- 1Department of Dermatology, Harvard Skin Disease Research Center, Brigham and Women’s Hospital, Harvard Medical School, Boston, MA, United States
- 2Program of Excellence in Glycosciences, Harvard Medical School, Boston, MA, United States
- 3UCIBIO, Departamento Ciências da Vida, Faculdade de Ciências e Tecnologia, Universidade NOVA de Lisboa, Lisboa, Portugal
- 4Professionals and Patient Associations International Network (CDG & Allies – PPAIN), Faculdade de Ciências e Tecnologia, Universidade NOVA de Lisboa, Lisboa, Portugal
- 5Department of Medicine, Brigham and Women’s Hospital, Harvard Medical School, Boston, MA, United States
The mononuclear phagocyte system comprises a network of circulating monocytes and dendritic cells (DCs), and “histiocytes” (tissue-resident macrophages and DCs) that are derived in part from blood-borne monocytes and DCs. The capacity of circulating monocytes and DCs to function as the body’s first-line defense against offending pathogens greatly depends on their ability to egress the bloodstream and infiltrate inflammatory sites. Extravasation involves a sequence of coordinated molecular events and is initiated by E-selectin-mediated deceleration of the circulating leukocytes onto microvascular endothelial cells of the target tissue. E-selectin is inducibly expressed by cytokines (tumor necrosis factor-α and IL-1β) on inflamed endothelium, and binds to sialofucosylated glycan determinants displayed on protein and lipid scaffolds of blood cells. Efficient extravasation of circulating monocytes and DCs to inflamed tissues is crucial in facilitating an effective immune response, but also fuels the immunopathology of several inflammatory disorders. Thus, insights into the structural and functional properties of the E-selectin ligands expressed by different monocyte and DC populations is key to understanding the biology of protective immunity and the pathobiology of several acute and chronic inflammatory diseases. This review will address the role of E-selectin in recruitment of human circulating monocytes and DCs to sites of tissue injury/inflammation, the structural biology of the E-selectin ligands expressed by these cells, and the molecular effectors that shape E-selectin ligand cell-specific display. In addition, therapeutic approaches targeting E-selectin receptor/ligand interactions, which can be used to boost host defense or, conversely, to dampen pathological inflammatory conditions, will also be discussed.
Introduction
The mononuclear phagocyte system (MPS) comprises monocytes, dendritic cells (DC), and tissue-resident macrophages. MPS cells have specialized phagocytic capabilities, and antigen processing and presenting functions, thereby initiating the immune response and linking innate and adaptive immune systems (1). In addition to their role as key sentinels and regulators of immunity, mononuclear phagocytes are also involved in several pathological inflammatory conditions, including autoimmune diseases, infection, cancer, and abnormal wound healing processes (2). To access inflammatory sites, circulating monocytes and DCs must first engage the vascular endothelial barrier against the prevailing forces of hemodynamic shear, a process that occurs via adhesive interactions between vascular E-selectin and its glycan counter-receptors (E-selectin ligands) on the circulating cells (3). This initial contact results in tethering and slow rolling of the cells along the endothelial surface at velocities well below that of blood flow (4). E-selectin-mediated slow rolling is a vital step in this cascade of events as it allows intimate contact between MPS cells and the inflamed endothelium, and the recognition of inflammatory molecules within the milieu (3). Consequently, a greater knowledge of how E-selectin ligand display is elaborated by different types of circulating monocytes and DCs is key to understanding the physiological and pathological events associated with the MPS. In this review, we will provide information on the structural biology and operation of the wide variety of E-selectin-binding glycoconjugates expressed by circulating MPS cells (i.e., blood monocyte and non-tissue-resident DC populations) in light of their impact on pathology and potential therapies. Furthermore, we will discuss the molecular basis of the biosynthesis of these glycoconjugates, and how such knowledge can frame novel strategies to inhibit or enforce trafficking of MPS cells.
Mononuclear Phagocyte Family: Heterogeneity and Migratory Capabilities
Monocytes
Monocytes constitute a heterogeneous cell population, comprising approximately 5–10% of total peripheral blood leukocytes. These cells arise from granulocyte–macrophage progenitors in the bone marrow and are subsequently released into peripheral blood, where they circulate for several days (5). At steady state (i.e., without any inflammatory cue), monocytes can enter non-lymphoid tissues, and there they either retain their blood monocytic behavior (6), or generate the immediate precursors of “monocyte-derived macrophages and DCs,” which constitute a small portion of tissue-resident macrophage and DC populations (7–9). On the other hand, under inflammatory conditions, monocytes transmigrate into injured tissues, where they then directly mediate antimicrobial activity or, depending on the local biochemical milieu, differentiate into inflammatory macrophages or monocyte-derived DCs (moDCs) (10) (Figure 1). Circulating monocytes, thus, function as a systemic reservoir of tissue-resident myeloid cells (11, 12).
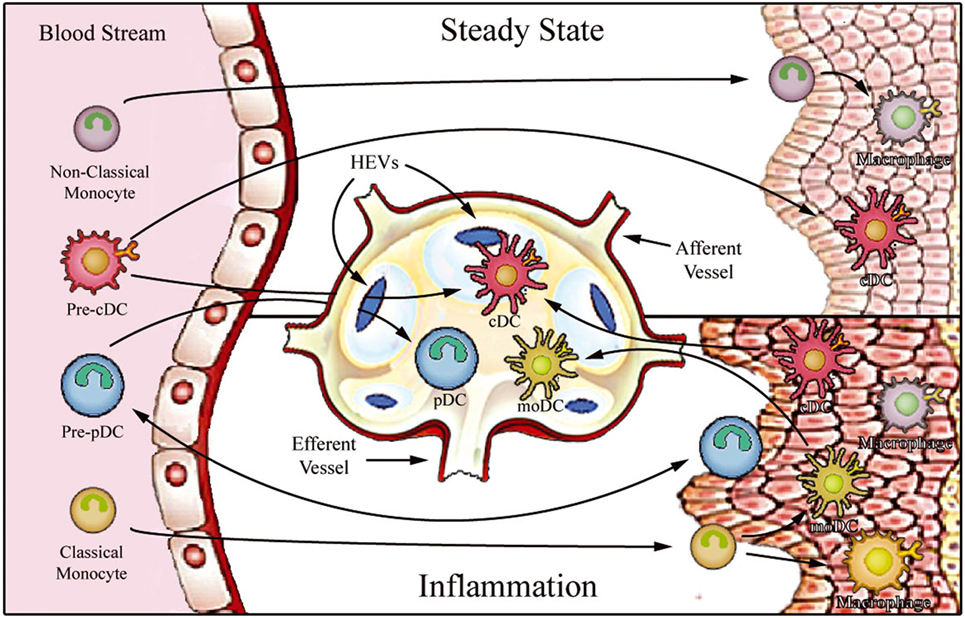
Figure 1. Proposed model for migration of human monocytes and dendritic cell (DC) progenitors into tissues in steady-state and inflammatory conditions. After differentiation in the bone barrow, precursors of DCs and monocytes enter the blood stream and are distributed to lymphoid organs [through high endothelial venules (HEV)] and to various peripheral tissues. In steady state, non-classical monocytes are preferentially recruited into the resting vasculature, where they patrol the endothelium and may contribute to the maintenance of tissue-resident macrophage and DC populations. Conventional DCs (cDCs) recirculate between peripheral tissues and lymphoid organs (migratory cDCs), participating in the induction of peripheral tolerance, or reside in the lymphoid organs (lymphoid-resident cDCs). By contrast, plasmacytoid DCs (pDCs) mostly populate lymphoid tissues (lymphoid-resident pDCs) and lack migratory ability under steady-state conditions. Upon inflammation, classical monocytes, cDCs, and pDCs are recruited to affected tissues. After antigen uptake and differentiation into fully functional mature DCs, monocyte-derived DCs (moDCs), and cDCs enter draining lymph nodes via afferent lymphatics. pDCs can only access reactive lymph nodes from the blood stream via HEVs.
There are three subsets of human monocytes, each of which display different functional and migratory abilities and can be distinguished based on their expression of specific chemokine receptors, CD14 [the lipopolysaccharide (LPS) receptor], and CD16 (Fcγ RIII) (13). “Classical” monocytes (CD14++CD16−), which account for about 90% of circulating monocytes in healthy individuals, express high levels of the C-C chemokine receptor type 2 (CCR2), display high phagocytic and myeloperoxidase activities, generate reactive oxygen species, and produce inflammatory cytokines, such as interleukin (IL)-1β, IL-6, and tumor necrosis factor (TNF)-α (14). On the other hand, the “non-classical” monocytes (CD14+CD16++) comprise a population that exhibits low phagocytic and myeloperoxidase activities (15, 16). Importantly, while classical monocytes are recruited preferentially to distressed tissues (17), non-classical monocytes are recruited to non-inflamed areas, where they patrol the microvasculature via the CX3C chemokine receptor 1 (CX3CR1) and leukocyte function-associated antigen (LFA)-1, monitoring the luminal surface of resting endothelium for signs of tissue damage or infection (18–20). In addition, non-classical monocytes are mainly responsive to virus-associated signals, via toll-like receptors (TLRs) 7 and 8, whereas classical monocytes respond mostly to bacteria-associated signals (21). An intermediate subset of monocytes, characterized as CD14++CD16+, is viewed as being a transitional population between classical and non-classical monocyte subsets, displaying significant production of TNF-α and IL-1β, but low peroxidase activity (16, 22). While the migratory ability of the intermediate subset is controversial, they express the chemokine receptor CCR2, a feature supporting their ability to infiltrate sites of inflammation (23). Still, overall, the intermediate monocyte population reportedly displays weaker ability to migrate across resting endothelium compared to the other two monocytic subsets (24).
Dendritic Cells
Dendritic cells are the antigen-presenting cells par excellence, showing a unique capacity to initiate immune responses. These specialized antigen-presenting cells constitute a unique leukocyte population that display high morphological and functional heterogeneity (25). DCs can be originated from common myeloid or lymphoid precursors and are divided into two main groups: conventional DCs (cDCs) and plasmacytoid DCs (pDCs) (26). After being released into the bloodstream, they are distributed to lymphoid organs (lymph nodes, spleen, and thymus) and various peripheral tissues. DC function is intrinsically related to their anatomical localization, and, therefore, a stringent DC functional-anatomical classification needs to be defined (Figure 1). At steady state, DCs are found to be immature (as indicated by high phagocytic and endocytic capacity and low expression of MHC and costimulatory molecules) and can be classified as either migratory or lymphoid-resident DCs (27, 28). Migratory DCs serve as immune sentinels screening peripheral tissues for signals of danger. They can also capture apoptotic cells or self-antigens in non-inflamed tissues and, after entering lymph nodes via afferent lymphatics, present these to T cells in the lymph nodes, thus playing a key role in antigen-mediated peripheral tolerance (29–31). On the other hand, lymphoid-resident DCs differentiate within lymphoid organs directly from blood DC precursors, and they function to continuously survey blood or lymph (27, 32). Both cDC and pDC hematopoietic progenitors contribute to the lymphoid-resident DC pool, whereas most migratory DCs arise from blood cDCs (33). Under infection or sterile inflammatory circumstances, both circulating cDCs and classical monocytes enter inflamed tissues, where they capture antigens and differentiate into highly functional mature DCs. The mature DCs migrate to the lymph nodes via the afferent lymph, initiating T cell-mediated immune responses.
In addition to cDCs and pDCs, a distinct subset of DCs are derived from monocytes (known as “moDCs”) which are considered to be “inflammatory DCs”; these cells prominently produce TNF-α, nitric oxide, and IL-23, and are potent inducers of TH17 cells (34–37). Interestingly, although pDCs are believed to be absent from peripheral tissues under steady-state conditions, a number of recent publications reported pDC extravasation into some inflamed tissues, where they secrete large amounts of type I interferon (38–42). In contrast to cDCs, pDCs do not enter reactive secondary lymphoid organs after trafficking from peripheral tissue via afferent lymphatics; instead, they apparently migrate directly from the bloodstream via high endothelial venules (HEVs) by an E-selectin-dependent mechanism (43–47).
Macrophages
Macrophages are a heterogeneous and versatile population of tissue-resident cells, mostly originating from self-renewing embryo-derived progenitors and from blood monocytes that have colonized tissues (48, 49). They exist virtually in every tissue throughout the body, where they survey for potential signs of infection/danger and perform phagocytic clearance of dying cells (50). In addition, macrophages play a role in adaptive immunity through antigen presentation and production of cytokines (51, 52).
There are two main macrophage subsets, the M1 and the M2 macrophages, with distinct responses to environmental signals. The M1 subset produces high amounts of pro-inflammatory cytokines and reactive oxygen and nitrogen species, thus playing a crucial role in Th1 polarization and promotion of cellular immunity. M2 macrophages are characterized by their ability to stimulate humoral immune responses, fight extracellular parasite infections, and promote tissue repair, angiogenesis, and tumor progression (53, 54). Whereas the major function of macrophages is to fight infections and kill target cells, they do not typically display hematogenous migration, nor leave sites of tissue injury (11, 55).
MPS Extravasation Cascade: The Multistep Model
Recruitment of circulating cells from blood to inflamed tissue involves a sequential and coordinated series of molecular actions mediated by adhesive interactions between circulating sentinels and endothelial cells in post-capillary venules (56). Here, we review the molecular effectors that regulate the initial phagocyte–endothelial binding interactions, which are essential for transendothelial migration of blood monocytes and DCs to sites of injury.
To initiate the extravasation process, circulating phagocytes establish low-affinity and reversible interactions (tethering) on target endothelial cells, achieving low velocity “rolling” adhesive interactions (Step 1, Figure 2). Rolling exposes these cells to chemokines that are immobilized by glycosaminoglycans on the endothelial surface, and, in turn, facilitates engagement of G-protein-coupled chemokine receptors (GPCRs) expressed on the mononuclear phagocyte cell surface (Step 2, Figure 2), with resultant G-protein-driven integrin activation (3, 57). Activated integrins on phagocytes, principally very late activation protein 4 and LFA-1, bind to their respective endothelial receptors vascular cell adhesion molecule-1 and intercellular adhesion molecule-1 (ICAM-1), leading to firm adhesion of MPS cells on the endothelium (Step 3, Figure 2) (3, 57). The binding of activated integrins then allows diapedesis into the tissue (Step 4, Figure 2). Two distinct mechanisms enable diapedesis: (1) transient dismantling of endothelial junctions (paracellular migration) or (2) migration through individual endothelial cells (transcellular migration) (58, 59).
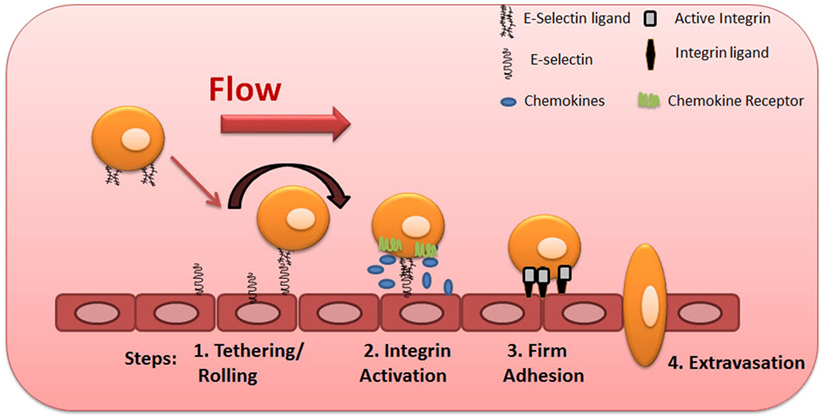
Figure 2. Multistep model of circulating blood cell adhesion and migration along the vascular endothelium. Cells make adhesive contacts onto the inflamed endothelial surface through engagement of their sialofucosylated glycan determinants to vascular E-selectin (Step 1—tethering and rolling). Subsequent engagement of chemokine receptors leads to integrin activation (Step 2) and firm adhesion of leukocytes to endothelium (Step 3), allowing their transmigration (Step 4).
