- Translational Research Center for Gastrointestinal Disorders (TARGID), Department of Chronic Diseases, Metabolism and Ageing, KU Leuven, Leuven, Belgium
In recent years, the bidirectional relationship between the nervous and immune system has become increasingly clear, and its role in both homeostasis and inflammation has been well documented over the years. Since the introduction of the cholinergic anti-inflammatory pathway, there has been an increased interest in parasympathetic regulation of both innate and adaptive immune responses, including T helper 2 responses. Increasing evidence has been emerging suggesting a role for the parasympathetic nervous system in the pathophysiology of allergic diseases, including allergic rhinitis, asthma, food allergy, and atopic dermatitis. In this review, we will highlight the role of cholinergic modulation by both nicotinic and muscarinic receptors in several key aspects of the allergic inflammatory response, including barrier function, innate and adaptive immune responses, and effector cells responses. A better understanding of these cholinergic processes mediating key aspects of type 2 immune disorders might lead to novel therapeutic approaches to treat allergic diseases.
Introduction
Evidence is accumulating that extrinsic nerves intensely and bidirectionally communicate with the immune system. Indeed, in 2000, Tracey and coworkers introduced the novel concept of the “inflammatory reflex,” where afferent vagal fibers sense peripheral inflammation and inform the brain on the inflammatory status to subsequently trigger vagal efferent fibers to dampen the inflammatory response (1). The cross talk between the vagus nerve and the immune system has been largely attributed to dampening of the innate immune system, in particular by reduction of cytokine release by splenic macrophages (2, 3). Since then, vagus nerve stimulation (VNS) has been evaluated in several inflammatory models characterized by not only activation of the innate immune system but also the adaptive immune system, particularly Th1 or Th17 immune responses (4, 5). Clinical observations, however, indirectly suggest that cholinergic immune modulation may also be of interest in atopic disorders characterized by T helper 2 (Th2) responses. Nicotine, a major constituent of cigarette smoke and a ligand for nicotinic acetylcholine receptors (nAChRs), has been implicated in anti-inflammatory effects seen in correlation with smoking. However, a rather complex relationship exists between smoking and the incidence of allergic diseases; on the one hand, cigarette smoke has been reported to cause impaired lung function, consequently aggravating asthma symptoms, and additionally also increase the risk to develop atopy, asthma, and food allergy (6–10). Oppositely, other reports indicated an inverse correlation between smoking and allergic disease incidence. Epidemiological studies demonstrated that the occurrence of asthma could be higher in former smokers, compared with active smokers (11–13). In addition, other studies have observed a negative association between smoking and the development of allergic sensitization (14, 15). In accordance with this, in a recent study Tilp et al. demonstrated that cigarette smoke reduces the allergen-induced Th2 response in the lung and the development of airway hyperreactivity (AHR) in a severe model of asthma in mice (16). Similarly, the adverse effects of excessive psychological stress on the incidence and severity of allergic disorders have been acknowledged for long time (17). Interestingly, anxiety and depression have been associated with a decrease in vagal tone, leading to the exploration of VNS as a treatment for depression (18–21). The possibility exists that the decrease in vagal tone leads to a reduction in cholinergic modulation promoting allergic inflammation. This theory is supported by a study demonstrating that impaired parasympathetic function increased susceptibility to inflammatory bowel disease in a mouse model of depression (22). Furthermore, acetylcholinesterase (AChE) inhibitors, which have been successfully used in animal models to activate the cholinergic anti-inflammatory pathway, are widely used in the clinic to treat Alzheimer’s disease (AD) (23–26). Curiously, AD patients treated with the AChE inhibitor, donepezil, showed increased serum levels of interleukin (IL)-4 and MCP-1, suggesting a possible shift toward a Th2 immune response (27). In addition, the effect of organophosphate compounds (OPCs), which are used as pesticides and are known AChE inhibitors, has been tested in allergic animal models. However, contrasting results have been obtained, whereas some studies mention an aggravation of allergic immune responses, other studies report an overall immunosuppressive effect (28–31). This discrepancy might be attributed to the timing of exposure, as a recent study showed that exposure to OPCs before allergen sensitization leads to worsening of allergic inflammation, while coinstantaneous exposure leads to suppression (31). Over the years, the importance of cholinergic modulation in inflammatory conditions has become increasingly clear. Although the role of parasympathetic regulation in allergic disorders has not been investigated extensively, more evidence is emerging supporting a role for cholinergic modulation in Th2-mediated disorders. Therefore, in this review, we aim to provide an overview of recent discoveries and its implications in relation to this research area.
Pathophysiology of Allergic Inflammation
At mucosal surfaces, the epithelium is crucial for maintaining a physical barrier and protect against environmental insults and allergens. In addition, it forms a functional barrier allowing communication between the internal and external environment. A whole array of different immune cells exists within the mucosal barrier, constantly surveying the environment and ready to provide innate and adaptive immunity. Many allergic diseases typically coincide with disorders of the epithelial barrier, which is associated with loss of epithelial differentiation, reduced junctional integrity, and impaired innate defense. For a long time, barrier dysfunction was seen as a consequence of the aberrant immunological response toward allergens. Recent evidence, however, suggests that barrier dysfunction also may act as an important initiator and player in the pathophysiology of allergic inflammation. The barrier can be disrupted by a number of factors arising from the external environment, such as proteases, toxins, or injury, but also endogenous factors such as hormones, diet, and circadian clock could contribute. Disruption of the barrier might result in increased antigen uptake and can drive predisposition to allergic sensitization (32, 33). On exposure, allergens are sensed by epithelial cells, which in turn lead to the rapid release of epithelial cytokines, IL-25, IL-33, and thymic stromal lymphopoietin (TSLP) (34). These epithelial cytokines are able to induce expression and release of type 2 cytokines by type 2 innate lymphoid cells (ILC2s). This creates an environment that promotes the generation of allergen-specific Th2 cells, a process that is mainly driven by antigen presenting cells, primarily dendritic cells (DCs). DCs are responsible for taking up, processing and presenting allergens to naïve T cells. Th2 cells then play a central role, orchestrating different events of the inflammatory allergic response, mainly through the release of type 2 cytokines such as IL-4, IL-5, IL-9, and IL-13. These events include the attraction of effector cells such as eosinophils, mast cells, and basophils to the site of inflammation. In addition, B-cell class switching will be promoted under the influence of IL-4 and IL-13, leading to an increased production of allergen-specific immunoglobulin E (IgE). Upon allergen reexposure, IgE is able to bind FcεRIα on the surface of mast cells and basophils leading to their activation. This cascade of events can occur at different sites throughout the body and represent the underlying mechanism of disorders such as allergic rhinitis, asthma, food allergy, and atopic dermatitis (Figure 1) (35, 36).
