R, Viener HL, Schatz D, et al. No alterations in the frequency of FOXP3+ regulatory T-cells in type 1 diabetes. Diabetes (2007) 56(3):604–12. doi:10.2337/db06-1248
141. Brusko TM, Wasserfall CH, Clare-Salzler MJ, Schatz DA, Atkinson MA. Functional defects and the influence of age on the frequency of CD4+ CD25+ T-cells in type 1 diabetes. Diabetes (2005) 54(5):1407–14. doi:10.2337/diabetes.54.5.1407
142. Qu HQ, Verlaan DJ, Ge B, Lu Y, Lam KC, Grabs R, et al. A cis-acting regulatory variant in the IL2RA locus. J Immunol (2009) 183(8):5158–62. doi:10.4049/jimmunol.0901337
143. Denny P, Lord CJ, Hill NJ, Goy JV, Levy ER, Podolin PL, et al. Mapping of the IDDM locus Idd3 to a 0.35-cM interval containing the interleukin-2 gene. Diabetes (1997) 46(4):695–700. doi:10.2337/diab.46.4.695
144. Lu L, Barbi J, Pan F. The regulation of immune tolerance by FOXP3. Nat Rev Immunol (2017) 17(11):703–17. doi:10.1038/nri.2017.75
145. Bailey-Bucktrout SL, Bluestone JA. Regulatory T cells: stability revisited. Trends Immunol (2011) 32(7):301–6. doi:10.1016/j.it.2011.04.002
146. Lindley S, Dayan CM, Bishop A, Roep BO, Peakman M, Tree TI. Defective suppressor function in CD4(+)CD25(+) T-cells from patients with type 1 diabetes. Diabetes (2005) 54(1):92–9. doi:10.2337/diabetes.54.1.92
147. Ryba-Stanislawowska M, Rybarczyk-Kapturska K, Mysliwiec M, Mysliwska J. Elevated levels of serum IL-12 and IL-18 are associated with lower frequencies of CD4(+)CD25 (high)FOXP3 (+) regulatory t cells in young patients with type 1 diabetes. Inflammation (2014) 37(5):1513–20. doi:10.1007/s10753-014-9878-1
148. Haseda F, Imagawa A, Murase-Mishiba Y, Terasaki J, Hanafusa T. CD4(+) CD45RA(-) FoxP3high activated regulatory T cells are functionally impaired and related to residual insulin-secreting capacity in patients with type 1 diabetes. Clin Exp Immunol (2013) 173(2):207–16. doi:10.1111/cei.12116
149. Hulme MA, Wasserfall CH, Atkinson MA, Brusko TM. Central role for interleukin-2 in type 1 diabetes. Diabetes (2012) 61(1):14–22. doi:10.2337/db11-1213
150. Garg G, Tyler JR, Yang JH, Cutler AJ, Downes K, Pekalski M, et al. Type 1 diabetes-associated IL2RA variation lowers IL-2 signaling and contributes to diminished CD4+CD25+ regulatory T cell function. J Immunol (2012) 188(9):4644–53. doi:10.4049/jimmunol.1100272
151. Hartemann A, Bensimon G, Payan CA, Jacqueminet S, Bourron O, Nicolas N, et al. Low-dose interleukin 2 in patients with type 1 diabetes: a phase 1/2 randomised, double-blind, placebo-controlled trial. Lancet Diabetes Endocrinol (2013) 1(4):295–305. doi:10.1016/S2213-8587(13)70113-X
152. Grinberg-Bleyer Y, Baeyens A, You S, Elhage R, Fourcade G, Gregoire S, et al. IL-2 reverses established type 1 diabetes in NOD mice by a local effect on pancreatic regulatory T cells. J Exp Med (2010) 207(9):1871–8. doi:10.1084/jem.20100209
153. Roep BO, Kracht MJ, van Lummel M, Zaldumbide A. A roadmap of the generation of neoantigens as targets of the immune system in type 1 diabetes. Curr Opin Immunol (2016) 43:67–73. doi:10.1016/j.coi.2016.09.007
154. McLaughlin RJ, de Haan A, Zaldumbide A, de Koning EJ, de Ru AH, van Veelen PA, et al. Human islets and dendritic cells generate post-translationally modified islet autoantigens. Clin Exp Immunol (2016) 185(2):133–40. doi:10.1111/cei.12775