Although several cell-associated proteins are specialized at mediating the first step of cell migration, the selectins and their ligands are the most potent effectors of tethering and rolling adhesive interactions. These molecules are responsible for the initial low-affinity binding interactions of leukocytes on endothelial layer (60), a property related to the unique biophysics of lectin–carbohydrate interactions under fluid shear conditions.
Selectins and Their Glycoconjugate Ligands
The Selectin Family
The selectins are a family of three carbohydrate-binding proteins that can be expressed on endothelial cells, leukocytes and platelets (Figure 3). Due to their requirement of calcium ions for binding, all three selectins, E-selectin (CD62E), P-selectin (CD62P), and L-selectin (CD62L), belong to the C-type lectin family (61). Selectins share a common structure of five different domains: an N-terminal carbohydrate recognition domain (CRD), an epidermal growth factor-like domain (EGF), a varying number of short consensus repeats that have homology to complement regulatory domains (“CRs” of which there are 2, 6, and 9 within L-, E-, and P-selectin, respectively), a transmembrane region, and a C-terminal cytoplasmatic domain (Figure 3) (62–64). While the CRD and EGF domains are highly homologous between the three selectins, the structure of the transmembrane and cytoplasmic portions, as well as the extracellular CR domains are not conserved across the selectins, resulting in structural diversity and varying molecular weights between selectins (61, 65).
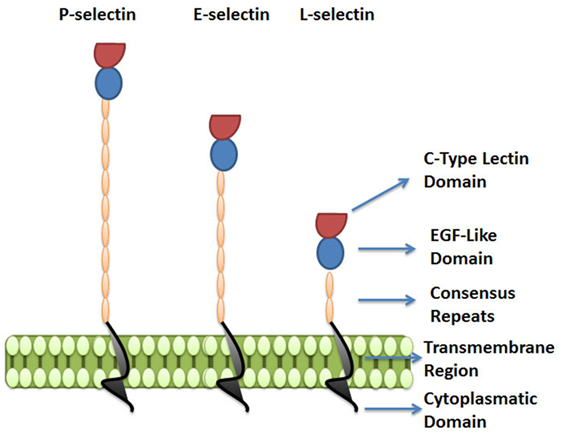
Figure 3. The selectin family. Selectins are a family of three carbohydrate-binding proteins: P-selectin, expressed on activated platelets and endothelial cells, E-selectin expressed on activated endothelial cells, and L-selectin expressed on leukocytes. The figure represents the five domains shared by selectins: C-type lectin domain, epidermal growth factor-like domain (EGF), a varying number of short consensus repeats having homology to complement regulatory proteins, a transmembrane region, and a cytoplasmatic domain.
Despite sharing common elements, the three selectins have different functions in diverse pathological and physiological processes and vary in their distribution and binding kinetics.
The biology of L-selectin was first elucidated by use of an in vitro assay in which suspensions of lymphocytes were overlaid onto lymph node sections (66). This assay then allowed for creation of mAb that could interrupt this binding, such as the mAb known as “MEL-14” described by Gallatin and coworkers (67) in 1983, and thereafter led investigators to cloning of this structure (62). L-selectin is highly expressed on hematopoietic stem cells and mature leukocytes, including all myeloid cells, subsets of natural killer cells, naïve T and B cells, and central memory T cells. When leukocytes are activated, cell surface levels of L-selectin are downregulated by proteolytic cleavage via metalloprotease-dependent shedding of the extracellular domain (61, 68, 69).
P-selectin was described in 1984 by McEver and coworkers (70, 71) and Furie and coworkers (72) as a glycoprotein expressed on the cell surface of activated platelets. P-selectin is constitutively expressed by circulating platelets and endothelial cells, where it is stored in α-granules and Weibel–Palade bodies, respectively. Because it can be expressed on endothelial cells, P-selectin together with E-selectin (described below) are known as the “vascular selectins.” Following pro-inflammatory stimulus by molecules such as thrombin or histamine, P-selectin is rapidly translocated from the granules to the cell surface by fusion of intracellular storage compartments with the plasma membrane. In murine endothelial cells, inflammatory mediators, such as TNF-α, IL-1β, and LPS, induce P-selectin mRNA transcription, which requires the cooperative binding of the nuclear factor κ-light chain-enhancer of activated B cells (NF-κB) and activating transcription factor-2 (ATF-2) to their response elements within the P-selectin promoter (73–75). However, importantly, the promoter of P-selectin in humans and other primates lacks binding sites for NF-κB and ATF-2 (76). For this reason, in human endothelial cells, the only vascular selectin inducibly expressed by TNF-α, LPS, and IL-1β is E-selectin (77).
E-selectin was first reported by Bevilacqua and coworkers (63, 78) in 1980s as a leukocyte adhesion molecule on activated endothelial cells. Skin and bone marrow microvessels express E-selectin constitutively (79), however, in other tissues, endothelial cells do not constitutively express E-selectin but its expression is strongly upregulated by inflammatory cytokines, such as TNF-α and IL-1β. These cytokines potently induce transient transcription (within hours of exposure) of E-selectin mRNA in both human and mouse endothelial cells (80). Cytokine-dependent activation of E-selectin is mediated by NF-κB binding to regulatory domains in the E-selectin promoter (81). Functionally, E-selectin slows leukocyte rolling to much lower velocities than do either L- or P-selectin, favoring subsequent leukocyte arrest (4, 82). This capacity, along with the inability of human endothelial cells to upregulate P-selectin in the presence of IL-1β and TNF-α, is why E-selectin is considered to be the most important selectin for cell trafficking to sites of inflammation in humans, and it plays a critical role in the recruitment of immune effectors to target inflammatory sites.
The Carbohydrate E-Selectin Ligands
E-selectin recognizes a range of structurally diverse glycan epitopes expressed by human leukocytes that typically contain α(1,3)-fucose (Fuc) and α(2,3)-sialic acid (Sia) modification(s) on a lactosamine backbone [consisting of galactose (Gal) linked to N-acetylglucosamine (GlcNAc)], as shown in Figure 4 (79). The terminal tetrasaccharide known as sialyl Lewis X (sLex—Siaα2-3Galβ1-4(Fucα1-3)GlcNAc) is the prototypical E-selectin-binding determinant (83–85). Some sLex-variant structures can also exhibit E-selectin binding activity, namely an internally fucosylated sLex-variant (VIM-2) and other polylactosamine structures, in which Fuc modifications occur at more than one GlcNAc residue along the polylactosamine chain (tri-fucosyl-sialyl Lewisx and di-fucosyl-sialyl Lewisx) (86–88). In addition, other glycan structures that are not natively expressed on leukocytes exhibit E-selectin binding activity, namely the sLex isomer, sialyl Lewis a (sLea—Siaα2-3Galβ1-3(Fucα1-4)GlcNAc) (89), some sulfated derivatives of Lex and Lea (3′-sulfo-Lex and 3′-sulfo-Lea, respectively) (90, 91), and a fucosylated glycoform of LacdiNac that displays a terminal N-acetylgalactosamine (GalNAc) instead of Sia (GalNAc-Lewis x) (92).
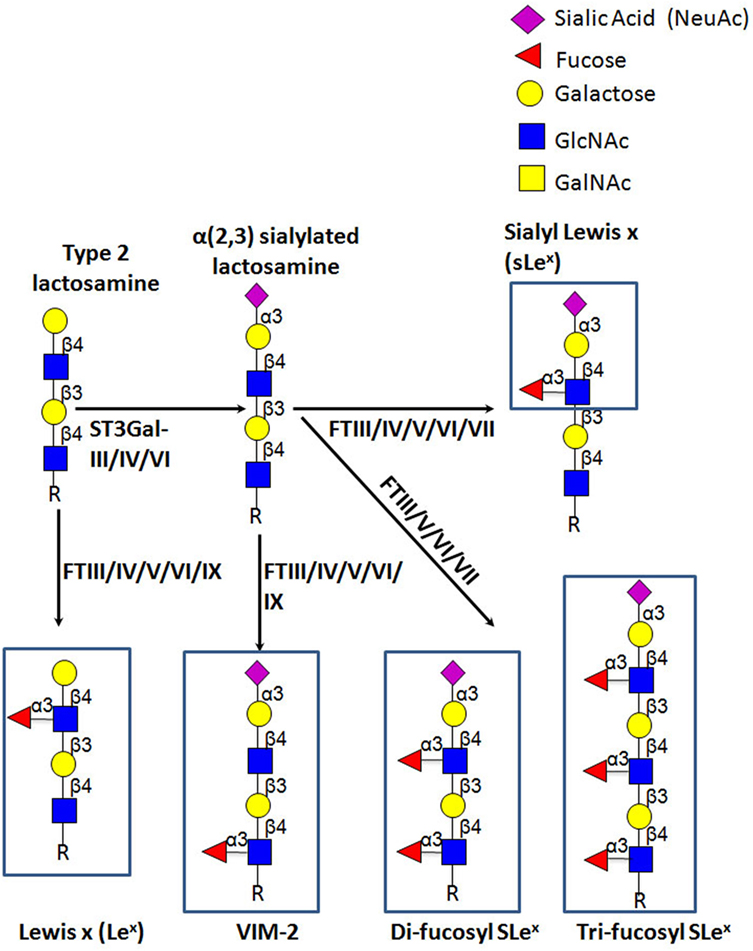
Figure 4. Schematic representation of biosynthesis of the E-selectin ligand determinants. The α(2,3)-sialyltransferases, ST3Gal-III, -IV, and -VI, terminate the elongation of both O- and N-glycans by creating sialylated type 2 lactosamine chains. These can be further fucosylated by the action of the specific fucosyltransferases, yielding different Lewis-related structures that display E-selectin binding activity.
Glycosyltransferases Involved in the Biosynthesis of Selectin–Carbohydrate-Binding Determinants
E-selectin binding determinants are typically displayed at the end of O-glycans, N-glycans, or glycolipid precursor structures, and require the coordinated and sequential action of specific glycosyltransferases localized within the lumen of the Golgi apparatus. Assembly of sLex is driven by the terminal addition of Sia (to Gal) and of Fuc (to GlcNAc) through the action of α(2,3)-sialyltransferases and α(1,3)-fucosyltransferases (FTs), respectively, on type 2 lactosamine (LacNAc) chains (i.e., Gal connected to GlcNAc through a β(1,4)-linkage) (Figure 4) (93, 94). The sialylated forms of Lewis antigens are synthesized by the action of the α(2,3)-sialyltransferases (ST3Gal isoenzymes). These enzymes transfer Sia residues to the Gal on the LacNAc chain, exclusively acting prior to fucosylation (95, 96). There are six members of the α(2,3)-sialyltransferase family (ST3Gal-I–ST3Gal-VI), but only ST3Gal-III, ST3Gal-IV, and ST3Gal-VI are reported to sialylate lactosamine chains (97). Importantly, ST3Gal-III exhibits preference for type 1 lactosamine chain acceptors (wherein Gal is connected to GlcNAc through a β(1,3)-linkage), whereas ST3Gal-IV and ST3Gal-VI preferentially act on type 2 polylactosamine chains (98–100). When Type 1 lactosamines are decorated with Sia in α(2,3)-linkage to Gal and with Fuc in β(1,3)-linkage to GlcNAc, this tetrasaccharide is known as sialyl Lewis A (sLeA).
So far, six human FTs have been found to catalyze the addition of Fuc at α(1,3) linkage to GlcNAc with a type 2 lactosamine—FTIII, FTIV, FTV, FTVI, FTVII, and FTIX. Each enzyme exhibits specificity for acceptor substrates and, therefore, has the ability to generate distinct fucosylated structures (101, 102). Particularly, FTIII and FTV are unique in that they exhibit both α(1,3) and α(1,4) FT activity on both sialylated and unsialylated type 2 and type 1 lactosamines thereby creating (s)Lex and (s)Lea epitopes, respectively (103, 104). On the other hand, FTIV and FTVI fucosylate both sialylated and unsialylated type 2 lactosamine chains, with FTIV creating VIM-2 and Lex (105, 106) and, modestly, sLex determinants (107, 108), and FTVI creating these structures as well as di-fucosyl-sLex (108–110). Uniquely, FTVII can only act on sialylated type 2 lactosamines, yielding sLex and di/tri-fucosyl-sLex-structures (111, 112), whereas FTIX is known to synthesize mostly Lex (101, 106).
Most of the reports that assess the role of the different glycosyltransferases involved in selectin ligand biosynthesis in leukocytes have been performed using knock-out mouse models, with a small proportion of these studies using human leukocytes or human hematopoietic cell lines. Concerning the role of the α(2,3)-sialyltransferases, murine studies suggest that ST3Gal-IV and ST3Gal-VI collaborate together in murine E-selectin ligand biosynthesis, with ST3Gal-IV having an important role in the regulation of E-selectin-dependent rolling velocity (113, 114). Interestingly, ST3Gal-III does not seem to contribute to the synthesis of murine E-selectin ligand moieties, since deficiency of this enzyme did not affect E-selectin ligand expression or activity on murine leukocytes (113). Surprisingly, ST3Gal-IV is reportedly the only human α(2,3)-sialyltransferase involved in the biosynthesis of E-selectin ligands in human myeloid leukocytes, since ST3Gal-IV-silenced HL-60 cells (a human promyelocytic cell line), and neutrophils derived from stable ST3Gal-IV knockdown hematopoietic stem cells fail to engage in tethering and rolling interactions on E-selectin-bearing substrates (115). In case of α(1,3)-FTs, studies demonstrate that mostly FTVII, and to a lesser extent FTIV, are the key murine α(1,3) FTs that mediate leukocyte selectin ligand biosynthesis. In fact, murine leukocytes lacking FTVII show poor adhesive contacts with E- and P-selectin, indicating that this FT plays a prominent role in murine E-selectin ligand biosynthesis (116, 117). However, others reported that FTIV is crucial for slow murine leukocyte rolling velocity (118, 119). Importantly, E-selectin binding activity conferred by murine FTIV, but not by FTVII, apparently occurs mainly on glycolipids rather than glycoproteins (120). Conversely, in human leukocytes, there is evidence that FTVII, FTIV, and FTIX could each act in synthesis of E-selectin ligand determinants (121). Notably, the mouse genome encodes only FTIV, FTVII, and FTIX (122), whereas primates possess an additional three FT gene products—FTIII, FTV, and FTIV. These additional FTs provide for a much wider capacity to create sLeX; in addition, the expression of FTIII and FTV in primates uniquely drives creation of sLeA determinants. Human circulating monocytes express all the α(1,3)-FTs, with the exception of FTV, heightening the potential for variability in glycoconjugates bearing sLex among human and mouse cells (123). Notably, sLeA is not expressed on any primate leukocytes as these cells do not synthesize Type 1 lactosamines (3).