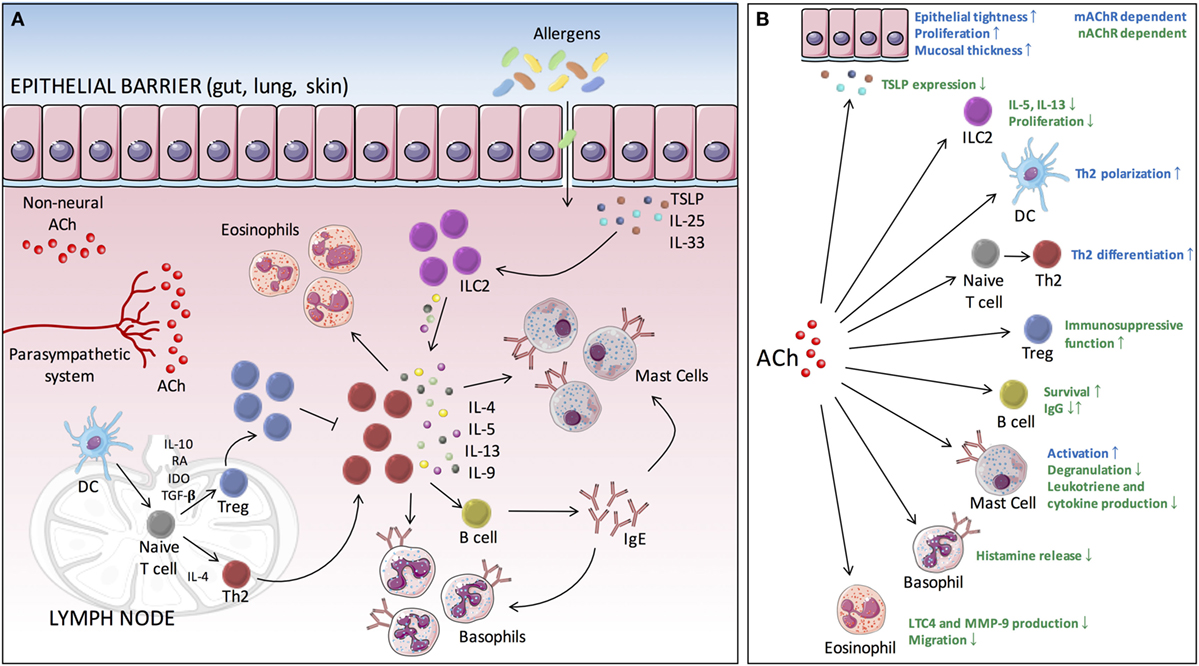
Figure 1. Cholinergic modulation of type 2-mediated inflammation. (A) Allergen exposure evokes the rapid release of epithelial cytokines (IL-25, IL-33, and TSLP), which in turn drives the production of type 2 cytokines by ILC2s. This creates an environment that further facilitates DCs to promote the differentiation of naive T cells toward Th2 cells. These Th2 cells will then orchestrate different events of the inflammatory allergic response, through the release of type 2 cytokines (IL-4, IL-5, IL-9, and IL-13), including the attraction of effector cells (eosinophils, mast cells, and basophils) to the site of inflammation. In addition, B-cell class switching is promoted, leading to the production of allergen-specific IgE. Binding of antigen to IgE bound on mast cells and basophils leads to IgE cross-linking and subsequent activation. (B) ACh is able to modulate several key processes of the allergic inflammatory response via muscarinic and nicotinic receptors. ACh, acetylcholine; TSLP, thymic stromal lymphopoietin; DC, dendritic cell; ILC2, type 2 innate lymphoid cell; Th, T helper cell; IgE, immunoglobulin E; mAChR, muscarinic acetylcholine receptor; nAChR, nicotinic acetylcholine receptor; IL, interleukin; Th2, T helper 2.
The Cholinergic Nervous System in Gut, Lung and Skin
Barrier surfaces such as the gastrointestinal (GI) tract, respiratory tract, and skin are densely populated by neurons and immune cells that constantly sense and respond to environmental challenges, including allergens. The peripheral nervous system (PNS) consists of the somatic nervous system and the autonomic nervous system. The latter can be further subdivided into the parasympathetic, sympathetic, and enteric nervous system (ENS). The different neurons of the PNS have been found to communicate with the immune system through the release of neuromediators from their nerve terminals. The parasympathetic nervous system primarily uses the neurotransmitter acetylcholine (ACh). As in this review, the focus will be on cholinergic modulation of the immune response, we will first describe the parasympathetic innervation and cholinergic input at the different epithelial barriers typically involved in allergic conditions.
The gut is densely innervated by the autonomic nervous system, consisting of the extrinsic innervation and the ENS, located within the intestine. The vagus nerve, providing a bidirectional connection between the brain and the gut, represents the main extrinsic parasympathetic nerve in the GI tract, where it mainly controls secretion, vascularization, and gastrointestinal motility. Preganglionic efferent vagal nerve fibers extensively innervate the GI tract, displaying the highest density in the stomach and further decreasing in the small bowel and colon, and establishing connections with postganglionic neurons primarily located in the myenteric plexus (37, 38). However, as vagal efferents only synapse with cholinergic enteric neurons in the myenteric plexus, it is likely that they affect mucosal immune responses indirectly through activation of cholinergic ENS neurons releasing ACh (39).
In the lung, the parasympathetic nervous system plays a prominent role in the control of airway smooth muscle tone. ACh released from postganglionic neurons induces bronchoconstriction, mucus secretion, and bronchial vasodilation, primarily mediated via binding on muscarinic receptor M3 (40, 41). For this reason, anticholinergic and muscarinic antagonists have been used to treat bronchoconstriction in asthma. The prominent role of the parasympathetic nervous system in the pathophysiology of asthma makes it challenging to investigate its role in the modulation of the immune response.
In contrast to the GI and the respiratory tract, the skin is devoid of parasympathetic innervation (41). This might question a role for cholinergic modulation of immune responses in the skin and in diseases, such as atopic dermatitis. However, the skin contains other sources of ACh, in particular keratinocytes (42), but in fact almost every cell, including epithelial, endothelial, and immune cells can produce ACh. Hence, this so-called “non-neural cholinergic system” might not only be of relevance in the skin but also in the gut and lung (43).
Cholinergic Modulation of Barrier Function
Improving epithelial barrier function could result into a decreased access of allergens, limiting the subsequent type 2 inflammatory response. Although there is no direct evidence for cholinergic modulation of epithelial barrier function in allergic disorders, some studies do suggest a role for ACh in modulating barrier integrity. ACh was shown to play a role in the regulation of epithelial tightness in pig colon cultures. Incubation with carbachol resulted into an increased transepithelial electrical resistance, an effect that was inhibited by atropine, suggesting involvement of muscarinic acetylcholine receptors (mAChRs) (44). In addition, muscarinic agonists where shown to stimulate epithelial cell proliferation, increasing mucosal thickness in the intestine. Moreover, in several inflammatory conditions, cholinergic modulation was seen to protect barrier integrity due to improved tight junction protein expression (45–48). However, this effect is probably indirectly regulated by the downregulation of pro-inflammatory cytokines. Although the cholinergic modulation of barrier function in type 2-mediated diseases has been relatively unexplored so far, it might hold yet undiscovered potential toward therapeutic interventions.
The epithelium should not be considered as merely a physical barrier controlling the uptake and transport of antigens; in addition, it should be seen as an active contributor to the mucosal environment helping to shape local immune reactions. Epithelial derived cytokines IL-25, IL-33, and TSLP have been shown to play a role in the initiation of type 2 allergic responses. Preventing the expression and release of epithelial cytokines might be sufficient to prevent the subsequent Th2 immune response. Evidence that cholinergic modulation might influence this process was recently provided by a study showing inhibition of TSLP expression both in vitro and in vivo by nicotine. This effect was abolished by pretreatment with α7 nAChR antagonists, showing the involvement of α7 nAChR (49).
Experimental and clinical data have supported a role for type 2 cytokines in the regulation of intestinal barrier function. IL-4 and IL-13 have been shown to increase intestinal permeability, an effect that is mediated via phosphoinositide 3-kinases (50–53). In addition, allergic effector cells such as mast cells and eosinophils release powerful inflammatory mediators, such as proteases and cytokines, further contributing to barrier dysfunction (52, 54–56). Cholinergic modulation of immune cells involved in the allergic inflammatory response might therefore, in addition to direct effects that will be described in detail below, also contribute to effects on barrier function.
Cholinergic Modulation of the Type 2 Innate Immune Response
The innate immune system plays an important role in the initiation of subsequent adaptive immune response. Key players of type 2 innate immunity include recently described ILC2s that produce cytokines promoting Th2 differentiation by DCs, which are considered as a crucial link between innate and adaptive immunity.
