- 1Mikrobiologisches Institut – Klinische Mikrobiologie, Immunologie und Hygiene, Universitätsklinikum Erlangen, Friedrich-Alexander-Universität (FAU) Erlangen-Nürnberg, Erlangen, Germany
- 2Medical Immunology Campus Erlangen, Interdisciplinary Center of the FAU, Erlangen, Germany
The kinetoplastid protozoan parasites belonging to the genus Leishmania are the causative agents of different clinical forms of leishmaniasis, a vector-borne infectious disease with worldwide prevalence. The protective host immune response against Leishmania parasites relies on myeloid cells such as dendritic cells and macrophages in which upon stimulation by cytokines (e.g., interferon-γ) a complex network of signaling pathways is switched on leading to strong antimicrobial activities directed against the intracellular parasite stage. The regulation of these pathways classically depends on post-translational modifications of proteins, with phosphorylation events playing a cardinal role. Leishmania parasites deactivate their phagocytic host cells by inducing specific mammalian phosphatases that are capable to impede signaling. On the other hand, there is now also evidence that Leishmania spp. themselves express phosphatases that might target host cell molecules and thereby facilitate the intracellular survival of the parasite. This review will present an overview on the modulation of host phosphatases by Leishmania parasites as well as on the known families of Leishmania phosphatases and their possible function as virulence factors. A more detailed understanding of the role of phosphatases in Leishmania–host cell interactions might open new avenues for the treatment of non-healing, progressive forms of leishmaniasis.
Introduction
Epidemiology and Disease Development
Leishmaniasis is a worldwide prevalent infectious disease. Among all parasitic infections, leishmaniasis ranks second in mortality after malaria (1). Leishmaniasis is primarily encountered in tropical and subtropical countries, with an estimated number of 0.7–1 million new cases of cutaneous leishmaniasis (CL) and 50,000 to 90,000 new cases of visceral leishmaniasis accompanied by 20,000–30,000 deaths per year (World Health Organization1 [WHO]). Due to the absence of an effective and well-tolerated vaccine, the lack of simple and efficient treatments and the strong correlation between disease and poor socioeconomic conditions, WHO has classified leishmaniasis as one of the 20 neglected tropical diseases worldwide.2
Leishmaniasis is caused by kinetoplastid parasites of the Trypanosomatidae family that comprises monogenetic genera often found in insects or plants as well as several human pathogens of the heteroxenous genera Trypanosoma and Leishmania (L.). In nature, Leishmania parasites are transmitted by the bites of sand flies, which inject the extracellular, flagellated parasite stage (so-called infective or metacyclic promastigote) into the dermis of the skin. Once promastigotes are released into the blood pool of the skin wound, they are endocytosed by phagocytic cells (initially polymorphonuclear, neutrophilic granulocytes [PMNs], then blood monocytes, dendritic cells, and macrophages) in which they differentiate into intracellular, so-called amastigote Leishmania (2–4). Amastigotes contain only a stumpy rest of the flagellum within the flagellar pocket of the parasite. Leishmania amastigotes will multiply in non-activated phagocytic cells and are transported via the lymph and blood stream to various tissues and organs (e.g., draining lymph nodes, spleen, liver, bone marrow, healthy skin), depending on the host immune response and the Leishmania species involved. The life cycle of the parasite will be completed once a sand fly ingests amastigote-infected tissue phagocytes during its blood meal. Within the digestive tract of the vector, the parasites retransform into promastigotes, which develop from a non-infective procyclic into the infective metacyclic stage (5).
Depending on the affected tissues, leishmaniasis can be divided into three major forms: CL, mucocutaneous leishmaniasis (MCL), and visceral leishmaniasis (VL, also known as Kala-Azar) (6). Members of the subgenus Leishmania (e.g., L. major, L. tropica, L. infantum, L. mexicana, L. amazonensis) and the subgenus Viannia (L. braziliensis, L. guyanensis, L. panamensis) can cause cutaneous disease. CL is characterized by chronic papular, erythematous, plaque-like, or ulcerative skin lesions. These lesions ultimately heal in immunocompetent patients after months to years of infection (7). MCL symptoms develop especially after an infection with the South American species L. braziliensis, L. panamensis or L. guyanensis. This form of the disease is usually associated with a higher morbidity because of the destruction of mucocutaneous tissue sites such as the nose and nasal septum, the oropharynx or the palate (7). VL is the most severe form of the disease due to the systemic spread of the parasite and usually results from an infection with viscerotropic L. infantum strains (identical with Leishmania chagasi) or Leishmania donovani. VL, which is characterized by persistent fever, enlargement of liver and spleen, and pancytopenia resulting from secondary hemophagocytosis in the bone marrow, is lethal if untreated (8, 9). All these various disease developments are the result of a complex equation influenced by the arsenal of virulence factors of the parasite and the immune status of the host (10).