Other glycosyltransferases involved in the biosynthesis of sLex have also been studied for their relevance in generating functional selectin ligands (Figure 5). Regarding sLex presentation on O-glycans, one study reported that leukocytes from mice deficient in the enzyme required for initiation of O-glycosylation, ppGalNAcT-1, showed impaired recruitment during inflammation due to a significant reduction in E- and P-selectin ligand levels (124). Mice lacking the O-glycan core 1 β3galactosyltransferase (C1GalT-I) showed dramatic loss of leukocyte rolling on E-selectin and, consequently, these leukocytes did not transmigrate into inflamed tissues (125). Transgenic mouse studies, where the O-glycan core 2 β6-N-acetylglucosaminyltransferase-I (C2GnT-I) was knocked out, also showed reduced E-selectin and P-selectin binding activity of leukocytes under static and shear-based rolling assays, with impaired leukocyte recruitment to sites of inflammation (126–128). In agreement, in human moDCs, the downregulation of C2GnT-I, with concurrent upregulation of ST3Gal-I and GalNAc α(2,6)sialyltransferase (ST6GalNAc)-II, results in a loss of the core 2 structures required for O-glycan display of sLex (Figure 5) (129). Furthermore, studies using HL-60 cells have revealed that the ST6GalNAc-II overexpression abrogates sLex cell surface display and reduces the number of adherent cells to E-selectin under flow conditions, reinforcing the notion that there exists a competition between ST6GalNAc-II and C2GnT-I for core 1 acceptors, affecting the biosynthesis of sLex-bearing core 2-O-glycan structures (Figure 5) (130). Interestingly, in mice, knockout of one of the β(1,4)galactosyltransferases (of the family of five isoenzymes) involved in Type 2 lactosamine synthesis, β(1,4)galactosyltransferase-I (β(1,4)GalT-I), showed reduced inflammatory responses and impaired P-selectin binding activity; however, the contribution of this enzyme in the synthesis of E-selectin counter-receptors remains to be elucidated (131).
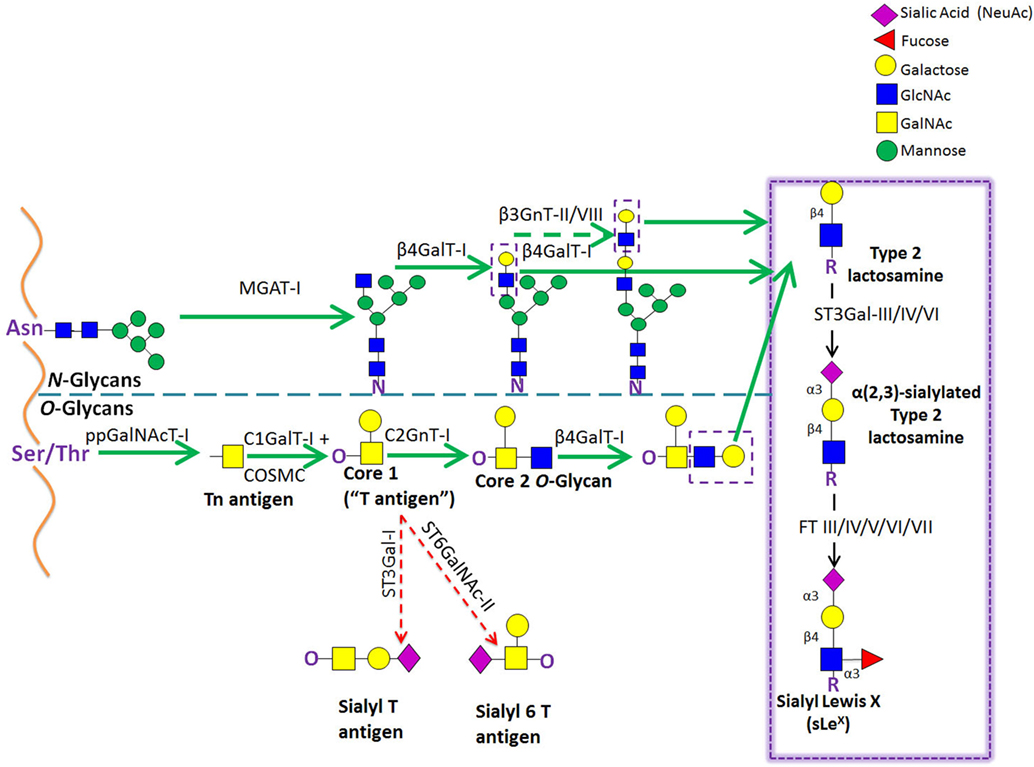
Figure 5. Schematic representation of the biosynthetic pathways leading to glycoprotein synthesis: O-linked and N-linked glycosylation. The O-glycosylation process is characterized by a stepwise sugar addition that occurs in the Golgi apparatus and involves a broad array of enzymes. This synthesis is initiated by one of the N-acetylgalactosaminyltransferase (ppGalNAcTs) family members, forming the Tn antigen. After the first sugar [N-acetylgalactosamine (GalNAc)] addition, Tn is typically elongated by Core 1 β(1,3)galactosyltransferase (Core1GalT or T synthase, whose Golgi expression requires the activity of its chaperone COSMC), creating the “Core 1” O-glycan (also known as “T antigen”). Core 1 is then further lengthened by C2GnT-I, which adds an N-acetylglucosamine (GlcNAc) to the GalNAc, forming the “Core 2” O-glycan structure. Alternatively, Core 1 sialylation, by ST3Gal-I or ST6GalNAc-II [forming sialyl-T (sT) or sialyl-6T (s6T) antigens, respectively] stops Core 2 formation. In contrast to O-glycosylation, the N-glycosylation process requires the production of an oligosaccharide precursor (GlcNAc2Man5) in the cytoplasmic face of the endoplasmic reticulum (ER) membrane. This glycan flipped in the ER lumen and is then transferred en block from a lipid donor to the Asn residue of a newly synthesized protein within the ER lumen, and then further processed in the Golgi compartment. Biosynthesis of hybrid and complex glycans is initiated by the action of MGAT-I, which adds a GlcNAc residue to the mannose (Man) present on the α(1,3)-arm of the Man5GlcNAc2 structure. Repetitive additions of galactose (Gal) and GlcNAc by β(1/4)GalT and β(1,3)GnT enzymes, respectively, can further elongate Core 2 O-glycan and hybrid- and complex-type N-glycan structures, creating the lactosaminyl type 2 chains that serve as acceptors for terminal sialofucosylation reactions.
One study has recently evaluated the contributions of N-glycans, O-glycans and glycosphingolipids (GSLs) to E-selectin binding by human myeloid cells under physiological flow conditions (132). To address this issue, O-glycan and GSL synthesis was abolished by, respectively, knocking-out the core 1 Gal transferase chaperone, i.e., the C1GalT-I-specific Molecular Chaperone (COSMC), β1,2 GlcNAc-transferase (MGAT-I), and UDP-glucose ceramide glucosyltransferase (UGCG). Notably, these studies indicate that while O-glycans are indispensable for myeloid cell binding to L- and P-selectins, N-glycans play the major role in the initial myeloid cell recruitment into E-selectin-bearing substrates, with O-glycans playing a more modest role. In addition, both glycolipids and N-glycans are responsible for the slowing down of rolling velocities that precede firm arrest (132).
Most studies that have assessed biologic modulators of E-selectin ligands in leukocytes have been performed using human and murine T cells. An array of cytokines has been shown to regulate E-selectin ligand expression via upregulation or downregulation of specific glycosyltrasferases that control sLex expression. Specifically, IL-2, IL-7, IL-15, and IL-12 increase the expression of glycosyltransferases involved in the biosynthesis of E-selectin ligand determinants, whereas IL-4 has the opposite effect (133). This selectin ligand upregulation in T cells in response to cytokine signaling was shown to be dependent on Th1 transcription factor T-bet (134) and on STAT4-mediated pathways (135). Interestingly, human myeloid cells treated with granulocyte-colony stimulating factor (G-CSF) show increased cell surface expression of E-selectin ligands associated with significant increases in gene expression of the glycosyltransferases ST3Gal-IV, FTIV, and FTVII (136).
E-Selectin Ligand Activity Displayed by Circulating MPS Subsets
Among the cells of MPS, human circulating monocytes and, to a lesser extent, human blood cDCs and moDCs are the most comprehensively analyzed group in terms of E-selectin ligand activity. In our studies, human classical monocytes (CD14++CD16−) showed significantly higher levels of sLex determinants as compared to intermediate monocytes (CD14++CD16+), whereas non-classical monocytes (CD14+CD16++) were almost devoid of sLex expression (123). Another study compared the trafficking capacity of human monocyte subsets by analyzing their ability to bind to activated endothelial monolayers, and commensurately, classical monocytes showed noticeably higher capability of adhering to reactive endothelium than did non-classical/intermediate monocytes (137). In agreement with human studies, murine classical monocytes (Ly-6Chi) exhibit greater binding to E-selectin under flow conditions and express higher levels of the scaffolds that bear sLex determinants compared to non-classical monocytes (Ly-6Clo) (138, 139). This differential pattern of E-selectin ligand display is in agreement with the specific migratory requirements among the monocyte subsets: classical monocytes are typically recruited to inflamed lesions (138, 139), whereas non-classical monocytes migrate to non-inflamed endothelium (14) upon which they patrol healthy tissues in a LFA-1-dependent manner (19). Indeed, although the first observations of non-classical monocytes were made in non-inflamed skin blood vessels (19), these cells were further described in the microvasculature of kidney under steady-state conditions (20). The patrolling profile that these cells exhibit is independent of the activation state of the endothelium, since non-classical monocytes constitutively scavenge the luminal side of non-reactive endothelium (18). Therefore, their ability to bind to endothelium seems to be independent of E-selectin receptor/ligand interactions, but, instead, appears critically regulated by LFA-1 expression and its interaction with endothelial ICAM (19, 20). Notably, although selectins play a major role in the initial adhesive contacts with endothelium surfaces, integrins can also support tethering and rolling events under flow conditions, albeit with less potency than do selectins (140, 141).
Multiple adhesion molecules are involved in monocyte attachment to endothelium. While E-selectin receptor/ligand interactions prominently mediate Step 1 events in transmigration for all leukocytes, L-selectin-dependent binding interaction have also been observed to potently mediate human peripheral blood monocyte binding to activated vascular endothelium under shear stress (142–144). Thus, even though the majority of the reports indicates that initial monocyte adhesion to activated endothelial cells is most critically dependent on E-selectin receptor/ligand interactions (123, 145–150), distinct interactions were also reported by other authors. The differences have to do with differences in the leukocyte populations under study, variations in the assay conditions employed (i.e., shear stress levels employed, rotatory shear versus fluid shear conditions, temperature, etc.), differences in the adhesion metrics (i.e., number of adhered cells, number of rolling cells, rolling velocity measurements, etc) altogether compounded by the innate biologic differences between mice and human cells, could alternatively emphasize the contribution(s) of other adhesion molecules.
Concerning DCs, human blood cDCs express high levels of sLex determinants, which allow them to tether and roll on E-selectin under flow conditions (151, 152). Importantly, in in vivo intravital microscopy studies, human blood cDCs adoptively transferred into mice were observed to roll along resting murine skin endothelium and extravasate at sites of inflammation (151). Notably, human moDCs significantly express sLex, especially on O-glycan structures. Upon maturation of moDCs with the TLR4 ligand, LPS, sLex expression is downregulated due to decreased C2GnT-I expression and upregulation of ST6GalNAc-II and ST3Gal-I (129). Biologically, these observations suggest that sLex is less relevant for transendothelial migration of TLR4-induced-mature moDCs, or that maturation is a step that follows transendothelial migration. By contrast, IFN-γ-induced maturation of moDCs leads to an upregulation of C2GnT-I, resulting in increased expression of core 2 O-glycan substrates for sLex decoration (153). These features suggest that sLex-bearing core 1-derived (or core 2) O-glycans are required for human moDC migration and are modulated according to specific maturation stimuli. Human pDCs also express sLex, allowing their recruitment to some inflamed tissues (38, 39, 154) and to reactive lymph nodes (43), a process believed to be mediated by the expression of E-selectin in HEVs (45, 47).
Glycoconjugate Structures That Display E-Selectin Ligand Determinants in Circulating MPS Cells
Several diverse and structurally singular glycostructures with E-selectin binding activity have been identified on human classical monocytes or human blood DCs. Human classical monocytes greatly display sLex decorations on an array of protein scaffolds, consisting of P-selectin glycoprotein ligand-1 (PSGL-1), CD43 and CD44, and GSLs (123), whereas human circulating DCs appear to display sLex solely on PSGL-1 (129, 155).
Cutaneous Lymphocyte Antigen
The cutaneous lymphocyte antigen (CLA) is the E-selectin-reactive glycoform of PSGL-1. PSGL-1 is a transmembrane 240-kDa homodimeric, mucin-like glycoprotein expressed on leukocytes (and, reportedly, on some activated endothelial cells) that plays a crucial role in the homing of leukocytes into inflamed tissue (156, 157). E-selectin binding activity of PSGL-1 is conferred by sialylated and fucosylated core 2-based-O-glycans that are cluster-distributed along the stalk region of the PSGL-1 extracellular domain (158). A number of studies have identified PSGL-1 as one of the several scaffolds expressed by human classical monocytes displaying E-selectin-binding activity (123, 155). On the other hand, PSGL-1 is the only known scaffold that presents sLex determinants on human circulating cDCs (151, 155) and moDCs (129). Yet, Silva et al. observed that although PSGL-1 is essential for P- and L-selectin recognition by human moDCs under fluid shear conditions, it is not mandatory for tethering to E-selectin (153). Moreover, there are reports that extravasation of murine immature DC to inflamed tissues requires both E- and P-selectin, but not PSGL-1 (159). Together, these data suggest the expression of ligands for E-selectin in addition to CLA by human and murine DCs. Still, PSGL-1 is the dominant ligand for P- and L- selectin and is the only known glycoprotein that binds all three selectins (160, 161). Accordingly, circulating monocytes that have already bound to E-selectin on inflamed endothelium can also interact with L-selectin expressed by other circulating monocytes/DCs via PSGL-1 and support their secondary capture, potentiating mononuclear phagocyte recruitment to sites of inflammation (162).
Hematopoietic Cell E- and L-Selectin Ligand
HCELL is a sialofucosylated glycoform of CD44 that exhibits potent E-selectin (and L-selectin) binding activity. CD44 is a transmembrane protein that exists in a wide variety of protein isoforms due to alternative splicing and extensive post-translational modifications (with molecular weight ranging from 80 to 220 kDa). CD44 is expressed by most mammalian cells, where it serves as the principal receptor for hyaloronic acid and participates in a broad range of cellular activities, including lymphocyte activation, leukocyte trafficking, hematopoiesis, cell growth and survival, and tumor dissemination (163). Post-translational modifications along with extensive alternative splicing allow the formation of multiple protein isoforms, expressed in a tissue-specific manner (164, 165). The standard protein isoform of CD44 (CD44s or CD44H) is encoded by mRNA transcripts comprising exons 1–5 and 16–20 (“s1–s5 and s6–s10”). CD44s is ubiquitously expressed by mammalian cells and is the form most often displayed by hematopoietic-lineage cells. In addition to CD44s, non-hematopoietic cells characteristically display CD44 variant isoforms contain peptide products of variant exons (exons “v2–v10”) in addition to the standard exon peptide products.
CD44 post-translational modifications include the addition of different glycan structures, namely glycosaminoglycans, and N- and O-glycan substitutions (166). While previous studies indicated that HCELL was only expressed by human hematopoietic stem and progenitor cells (HSPCs) (167, 168), and some hematologic (167, 169) and solid malignancies (170), HCELL was recently reported to be expressed by classical human monocytes (123). Importantly, for human HSPCs, the sLex determinant is exclusively displayed on N-glycan lactosamines on CD44s, but classical monocytes express sLex on O-glycans of CD44s (123). Because of its ability to engage E- and L-selectin under relatively high fluid shear conditions (i.e., in excess of 20 dynes/cm2 shear stress), HCELL is considered the most potent L- and E-selectin ligand expressed on mammalian cells (167, 171).