Interestingly, a recent study showed that ILC2 might be modulated by cholinergic signals. Indeed, α7 nAChR expression has been shown on ILC2, whereas in vitro incubation of pulmonary ILC2s with GTS-21, a specific α7 nAChR agonist suppressed production of IL-5 and IL-13 cytokines, proliferation, and GATA3 expression. In addition, GTS-21 treatment ameliorated ILC2 induced AHR. Similar observations were made in a humanized ILC2 AHR mice model (57). These findings suggest that cholinergic modulation of ILC2s via the α7 nAChR is a potential target for therapeutic intervention in type 2-mediated disorders.
In contrast to the well described anti-inflammatory effect of ACh on macrophages (39, 58–60), the effect of cholinergic modulation on DCs has been far less studied. Aicher et al. demonstrated the expression of α7 nAChR on human monocyte-derived DCs, which was upregulated in response to its ligand nicotine (61). In addition, murine bone marrow-derived DCs expressed mRNAs encoding the five mAChR subtypes (M1–M5) and the α2, α5, α6, α7, α10, and β2 nAChR subunits (62). Earlier reports suggested an anti-inflammatory effect of nicotine reducing endocytosis, phagocytosis, the production of pro-inflammatory cytokines IL-12, IL-1β, and TNF-α and the ability to induce a Th1 response (63). By contrast, other reports stated that nicotine activated DC function, enhancing endocytosis, stimulation of T cell proliferation, and the production of IL-12 (61, 64). These opposing results might be due to differences in maturation and activation state. More recently, Gori et al. demonstrated a role for ACh in polarizing DCs toward a Th2-promoting phenotype. Treatment of DC with ACh stimulated the expression of the Th2-promoter OX40L, the production of Th2-chemokines CCL22 and CCL17, and the synthesis of IL-4, IL-5, and IL-13 by T cells (65). This study was in line with earlier findings by Liu et al. showing that the muscarinic receptor agonist, methacholine, increased OX40L in DCs (66). These data suggest an involvement of mAChRs in the Th2 polarizing effect of ACh on DCs.
Cholinergic Modulation of the Type 2 Adaptive Immune Response
Given that DCs form a bridge between the innate and adaptive immune responses, cholinergic modulation of these cells as described earlier will definitely influence consequent adaptive immune responses. However, both T and B lymphocytes are also directly modulated by ACh, as several nAChR and mAChRs subtypes have been reported in both cell types (67–70).
As for other immune cells, also T cells show a high degree of plasticity with respect to their cholinergic receptor network. Depending on their activation state, differentiation state or even environmental cues, T cells will express a different repertoire of ACh receptors (71). So far, especially α7 nAChR has been investigated in the context of the cholinergic anti-inflammatory pathway (1). Although the expression of the α7 nAChR subunit has been demonstrated in activated CD4 T cells, surprisingly Th1, Th2, or Th17 cells do not express detectable levels of this receptor (71). In addition, Although vagotomy has been shown to increase T cell proliferation and production of pro-inflammatory cytokines, this effect is not attenuated by selective α7 nAChR agonists, suggesting the involvement of other subunits such as α4, β2, and α5 (69, 72, 73). Other studies, however, do highlight the importance of the α7 nAChR in the anti-inflammatory effect of nicotine. Interestingly, a study by Galitovskiy et al. showed that α7 nAChR mRNA expression in colonic CD4 T cells was highly dependent on the inflammatory milieu. IL-4 was shown to upregulate α7 nAChR gene expression, whereas it was reduced by IL-12, possibly explaining the dualistic effect of nicotine in ulcerative colitis (Th2) versus Crohn’s disease (Th1/Th17) (74). This finding is especially of interest in allergic disorders, which are characterized by a strong type 2 inflammatory environment. In addition, a more recent study proposed α7 nAChR as a critical regulator for the immunosuppressive function of Tregs, mainly due to upregulation of immunoregulatory molecules CTLA-4 and FoxP3, and decreasing IL-2 levels (75). Considering the crucial role of Tregs in immune homeostasis and their capacity to suppress Th2 responses to allergens and other type 2 effector cells, novel ways to promote Treg cell stability and function, are being explored extensively in the treatment for allergic diseases. Whereas there has been an increasing appreciation for nicotinic receptors in the modulation of inflammation and homeostasis, muscarinic receptors have received much less attention so far. Of note, several studies have highlighted the importance of the M3 receptor in the generation of type 2 immune responses. Stimulation of mAChRs on naïve CD4 T cells promotes Th2 and Th17 lineages, while blockade of mAChR will lead to Th1 differentiation (71). Moreover, two recent studies demonstrated the importance of M3 receptor activity in the generation of Th2 responses in an enteric nematode infection model and an allergic airway inflammation model (76, 77). These studies suggest that the M3 receptor opposes the effect of α7 nAChR receptor activation (77). It should be emphasized though that the range of doses that activates each receptor is some log units apart, questioning if this interplay is indeed of physiological relevance. So far, most findings concerning cholinergic modulation of T cells are based on in vitro studies, hardly representing the complex nature of the inflammatory environment. Therefore, to better understand the functional consequences of cholinergic activation on T cells in vivo, further studies in experimental models are needed.
As mentioned earlier, functional expression of nAChR subunits and mAChRs subtypes has also been demonstrated in B cells (67–70). Information on the functional implications of cholinergic receptor activation is, however, rather limited. Nicotine affects the survival of B lymphocyte precursors during differentiation, an effect that was dependent on both α7 and β2 subunits (78). In addition, effects on antibody responses have been reported. A study by Skok et al. described a role α4β2 and α7 nAChRs in the formation of the pre-immune status and the regulation of antibody response, possibly by affecting CD40 expression and/or signaling. Mice lacking α4, β2, or α7 nAChR subunits exhibited lower levels of IgG antibodies (79, 80). By contrast, Fuji et al. reported higher levels of IgG1 antibodies in α7 nAChR knockout mice (81). The reason for this discrepancy is still unclear. However, both studies reported increased levels of IgG antibodies after immunization in the absence of α4, β2, or α7 nAChR subunits. Of note, while the effect on antibody response could be directly regulated via cholinergic receptors on B cells, it could also be attributed to cholinergic inhibition of pro-inflammatory cytokines by other immune cells, indirectly leading to the suppression of IgG antibodies. Also in humans, effects on antibody responses have been observed. When comparing smokers with non-smokers, the former were shown to exhibit lower levels of all serum immunoglobulins, except for IgE (82, 83). Unfortunately, cholinergic modulation of B cells in inflammatory conditions has been relatively unexplored until now, so its role in allergic inflammation remains unclear.
Cholinergic Modulation of Allergic Effector Cells
Mast cells, basophils, and eosinophils are considered as central effector cells in allergic inflammation. Their activation and subsequent release of granule stored mediators leads to many of the characteristic pathophysiological features observed in allergic responses. Ways to modulate these effector cells and to suppress their activation hold promise for the development of therapeutics for allergic diseases. All three types of granulocytes are shown to express cholinergic receptors, and are thus prone to cholinergic modulation.