155. van Lummel M, Duinkerken G, van Veelen PA, de Ru A, Cordfunke R, Zaldumbide A, et al. Posttranslational modification of HLA-DQ binding islet autoantigens in type 1 diabetes. Diabetes (2014) 63(1):237–47. doi:10.2337/db12-1214
156. Delong T, Wiles TA, Baker RL, Bradley B, Barbour G, Reisdorph R, et al. Pathogenic CD4 T cells in type 1 diabetes recognize epitopes formed by peptide fusion. Science (2016) 351(6274):711–4. doi:10.1126/science.aad2791
157. Phelps EA, Cianciaruso C, Michael IP, Pasquier M, Kanaani J, Nano R, et al. Aberrant accumulation of the diabetes autoantigen GAD65 in Golgi membranes in conditions of ER stress and autoimmunity. Diabetes (2016) 65(9):2686–99. doi:10.2337/db16-0180
158. Kracht MJ, van Lummel M, Nikolic T, Joosten AM, Laban S, van der Slik AR, et al. Autoimmunity against a defective ribosomal insulin gene product in type 1 diabetes. Nat Med (2017) 23(4):501–7. doi:10.1038/nm.4289
159. Eizirik DL, Sammeth M, Bouckenooghe T, Bottu G, Sisino G, Igoillo-Esteve M, et al. The human pancreatic islet transcriptome: expression of candidate genes for type 1 diabetes and the impact of pro-inflammatory cytokines. PLoS Genet (2012) 8(3):e1002552. doi:10.1371/journal.pgen.1002552
160. Yoshimatsu G, Kunnathodi F, Saravanan PB, Shahbazov R, Chang C, Darden CM, et al. Pancreatic beta cell-derived IP-10/CXCL10 isletokine mediates early loss of graft function in islet cell transplantation. Diabetes (2017) 66(11):2857–67. doi:10.2337/db17-0578
161. Tanaka S, Nishida Y, Aida K, Maruyama T, Shimada A, Suzuki M, et al. Enterovirus infection, CXC chemokine ligand 10 (CXCL10), and CXCR3 circuit: a mechanism of accelerated beta-cell failure in fulminant type 1 diabetes. Diabetes (2009) 58(10):2285–91. doi:10.2337/db09-0091
162. Homann D. Back from the brink: the uses of targeting the CXCL10:CXCR3 axis in type 1 diabetes. Diabetes (2015) 64(12):3990–2. doi:10.2337/dbi15-0019
163. Tan TG, Mathis D, Benoist C. Singular role for T-BET+CXCR3+ regulatory T cells in protection from autoimmune diabetes. Proc Natl Acad Sci U S A (2016) 113(49):14103–8. doi:10.1073/pnas.1616710113
164. Kriegel MA, Rathinam C, Flavell RA. Pancreatic islet expression of chemokine CCL2 suppresses autoimmune diabetes via tolerogenic CD11c+ CD11b+ dendritic cells. Proc Natl Acad Sci U S A (2012) 109(9):3457–62. doi:10.1073/pnas.1115308109
165. Bouma G, Nikolic T, Coppens JM, van Helden-Meeuwsen CG, Leenen PJ, Drexhage HA, et al. NOD mice have a severely impaired ability to recruit leukocytes into sites of inflammation. Eur J Immunol (2005) 35(1):225–35. doi:10.1002/eji.200425513
166. Guan R, Purohit S, Wang H, Bode B, Reed JC, Steed RD, et al. Chemokine (C-C motif) ligand 2 (CCL2) in sera of patients with type 1 diabetes and diabetic complications. PLoS One (2011) 6(4):e17822. doi:10.1371/journal.pone.0017822
167. Diana J, Lehuen A. Macrophages and beta-cells are responsible for CXCR2-mediated neutrophil infiltration of the pancreas during autoimmune diabetes. EMBO Mol Med (2014) 6(8):1090–104. doi:10.15252/emmm.201404144
168. Citro A, Cantarelli E, Piemonti L. The CXCR1/2 pathway: involvement in diabetes pathophysiology and potential target for T1D interventions. Curr Diab Rep (2015) 15(10):68. doi:10.1007/s11892-015-0638-x
169. Maedler K, Sergeev P, Ris F, Oberholzer J, Joller-Jemelka HI, Spinas GA, et al. Glucose-induced beta cell production of IL-1beta contributes to glucotoxicity in human pancreatic islets. J Clin Invest (2002) 110(6):851–60. doi:10.1172/JCI15318