CD43E
CD43, also known as sialophorin or leukosialin, is a cell surface glycoprotein expressed by nearly all hematopoietic cells and is involved in several important processes, including cell development, activation, survival, and migration (172–176). Glycosylation of CD43 molecules with either core 1 or core 2 O-glycan structures produces the ~115 and ~135 kDa glycoforms, respectively (177). Recently, we reported that human classical monocytes display E-selectin binding activity on CD43 (123). CD43 that displays sLex and binds E-selectin is known as “CD43E” and this protein harbors sLex on O-glycans (178).
Glycosphingolipids
In contrast to results in mouse leukocytes, reports using human cells have suggested that sialofucosylated determinants displayed on lipid scaffolds can mediate adhesion to E-selectin under static and flow conditions. In native human leukocytes and in the myelocytic leukemia cell line HL-60, the glycolipid structures that display ability to bind to E-selectin consist of sialylated lactosylceramides decorated with internal fucosylated GlcNAc structures (myeloglycans) (86, 87, 179). It has been reported that the disruption of myeloglycan biosynthesis via knockdown of ceramide glucosyltransferase (UGCG) in HL-60 cells disturbed stable cell rolling and impaired transmigration across inflamed endothelial cells (180). Finally, human classical monocytes treated with a broadly active protease did not show a complete abrogation of sLex staining, suggesting that although the majority of sialofucosylated moieties required for E-selectin binding are preferentially expressed on proteins rather than lipids, a considerable amount of sialofucosylated determinants are present on glycolipids (123).
Pathophysiological Importance of the Selectins
Due to its crucial role in the transmigration of specific myeloid populations to sites of inflammation, the selectin/selectin–ligand axis is involved in the development of many acute and chronic inflammatory conditions (181, 182). In fact, cutaneous inflammatory disorders, such as allergic contact dermatitis (183), atopic dermatitis (184–188), and psoriasis (183, 184, 189–191), are known to be promoted by the upregulation of selectins on dermal microvasculature. Specifically, P- and E-selectins are upregulated in skin lesions of cutaneous inflammatory patients, which enables inflammatory mononuclear phagocyte infiltration with subsequent release of soluble cytotoxic mediators, T cell activation, and destruction of the dermal layer of skin (154, 192–194). Atherosclerosis is another chronic inflammatory disease in which the recruitment of monocytes into selectin-expressing endothelial beds constitutes a key molecular event in the pathogenesis of the disease (195). Both E-and P-selectins are expressed on human arterial luminal endothelial cells of atherosclerotic plaques (195, 196), and mouse studies have shown that E-selectin and/or P-selectin deficiency substantially reduces the formation of atherosclerotic plaques, suggesting an overlapping function of these two selectins in the development of atherosclerotic lesions (197, 198). Murine classical monocytes (Ly6Chi) preferentially migrate into activated endothelium and infiltrate developing atheromas to become atherosclerotic macrophages, inflammatory DCs, or foam cells (139). Indeed, murine classical monocytes display high levels of PSGL-1 and higher binding affinity to E-/P-selectin expressing cells than do the non-classical patrolling monocytes, which might explain why they are preferentially recruited to sites of endothelial inflammation and thrombosis (138).
Inflammatory bowel disease (199–202), multiple sclerosis (203, 204), rheumatoid arthritis (205–207), and type 1 diabetes (208, 209) are other examples of inflammatory diseases in which upregulated E-/P-selectin expression in tissue microvasculature drives myeloid cell recruitment crucial for the development of the disease. Increased E-selectin expression and mononuclear phagocyte infiltration have been similarly observed in cases of transplantation rejection, including human renal (210, 211), lung (212–214), and cardiac (215–217) rejection and in acute graft versus host disease (218–221). Recruitment of inflammatory classical monocytes (222, 223) and pDCs (38, 41, 224) to tumor-cell-activated endothelium has also been reported to be dependent on E-selectin expression, which can lead to an inhibition of the tumor-specific immune defense response and induction of tolerance (225, 226). Some studies have also indicated that inflammatory monocytes license extravasation of tumor cells via the induction of E-selectin-dependent adhesive interactions (222).
Therapeutic Strategies Targeting the Selectin/Selectin–Ligand Axis
The critical role of selectins and their ligands in the pathogenesis of many inflammatory conditions makes them potential molecular targets for therapy and, therefore, several strategies have been developed to interfere with this biology (182, 227, 228). One strategy relies on the development of pan-selectin competitive inhibitors that inhibit leukocyte/endothelial cell interaction and, therefore, cell recruitment to affected inflammatory or metastatic tissues. One example is the molecule Bimosiamose (Encysive Pharmaceuticals), a sLex mimetic that showed clinical efficacy in both asthma and psoriasis (229, 230). Other examples of pan-selectin inhibitors include sLex-peptides (231), sLex-bearing liposomes (232) or heparin oligosaccharides (233, 234). Also, GMI-127 and GMI-1271, E-selectin antagonists developed by GlycoMimetics based on the bioactive conformation of sLex in the carbohydrate-binding domain of E-selectin, have been used in treatment of sickle cell crisis (ClinicalTrials.gov Identifier: NCT00911495) and as an adjuvant for chemotherapy of hematological malignancies, including multiple myeloma and acute myeloid leukemia (NCT02811822, NCT02306291). In addition, an alternative approach involves the inhibition of the selectin ligand synthesis, either by interfering with the expression of key glycosyltransferases involved in sLex biosynthesis (235), or by using fluorinated analogs of Sia and/or Fuc residues (232, 236) that inhibit sLex synthesis. Finally, competing antibodies that bind to vascular E- and P-selectins and/or to selectin ligands expressed on the leukocyte cell surface constitute alternative methods that have been explored to prevent inflammatory exacerbation (182, 228). Indeed, a soluble form of PSGL-1 linked to the Fc portion of human IgG1 (PSGL-1-Ig) has been shown to inhibit leukocyte rolling in several disease models in mice (237–240).
On the other hand, the disruption of the selectin–leukocyte interaction has been reported to trigger severe immune-deficiency by disruption of immunosurveillance (241). Leukocyte adhesion deficiency Type II is a rare genetic disorder characterized by defective neutrophil and monocyte migration caused by a mutation in a GDP-Fuc transporter gene (242). This mutation leads to the formation of glycans that lack fucosylation, resulting in impaired leukocyte rolling and consequent leukocytosis and recurrent infections (242, 243). To overcome deficient cellular rolling, our lab developed a FT-driven sLex biosynthesis approach to enforce cell surface expression of E-selectin ligands while preserving cell viability, called glycosyltransferase-programmed stereosubstitution (GPS) (244, 245). This platform uses optimized reaction conditions, which enables the efficient α(1,3)-fucosylation of underfucosylatated sialylated type 2 lactosamine acceptors (Siaα2-3Galβ1-4GlcNAc), via a soluble α(1,3) FT, installing expression of sLex (Siaα2-3Galβ1-4GlcNAcα1-3Fuc) (3). GPS has been used to improve the recruitment of a variety of human and mouse cells into E-selectin-bearing endothelial beds, driving cell migration into bone marrow and inflammatory sites (123, 178, 208, 244, 246–248).
Concluding Remarks
Specific migratory routes and distinct localization in steady and inflammatory conditions of the different human MPS subsets suggests that they play differential roles in immunity. These cells are operational in multiple beneficial processes, including immediate antimicrobial host defense, activation of the adaptive immune system, and tissue healing processes. However, they may also contribute to the pathobiology of several inflammatory conditions. A better understanding of the molecular basis of glycosylation-dependent creation of E-selectin ligands could yield the development of novel therapeutic approaches for inflammatory diseases, or, alternatively, could yield enhanced ability to infiltrate sites where immunity is needed (e.g., tumors or infection). To our knowledge, only a limited number of studies have analyzed the functional and structural biology of the full spectrum of E-selectin ligands expressed by different circulating human MPS subsets. Future work will be required to address this issue and to elucidate how custom-modified expression of these homing receptors can be achieved to preferentially influence the specific migratory routes of the different subsets of circulating MPS cells.
Author Contributions
All authors listed have made a substantial, direct, and intellectual contribution to the work and approved it for publication.
Conflict of Interest Statement
According to the National Institutes of Health policies and procedures, the Brigham and Women’s Hospital has assigned intellectual property rights regarding HCELL and GPS to the inventor (RS), who may benefit financially if the technology is licensed. RS’s ownership interests were reviewed and are managed by the Brigham and Women’s Hospital and Partners HealthCare in accordance with their conflict of interest policy. All other authors declare no competing financial interests.
Acknowledgments
We thank Dr. Nandini Mondal and Kyle Martin for their helpful review of the manuscript.
Funding
This work was supported by the National Institutes of Health National Heart Lung Blood Institute grant PO1 HL107146 (Program of Excellence in Glycosciences) (RS), and by the Team Jobie Fund (RS) and the Faye Geronemus Leukemia Research Fund (RS). INclude Fulbright Commission fellowship (to PAV).
References
1. Haniffa M, Bigley V, Collin M. Human mononuclear phagocyte system reunited. Semin Cell Dev Biol (2015) 41:59–69. doi:10.1016/j.semcdb.2015.05.004
2. Chow A, Brown BD, Merad M. Studying the mononuclear phagocyte system in the molecular age. Nat Rev Immunol (2011) 11(11):788–98. doi:10.1038/nri3087
3. Sackstein R. Glycosyltransferase-programmed stereosubstitution (GPS) to create HCELL: engineering a roadmap for cell migration. Immunol Rev (2009) 230(1):51–74. doi:10.1111/j.1600-065X.2009.00792.x
4. Kunkel EJ, Ley K. Distinct phenotype of E-selectin-deficient mice. E-selectin is required for slow leukocyte rolling in vivo. Circ Res (1996) 79(6):1196–204. doi:10.1161/01.RES.79.6.1196
5. van Furth R, Cohn ZA. The origin and kinetics of mononuclear phagocytes. J Exp Med (1968) 128(3):415–35. doi:10.1084/jem.128.3.415
6. Jakubzick C, Gautier EL, Gibbings SL, Sojka DK, Schlitzer A, Johnson TE, et al. Minimal differentiation of classical monocytes as they survey steady-state tissues and transport antigen to lymph nodes. Immunity (2013) 39(3):599–610. doi:10.1016/j.immuni.2013.08.007
7. Epelman S, Lavine KJ, Beaudin AE, Sojka DK, Carrero JA, Calderon B, et al. Embryonic and adult-derived resident cardiac macrophages are maintained through distinct mechanisms at steady state and during inflammation. Immunity (2014) 40(1):91–104. doi:10.1016/j.immuni.2013.11.019
8. Bain CC, Scott CL, Uronen-Hansson H, Gudjonsson S, Jansson O, Grip O, et al. Resident and pro-inflammatory macrophages in the colon represent alternative context-dependent fates of the same Ly6Chi monocyte precursors. Mucosal Immunol (2013) 6(3):498–510. doi:10.1038/mi.2012.89
9. Rivollier A, He J, Kole A, Valatas V, Kelsall BL. Inflammation switches the differentiation program of Ly6Chi monocytes from antiinflammatory macrophages to inflammatory dendritic cells in the colon. J Exp Med (2012) 209(1):139–55. doi:10.1084/jem.20101387
10. Serbina NV, Jia T, Hohl TM, Pamer EG. Monocyte-mediated defense against microbial pathogens. Annu Rev Immunol (2008) 26:421–52. doi:10.1146/annurev.immunol.26.021607.090326
11. Gordon S, Taylor PR. Monocyte and macrophage heterogeneity. Nat Rev Immunol (2005) 5(12):953–64. doi:10.1038/nri1733
12. Shi C, Pamer EG. Monocyte recruitment during infection and inflammation. Nat Rev Immunol (2011) 11(11):762–74. doi:10.1038/nri3070
13. Stansfield BK, Ingram DA. Clinical significance of monocyte heterogeneity. Clin Transl Med (2015) 4:5. doi:10.1186/s40169-014-0040-3
14. Geissmann F, Jung S, Littman DR. Blood monocytes consist of two principal subsets with distinct migratory properties. Immunity (2003) 19(1):71–82. doi:10.1016/S1074-7613(03)00174-2
15. Ziegler-Heitbrock L, Ancuta P, Crowe S, Dalod M, Grau V, Hart DN, et al. Nomenclature of monocytes and dendritic cells in blood. Blood (2010) 116(16):e74–80. doi:10.1182/blood-2010-02-258558
16. Kratofil RM, Kubes P, Deniset JF. Monocyte conversion during inflammation and injury. Arterioscler Thromb Vasc Biol (2016) 37(1):35–42. doi:10.1161/ATVBAHA.116.308198
17. Ziegler-Heitbrock L, Hofer TP. Toward a refined definition of monocyte subsets. Front Immunol (2013) 4:23. doi:10.3389/fimmu.2013.00023
18. Thomas G, Tacke R, Hedrick CC, Hanna RN. Nonclassical patrolling monocyte function in the vasculature. Arterioscler Thromb Vasc Biol (2015) 35(6):1306–16. doi:10.1161/ATVBAHA.114.304650
19. Auffray C, Fogg D, Garfa M, Elain G, Join-Lambert O, Kayal S, et al. Monitoring of blood vessels and tissues by a population of monocytes with patrolling behavior. Science (2007) 317(5838):666–70. doi:10.1126/science.1142883
20. Carlin LM, Stamatiades EG, Auffray C, Hanna RN, Glover L, Vizcay-Barrena G, et al. Nr4a1-dependent Ly6C(low) monocytes monitor endothelial cells and orchestrate their disposal. Cell (2013) 153(2):362–75. doi:10.1016/j.cell.2013.03.010
21. Cros J, Cagnard N, Woollard K, Patey N, Zhang SY, Senechal B, et al. Human CD14dim monocytes patrol and sense nucleic acids and viruses via TLR7 and TLR8 receptors. Immunity (2010) 33(3):375–86. doi:10.1016/j.immuni.2010.08.012
22. Yang J, Zhang L, Yu C, Yang XF, Wang H. Monocyte and macrophage differentiation: circulation inflammatory monocyte as biomarker for inflammatory diseases. Biomark Res (2014) 2(1):1. doi:10.1186/2050-7771-2-1
23. Passos S, Carvalho LP, Costa RS, Campos TM, Novais FO, Magalhaes A, et al. Intermediate monocytes contribute to pathologic immune response in Leishmania braziliensis infections. J Infect Dis (2015) 211(2):274–82. doi:10.1093/infdis/jiu439
24. Tolouei Semnani R, Moore V, Bennuru S, McDonald-Fleming R, Ganesan S, Cotton R, et al. Human monocyte subsets at homeostasis and their perturbation in numbers and function in filarial infection. Infect Immun (2014) 82(11):4438–46. doi:10.1128/IAI.01973-14
25. Lin KW, Jacek T, Jacek R. Dendritic cells heterogeneity and its role in cancer immunity. J Cancer Res Ther (2006) 2(2):35–40. doi:10.4103/0973-1482.25847
26. Ishikawa F, Niiro H, Iino T, Yoshida S, Saito N, Onohara S, et al. The developmental program of human dendritic cells is operated independently of conventional myeloid and lymphoid pathways. Blood (2007) 110(10):3591–660. doi:10.1182/blood-2007-02-071613
27. Shortman K, Naik SH. Steady-state and inflammatory dendritic-cell development. Nat Rev Immunol (2007) 7(1):19–30. doi:10.1038/nri1996
28. Boltjes A, van Wijk F. Human dendritic cell functional specialization in steady-state and inflammation. Front Immunol (2014) 5:131. doi:10.3389/fimmu.2014.00131
29. Hawiger D, Inaba K, Dorsett Y, Guo M, Mahnke K, Rivera M, et al. Dendritic cells induce peripheral T cell unresponsiveness under steady state conditions in vivo. J Exp Med (2001) 194(6):769–79. doi:10.1084/jem.194.6.769
30. Huang FP, Platt N, Wykes M, Major JR, Powell TJ, Jenkins CD, et al. A discrete subpopulation of dendritic cells transports apoptotic intestinal epithelial cells to T cell areas of mesenteric lymph nodes. J Exp Med (2000) 191(3):435–44. doi:10.1084/jem.191.3.435
31. Matsuno K, Ezaki T, Kudo S, Uehara Y. A life stage of particle-laden rat dendritic cells in vivo: their terminal division, active phagocytosis, and translocation from the liver to the draining lymph. J Exp Med (1996) 183(4):1865–78. doi:10.1084/jem.183.4.1865
32. Crespo HJ, Lau JT, Videira PA. Dendritic cells: a spot on sialic Acid. Front Immunol (2013) 4:491. doi:10.3389/fimmu.2013.00491
33. Segura E, Valladeau-Guilemond J, Donnadieu MH, Sastre-Garau X, Soumelis V, Amigorena S. Characterization of resident and migratory dendritic cells in human lymph nodes. J Exp Med (2012) 209(4):653–60. doi:10.1084/jem.20111457
34. Segura E, Amigorena S. Inflammatory dendritic cells in mice and humans. Trends Immunol (2013) 34(9):440–5. doi:10.1016/j.it.2013.06.001
35. Segura E, Amigorena S. Identification of human inflammatory dendritic cells. Oncoimmunology (2013) 2(5):e23851. doi:10.4161/onci.23851
36. Serbina NV, Salazar-Mather TP, Biron CA, Kuziel WA, Pamer EG. TNF/iNOS-producing dendritic cells mediate innate immune defense against bacterial infection. Immunity (2003) 19(1):59–70. doi:10.1016/S1074-7613(03)00171-7
37. Mathan TS, Figdor CG, Buschow SI. Human plasmacytoid dendritic cells: from molecules to intercellular communication network. Front Immunol (2013) 4:372. doi:10.3389/fimmu.2013.00372
38. Salio M, Cella M, Vermi W, Facchetti F, Palmowski MJ, Smith CL, et al. Plasmacytoid dendritic cells prime IFN-gamma-secreting melanoma-specific CD8 lymphocytes and are found in primary melanoma lesions. Eur J Immunol (2003) 33(4):1052–62. doi:10.1002/eji.200323676
39. Hartmann E, Wollenberg B, Rothenfusser S, Wagner M, Wellisch D, Mack B, et al. Identification and functional analysis of tumor-infiltrating plasmacytoid dendritic cells in head and neck cancer. Cancer Res (2003) 63(19):6478–87.