Both muscarinic and nicotinic cholinergic receptors have been identified on mast cells (84–86). Moreover, mast cell–nerve associations have been shown in both small intestine and colon (87–89). Vagotomy in rats resulted into a decrease of mast cells in the jejunal mucosa (90). VNS on the other hand was shown to elevate histamine levels in the jejunal wall. To what extent this effect was due to increased synthesis or decreased release of histamine remains unclear (91). Thus far, studies describing the functional effects of cholinergic mast cell modulation have been rather conflicting. Early studies reported activation rather than inhibition of mast cell degranulation by ACh via muscarinic receptors (92–94). Other studies, however, failed to show an effect of ACh on mast cell degranulation (95, 96). This discrepancy was addressed by Masini et al., demonstrating that incubation with IgE increased the sensitivity of mast cells to ACh and induced a more homogeneous response (94). Nicotinic receptors on the other hand seem to mediate an anti-inflammatory effect. Expression of nicotinic receptor subunits α4, α7, and β2 was found in mouse bone marrow-derived mast cells. In addition, nicotine was shown to inhibit antigen-IgE-induced degranulation of mast cells in a dose dependent manner, an effect that was mimicked by the α7 nAChR subunit agonist, GTS-21. α-Bungarotoxin, a specific α7 antagonist, significantly inhibited the suppressive effect of GTS-21, further confirming the observed effect is α7 nAChR dependent (85). In line, α7, α9, and α10 receptor subunits are expressed in a rat mast cell/basophil cell line, RBL-2H3, in which nicotine induces suppression. Although nicotine did not inhibit degranulation, it suppressed late phase FcεRI-induced leukotriene and cytokine production, an effect that could be abrogated by siRNA mediated ablation of α7, α9, or α10 nAChRs (97). Interestingly, a recent study by Yamamoto et al. demonstrated a potential therapeutic effect for the cholinergic anti-inflammatory pathway in an experimental murine model of food allergy. Vagal stimulation by using 2-deoxy-d-glucose, a central vagal stimulant, ameliorated the development of food allergy, and was reversed by the nAChR antagonist, hexamethonium. Moreover, similar effects were obtained with nicotine and GTS-21, indicating the involvement of α7 nAChR. It was suggested that the amelioration of allergic symptoms where due to the suppression of mucosal mast cells; however, no direct evidence was provided. The possible involvement of other immune cells can thus not be excluded (89).
Basophils share many similarities with mast cells and are often considered as their circulating counterparts. However, the cholinergic modulation of basophils has been far less investigated compared with mast cells. So far, the earlier mentioned rat mast cell/basophil cell line, RBL-2H3 was shown to express α7, α9, and α10 nAChR receptor subunits (97). In addition, fluorescently labeled α-bungarotoxin was shown to bind to the surface of a human basophil cell line, and more recently the presence of nAChR α4, α7, and α1/α3/α5 subunits was shown in human blood basophils by flow cytometry (98, 99). In parallel with the observations in mast cells, Thompson-Cree et al. showed that nicotine agonists were able to inhibit IgE-induced histamine release from human peripheral blood basophils (100). In addition, a recent study showed similar in vitro and in vivo inhibition of human blood basophils from mild allergic asthma patients using ASM-024, a novel compound with nicotinic and muscarinic effects (99). The anti-inflammatory effect of ASM-024 was moreover confirmed in a murine model of allergic airway inflammation (101).
Eosinophil–cholinergic nerve interactions have been documented in several tissue compartments under inflammatory conditions, including lung, intestine, nose, and skin (102–108). The interaction between eosinophils and nerves seems to be mediated via adhesion molecules ICAM-1 and VCAM-1 and has been correlated with cholinergic nerve remodeling (103, 109). Eosinophil adhesion results in degranulation leading to the release of mediators such as major basic protein (MBP) and eosinophil peroxidase (EPO) (103). Binding of MBP and EPO leads to increased release of ACh due to upregulation of choline acetyltransferase and vesicular ACh transporter gene expression, and in addition due to antagonistic effect on inhibitory M2 receptors (110–112). Recently, modulation of cholinergic nerves by eosinophils has become increasingly clear and might contribute to pathological processes, such as smooth muscle contraction and increased mucus production, observed in allergic conditions. However, a possible anti-inflammatory effect of ACh on eosinophils should not be discarded. Expression of α3, α4, and α7 nAChR subunit transcripts and protein has been demonstrated in human blood cells. Moreover, it was shown that stimulation of these cells in vitro with DMPP, a nicotinic agonist, downregulated eosinophil function. DMPP inhibited LTC4 production, eosinophil migration, MMP-9 production, and intracellular calcium mobilization (113). In addition, DMPP was used earlier in an in vivo model of asthma, where it prevented airway hyperresponsiveness and reduced the number of lymphocytes and eosinophils. The obtained results could be in part attributed to the observed effects on eosinophil function; however, additional effects on other immune cells cannot be excluded (113, 114).
Conclusion
In recent years substantial advances have been made with respect to understanding the complex interactions between the nervous system and the immune system. The findings described in this review have highlighted a so far underappreciated role for the parasympathetic nervous system and cholinergic modulation in controlling type 2 adaptive immune responses. Several key players of the pathophysiological mechanism underlying allergic disorders are subject to cholinergic modulation (Figure 1) and could thus be exploited in the search for new therapeutic approaches to treat allergies. However, it should be noted that neuroimmune modulation is not only limited to the parasympathetic nervous system. Different neurotransmitters, originating from the parasympathetic, sympathetic, and ENS, are able to influence each other. For instance, neurotransmitters of the parasympathetic and the sympathetic nervous system are often found to exert antagonistic effects. In addition, immune cells render an enormous plasticity what expression of neurotransmitter receptors is concerned. A number of factors including the local microenvironment, which will differ in health versus disease, the maturation or activation state of cells, will influence receptor expression. Predicting the outcome of therapeutic interventions using specific receptor agonists and antagonists will therefore be challenging. A deeper understanding of how the different branches of the nervous system interact with each other and with the immune system, in both homeostasis and inflammation, will hold considerable promise for the development of new and innovative therapeutic strategies for allergic disorders and other inflammatory conditions.
Author Contributions
Drafting the manuscript: GB and GEB; revising the manuscript critically for important intellectual content: GEB, GSB, MF, EG-D, and GM.
Conflict of Interest Statement
The authors declare that the research was conducted in the absence of any commercial or financial relationships that could be construed as a potential conflict of interest.
Funding
MF is supported by a research fellowship from the Research Foundation—Flanders (FWO), Belgium. GEB is supported by grants of the Research Foundation—Flanders (FWO), the agency for Innovation by Science and Technology (IWT), Belgium, and European Research Council (ERC).