Innate Immune Response against Leishmania and Importance of Phosphorylation Events: A Brief Overview
To survive during the infection of mammalian hosts, Leishmania parasites must quickly adapt to their new and hostile environment. Expression of the stress response protein A2 specifically by Leishmania species causing VL is a good example of such adaptation. Indeed, A2 promotes heat shock resistance and thereby supports parasite survival and visceralization in inner and warmer organs (11). However, Leishmania parasites could not survive in their new host organisms without the development of additional strategies to escape the host defense mechanisms.
Phagocytic cells, such as neutrophils, dermal dendritic cells, and dermal macrophages, are the first immune cells encountered by the promastigotes injected into the skin by sand flies. In addition to being a primary source of antileishmanial effector molecules, they also represent the cellular niche chosen by Leishmania promastigotes to evade the cytotoxic humoral components (such as complement) and/or to differentiate into replicating amastigotes (12–14). Therefore, it is crucial to understand how Leishmania convert these microbicidal phagocytes into safe target cells.
Uptake of pathogens by phagocytes usually results in a proinflammatory innate immune response, which fosters their microbicidal activity and promotes the recruitment and activation of other immune cells. In the case of Leishmania parasites, PMNs are the first line of defense and exert protective effects via release of reactive oxygen species (ROS) and the formation of neutrophil extracellular traps. At the same time Leishmania-infected apoptotic PMNs also function as deactivating vehicles for the transfer of the parasites into macrophages and dendritic cells [reviewed in Refs. (2, 15, 16)]. Subsequently, macrophages and dendritic cells become activated by interferon (IFN)-γ and tumor necrosis factor (TNF) generated by natural killer cells and CD4+ T lymphocytes, which leads to the expression of inducible or type 2 nitric oxide (NO) synthase (iNOS, NOS2) [reviewed in Ref. (17)]. iNOS-derived NO is not only a central antileishmanial effector molecule (18–21) but also paves the ensuing type 1 T helper (Th1) cell response [reviewed in Ref. (22)]. All cellular signaling pathways engaged in this response are tightly controlled and depend on numerous posttranslational protein modifications. Among them, versatile phosphorylation of molecules plays major and ubiquitous functions. It is therefore not a surprise that Leishmania parasites have been described to manipulate such a cardinal modification to attenuate the host immune response.
Phagocytosis is the first action undertaken by macrophages against transmitted Leishmania. However, maturation of the phagosome toward a lytic phagolysosome can be altered by one of the most abundant virulence factors produced by Leishmania promastigotes, the lipophosphoglycan (LPG). This surface molecule was found to target the phagosome membrane where it precluded the recruitment of the acidifying vesicular proton-ATPase (23) and the correct recruitment of protein kinase C (PKC) α (24). Other PKC isoforms, like PKCβ and PKCδ, also showed a disturbed activation during Leishmania infections (25–27). Like for PKCα, LPG impaired the proper activation of PKCβ resulting in an inhibition of ROS production (28). This important leishmanicidal mechanism of macrophages was also described as a target of another, intensely studied Leishmania virulence factor, the metalloprotease GP63 (also termed leishmanolysin or Leishmania major surface protease). The activity of PKC was attenuated by the proteolytic effect of GP63 leading to a diminished release of ROS (29). In this context, the role of mitogen-activated protein kinases (MAPKs), including p38, Jun N-terminal kinase (JNK), and extracellular signal-related kinases (ERK1/2), has been investigated by several groups since they represent major players of the immune pathways present in myeloid cells. In early studies, an absence of MAPK activation during Leishmania infection was noted, which was a plausible explanation for the observed hypo-responsiveness of macrophages (30, 31). The ability of Leishmania to hinder ERK and p38 phosphorylation was linked to the inability of IFN-γ to induce TNF production in these cells (32). Likewise, the suppression of another proinflammatory cytokine, IL-12, by L. major might result from the inhibition of the PKCδ kinase. Mice deficient for PKCδ kinase were more sensitive to L. major infection than wild-type mice because of a reduced IL-12p40 and IL-12p70 release by macrophages and dendritic cells and an ensuing Th2-like immune response (33).
Protein kinases are not the only targets of Leishmania during infection. Inhibition of IL-12 production by activation of the phosphoinositide 3-kinase (PI3K) during Leishmania infection has been convincingly demonstrated using PI3K-deficient mice. These mice developed an improved Th1 response protecting them against L. major infection (34). Similarly, activation of the protein kinase RNA-activated (PKR) was important for the intracellular growth of L. amazonensis as it promoted the expression of anti-inflammatory cytokine IL-10 and subsequent deactivation of macrophages (35). Interestingly, PKR activation was not observed for all Leishmania species. In the case of L. major-infected macrophages, PKR activation was actively suppressed by the serine peptidase inhibitor ISP2 of the parasite; activation of PKR by exogenous poly I:C resulted in rapid parasite death due to the release of proinflammatory cytokines (36). Nevertheless, the disease-promoting role of IL-10 in both cutaneous and visceral leishmaniasis has been clearly documented (37–41). Similar to IL-12, its regulation depends on diverse pathways controlled by reversible phosphorylation events. PI3K and the serine/threonine-specific protein kinase AKT (also known as protein kinase B) were shown to be activated during macrophages infection by L. donovani. These kinase activities resulted in the inactivation of the glycogen synthase kinase-3β leading to the binding of an activating transcription factor, the cyclic AMP-responsive element-binding protein, to the IL-10 promoter (42).