40. Zou W, Machelon V, Coulomb-L’Hermin A, Borvak J, Nome F, Isaeva T, et al. Stromal-derived factor-1 in human tumors recruits and alters the function of plasmacytoid precursor dendritic cells. Nat Med (2001) 7(12):1339–46. doi:10.1038/nm1201-1339
41. Vermi W, Bonecchi R, Facchetti F, Bianchi D, Sozzani S, Festa S, et al. Recruitment of immature plasmacytoid dendritic cells (plasmacytoid monocytes) and myeloid dendritic cells in primary cutaneous melanomas. J Pathol (2003) 200(2):255–68. doi:10.1002/path.1344
42. Conrad C, Meller S, Gilliet M. Plasmacytoid dendritic cells in the skin: to sense or not to sense nucleic acids. Semin Immunol (2009) 21(3):101–9. doi:10.1016/j.smim.2009.01.004
43. Cella M, Jarrossay D, Facchetti F, Alebardi O, Nakajima H, Lanzavecchia A, et al. Plasmacytoid monocytes migrate to inflamed lymph nodes and produce large amounts of type I interferon. Nat Med (1999) 5(8):919–23. doi:10.1038/11360
44. Banchereau J, Pulendran B, Steinman R, Palucka K. Will the making of plasmacytoid dendritic cells in vitro help unravel their mysteries? J Exp Med (2000) 192(12):F39–44. doi:10.1084/jem.192.12.F39
45. Yoneyama H, Matsuno K, Zhang Y, Nishiwaki T, Kitabatake M, Ueha S, et al. Evidence for recruitment of plasmacytoid dendritic cell precursors to inflamed lymph nodes through high endothelial venules. Int Immunol (2004) 16(7):915–28. doi:10.1093/intimm/dxh093
46. Yrlid U, Cerovic V, Milling S, Jenkins CD, Zhang J, Crocker PR, et al. Plasmacytoid dendritic cells do not migrate in intestinal or hepatic lymph. J Immunol (2006) 177(9):6115–21. doi:10.4049/jimmunol.177.9.6115
47. Diacovo TG, Blasius AL, Mak TW, Cella M, Colonna M. Adhesive mechanisms governing interferon-producing cell recruitment into lymph nodes. J Exp Med (2005) 202(5):687–96. doi:10.1084/jem.20051035
48. Ginhoux F, Greter M, Leboeuf M, Nandi S, See P, Gokhan S, et al. Fate mapping analysis reveals that adult microglia derive from primitive macrophages. Science (2010) 330(6005):841–5. doi:10.1126/science.1194637
49. Sieweke MH, Allen JE. Beyond stem cells: self-renewal of differentiated macrophages. Science (2013) 342(6161):1242974. doi:10.1126/science.1242974
50. Hoefsmit EC. Macrophages, Langerhans cells, interdigitating and dendritic accessory cells: a summary. Adv Exp Med Biol (1982) 149:463–8. doi:10.1007/978-1-4684-9066-4_65
51. Gordon S, Plüddemann A, Martinez Estrada F. Macrophage heterogeneity in tissues: phenotypic diversity and functions. Immunol Rev (2014) 262(1):36–55. doi:10.1111/imr.12223
52. Kindt TJ, Goldsby RA, Osborne BA. Overview of the immune system. Kuby Immunology, Chapter 1, 6th ed. New York, United States: WH Freeman & Company (2007). 574 p.
53. Mosser DM, Edwards JP. Exploring the full spectrum of macrophage activation. Nat Rev Immunol (2008) 8(12):958–69. doi:10.1038/nri2448
54. Italiani P, Boraschi D. From monocytes to M1/M2 macrophages: phenotypical vs. functional differentiation. Front Immunol (2014) 5:514. doi:10.3389/fimmu.2014.00514
55. Ferenbach D, Hughes J. Macrophages and dendritic cells: what is the difference? Kidney Int (2008) 74(1):5–7. doi:10.1038/ki.2008.189
56. Butcher EC. Leukocyte-endothelial cell recognition: three (or more) steps to specificity and diversity. Cell (1991) 67(6):1033–6. doi:10.1016/0092-8674(91)90279-8
57. Ley K, Laudanna C, Cybulsky MI, Nourshargh S. Getting to the site of inflammation: the leukocyte adhesion cascade updated. Nat Rev Immunol (2007) 7(9):678–89. doi:10.1038/nri2156
58. Vestweber D. Novel insights into leukocyte extravasation. Curr Opin Hematol (2012) 19(3):212–7. doi:10.1097/MOH.0b013e3283523e78
59. Jacobs PP, Sackstein R. CD44 and HCELL: preventing hematogenous metastasis at step 1. FEBS Lett (2011) 585(20):3148–58. doi:10.1016/j.febslet.2011.07.039
60. Lawrence MB, Kansas GS, Kunkel EJ, Ley K. Threshold levels of fluid shear promote leukocyte adhesion through selectins (CD62L,P,E). J Cell Biol (1997) 136(3):717–27. doi:10.1083/jcb.136.3.717
61. Tedder TF, Steeber DA, Chen A, Engel P. The selectins: vascular adhesion molecules. FASEB J (1995) 9(10):866–73.
62. Tedder TF, Isaacs CM, Ernst TJ, Demetri GD, Adler DA, Disteche CM. Isolation and chromosomal localization of cDNAs encoding a novel human lymphocyte cell surface molecule, LAM-1. Homology with the mouse lymphocyte homing receptor and other human adhesion proteins. J Exp Med (1989) 170(1):123–33. doi:10.1084/jem.170.1.123
63. Bevilacqua MP, Stengelin S, Gimbrone MA Jr, Seed B. Endothelial leukocyte adhesion molecule 1: an inducible receptor for neutrophils related to complement regulatory proteins and lectins. Science (1989) 243(4895):1160–5. doi:10.1126/science.2466335
64. Johnston GI, Cook RG, McEver RP. Cloning of GMP-140, a granule membrane protein of platelets and endothelium: sequence similarity to proteins involved in cell adhesion and inflammation. Cell (1989) 56(6):1033–44. doi:10.1016/0092-8674(89)90636-3
65. Lasky LA. Selectin-carbohydrate interactions and the initiation of the inflammatory response. Annu Rev Biochem (1995) 64:113–39. doi:10.1146/annurev.bi.64.070195.000553
66. Stamper HB Jr, Woodruff JJ. Lymphocyte homing into lymph nodes: in vitro demonstration of the selective affinity of recirculating lymphocytes for high-endothelial venules. J Exp Med (1976) 144(3):828–33. doi:10.1084/jem.144.3.828
67. Gallatin WM, Weissman IL, Butcher EC. A cell-surface molecule involved in organ-specific homing of lymphocytes. Nature (1983) 304(5921):30–4. doi:10.1038/304030a0
68. Smalley DM, Ley K. L-selectin: mechanisms and physiological significance of ectodomain cleavage. J Cell Mol Med (2005) 9(2):255–66. doi:10.1111/j.1582-4934.2005.tb00354.x
69. Rosen SD. Ligands for L-selectin: homing, inflammation, and beyond. Annu Rev Immunol (2004) 22:129–56. doi:10.1146/annurev.immunol.21.090501.080131
70. McEver RP, Martin MN. A monoclonal antibody to a membrane glycoprotein binds only to activated platelets. J Biol Chem (1984) 259(15):9799–804.
71. Stenberg PE, McEver RP, Shuman MA, Jacques YV, Bainton DF. A platelet alpha-granule membrane protein (GMP-140) is expressed on the plasma membrane after activation. J Cell Biol (1985) 101(3):880–6. doi:10.1083/jcb.101.3.880
72. Hsu-Lin S, Berman CL, Furie BC, August D, Furie B. A platelet membrane protein expressed during platelet activation and secretion. Studies using a monoclonal antibody specific for thrombin-activated platelets. J Biol Chem (1984) 259(14):9121–6.
73. Sanders WE, Wilson RW, Ballantyne CM, Beaudet AL. Molecular cloning and analysis of in vivo expression of murine P-selectin. Blood (1992) 80(3):795–800.
74. Pan J, Xia L, Yao L, McEver RP. Tumor necrosis factor-alpha- or lipopolysaccharide-induced expression of the murine P-selectin gene in endothelial cells involves novel kappaB sites and a variant activating transcription factor/cAMP response element. J Biol Chem (1998) 273(16):10068–77. doi:10.1074/jbc.273.16.10068
75. Sperandio M. Selectins and glycosyltransferases in leukocyte rolling in vivo. FEBS J (2006) 273(19):4377–89. doi:10.1111/j.1742-4658.2006.05437.x
76. Pan J, Xia L, McEver RP. Comparison of promoters for the murine and human P-selectin genes suggests species-specific and conserved mechanisms for transcriptional regulation in endothelial cells. J Biol Chem (1998) 273(16):10058–67. doi:10.1074/jbc.273.16.10058
77. Yao L, Setiadi H, Xia L, Laszik Z, Taylor FB, McEver RP. Divergent inducible expression of P-selectin and E-selectin in mice and primates. Blood (1999) 94(11):3820–8.
78. Bevilacqua MP, Pober JS, Mendrick DL, Cotran RS, Gimbrone MA. Identification of an inducible endothelial-leukocyte adhesion molecule. Proc Natl Acad Sci U S A (1987) 84(24):9238–42. doi:10.1073/pnas.84.24.9238
79. Sackstein R. Glycoengineering of HCELL, the human bone marrow homing receptor: sweetly programming cell migration. Ann Biomed Eng (2012) 40(4):766–76. doi:10.1007/s10439-011-0461-8
80. Vestweber D, Blanks JE. Mechanisms that regulate the function of the selectins and their ligands. Physiol Rev (1999) 79(1):181–213.
81. Lewis H, Kaszubska W, DeLamarter JF, Whelan J. Cooperativity between two NF-kappa B complexes, mediated by high-mobility-group protein I(Y), is essential for cytokine-induced expression of the E-selectin promoter. Mol Cell Biol (1994) 14(9):5701–9. doi:10.1128/MCB.14.9.5701
82. Ley K, Allietta M, Bullard DC, Morgan S. Importance of E-selectin for firm leukocyte adhesion in vivo. Circ Res (1998) 83(3):287–94. doi:10.1161/01.RES.83.3.287
83. Phillips ML, Nudelman E, Gaeta FC, Perez M, Singhal AK, Hakomori S, et al. ELAM-1 mediates cell adhesion by recognition of a carbohydrate ligand, sialyl-Lex. Science (1990) 250(4984):1130–2. doi:10.1126/science.1701274
84. Tyrrell D, James P, Rao N, Foxall C, Abbas S, Dasgupta F, et al. Structural requirements for the carbohydrate ligand of E-selectin. Proc Natl Acad Sci U S A (1991) 88(22):10372–6. doi:10.1073/pnas.88.22.10372
85. Munro JM, Lo SK, Corless C, Robertson MJ, Lee NC, Barnhill RL, et al. Expression of sialyl-Lewis X, an E-selectin ligand, in inflammation, immune processes, and lymphoid tissues. Am J Pathol (1992) 141(6):1397–408.
86. Handa K, Stroud MR, Hakomori S. Sialosyl-fucosyl poly-LacNAc without the sialosyl-Lex epitope as the physiological myeloid cell ligand in E-selectin-dependent adhesion: studies under static and dynamic flow conditions. Biochemistry (1997) 36(41):12412–20. doi:10.1021/bi971181t
87. Stroud MR, Handa K, Salyan ME, Ito K, Levery SB, Hakomori S, et al. Monosialogangliosides of human myelogenous leukemia HL60 cells and normal human leukocytes. 2. Characterization of E-selectin binding fractions, and structural requirements for physiological binding to E-selectin. Biochemistry (1996) 35(3):770–8. doi:10.1021/bi952461g
88. Nimrichter L, Burdick MM, Aoki K, Laroy W, Fierro MA, Hudson SA, et al. E-selectin receptors on human leukocytes. Blood (2008) 112(9):3744–52. doi:10.1182/blood-2008-04-149641
89. Ugorski M, Laskowska A. Sialyl Lewis(a): a tumor-associated carbohydrate antigen involved in adhesion and metastatic potential of cancer cells. Acta Biochim Pol (2002) 49(2):303–11.
90. Yuen CT, Bezouska K, O’Brien J, Stoll M, Lemoine R, Lubineau A, et al. Sulfated blood group Lewis(a). A superior oligosaccharide ligand for human E-selectin. J Biol Chem (1994) 269(3):1595–8.