References
1. Tracey KJ, Borovikova LV, Ivanova S, Zhang M, Yang H, Botchkina GI, et al. Vagus nerve stimulation attenuates the systemic inflammatory response to endotoxin. Nature (2000) 405:458–62. doi:10.1038/35013070
2. Rosas-Ballina M, Ochani M, Parrish WR, Ochani K, Harris YT, Huston JM, et al. Splenic nerve is required for cholinergic antiinflammatory pathway control of TNF in endotoxemia. Proc Natl Acad Sci U S A (2008) 105:11008–13. doi:10.1073/pnas.0803237105
3. Pavlov VA, Ochani M, Yang L-H, Gallowitsch-Puerta M, Ochani K, Lin X, et al. Selective α7-nicotinic acetylcholine receptor agonist GTS-21 improves survival in murine endotoxemia and severe sepsis*. Crit Care Med (2007) 35:1139–44. doi:10.1097/01.CCM.0000259381.56526.96
4. Jin H, Guo J, Liu J, Lyu B, Foreman RD, Yin J, et al. Anti-inflammatory effects and mechanisms of vagal nerve stimulation combined with electroacupuncture in a rodent model of TNBS-induced colitis. Am J Physiol Gastrointest Liver Physiol (2017) 313:G192–202. doi:10.1152/ajpgi.00254.2016
5. Bonaz B, Sinniger V, Pellissier S. Vagus nerve stimulation: a new promising therapeutic tool in inflammatory bowel disease. J Intern Med (2017) 282:46–63. doi:10.1111/joim.12611
6. Sherrill DL, Martinez FD, Lebowitz MD, Holdaway MD, Flannery EM, Herbison GP, et al. Longitudinal effects of passive smoking on pulmonary function in New Zealand children. Am Rev Respir Dis (1992) 145:1136–41. doi:10.1164/ajrccm/145.5.1136
7. Troisi RJ, Speizer FE, Rosner B, Trichopoulos D, Willett WC. Cigarette smoking and incidence of chronic bronchitis and asthma in women. Chest (1995) 108:1557–61. doi:10.1378/chest.108.6.1557
8. Siroux V, Pin I, Oryszczyn MP, Le Moual N, Kauffmann F. Relationships of active smoking to asthma and asthma severity in the EGEA study. Epidemiological study on the genetics and environment of asthma. Eur Respir J (2000) 15:470–7. doi:10.1034/j.1399-3003.2000.15.08.x
9. Pietinalho A, Pelkonen A, Rytilä P. Linkage between smoking and asthma. Allergy (2009) 64:1722–7. doi:10.1111/j.1398-9995.2009.02208.x
10. Saulyte J, Regueira C, Montes-Martínez A, Khudyakov P, Takkouche B. Active or passive exposure to tobacco smoking and allergic rhinitis, allergic dermatitis, and food allergy in adults and children: a systematic review and meta-analysis. PLoS Med (2014) 11:e1001611. doi:10.1371/journal.pmed.1001611
11. Hillderdahl G, Rylander R. Asthma and cessation of smoking. Clin Exp Allergy (1984) 14:45–7. doi:10.1111/j.1365-2222.1984.tb02188.x
12. Langhammer A, Johnsen R, Holmen J, Gulsvik A, Bjermer L. Cigarette smoking gives more respiratory symptoms among women than among men. The Nord-Trondelag Health Study (HUNT). J Epidemiol Community Health (2000) 54:917–22. doi:10.1136/JECH.54.12.917
13. Godtfredsen NS, Lange P, Prescott E, Osler M, Vestbo J. Changes in smoking habits and risk of asthma: a longitudinal population based study. Eur Respir J (2001) 18:549–54. doi:10.1183/09031936.01.00100801
14. Linneberg A, Nielsen NH, Madsen F, Frølund L, Dirksen A, Jørgensen T. Smoking and the development of allergic sensitization to aeroallergens in adults: a prospective population-based study. The Copenhagen Allergy Study. Allergy (2001) 56:328–32. doi:10.1034/j.1398-9995.2000.00509.x-i1
15. Hancox RJ, Welch D, Poulton R, Taylor DR, McLachlan CR, Greene JM, et al. Cigarette smoking and allergic sensitization: a 32-year population-based cohort study. J Allergy Clin Immunol (2008) 121:38–42.e3. doi:10.1016/j.jaci.2007.09.052
16. Tilp C, Bucher H, Haas H, Duechs MJ, Wex E, Erb KJ. Effects of conventional tobacco smoke and nicotine-free cigarette smoke on airway inflammation, airway remodelling and lung function in a triple allergen model of severe asthma. Clin Exp Allergy (2016) 46:957–72. doi:10.1111/cea.12665
17. Montoro J, Mullol J, Jáuregui I, Dávila I, Ferrer M, Bartra J, et al. Stress and allergy. J Investig Allergol Clin Immunol (2009) 19:40–7.
18. Gorman JM, Sloan RP. Heart rate variability in depressive and anxiety disorders. Am Heart J (2000) 140:77–83. doi:10.1067/mhj.2000.109981
19. Goodnick PJ, Rush AJ, George MS, Marangell LB, Sackeim HA. Vagus nerve stimulation in depression. Expert Opin Pharmacother (2001) 2:1061–3. doi:10.1517/14656566.2.7.1061
20. Nahas Z, Teneback C, Chae J-H, Mu Q, Molnar C, Kozel FA, et al. Serial vagus nerve stimulation functional MRI in treatment-resistant depression. Neuropsychopharmacology (2007) 32:1649–60. doi:10.1038/sj.npp.1301288
21. Miu AC, Heilman RM, Miclea M. Reduced heart rate variability and vagal tone in anxiety: trait versus state, and the effects of autogenic training. Auton Neurosci (2009) 145:99–103. doi:10.1016/j.autneu.2008.11.010
22. Ghia J-E, Blennerhassett P, Collins SM. Impaired parasympathetic function increases susceptibility to inflammatory bowel disease in a mouse model of depression. J Clin Invest (2008) 118:2209–18. doi:10.1172/JCI32849
23. Shifrin H, Mouhadeb O, Gluck N, Varol C, Weinstock M. Cholinergic anti-inflammatory pathway does not contribute to prevention of ulcerative colitis by novel indoline carbamates. J Neuroimmune Pharmacol (2017) 12:484–91. doi:10.1007/s11481-017-9735-8
24. Kanashiro A, Talbot J, Peres RS, Pinto LG, Bassi GS, Cunha TM, et al. Neutrophil recruitment and articular hyperalgesia in antigen-induced arthritis are modulated by the cholinergic anti-inflammatory pathway. Basic Clin Pharmacol Toxicol (2016) 119:453–7. doi:10.1111/bcpt.12611
25. Pohanka M, Pavlis O, Pikula J. Galantamine effect on tularemia pathogenesis in a BALB/c mouse model. Iran Biomed J (2012) 16:156–61. doi:10.6091/ibj.993.2012
26. Pavlov VA, Parrish WR, Rosas-Ballina M, Ochani M, Puerta M, Ochani K, et al. Brain acetylcholinesterase activity controls systemic cytokine levels through the cholinergic anti-inflammatory pathway. Brain Behav Immun (2009) 23:41–5. doi:10.1016/j.bbi.2008.06.011
27. Reale M, Iarlori C, Gambi F, Feliciani C, Isabella L, Gambi D. The acetylcholinesterase inhibitor, donepezil, regulates a Th2 bias in Alzheimer’s disease patients. Neuropharmacology (2006) 50:606–13. doi:10.1016/j.neuropharm.2005.11.006
28. Corsini E, Sokooti M, Galli CL, Moretto A, Colosio C. Pesticide induced immunotoxicity in humans: a comprehensive review of the existing evidence. Toxicology (2013) 307:123–35. doi:10.1016/j.tox.2012.10.009
29. Zabrodskii PF, Gromov MS, Yafarova IK. Dysfunction of Th1 and Th2 lymphocytes and change in blood cytokine concentration at various stages of chronic intoxication with organophosphorus compounds. Bull Exp Biol Med (2014) 156:789–92. doi:10.1007/s10517-014-2451-y
30. Fukuyama T, Nishino R, Kosaka T, Watanabe Y, Kurosawa Y, Ueda H, et al. Effects of prior oral exposure to combinations of environmental immunosuppressive agents on ovalbumin allergen-induced allergic airway inflammation in Balb/c mice. Immunopharmacol Immunotoxicol (2014) 36:261–70. doi:10.3109/08923973.2014.926915
31. Fukuyama T, Tajima Y, Hayashi K, Ueda H, Kosaka T. Prior or coinstantaneous oral exposure to environmental immunosuppressive agents aggravates mite allergen-induced atopic dermatitis-like immunoreaction in NC/Nga mice. Toxicology (2011) 289:132–40. doi:10.1016/j.tox.2011.08.003
32. Mattila P, Joenväärä S, Renkonen J, Toppila-Salmi S, Renkonen R. Allergy as an epithelial barrier disease. Clin Transl Allergy (2011) 1:5. doi:10.1186/2045-7022-1-5
33. Schleimer RP, Berdnikovs S. Etiology of epithelial barrier dysfunction in patients with type 2 inflammatory diseases. J Allergy Clin Immunol (2017) 139:1752–61. doi:10.1016/j.jaci.2017.04.010
34. Bulek K, Swaidani S, Aronica M, Li X. Epithelium: the interplay between innate and Th2 immunity. Immunol Cell Biol (2010) 88:257–68. doi:10.1038/icb.2009.113
35. Barnes PJ. Pathophysiology of allergic inflammation. Immunol Rev (2011) 242:31–50. doi:10.1111/j.1600-065X.2011.01020.x
36. Cheng LE, Locksley RM. Allergic inflammation—innately homeostatic. Cold Spring Harb Perspect Biol (2015) 7:a016352. doi:10.1101/cshperspect.a016352
37. Berthoud HR, Carlson NR, Powley TL. Topography of efferent vagal innervation of the rat gastrointestinal tract. Am J Physiol (1991) 260:R200–7.