170. Cardozo AK, Proost P, Gysemans C, Chen MC, Mathieu C, Eizirik DL. IL-1beta and IFN-gamma induce the expression of diverse chemokines and IL-15 in human and rat pancreatic islet cells, and in islets from pre-diabetic NOD mice. Diabetologia (2003) 46(2):255–66. doi:10.1007/s00125-002-1017-0
171. Timper K, Dalmas E, Dror E, Rutti S, Thienel C, Sauter NS, et al. Glucose-dependent insulinotropic peptide stimulates glucagon-like peptide 1 production by pancreatic islets via interleukin 6, produced by alpha cells. Gastroenterology (2016) 151(1):165–79. doi:10.1053/j.gastro.2016.03.003
172. Anquetil F, Sabouri S, Thivolet C, Rodriguez-Calvo T, Zapardiel-Gonzalo J, Amirian N, et al. Alpha cells, the main source of IL-1beta in human pancreas. J Autoimmun (2017) 81:68–73. doi:10.1016/j.jaut.2017.03.006
173. Thomas HE, Parker JL, Schreiber RD, Kay TW. IFN-gamma action on pancreatic beta cells causes class I MHC upregulation but not diabetes. J Clin Invest (1998) 102(6):1249–57. doi:10.1172/JCI2899
174. Rui J, Deng S, Arazi A, Perdigoto AL, Liu Z, Herold KC. Beta cells that resist immunological attack develop during progression of autoimmune diabetes in NOD mice. Cell Metab (2017) 25(3):727–38. doi:10.1016/j.cmet.2017.01.005
175. Khan IS, Mouchess ML, Zhu ML, Conley B, Fasano KJ, Hou Y, et al. Enhancement of an anti-tumor immune response by transient blockade of central T cell tolerance. J Exp Med (2014) 211(5):761–8. doi:10.1084/jem.20131889
176. Bakhru P, Zhu ML, Wang HH, Hong LK, Khan I, Mouchess M, et al. Combination central tolerance and peripheral checkpoint blockade unleashes antimelanoma immunity. JCI Insight (2017) 2(18):93265. doi:10.1172/jci.insight.93265
Keywords: autoimmunity, type 1 diabetes, immunoregulation, inflammation, T cells
Citation: Clark M, Kroger CJ and Tisch RM (2017) Type 1 Diabetes: A Chronic Anti-Self-Inflammatory Response. Front. Immunol. 8:1898. doi: 10.3389/fimmu.2017.01898
Received: 20 October 2017; Accepted: 12 December 2017;
Published: 22 December 2017
Edited by:
Philippe Saas, INSERM UMR1098 Interactions Hôte-Greffon-Tumeur & Ingénierie Cellulaire et Génique, FranceReviewed by:
Joanne E. Konkel, University of Manchester, United KingdomCopyright: © 2017 Clark, Kroger and Tisch. This is an open-access article distributed under the terms of the Creative Commons Attribution License (CC BY). The use, distribution or reproduction in other forums is permitted, provided the original author(s) or licensor are credited and that the original publication in this journal is cited, in accordance with accepted academic practice. No use, distribution or reproduction is permitted which does not comply with these terms.
*Correspondence: Roland M. Tisch, cm10aXNjaEBtZWQudW5jLmVkdQ==
†These authors have contributed equally to this work.
Disclaimer: All claims expressed in this article are solely those of the authors and do not necessarily represent those of their affiliated organizations, or those of the publisher, the editors and the reviewers. Any product that may be evaluated in this article or claim that may be made by its manufacturer is not guaranteed or endorsed by the publisher.
Research integrity at Frontiers
Learn more about the work of our research integrity team to safeguard the quality of each article we publish.