91. Yuen CT, Lawson AM, Chai W, Larkin M, Stoll MS, Stuart AC, et al. Novel sulfated ligands for the cell adhesion molecule E-selectin revealed by the neoglycolipid technology among O-linked oligosaccharides on an ovarian cystadenoma glycoprotein. Biochemistry (1992) 31(38):9126–31. doi:10.1021/bi00153a003
92. Grinnell BW, Hermann RB, Yan SB. Human protein C inhibits selectin-mediated cell adhesion: role of unique fucosylated oligosaccharide. Glycobiology (1994) 4(2):221–5. doi:10.1093/glycob/4.2.221
93. Lowe JB. Glycan-dependent leukocyte adhesion and recruitment in inflammation. Curr Opin Cell Biol (2003) 15(5):531–8. doi:10.1016/j.ceb.2003.08.002
94. Lowe JB. Glycosylation in the control of selectin counter-receptor structure and function. Immunol Rev (2002) 186:19–36. doi:10.1034/j.1600-065X.2002.18603.x
95. Dall’Olio F, Chiricolo M. Sialyltransferases in cancer. Glycoconj J (2001) 18(11–12):841–50. doi:10.1023/A:1022288022969
96. Cazet A, Julien S, Bobowski M, Burchell J, Delannoy P. Tumour-associated carbohydrate antigens in breast cancer. Breast Cancer Res (2010) 12(3):204. doi:10.1186/bcr2577
97. Harduin-Lepers A, Vallejo-Ruiz V, Krzewinski-Recchi MA, Samyn-Petit B, Julien S, Delannoy P. The human sialyltransferase family. Biochimie (2001) 83(8):727–37. doi:10.1016/S0300-9084(01)01301-3
98. Sasaki K, Watanabe E, Kawashima K, Sekine S, Dohi T, Oshima M, et al. Expression cloning of a novel Gal beta (1-3/1-4) GlcNAc alpha 2,3-sialyltransferase using lectin resistance selection. J Biol Chem (1993) 268(30):22782–7.
99. Kitagawa H, Paulson JC. Cloning of a novel alpha 2,3-sialyltransferase that sialylates glycoprotein and glycolipid carbohydrate groups. J Biol Chem (1994) 269(2):1394–401.
100. Okajima T, Fukumoto S, Miyazaki H, Ishida H, Kiso M, Furukawa K, et al. Molecular cloning of a novel alpha2,3-sialyltransferase (ST3Gal VI) that sialylates type II lactosamine structures on glycoproteins and glycolipids. J Biol Chem (1999) 274(17):11479–86. doi:10.1074/jbc.274.17.11479
101. de Vries T, Knegtel RM, Holmes EH, Macher BA. Fucosyltransferases: structure/function studies. Glycobiology (2001) 11(10):119R–28R. doi:10.1093/glycob/11.10.119R
102. Mollicone R, Reguigne I, Kelly RJ, Fletcher A, Watt J, Chatfield S, et al. Molecular basis for Lewis alpha(1,3/1,4)-fucosyltransferase gene deficiency (FUT3) found in Lewis-negative Indonesian pedigrees. J Biol Chem (1994) 269(33):20987–94.
103. Kukowska-Latallo JF, Larsen RD, Nair RP, Lowe JB. A cloned human cDNA determines expression of a mouse stage-specific embryonic antigen and the Lewis blood group alpha(1,3/1,4)fucosyltransferase. Genes Dev (1990) 4(8):1288–303. doi:10.1101/gad.4.8.1288
104. Weston BW, Nair RP, Larsen RD, Lowe JB. Isolation of a novel human alpha (1,3)fucosyltransferase gene and molecular comparison to the human Lewis blood group alpha (1,3/1,4)fucosyltransferase gene. Syntenic, homologous, nonallelic genes encoding enzymes with distinct acceptor substrate specificities. J Biol Chem (1992) 267(6):4152–60.
105. Lowe JB, Kukowska-Latallo JF, Nair RP, Larsen RD, Marks RM, Macher BA, et al. Molecular cloning of a human fucosyltransferase gene that determines expression of the Lewis x and VIM-2 epitopes but not ELAM-1-dependent cell adhesion. J Biol Chem (1991) 266(26):17467–77.
106. Nakayama F, Nishihara S, Iwasaki H, Kudo T, Okubo R, Kaneko M, et al. CD15 expression in mature granulocytes is determined by alpha 1,3-fucosyltransferase IX, but in promyelocytes and monocytes by alpha 1,3-fucosyltransferase IV. J Biol Chem (2001) 276(19):16100–6. doi:10.1074/jbc.M007272200
107. Goelz S, Kumar R, Potvin B, Sundaram S, Brickelmaier M, Stanley P. Differential expression of an E-selectin ligand (SLex) by two Chinese hamster ovary cell lines transfected with the same alpha (1,3)-fucosyltransferase gene (ELFT). J Biol Chem (1994) 269(2):1033–40.
108. Barthel SR, Wiese GK, Cho J, Opperman MJ, Hays DL, Siddiqui J, et al. Alpha 1,3 fucosyltransferases are master regulators of prostate cancer cell trafficking. Proc Natl Acad Sci U S A (2009) 106(46):19491–6. doi:10.1073/pnas.0906074106
109. De Vries T, Palcic MP, Schoenmakers PS, Van Den Eijnden DH, Joziasse DH. Acceptor specificity of GDP-Fuc:Gal beta 1 – >4GlcNAc-R alpha 3-fucosyltransferase VI (FucT VI) expressed in insect cells as soluble, secreted enzyme. Glycobiology (1997) 7(7):921–7. doi:10.1093/glycob/7.7.921
110. Weston BW, Smith PL, Kelly RJ, Lowe JB. Molecular cloning of a fourth member of a human alpha (1,3)fucosyltransferase gene family. Multiple homologous sequences that determine expression of the Lewis x, sialyl Lewis x, and difucosyl sialyl Lewis x epitopes. J Biol Chem (1992) 267(34):24575–84.
111. Britten CJ, van den Eijnden DH, McDowell W, Kelly VA, Witham SJ, Edbrooke MR, et al. Acceptor specificity of the human leukocyte alpha3 fucosyltransferase: role of FucT-VII in the generation of selectin ligands. Glycobiology (1998) 8(4):321–7. doi:10.1093/glycob/8.4.321
112. Sasaki K, Kurata K, Funayama K, Nagata M, Watanabe E, Ohta S, et al. Expression cloning of a novel alpha 1,3-fucosyltransferase that is involved in biosynthesis of the sialyl Lewis x carbohydrate determinants in leukocytes. J Biol Chem (1994) 269(20):14730–7.
113. Ellies LG, Sperandio M, Underhill GH, Yousif J, Smith M, Priatel JJ, et al. Sialyltransferase specificity in selectin ligand formation. Blood (2002) 100(10):3618–25. doi:10.1182/blood-2002-04-1007
114. Yang WH, Nussbaum C, Grewal PK, Marth JD, Sperandio M. Coordinated roles of ST3Gal-VI and ST3Gal-IV sialyltransferases in the synthesis of selectin ligands. Blood (2012) 120(5):1015–26. doi:10.1182/blood-2012-04-424366
115. Mondal N, Buffone A, Stolfa G, Antonopoulos A, Lau JT, Haslam SM, et al. ST3Gal-4 is the primary sialyltransferase regulating the synthesis of E-, P-, and L-selectin ligands on human myeloid leukocytes. Blood (2015) 125(4):687–96. doi:10.1182/blood-2014-07-588590
116. Maly P, Thall A, Petryniak B, Rogers CE, Smith PL, Marks RM, et al. The alpha(1,3)fucosyltransferase Fuc-TVII controls leukocyte trafficking through an essential role in L-, E-, and P-selectin ligand biosynthesis. Cell (1996) 86(4):643–53. doi:10.1016/S0092-8674(00)80137-3
117. Smithson G, Rogers CE, Smith PL, Scheidegger EP, Petryniak B, Myers JT, et al. Fuc-TVII is required for T helper 1 and T cytotoxic 1 lymphocyte selectin ligand expression and recruitment in inflammation, and together with Fuc-TIV regulates naive T cell trafficking to lymph nodes. J Exp Med (2001) 194(5):601–14. doi:10.1084/jem.194.5.601
118. Homeister JW, Thall AD, Petryniak B, Malý P, Rogers CE, Smith PL, et al. The alpha(1,3)fucosyltransferases FucT-IV and FucT-VII exert collaborative control over selectin-dependent leukocyte recruitment and lymphocyte homing. Immunity (2001) 15(1):115–26. doi:10.1016/S1074-7613(01)00166-2
119. Weninger W, Ulfman LH, Cheng G, Souchkova N, Quackenbush EJ, Lowe JB, et al. Specialized contributions by alpha(1,3)-fucosyltransferase-IV and FucT-VII during leukocyte rolling in dermal microvessels. Immunity (2000) 12(6):665–76. doi:10.1016/S1074-7613(00)80217-4
120. Huang MC, Laskowska A, Vestweber D, Wild MK. The alpha (1,3)-fucosyltransferase Fuc-TIV, but not Fuc-TVII, generates sialyl Lewis X-like epitopes preferentially on glycolipids. J Biol Chem (2002) 277(49):47786–95. doi:10.1074/jbc.M208283200
121. Buffone A, Mondal N, Gupta R, McHugh KP, Lau JT, Neelamegham S. Silencing α1,3-fucosyltransferases in human leukocytes reveals a role for FUT9 enzyme during E-selectin-mediated cell adhesion. J Biol Chem (2013) 288(3):1620–33. doi:10.1074/jbc.M112.400929
122. Kaneko M, Kudo T, Iwasaki H, Ikehara Y, Nishihara S, Nakagawa S, et al. Alpha1,3-fucosyltransferase IX (Fuc-TIX) is very highly conserved between human and mouse; molecular cloning, characterization and tissue distribution of human Fuc-TIX. FEBS Lett (1999) 452(3):237–42. doi:10.1016/S0014-5793(99)00640-7
123. Silva M, Fung RKF, Donnelly CB, Videira PA, Sackstein R. Cell-specific variation in E-selectin ligand expression among human peripheral blood mononuclear cells: implications for immunosurveillance and pathobiology. J Immunol (2017) 198(9):3576–87. doi:10.4049/jimmunol.1601636
124. Tenno M, Ohtsubo K, Hagen FK, Ditto D, Zarbock A, Schaerli P, et al. Initiation of protein O glycosylation by the polypeptide GalNAcT-1 in vascular biology and humoral immunity. Mol Cell Biol (2007) 27(24):8783–96. doi:10.1128/MCB.01204-07
125. Yago T, Fu J, McDaniel JM, Miner JJ, McEver RP, Xia L. Core 1-derived O-glycans are essential E-selectin ligands on neutrophils. Proc Natl Acad Sci U S A (2010) 107(20):9204–9. doi:10.1073/pnas.1003110107
126. Ellies LG, Tsuboi S, Petryniak B, Lowe JB, Fukuda M, Marth JD. Core 2 oligosaccharide biosynthesis distinguishes between selectin ligands essential for leukocyte homing and inflammation. Immunity (1998) 9(6):881–90. doi:10.1016/S1074-7613(00)80653-6
127. Sperandio M, Thatte A, Foy D, Ellies LG, Marth JD, Ley K. Severe impairment of leukocyte rolling in venules of core 2 glucosaminyltransferase-deficient mice. Blood (2001) 97(12):3812–9. doi:10.1182/blood.V97.12.3812
128. Snapp KR, Heitzig CE, Ellies LG, Marth JD, Kansas GS. Differential requirements for the O-linked branching enzyme core 2 beta1-6-N-glucosaminyltransferase in biosynthesis of ligands for E-selectin and P-selectin. Blood (2001) 97(12):3806–11. doi:10.1182/blood.V97.12.3806
129. Julien S, Grimshaw MJ, Sutton-Smith M, Coleman J, Morris HR, Dell A, et al. Sialyl-Lewis(x) on P-selectin glycoprotein ligand-1 is regulated during differentiation and maturation of dendritic cells: a mechanism involving the glycosyltransferases C2GnT1 and ST3Gal I. J Immunol (2007) 179(9):5701–10. doi:10.4049/jimmunol.179.9.5701
130. Lo CY, Antonopoulos A, Gupta R, Qu J, Dell A, Haslam SM, et al. Competition between core-2 GlcNAc-transferase and ST6GalNAc-transferase regulates the synthesis of the leukocyte selectin ligand on human P-selectin glycoprotein ligand-1. J Biol Chem (2013) 288(20):13974–87. doi:10.1074/jbc.M113.463653
131. Asano M, Nakae S, Kotani N, Shirafuji N, Nambu A, Hashimoto N, et al. Impaired selectin-ligand biosynthesis and reduced inflammatory responses in beta-1,4-galactosyltransferase-I-deficient mice. Blood (2003) 102(5):1678–85. doi:10.1182/blood-2003-03-0836
132. Stolfa G, Mondal N, Zhu Y, Yu X, Buffone A Jr, Neelamegham S. Using CRISPR-Cas9 to quantify the contributions of O-glycans, N-glycans and glycosphingolipids to human leukocyte-endothelium adhesion. Sci Rep (2016) 6:30392. doi:10.1038/srep30392
133. Wagers AJ, Waters CM, Stoolman LM, Kansas GS. Interleukin 12 and interleukin 4 control T cell adhesion to endothelial selectins through opposite effects on alpha1, 3-fucosyltransferase VII gene expression. J Exp Med (1998) 188(12):2225–31. doi:10.1084/jem.188.12.2225
134. Underhill GH, Zisoulis DG, Kolli KP, Ellies LG, Marth JD, Kansas GS. A crucial role for T-bet in selectin ligand expression in T helper 1 (Th1) cells. Blood (2005) 106(12):3867–73. doi:10.1182/blood-2005-03-0984
135. Lim YC, Xie H, Come CE, Alexander SI, Grusby MJ, Lichtman AH, et al. IL-12, STAT4-dependent up-regulation of CD4(+) T cell core 2 beta-1,6-n-acetylglucosaminyltransferase, an enzyme essential for biosynthesis of P-selectin ligands. J Immunol (2001) 167(8):4476–84. doi:10.4049/jimmunol.167.8.4476
136. Dagia NM, Gadhoum SZ, Knoblauch CA, Spencer JA, Zamiri P, Lin CP, et al. G-CSF induces E-selectin ligand expression on human myeloid cells. Nat Med (2006) 12(10):1185–90. doi:10.1038/nm1470
137. Chimen M, Yates CM, McGettrick HM, Ward LS, Harrison MJ, Apta B, et al. Monocyte subsets coregulate inflammatory responses by integrated signaling through TNF and IL-6 at the endothelial cell interface. J Immunol (2017) 198(7):2834–43. doi:10.4049/jimmunol.1601281
138. An G, Wang H, Tang R, Yago T, McDaniel JM, McGee S, et al. P-selectin glycoprotein ligand-1 is highly expressed on Ly-6Chi monocytes and a major determinant for Ly-6Chi monocyte recruitment to sites of atherosclerosis in mice. Circulation (2008) 117(25):3227–37. doi:10.1161/CIRCULATIONAHA.108.771048
139. Swirski FK, Libby P, Aikawa E, Alcaide P, Luscinskas FW, Weissleder R, et al. Ly-6Chi monocytes dominate hypercholesterolemia-associated monocytosis and give rise to macrophages in atheromata. J Clin Invest (2007) 117(1):195–205. doi:10.1172/JCI29950
140. Alon R, Kassner PD, Carr MW, Finger EB, Hemler ME, Springer TA. The integrin VLA-4 supports tethering and rolling in flow on VCAM-1. J Cell Biol (1995) 128(6):1243–53. doi:10.1083/jcb.128.6.1243
141. Berlin C, Bargatze RF, Campbell JJ, von Andrian UH, Szabo MC, Hasslen SR, et al. alpha 4 integrins mediate lymphocyte attachment and rolling under physiologic flow. Cell (1995) 80(3):413–22. doi:10.1016/0092-8674(95)90491-3
142. Spertini O, Luscinskas FW, Gimbrone MA Jr, Tedder TF. Monocyte attachment to activated human vascular endothelium in vitro is mediated by leukocyte adhesion molecule-1 (L-selectin) under nonstatic conditions. J Exp Med (1992) 175(6):1789–92. doi:10.1084/jem.175.6.1789
143. Luscinskas FW, Kansas GS, Ding H, Pizcueta P, Schleiffenbaum BE, Tedder TF, et al. Monocyte rolling, arrest and spreading on IL-4-activated vascular endothelium under flow is mediated via sequential action of L-selectin, beta 1-integrins, and beta 2-integrins. J Cell Biol (1994) 125(6):1417–27. doi:10.1083/jcb.125.6.1417
144. Luscinskas FW, Ding H, Tan P, Cumming D, Tedder TF, Gerritsen ME. L- and P-selectins, but not CD49d (VLA-4) integrins, mediate monocyte initial attachment to TNF-alpha-activated vascular endothelium under flow in vitro. J Immunol (1996) 157(1):326–35.