38. Berthoud HR, Jedrzejewska A, Powley TL. Simultaneous labeling of vagal innervation of the gut and afferent projections from the visceral forebrain with dil injected into the dorsal vagal complex in the rat. J Comp Neurol (1990) 301:65–79. doi:10.1002/cne.903010107
39. Matteoli G, Gomez-Pinilla PJ, Nemethova A, Di Giovangiulio M, Cailotto C, van Bree SH, et al. A distinct vagal anti-inflammatory pathway modulates intestinal muscularis resident macrophages independent of the spleen. Gut (2013) 63:938–48. doi:10.1136/gutjnl-2013-304676
40. van der Velden VHJ, Hulsmann AR. Autonomic innervation of human airways: structure, function, and pathophysiology in asthma. Neuroimmunomodulation (1999) 6:145–59. doi:10.1159/000026376
41. Scott GD, Fryer AD. Role of parasympathetic nerves and muscarinic receptors in allergy and asthma. Chem Immunol Allergy (2012) 98:48–69. doi:10.1159/000336498
42. Curtis BJ, Radek KA. Cholinergic regulation of keratinocyte innate immunity and permeability barrier integrity: new perspectives in epidermal immunity and disease. J Invest Dermatol (2012) 132:28–42. doi:10.1038/jid.2011.264
43. Wessler I, Kirkpatrick CJ. Acetylcholine beyond neurons: the non-neuronal cholinergic system in humans. Br J Pharmacol (2009) 154:1558–71. doi:10.1038/bjp.2008.185
44. Lesko S, Wessler I, Gäbel G, Petto C, Pfannkuche H. Cholinergic modulation of epithelial integrity in the proximal colon of pigs. Cells Tissues Organs (2013) 197:411–20. doi:10.1159/000345437
45. Costantini TW, Bansal V, Peterson CY, Loomis WH, Putnam JG, Rankin F, et al. Efferent vagal nerve stimulation attenuates gut barrier injury after burn: modulation of intestinal occludin expression. J Trauma (2010) 68:1349–56. doi:10.1097/TA.0b013e3181dccea0
46. Krzyzaniak M, Ortiz-Pomales Y, Lopez N, Reys LG, Cheadle G, Wolf P, et al. CPSI-121 pharmacologically prevents intestinal barrier dysfunction after cutaneous burn through a vagus nerve-dependent mechanism. J Trauma Acute Care Surg (2012) 72:355–63. doi:10.1097/TA.0b013e31824484fe
47. Hu S, Du M-H, Luo H-M, Wang H, Lv Y, Ma L, et al. Electroacupuncture at zusanli (ST36) prevents intestinal barrier and remote organ dysfunction following gut ischemia through activating the cholinergic anti-inflammatory-dependent mechanism. Evid Based Complement Alternat Med (2013) 2013:592127. doi:10.1155/2013/592127
48. Dhawan S, Hiemstra IH, Verseijden C, Hilbers FW, te Velde AA, Willemsen LEM, et al. Cholinergic receptor activation on epithelia protects against cytokine-induced barrier dysfunction. Acta Physiol (2015) 213:846–59. doi:10.1111/apha.12469
49. Dong J, Segawa R, Mizuno N, Hiratsuka M, Hirasawa N. Inhibitory effects of nicotine derived from cigarette smoke on thymic stromal lymphopoietin production in epidermal keratinocytes. Cell Immunol (2016) 302:19–25. doi:10.1016/j.cellimm.2016.01.001
50. Berin MC, Yang PC, Ciok L, Waserman S, Perdue MH. Role for IL-4 in macromolecular transport across human intestinal epithelium. Am J Physiol (1999) 276:C1046–52.
51. Ceponis PJM, Botelho F, Richards CD, McKay DM. Interleukins 4 and 13 increase intestinal epithelial permeability by a phosphatidylinositol 3-kinase pathway. J Biol Chem (2000) 275:29132–7. doi:10.1074/jbc.M003516200
52. Madden KB, Whitman L, Sullivan C, Gause WC, Urban JF, Katona IM, et al. Role of STAT6 and mast cells in IL-4- and IL-13-induced alterations in murine intestinal epithelial cell function. J Immunol (2002) 169:4417–22. doi:10.4049/jimmunol.169.8.4417
53. Prasad S, Mingrino R, Kaukinen K, Hayes KL, Powell RM, MacDonald TT, et al. Inflammatory processes have differential effects on claudins 2, 3 and 4 in colonic epithelial cells. Lab Invest (2005) 85:1139–62. doi:10.1038/labinvest.3700316
54. McDermott JR, Bartram RE, Knight PA, Miller HRP, Garrod DR, Grencis RK. Mast cells disrupt epithelial barrier function during enteric nematode infection. Proc Natl Acad Sci U S A (2003) 100:7761–6. doi:10.1073/pnas.1231488100
55. Furuta GT, Nieuwenhuis EES, Karhausen J, Gleich G, Blumberg RS, Lee JJ, et al. Eosinophils alter colonic epithelial barrier function: role for major basic protein. Am J Physiol Gastrointest Liver Physiol (2005) 289:G890–7. doi:10.1152/ajpgi.00015.2005
56. Groschwitz KR, Hogan SP. Intestinal barrier function: molecular regulation and disease pathogenesis. J Allergy Clin Immunol (2009) 124:3–20. doi:10.1016/j.jaci.2009.05.038
57. Galle-Treger L, Suzuki Y, Patel N, Sankaranarayanan I, Aron JL, Maazi H, et al. Nicotinic acetylcholine receptor agonist attenuates ILC2-dependent airway hyperreactivity. Nat Commun (2016) 7:13202. doi:10.1038/ncomms13202
58. de Jonge WJ, van der Zanden EP, The FO, Bijlsma MF, van Westerloo DJ, Bennink RJ, et al. Stimulation of the vagus nerve attenuates macrophage activation by activating the Jak2-STAT3 signaling pathway. Nat Immunol (2005) 6:844–51. doi:10.1038/ni1229
59. Cailotto C, Gomez-Pinilla PJ, Costes LM, van der Vliet J, Di Giovangiulio M, Némethova A, et al. Neuro-anatomical evidence indicating indirect modulation of macrophages by vagal efferents in the intestine but not in the spleen. PLoS One (2014) 9:e87785. doi:10.1371/journal.pone.0087785
60. Olofsson P, Katz D, Rosas-Ballina M, Levine YA, Ochani M, Valdés-Ferrer SI, et al. α7 nicotinic acetylcholine receptor (α7nAChR) expression in bone marrow-derived non-T cells is required for the inflammatory reflex. Mol Med (2012) 18:1. doi:10.2119/molmed.2011.00405