145. Carlos T, Kovach N, Schwartz B, Rosa M, Newman B, Wayner E, et al. Human monocytes bind to two cytokine-induced adhesive ligands on cultured human endothelial cells: endothelial-leukocyte adhesion molecule-1 and vascular cell adhesion molecule-1. Blood (1991) 77(10):2266–71.
146. Leeuwenberg JF, Jeunhomme TM, Buurman WA. Role of ELAM-1 in adhesion of monocytes to activated human endothelial cells. Scand J Immunol (1992) 35(3):335–41. doi:10.1111/j.1365-3083.1992.tb02866.x
147. Wojciak-Stothard B, Williams L, Ridley AJ. Monocyte adhesion and spreading on human endothelial cells is dependent on Rho-regulated receptor clustering. J Cell Biol (1999) 145(6):1293–307. doi:10.1083/jcb.145.6.1293
148. Chen L, Zhao Q, Wang XL, You R, Zhang YH, Ji H, et al. ZLJ-6, a novel COX/5-LOX inhibitor, attenuates TNF-alpha-induced endothelial E-selectin, ICAM-1 and VCAM-1 expression and monocyte-endothelial interactions via a COX/5-LOX-independent mechanism. Vascul Pharmacol (2011) 55(5–6):135–42. doi:10.1016/j.vph.2011.07.003
149. Burns MP, DePaola N. Flow-conditioned HUVECs support clustered leukocyte adhesion by coexpressing ICAM-1 and E-selectin. Am J Physiol Heart Circ Physiol (2005) 288(1):H194–204. doi:10.1152/ajpheart.01078.2003
150. Gerszten RE, Garcia-Zepeda EA, Lim YC, Yoshida M, Ding HA, Gimbrone MA Jr, et al. MCP-1 and IL-8 trigger firm adhesion of monocytes to vascular endothelium under flow conditions. Nature (1999) 398(6729):718–23. doi:10.1038/19546
151. Robert C, Fuhlbrigge RC, Kieffer JD, Ayehunie S, Hynes RO, Cheng G, et al. Interaction of dendritic cells with skin endothelium: a new perspective on immunosurveillance. J Exp Med (1999) 189(4):627–36. doi:10.1084/jem.189.4.627
152. Srinivas U, Larsson M, Lundblad A, Forsum U. E-selectin involvement in in vitro adhesion of blood dendritic cells to human umbilical cord endothelial cells. Scand J Immunol (1993) 38(3):273–8. doi:10.1111/j.1365-3083.1993.tb01725.x
153. Silva Z, Tong Z, Cabral MG, Martins C, Castro R, Reis C, et al. Sialyl Lewisx-dependent binding of human monocyte-derived dendritic cells to selectins. Biochem Biophys Res Commun (2011) 409(3):459–64. doi:10.1016/j.bbrc.2011.05.026
154. Bangert C, Friedl J, Stary G, Stingl G, Kopp T. Immunopathologic features of allergic contact dermatitis in humans: participation of plasmacytoid dendritic cells in the pathogenesis of the disease? J Invest Dermatol (2003) 121(6):1409–18. doi:10.1111/j.1523-1747.2003.12623.x
155. Kieffer JD, Fuhlbrigge RC, Armerding D, Robert C, Ferenczi K, Camphausen RT, et al. Neutrophils, monocytes, and dendritic cells express the same specialized form of PSGL-1 as do skin-homing memory T cells: cutaneous lymphocyte antigen. Biochem Biophys Res Commun (2001) 285(3):577–87. doi:10.1006/bbrc.2001.5230
156. McEver RP, Moore KL, Cummings RD. Leukocyte trafficking mediated by selectin-carbohydrate interactions. J Biol Chem (1995) 270(19):11025–8. doi:10.1074/jbc.270.19.11025
157. Laszik Z, Jansen PJ, Cummings RD, Tedder TF, McEver RP, Moore KL. P-selectin glycoprotein ligand-1 is broadly expressed in cells of myeloid, lymphoid, and dendritic lineage and in some nonhematopoietic cells. Blood (1996) 88(8):3010–21.
158. Moore KL, Eaton SF, Lyons DE, Lichenstein HS, Cummings RD, McEver RP. The P-selectin glycoprotein ligand from human neutrophils displays sialylated, fucosylated, O-linked poly-N-acetyllactosamine. J Biol Chem (1994) 269(37):23318–27.
159. Pendl GG, Robert C, Steinert M, Thanos R, Eytner R, Borges E, et al. Immature mouse dendritic cells enter inflamed tissue, a process that requires E- and P-selectin, but not P-selectin glycoprotein ligand 1. Blood (2002) 99(3):946–56. doi:10.1182/blood.V99.3.946
160. Moore KL. Structure and function of P-selectin glycoprotein ligand-1. Leuk Lymphoma (1998) 29(1–2):1–15. doi:10.3109/10428199809058377
161. Somers WS, Tang J, Shaw GD, Camphausen RT. Insights into the molecular basis of leukocyte tethering and rolling revealed by structures of P- and E-selectin bound to SLe(X) and PSGL-1. Cell (2000) 103(3):467–79. doi:10.1016/S0092-8674(00)00138-0
162. Lim YC, Snapp K, Kansas GS, Camphausen R, Ding H, Luscinskas FW. Important contributions of P-selectin glycoprotein ligand-1-mediated secondary capture to human monocyte adhesion to P-selectin, E-selectin, and TNF-alpha-activated endothelium under flow in vitro. J Immunol (1998) 161(5):2501–8.
163. Ponta H, Sherman L, Herrlich PA. CD44: from adhesion molecules to signalling regulators. Nat Rev Mol Cell Biol (2003) 4(1):33–45. doi:10.1038/nrm1004
164. Lesley J, Hyman R, Kincade PW. CD44 and its interaction with extracellular matrix. Adv Immunol (1993) 54:271–335. doi:10.1016/S0065-2776(08)60537-4
165. Goodison S, Urquidi V, Tarin D. CD44 cell adhesion molecules. Mol Pathol (1999) 52(4):189–96. doi:10.1136/mp.52.4.189
166. Sackstein R. The biology of CD44 and HCELL in hematopoiesis: the ‘step 2-bypass pathway’ and other emerging perspectives. Curr Opin Hematol (2011) 18(4):239–48. doi:10.1097/MOH.0b013e3283476140
167. Dimitroff CJ, Lee JY, Rafii S, Fuhlbrigge RC, Sackstein R. CD44 is a major E-selectin ligand on human hematopoietic progenitor cells. J Cell Biol (2001) 153(6):1277–86. doi:10.1083/jcb.153.6.1277
168. Sackstein R. The bone marrow is akin to skin: HCELL and the biology of hematopoietic stem cell homing. J Invest Dermatol (2004) 122(5):1061–9. doi:10.1111/j.0022-202X.2004.09301.x
169. Sackstein R, Dimitroff CJ. A hematopoietic cell L-selectin ligand that is distinct from PSGL-1 and displays N-glycan-dependent binding activity. Blood (2000) 96(8):2765–74.
170. Hanley WD, Burdick MM, Konstantopoulos K, Sackstein R. CD44 on LS174T colon carcinoma cells possesses E-selectin ligand activity. Cancer Res (2005) 65(13):5812–7. doi:10.1158/0008-5472.CAN-04-4557
171. Burdick MM, Chu JT, Godar S, Sackstein R. HCELL is the major E- and L-selectin ligand expressed on LS174T colon carcinoma cells. J Biol Chem (2006) 281(20):13899–905. doi:10.1074/jbc.M513617200
172. Stockton BM, Cheng G, Manjunath N, Ardman B, von Andrian UH. Negative regulation of T cell homing by CD43. Immunity (1998) 8(3):373–81. doi:10.1016/S1074-7613(00)80542-7
173. McEvoy LM, Jutila MA, Tsao PS, Cooke JP, Butcher EC. Anti-CD43 inhibits monocyte-endothelial adhesion in inflammation and atherogenesis. Blood (1997) 90(9):3587–94.
174. Fanales-Belasio E, Zambruno G, Cavani A, Girolomoni G. Antibodies against sialophorin (CD43) enhance the capacity of dendritic cells to cluster and activate T lymphocytes. J Immunol (1997) 159(5):2203–11.
175. Hamad M, Mosley RL, Wang J, Klein JR. Stimulation via the CD43 coreceptor augments T cell proliferation during the early phase of antigen-induced activation. Dev Comp Immunol (1996) 20(1):77–82. doi:10.1016/0145-305X(95)00037-T
176. Mody PD, Cannon JL, Bandukwala HS, Blaine KM, Schilling AB, Swier K, et al. Signaling through CD43 regulates CD4 T-cell trafficking. Blood (2007) 110(8):2974–82. doi:10.1182/blood-2007-01-065276
177. Barran P, Fellinger W, Warren CE, Dennis JW, Ziltener HJ. Modification of CD43 and other lymphocyte O-glycoproteins by core 2 N-acetylglucosaminyltransferase. Glycobiology (1997) 7(1):129–36. doi:10.1093/glycob/7.1.129
178. Merzaban JS, Burdick MM, Gadhoum SZ, Dagia NM, Chu JT, Fuhlbrigge RC, et al. Analysis of glycoprotein E-selectin ligands on human and mouse marrow cells enriched for hematopoietic stem/progenitor cells. Blood (2011) 118(7):1774–83. doi:10.1182/blood-2010-11-320705
179. Tiemeyer M, Swiedler SJ, Ishihara M, Moreland M, Schweingruber H, Hirtzer P, et al. Carbohydrate ligands for endothelial-leukocyte adhesion molecule 1. Proc Natl Acad Sci U S A (1991) 88(4):1138–42. doi:10.1073/pnas.88.4.1138
180. Mondal N, Stolfa G, Antonopoulos A, Zhu Y, Wang SS, Buffone A Jr, et al. Glycosphingolipids on human myeloid cells stabilize E-selectin-dependent rolling in the multistep leukocyte adhesion cascade. Arterioscler Thromb Vasc Biol (2016) 36(4):718–27. doi:10.1161/ATVBAHA.115.306748
181. Angiari S. Selectin-mediated leukocyte trafficking during the development of autoimmune disease. Autoimmun Rev (2015) 14(11):984–95. doi:10.1016/j.autrev.2015.06.006
182. Barthel SR, Gavino JD, Descheny L, Dimitroff CJ. Targeting selectins and selectin ligands in inflammation and cancer. Expert Opin Ther Targets (2007) 11(11):1473–91. doi:10.1517/14728222.11.11.1473
183. Das PK, de Boer OJ, Visser A, Verhagen CE, Bos JD, Pals ST. Differential expression of ICAM-1, E-selectin and VCAM-1 by endothelial cells in psoriasis and contact dermatitis. Acta Derm Venereol Suppl (Stockh) (1994) 186:21–2.
184. Czech W, Schöpf E, Kapp A. Soluble E-selectin in sera of patients with atopic dermatitis and psoriasis – correlation with disease activity. Br J Dermatol (1996) 134(1):17–21. doi:10.1111/j.1365-2133.1996.tb07833.x
185. Hirai S, Kageshita T, Kimura T, Tsujisaki M, Okajima K, Imai K, et al. Soluble intercellular adhesion molecule-1 and soluble E-selectin levels in patients with atopic dermatitis. Br J Dermatol (1996) 134(4):657–61. doi:10.1111/j.1365-2133.1996.tb06965.x
186. Kowalzick L, Kleinheinz A, Neuber K, Weichenthal M, Köhler I, Ring J. Elevated serum levels of soluble adhesion molecules ICAM-1 and ELAM-1 in patients with severe atopic eczema and influence of UVA1 treatment. Dermatology (1995) 190(1):14–8. doi:10.1159/000246627
187. Morita H, Kitano Y, Kawasaki N. Elevation of serum-soluble E-selectin in atopic dermatitis. J Dermatol Sci (1995) 10(2):145–50. doi:10.1016/0923-1811(95)96802-T
188. Wakita H, Sakamoto T, Tokura Y, Takigawa M. E-selectin and vascular cell adhesion molecule-1 as critical adhesion molecules for infiltration of T lymphocytes and eosinophils in atopic dermatitis. J Cutan Pathol (1994) 21(1):33–9. doi:10.1111/j.1600-0560.1994.tb00688.x
189. Krasowska D, Pietrzak A, Lecewicz-Torun B. Serum level of sELAM-1 in psoriatic patients correlates with disease activity. J Eur Acad Dermatol Venereol (1999) 12(2):140–2. doi:10.1111/j.1468-3083.1999.tb01004.x
190. Kowalzick L, Neuber K, Weichenthal M, Köhler I, Ring J. Elevated serum-soluble ELAM-1 levels in patients with severe plaque-type psoriasis. Arch Dermatol Res (1994) 286(7):414–6. doi:10.1007/BF00371802
191. Wakita H, Takigawa M. E-selectin and vascular cell adhesion molecule-1 are critical for initial trafficking of helper-inducer/memory T cells in psoriatic plaques. Arch Dermatol (1994) 130(4):457–63. doi:10.1001/archderm.1994.01690040061008
192. Singh TP, Zhang HH, Borek I, Wolf P, Hedrick MN, Singh SP, et al. Monocyte-derived inflammatory Langerhans cells and dermal dendritic cells mediate psoriasis-like inflammation. Nat Commun (2016) 7:13581. doi:10.1038/ncomms13581
193. Steinhoff M, Steinhoff A, Homey B, Luger TA, Schneider SW. Role of vasculature in atopic dermatitis. J Allergy Clin Immunol (2006) 118(1):190–7. doi:10.1016/j.jaci.2006.04.025
194. Wollenberg A, Wagner M, Gunther S, Towarowski A, Tuma E, Moderer M, et al. Plasmacytoid dendritic cells: a new cutaneous dendritic cell subset with distinct role in inflammatory skin diseases. J Invest Dermatol (2002) 119(5):1096–102. doi:10.1046/j.1523-1747.2002.19515.x
195. Davies MJ, Gordon JL, Gearing AJ, Pigott R, Woolf N, Katz D, et al. The expression of the adhesion molecules ICAM-1, VCAM-1, PECAM, and E-selectin in human atherosclerosis. J Pathol (1993) 171(3):223–9. doi:10.1002/path.1711710311
196. O’Brien KD, McDonald TO, Chait A, Allen MD, Alpers CE. Neovascular expression of E-selectin, intercellular adhesion molecule-1, and vascular cell adhesion molecule-1 in human atherosclerosis and their relation to intimal leukocyte content. Circulation (1996) 93(4):672–82. doi:10.1161/01.CIR.93.4.672
197. Collins RG, Velji R, Guevara NV, Hicks MJ, Chan L, Beaudet AL. P-Selectin or intercellular adhesion molecule (ICAM)-1 deficiency substantially protects against atherosclerosis in apolipoprotein E-deficient mice. J Exp Med (2000) 191(1):189–94. doi:10.1084/jem.191.1.189
198. Dong ZM, Chapman SM, Brown AA, Frenette PS, Hynes RO, Wagner DD. The combined role of P- and E-selectins in atherosclerosis. J Clin Invest (1998) 102(1):145–52. doi:10.1172/JCI3001
199. Pooley N, Ghosh L, Sharon P. Up-regulation of E-selectin and intercellular adhesion molecule-1 differs between Crohn’s disease and ulcerative colitis. Dig Dis Sci (1995) 40(1):219–25. doi:10.1007/BF02063969
200. Lazaris AC, Dicoglou C, Tseleni-Balafouta S, Paraskevakou H, Davaris PS. In situ expression of E-selectin and intercellular adhesion molecule-1 in chronic inflammatory diseases of the gastrointestinal tract. APMIS (1999) 107(9):819–27. doi:10.1111/j.1699-0463.1999.tb01477.x
201. Vainer B, Nielsen OH, Horn T. Expression of E-selectin, sialyl Lewis X, and macrophage inflammatory protein-1alpha by colonic epithelial cells in ulcerative colitis. Dig Dis Sci (1998) 43(3):596–608. doi:10.1023/A:1018875410987
202. Rugtveit J, Brandtzaeg P, Halstensen TS, Fausa O, Scott H. Increased macrophage subset in inflammatory bowel disease: apparent recruitment from peripheral blood monocytes. Gut (1994) 35(5):669–74. doi:10.1136/gut.35.5.669
203. Zhao J, Gao Y, Cheng C, Yan M, Wang J. Upregulation of β-1,4-galactosyltransferase I in rat spinal cord with experimental autoimmune encephalomyelitis. J Mol Neurosci (2013) 49(3):437–45. doi:10.1007/s12031-012-9824-3
204. King IL, Dickendesher TL, Segal BM. Circulating Ly-6C+ myeloid precursors migrate to the CNS and play a pathogenic role during autoimmune demyelinating disease. Blood (2009) 113(14):3190–7. doi:10.1182/blood-2008-07-168575
205. Chapman PT, Jamar F, Keelan ET, Peters AM, Haskard DO. Use of a radiolabeled monoclonal antibody against E-selectin for imaging of endothelial activation in rheumatoid arthritis. Arthritis Rheum (1996) 39(8):1371–5. doi:10.1002/art.1780390815
206. Ishikawa H, Nishibayashi Y, Kita K, Ohno O, Imura S, Hirata S. Adhesion molecules in the lymphoid cell distribution in rheumatoid synovial membrane. Bull Hosp Jt Dis (1993) 53(2):23–8.