61. Aicher A, Heeschen C, Mohaupt M, Cooke JP, Zeiher AM, Dimmeler S. Nicotine strongly activates dendritic cell-mediated adaptive immunity: potential role for progression of atherosclerotic lesions. Circulation (2003) 107:604–11. doi:10.1161/01.CIR.0000047279.42427.6D
62. Kawashima K, Yoshikawa K, Fujii YX, Moriwaki Y, Misawa H. Expression and function of genes encoding cholinergic components in murine immune cells. Life Sci (2007) 80:2314–9. doi:10.1016/j.lfs.2007.02.036
63. Nouri-Shirazi M, Guinet E. Evidence for the immunosuppressive role of nicotine on human dendritic cell functions. Immunology (2003) 109:365–73. doi:10.1046/j.1365-2567.2003.01655.x
64. Gao FG, Wan DF, Gu JR. Ex vivo nicotine stimulation augments the efficacy of therapeutic bone marrow-derived dendritic cell vaccination. Clin Cancer Res (2007) 13:3706–12. doi:10.1158/1078-0432.CCR-07-0028
65. Gori S, Vermeulen M, Remes-Lenicov F, Jancic C, Scordo W, Ceballos A, et al. Acetylcholine polarizes dendritic cells toward a Th2-promoting profile. Allergy (2017) 72:221–31. doi:10.1111/all.12926
66. Liu T, Xie C, Chen X, Zhao F, Liu A-M, Cho D-B, et al. Role of muscarinic receptor activation in regulating immune cell activity in nasal mucosa. Allergy (2009) 65:969–77. doi:10.1111/j.1398-9995.2009.02281.x
67. Skok MV, Kalashnik EN, Koval LN, Tsetlin VI, Utkin YN, Changeux J-P, et al. Functional nicotinic acetylcholine receptors are expressed in B lymphocyte-derived cell lines. Mol Pharmacol (2003) 64:885–9. doi:10.1124/mol.64.4.885
68. Kawashima K, Fujii T. The lymphocytic cholinergic system and its contribution to the regulation of immune activity. Life Sci (2003) 74:675–96. doi:10.1016/j.lfs.2003.09.037
69. Skok MV. Non-neuronal nicotinic acetylcholine receptors: cholinergic regulation of the immune processes. Neurophysiology (2007) 39:264–71. doi:10.1007/s11062-007-0037-2
70. Fujii T, Mashimo M, Moriwaki Y, Misawa H, Ono S, Horiguchi K, et al. Physiological functions of the cholinergic system in immune cells. J Pharmacol Sci (2017) 134:1–21. doi:10.1016/j.jphs.2017.05.002
71. Qian J, Galitovskiy V, Chernyavsky AI, Marchenko S, Grando SA. Plasticity of the murine spleen T-cell cholinergic receptors and their role in in vitro differentiation of naïve CD4 T cells toward the Th1, Th2 and Th17 lineages. Genes Immun (2011) 12:222–30. doi:10.1038/gene.2010.72
72. Matsunaga K, Klein TW, Friedman H, Yamamoto Y. Involvement of nicotinic acetylcholine receptors in suppression of antimicrobial activity and cytokine responses of alveolar macrophages to Legionella pneumophila infection by nicotine. J Immunol (2001) 167:6518–24. doi:10.4049/jimmunol.167.11.6518
73. Orr-Urtreger A, Kedmi M, Rosner S, Karmeli F, Rachmilewitz D. Increased severity of experimental colitis in alpha 5 nicotinic acetylcholine receptor subunit-deficient mice. Neuroreport (2005) 16:1123–7. doi:10.1097/00001756-200507130-00018
74. Galitovskiy V, Qian J, Chernyavsky AI, Marchenko S, Gindi V, Edwards RA, et al. Cytokine-induced alterations of α7 nicotinic receptor in colonic CD4 T cells mediate dichotomous response to nicotine in murine models of Th1/Th17- versus Th2-mediated colitis. J Immunol (2011) 187:2677–87. doi:10.4049/jimmunol.1002711
75. Wang D-W, Zhou R-B, Yao Y-M, Zhu X-M, Yin Y-M, Zhao G-J, et al. Stimulation of α7 nicotinic acetylcholine receptor by nicotine increases suppressive capacity of naturally occurring CD4+CD25+ regulatory T cells in mice in vitro. J Pharmacol Exp Ther (2010) 335:553–61. doi:10.1124/jpet.110.169961
76. Cao R, Dong X-W, Jiang J-X, Yan X-F, He J-S, Deng Y-M, et al. M3 muscarinic receptor antagonist bencycloquidium bromide attenuates allergic airway inflammation, hyperresponsiveness and remodeling in mice. Eur J Pharmacol (2011) 655:83–90. doi:10.1016/j.ejphar.2011.01.024
77. McLean LP, Smith A, Cheung L, Urban JF, Sun R, Grinchuk V, et al. Type 3 muscarinic receptors contribute to intestinal mucosal homeostasis and clearance of Nippostrongylus brasiliensis through induction of TH2 cytokines. Am J Physiol Gastrointest Liver Physiol (2016) 311:G130–41. doi:10.1152/ajpgi.00461.2014
78. Skok M, Grailhe R, Agenes F, Changeux J-P. The role of nicotinic acetylcholine receptors in lymphocyte development. J Neuroimmunol (2006) 171:86–98. doi:10.1016/j.jneuroim.2005.09.011
79. Skok M, Grailhe R, Changeux J-P. Nicotinic receptors regulate B lymphocyte activation and immune response. Eur J Pharmacol (2005) 517:246–51. doi:10.1016/j.ejphar.2005.05.011
80. Skok MV, Grailhe R, Agenes F, Changeux J-P. The role of nicotinic receptors in B-lymphocyte development and activation. Life Sci (2007) 80:2334–6. doi:10.1016/j.lfs.2007.02.005
81. Fujii YX, Fujigaya H, Moriwaki Y, Misawa H, Kasahara T, Grando SA, et al. Enhanced serum antigen-specific IgG1 and proinflammatory cytokine production in nicotinic acetylcholine receptor alpha7 subunit gene knockout mice. J Neuroimmunol (2007) 189:69–74. doi:10.1016/j.jneuroim.2007.07.003
82. Burrows B, Halonen M, Barbee RA, Lebowitz MD. The relationship of serum immunoglobulin E to cigarette smoking. Am Rev Respir Dis (1981) 124:523–5. doi:10.1164/arrd.1981.124.5.523
83. Andersen P, Pedersen OF, Bach B, Bonde GJ. Serum antibodies and immunoglobulins in smokers and nonsmokers. Clin Exp Immunol (1982) 47:467–73.