207. Kinne RW, Brauer R, Stuhlmuller B, Palombo-Kinne E, Burmester GR. Macrophages in rheumatoid arthritis. Arthritis Res (2000) 2(3):189–202. doi:10.1186/ar86
208. Abdi R, Moore R, Sakai S, Donnelly CB, Mounayar M, Sackstein R. HCELL expression on murine MSC licenses pancreatotropism and confers durable reversal of autoimmune diabetes in NOD mice. Stem Cells (2015) 33(5):1523–31. doi:10.1002/stem.1948
209. Martin AP, Rankin S, Pitchford S, Charo IF, Furtado GC, Lira SA. Increased expression of CCL2 in insulin-producing cells of transgenic mice promotes mobilization of myeloid cells from the bone marrow, marked insulitis, and diabetes. Diabetes (2008) 57(11):3025–33. doi:10.2337/db08-0625
210. Brockmeyer C, Ulbrecht M, Schendel DJ, Weiss EH, Hillebrand G, Burkhardt K, et al. Distribution of cell adhesion molecules (ICAM-1, VCAM-1, ELAM-1) in renal tissue during allograft rejection. Transplantation (1993) 55(3):610–5. doi:10.1097/00007890-199303000-00027
211. Magil AB. Monocytes/macrophages in renal allograft rejection. Transplant Rev (2009) 23(4):199–208. doi:10.1016/j.trre.2009.06.005
212. Shreeniwas R, Schulman LL, Narasimhan M, McGregor CC, Marboe CC. Adhesion molecules (E-selectin and ICAM-1) in pulmonary allograft rejection. Chest (1996) 110(5):1143–9. doi:10.1378/chest.110.5.1143
213. Rothman A, Mann D, Behling CA, McGraw M, Seslar S, Shiu P, et al. Increased expression of endoarterial vascular cell adhesion molecule-1 mRNA in an experimental model of lung transplant rejection: diagnosis by pulmonary arterial biopsy. Transplantation (2003) 75(7):960–5. doi:10.1097/01.TP.0000057530.32397.6D
214. Gelman AE, Okazaki M, Sugimoto S, Li W, Kornfeld CG, Lai J, et al. CCR2 regulates monocyte recruitment as well as CD4 T1 allorecognition after lung transplantation. Am J Transplant (2010) 10(5):1189–99. doi:10.1111/j.1600-6143.2010.03101.x
215. Ferran C, Peuchmaur M, Desruennes M, Ghoussoub JJ, Cabrol A, Brousse N, et al. Implications of de novo ELAM-1 and VCAM-1 expression in human cardiac allograft rejection. Transplantation (1993) 55(3):605–9. doi:10.1097/00007890-199303000-00026
216. Morel O, Ohlmann P, Epailly E, Bakouboula B, Zobairi F, Jesel L, et al. Endothelial cell activation contributes to the release of procoagulant microparticles during acute cardiac allograft rejection. J Heart Lung Transplant (2008) 27(1):38–45. doi:10.1016/j.healun.2007.09.031
217. Salama M, Andrukhova O, Roedler S, Zuckermann A, Laufer G, Aharinejad S. Association of CD14+ monocyte-derived progenitor cells with cardiac allograft vasculopathy. J Thorac Cardiovasc Surg (2011) 142(5):1246–53. doi:10.1016/j.jtcvs.2011.07.032
218. Norton J, Sloane JP, al-Saffar N, Haskard DO. Vessel associated adhesion molecules in normal skin and acute graft-versus-host disease. J Clin Pathol (1991) 44(7):586–91. doi:10.1136/jcp.44.7.586
219. Norton J, Sloane JP, al-Saffar N, Haskard DO. Expression of adhesion molecules in human intestinal graft-versus-host disease. Clin Exp Immunol (1992) 87(2):231–6. doi:10.1111/j.1365-2249.1992.tb02980.x
220. Reddy P. Pathophysiology of acute graft-versus-host disease. Hematol Oncol (2003) 21(4):149–61. doi:10.1002/hon.716
221. Nishiwaki S, Terakura S, Ito M, Goto T, Seto A, Watanabe K, et al. Impact of macrophage infiltration of skin lesions on survival after allogeneic stem cell transplantation: a clue to refractory graft-versus-host disease. Blood (2009) 114(14):3113–6. doi:10.1182/blood-2009-03-209635
222. Hauselmann I, Roblek M, Protsyuk D, Huck V, Knopfova L, Grassle S, et al. Monocyte induction of E-selectin-mediated endothelial activation releases VE-cadherin junctions to promote tumor cell extravasation in the metastasis cascade. Cancer Res (2016) 76(18):5302–12. doi:10.1158/0008-5472.CAN-16-0784
223. Hoos A, Protsyuk D, Borsig L. Metastatic growth progression caused by PSGL-1-mediated recruitment of monocytes to metastatic sites. Cancer Res (2014) 74(3):695–704. doi:10.1158/0008-5472.CAN-13-0946
224. Schettini J, Mukherjee P. Physiological role of plasmacytoid dendritic cells and their potential use in cancer immunity. Clin Dev Immunol (2008) 2008:106321. doi:10.1155/2008/106321
225. Harimoto H, Shimizu M, Nakagawa Y, Nakatsuka K, Wakabayashi A, Sakamoto C, et al. Inactivation of tumor-specific CD8(+) CTLs by tumor-infiltrating tolerogenic dendritic cells. Immunol Cell Biol (2013) 91(9):545–55. doi:10.1038/icb.2013.38
226. Conrad C, Gregorio J, Wang YH, Ito T, Meller S, Hanabuchi S, et al. Plasmacytoid dendritic cells promote immunosuppression in ovarian cancer via ICOS costimulation of Foxp3(+) T-regulatory cells. Cancer Res (2012) 72(20):5240–9. doi:10.1158/0008-5472.CAN-12-2271
227. Ludwig RJ, Schön MP, Boehncke WH. P-selectin: a common therapeutic target for cardiovascular disorders, inflammation and tumour metastasis. Expert Opin Ther Targets (2007) 11(8):1103–17. doi:10.1517/14728222.11.8.1103
228. Fuhlbrigge RC, Weishaupt C. Adhesion molecules in cutaneous immunity. Semin Immunopathol (2007) 29(1):45–57. doi:10.1007/s00281-007-0065-4
229. Beeh KM, Beier J, Meyer M, Buhl R, Zahlten R, Wolff G. Bimosiamose, an inhaled small-molecule pan-selectin antagonist, attenuates late asthmatic reactions following allergen challenge in mild asthmatics: a randomized, double-blind, placebo-controlled clinical cross-over-trial. Pulm Pharmacol Ther (2006) 19(4):233–41. doi:10.1016/j.pupt.2005.07.004
230. Friedrich M, Bock D, Philipp S, Ludwig N, Sabat R, Wolk K, et al. Pan-selectin antagonism improves psoriasis manifestation in mice and man. Arch Dermatol Res (2006) 297(8):345–51. doi:10.1007/s00403-005-0626-0
231. Stahn R, Schäfer H, Kernchen F, Schreiber J. Multivalent sialyl Lewis x ligands of definite structures as inhibitors of E-selectin mediated cell adhesion. Glycobiology (1998) 8(4):311–9. doi:10.1093/glycob/8.4.311
232. Dimitroff CJ, Kupper TS, Sackstein R. Prevention of leukocyte migration to inflamed skin with a novel fluorosugar modifier of cutaneous lymphocyte-associated antigen. J Clin Invest (2003) 112(7):1008–18. doi:10.1172/JCI19220
233. Nelson RM, Cecconi O, Roberts WG, Aruffo A, Linhardt RJ, Bevilacqua MP. Heparin oligosaccharides bind L- and P-selectin and inhibit acute inflammation. Blood (1993) 82(11):3253–8.
234. Ludwig RJ, Alban S, Bistrian R, Boehncke WH, Kaufmann R, Henschler R, et al. The ability of different forms of heparins to suppress P-selectin function in vitro correlates to their inhibitory capacity on bloodborne metastasis in vivo. Thromb Haemost (2006) 95(3):535–40. doi:10.1267/THRO06030535
235. Schön MP. Inhibitors of selectin functions in the treatment of inflammatory skin disorders. Ther Clin Risk Manag (2005) 1(3):201–8.
236. Rillahan CD, Brown SJ, Register AC, Rosen H, Paulson JC. High-throughput screening for inhibitors of sialyl- and fucosyltransferases. Angew Chem Int Ed Engl (2011) 50(52):12534–7. doi:10.1002/anie.201105065
237. Scalia R, Hayward R, Armstead VE, Minchenko AG, Lefer AM. Effect of recombinant soluble P-selectin glycoprotein ligand-1 on leukocyte-endothelium interaction in vivo. Role in rat traumatic shock. Circ Res (1999) 84(1):93–102. doi:10.1161/01.RES.84.1.93
238. Hayward R, Campbell B, Shin YK, Scalia R, Lefer AM. Recombinant soluble P-selectin glycoprotein ligand-1 protects against myocardial ischemic reperfusion injury in cats. Cardiovasc Res (1999) 41(1):65–76. doi:10.1016/S0008-6363(98)00266-1
239. Kyriakides C, Austen W, Wang Y, Favuzza J, Moore FD, Hechtman HB. Endothelial selectin blockade attenuates lung permeability of experimental acid aspiration. Surgery (2000) 128(2):327–31. doi:10.1067/msy.2000.108216
240. Hayward R, Lefer AM. Acute mesenteric ischemia and reperfusion: protective effects of recombinant soluble P-selectin glycoprotein ligand-1. Shock (1999) 12(3):201–7. doi:10.1097/00024382-199909000-00006
241. Etzioni A, Frydman M, Pollack S, Avidor I, Phillips ML, Paulson JC, et al. Brief report: recurrent severe infections caused by a novel leukocyte adhesion deficiency. N Engl J Med (1992) 327(25):1789–92. doi:10.1056/NEJM199212173272505
242. Karsan A, Cornejo CJ, Winn RK, Schwartz BR, Way W, Lannir N, et al. Leukocyte adhesion deficiency type II is a generalized defect of de novo GDP-fucose biosynthesis. Endothelial cell fucosylation is not required for neutrophil rolling on human nonlymphoid endothelium. J Clin Invest (1998) 101(11):2438–45. doi:10.1172/JCI905
243. Etzioni A, Harlan JM, Pollack S, Phillips LM, Gershoni-Baruch R, Paulson JC. Leukocyte adhesion deficiency (LAD) II: a new adhesion defect due to absence of sialyl Lewis X, the ligand for selectins. Immunodeficiency (1993) 4(1–4):307–8.
244. Sackstein R, Merzaban JS, Cain DW, Dagia NM, Spencer JA, Lin CP, et al. Ex vivo glycan engineering of CD44 programs human multipotent mesenchymal stromal cell trafficking to bone. Nat Med (2008) 14(2):181–7. doi:10.1038/nm1703
245. Sackstein R. Engineering cellular trafficking via glycosyltransferase-programmed stereosubstitution. Ann N Y Acad Sci (2012) 1253:193–200. doi:10.1111/j.1749-6632.2011.06421.x
246. Dykstra B, Lee J, Mortensen LJ, Yu H, Wu ZL, Lin CP, et al. Glycoengineering of E-selectin ligands by intracellular versus extracellular fucosylation differentially affects osteotropism of human mesenchymal stem cells. Stem Cells (2016) 34(10):2501–11. doi:10.1002/stem.2435
247. Pachon-Pena G, Donnelly C, Ruiz-Canada C, Katz A, Fernandez-Veledo S, Vendrell J, et al. A glycovariant of human CD44 is characteristically expressed on human mesenchymal stem cells. Stem Cells (2017) 35(4):1080–92. doi:10.1002/stem.2549
Keywords: mononuclear phagocyte, HCELL, E-selectin ligand, cell migration, E-selectin, sialyl Lewis X
Citation: Silva M, Videira PA and Sackstein R (2018) E-Selectin Ligands in the Human Mononuclear Phagocyte System: Implications for Infection, Inflammation, and Immunotherapy. Front. Immunol. 8:1878. doi: 10.3389/fimmu.2017.01878
Received: 30 September 2017; Accepted: 08 December 2017;
Published: 19 January 2018
Edited by:
Yoann Rombouts, UMR5089 Institut de Pharmacologie et de Biologie Structurale (IPBS), FranceReviewed by:
Michael Hickey, Monash University, AustraliaLuciana Barros Arruda, Universidade Federal do Rio de Janeiro, Brazil
Copyright: © 2018 Silva, Videira and Sackstein. This is an open-access article distributed under the terms of the Creative Commons Attribution License (CC BY). The use, distribution or reproduction in other forums is permitted, provided the original author(s) or licensor are credited and that the original publication in this journal is cited, in accordance with accepted academic practice. No use, distribution or reproduction is permitted which does not comply with these terms.
*Correspondence: Paula A. Videira, cC52aWRlaXJhJiN4MDAwNDA7ZmN0LnVubC5wdA==;
Robert Sackstein, cnNhY2tzdGVpbiYjeDAwMDQwO3JpY3MuYndoLmhhcnZhcmQuZWR1
†Co-first authorship.