84. Masini E, Fantozzi R, Blandina P, Brunelleschi S, Mannaioni PF. Muscarinic cholinergic receptor binding in rat mast cells. Agents Actions (1983) 13:327–32. doi:10.1007/BF01971484
85. Kageyama-Yahara N, Suehiro Y, Yamamoto T, Kadowaki M. IgE-induced degranulation of mucosal mast cells is negatively regulated via nicotinic acetylcholine receptors. Biochem Biophys Res Commun (2008) 377:321–5. doi:10.1016/j.bbrc.2008.10.004
86. Kindt F, Wiegand S, Niemeier V, Kupfer J, Löser C, Nilles M, et al. Reduced expression of nicotinic α subunits 3, 7, 9 and 10 in lesional and nonlesional atopic dermatitis skin but enhanced expression of α subunits 3 and 5 in mast cells. Br J Dermatol (2008) 159:847–57. doi:10.1111/j.1365-2133.2008.08774.x
87. Stead RH, Dixon MF, Bramwell NH, Riddell RH, Bienenstock J. Mast cells are closely apposed to nerves in the human gastrointestinal mucosa. Gastroenterology (1989) 97:575–85. doi:10.1016/0016-5085(89)90627-6
88. Williams RM, Berthoud HR, Stead RH. Vagal afferent nerve fibres contact mast cells in rat small intestinal mucosa. Neuroimmunomodulation (1997) 4:266–70. doi:10.1159/000097346
89. Yamamoto T, Kodama T, Lee J, Utsunomiya N, Hayashi S, Sakamoto H, et al. Anti-allergic role of cholinergic neuronal pathway via α7 nicotinic ACh receptors on mucosal mast cells in a murine food allergy model. PLoS One (2014) 9:e85888. doi:10.1371/journal.pone.0085888
90. Gottwald T, LhotAk S, Stead RH. Effect of truncal vagotomy and capsaicin on mast cells and IgA-positive plasma cells in rat jejunal mucosa. Neurogastroenterol Motil (1997) 9:25–32. doi:10.1046/j.1365-2982.1997.d01-4.x
91. Gottwald TP, Hewlett BR, Lhoták S, Stead RH. Electrical stimulation of the vagus nerve modulates the histamine content of mast cells in the rat jejunal mucosa. Neuroreport (1995) 7:313–7. doi:10.1097/00001756-199512290-00075
92. Fantozzi R, Masini E, Blandina P, Mannaioni PF, Bani-Sacchi T. Release of histamine from rat mast cells by acetylcholine. Nature (1978) 273:473–4. doi:10.1038/273473a0
93. Blandina P, Fantozzi R, Mannaioni PF, Masini E. Characteristics of histamine release evoked by acetylcholine in isolated rat mast cells. J Physiol (1980) 301:281–93. doi:10.1113/jphysiol.1980.sp013205
94. Masini E, Fantozzi R, Conti A, Blandina P, Brunelleschi S, Mannaioni PF. Mast cell heterogeneity in response to cholinergic stimulation. Int Arch Allergy Appl Immunol (1985) 77:184–5. doi:10.1159/000233780
95. Kazimierczak W, Adamas B, Maśliński C. Failure of acetylcholine to release histamine from rat mast cells. Agents Actions (1980) 10:1–3. doi:10.1007/BF02024170
96. Leung KB, Pearce FL. A comparison of histamine secretion from peritoneal mast cells of the rat and hamster. Br J Pharmacol (1984) 81:693–701. doi:10.1111/j.1476-5381.1984.tb16136.x
97. Mishra NC, Rir-sima-ah J, Boyd RT, Singh SP, Gundavarapu S, Langley RJ, et al. Nicotine inhibits Fc epsilon RI-induced cysteinyl leukotrienes and cytokine production without affecting mast cell degranulation through alpha 7/alpha 9/alpha 10-nicotinic receptors. J Immunol (2010) 185:588–96. doi:10.4049/jimmunol.0902227
98. Sudheer PS, Hall JE, Donev R, Read G, Rowbottom A, Williams PE. Nicotinic acetylcholine receptors on basophils and mast cells. Anaesthesia (2006) 61:1170–4. doi:10.1111/j.1365-2044.2006.04870.x
99. Watson BM, Oliveria JP, Nusca GM, Smith SG, Beaudin S, Dua B, et al. Inhibition of allergen-induced basophil activation by ASM-024, a nicotinic receptor ligand. Int Arch Allergy Immunol (2014) 165:255–64. doi:10.1159/000370068
100. Thompson-Cree MEM, Shields MD, Stevenson MR, Ennis M. The effect of nicotine on basophil histamine release. Inflamm Res (2004) 53:211–4. doi:10.1007/s00011-004-1249-1
101. Assayag EI, Beaulieu M-J, Cormier Y. Bronchodilatory and anti-inflammatory effects of ASM-024, a nicotinic receptor ligand, developed for the treatment of asthma. PLoS One (2014) 9:e86091. doi:10.1371/journal.pone.0086091
102. Hogan SP, Mishra A, Brandt EB, Royalty MP, Pope SM, Zimmermann N, et al. A pathological function for eotaxin and eosinophils in eosinophilic gastrointestinal inflammation. Nat Immunol (2001) 2:353–60. doi:10.1038/86365
103. Sawatzky DA, Kingham PJ, Court E, Kumaravel B, Fryer AD, Jacoby DB, et al. Eosinophil adhesion to cholinergic nerves via ICAM-1 and VCAM-1 and associated eosinophil degranulation. Am J Physiol Lung Cell Mol Physiol (2002) 282:L1279–88. doi:10.1152/ajplung.00279.2001
104. Kingham PJ, Costello RW, McLean WG. Eosinophil and airway nerve interactions. Pulm Pharmacol Ther (2003) 16:9–13. doi:10.1016/S1094-5539(02)00093-7
105. Foster EL, Simpson EL, Fredrikson LJ, Lee JJ, Lee NA, Fryer AD, et al. Eosinophils increase neuron branching in human and murine skin and in vitro. PLoS One (2011) 6:e22029. doi:10.1371/journal.pone.0022029
106. Smyth CM, Akasheh N, Woods S, Kay E, Morgan RK, Thornton MA, et al. Activated eosinophils in association with enteric nerves in inflammatory bowel disease. PLoS One (2013) 8:e64216. doi:10.1371/journal.pone.0064216
107. Cirillo C, Bessissow T, Desmet A-S, Vanheel H, Tack J, Vanden Berghe P. Evidence for neuronal and structural changes in submucous ganglia of patients with functional dyspepsia. Am J Gastroenterol (2015) 110:1205–15. doi:10.1038/ajg.2015.158
108. Thornton MA, Akasheh N, Walsh M-T, Moloney M, Sheahan PO, Smyth CM, et al. Eosinophil recruitment to nasal nerves after allergen challenge in allergic rhinitis. Clin Immunol (2013) 147:50–7. doi:10.1016/j.clim.2013.02.008
109. Durcan N, Costello RW, McLean WG, Blusztajn J, Madziar B, Fenech AG, et al. Eosinophil-mediated cholinergic nerve remodeling. Am J Respir Cell Mol Biol (2006) 34:775–86. doi:10.1165/rcmb.2005-0196OC
110. Jacoby DB, Gleich GJ, Fryer AD. Human eosinophil major basic protein is an endogenous allosteric antagonist at the inhibitory muscarinic M2 receptor. J Clin Invest (1993) 91:1314–8. doi:10.1172/JCI116331
111. Adamko DJ, Yost BL, Gleich GJ, Fryer AD, Jacoby DB. Ovalbumin sensitization changes the inflammatory response to subsequent parainfluenza infection. Eosinophils mediate airway hyperresponsiveness, m(2) muscarinic receptor dysfunction, and antiviral effects. J Exp Med (1999) 190:1465–78. doi:10.1084/jem.190.10.1465
112. Akasheh N, Walsh M-T, Costello RW. Eosinophil peroxidase induces expression of cholinergic genes via cell surface neural interactions. Mol Immunol (2014) 62:37–45. doi:10.1016/j.molimm.2014.05.014
113. Blanchet M-R, Langlois A, Israël-Assayag E, Beaulieu M-J, Ferland C, Laviolette M, et al. Modulation of eosinophil activation in vitro by a nicotinic receptor agonist. J Leukoc Biol (2007) 81:1245–51. doi:10.1189/jlb.0906548
Keywords: parasympathetic nervous system, allergic inflammation, type 2 immunity, cholinergic anti-inflammatory pathway, neuroimmune interactions, vagus nerve
Citation: Bosmans G, Shimizu Bassi G, Florens M, Gonzalez-Dominguez E, Matteoli G and Boeckxstaens GE (2017) Cholinergic Modulation of Type 2 Immune Responses. Front. Immunol. 8:1873. doi: 10.3389/fimmu.2017.01873
Received: 29 September 2017; Accepted: 08 December 2017;
Published: 19 December 2017
Edited by:
Valentin A. Pavlov, Northwell Health, United StatesReviewed by:
Maryna Skok, Palladin Institute of Biochemistry (NAS Ukraine), UkraineRegina Pekelmann Markus, University of São Paulo, Brazil
Copyright: © 2017 Bosmans, Shimizu Bassi, Florens, Gonzalez-Dominguez, Matteoli and Boeckxstaens. This is an open-access article distributed under the terms of the Creative Commons Attribution License (CC BY). The use, distribution or reproduction in other forums is permitted, provided the original author(s) or licensor are credited and that the original publication in this journal is cited, in accordance with accepted academic practice. No use, distribution or reproduction is permitted which does not comply with these terms.
*Correspondence: Guy E. Boeckxstaens, Z3V5LmJvZWNreHN0YWVucyYjeDAwMDQwO2t1bGV1dmVuLmJl