Not only the production but also the response to proinflammatory cytokines is altered during Leishmania infections. JAK2, a tyrosine kinase binding to the receptor of IFN-γ, the key cytokine of protective Th1 cells, was deactivated by a host phosphatase following Leishmania infection (43, 44).
Altogether, these few examples illustrate the delicate balance between tightly controlled phosphorylation events in the host cells, which are necessary for initiating pathogen control mechanisms, and the ability of the parasite to subvert these reactions. Biochemically, the host-pathogen interaction is governed by kinases (which catalyze the phosphorylation) and by phosphatases, which hydrolyze phosphoester bounds. In the section above, we have briefly mentioned important host kinases involved in the interplay between infected cells and Leishmania parasites. In the following part of this review, the role of the phosphatases will be presented. We will focus not only on host phosphatases and how they are modulated by the parasite (e.g., by parasite proteases) but also on Leishmania phosphatases and their putative interference with immune cells functions (Figure 1).
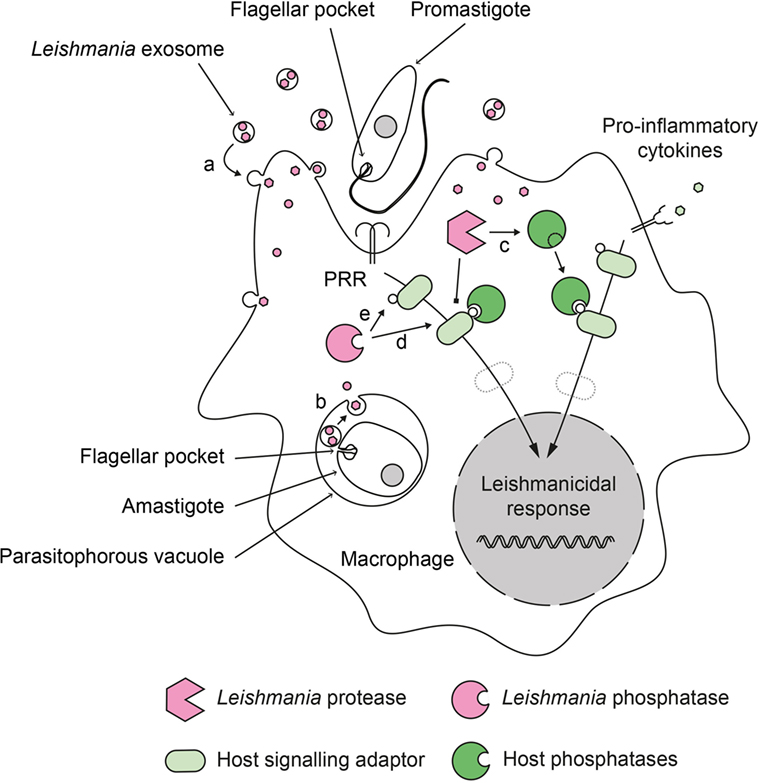
Figure 1. Role of phosphatases in the deactivation of host cell during Leishmania infection. Leishmania parasites have developed a dual strategy to inactivate important signaling pathways in their host cell. During infection, promastigotes and amastigotes secrete exosomes containing virulence factors. These exosomes release their content into the infected cells after fusing with (a) cytoplasmic membranes (in the case of extracellular promastigotes) or (b) phagosomal membranes (in the case of intracellular amastigotes). Secreted Leishmania proteases can proteolytically activate host phosphatases (c) that subsequently down regulate activating cellular pathways. Secreted Leishmania phosphatases could synergize with host phosphatases (d) or act independently (e) to modulate the infected cell response.
Host Phosphatases
Protein phosphatases can be classified into two main families of enzymes depending on substrate specificity and catalytic signature. The serine threonine phosphatase (STP) family comprises three main groups: the phosphoprotein phosphatases (PPPs), the metal-dependent protein phosphatases (PPMs), and the aspartate-based phosphatases (F cell production phosphatase [FCP]/small carboxy-terminal domain phosphatase [SCP]) (45). The second family of phosphatases contains enzymes that are able to dephosphorylate phosphotyrosine residues (phosphotyrosine phosphatases [PTPs]), which are also categorized in four different groups depending on their modular organization and their substrate restriction (46) (see Figure 2).
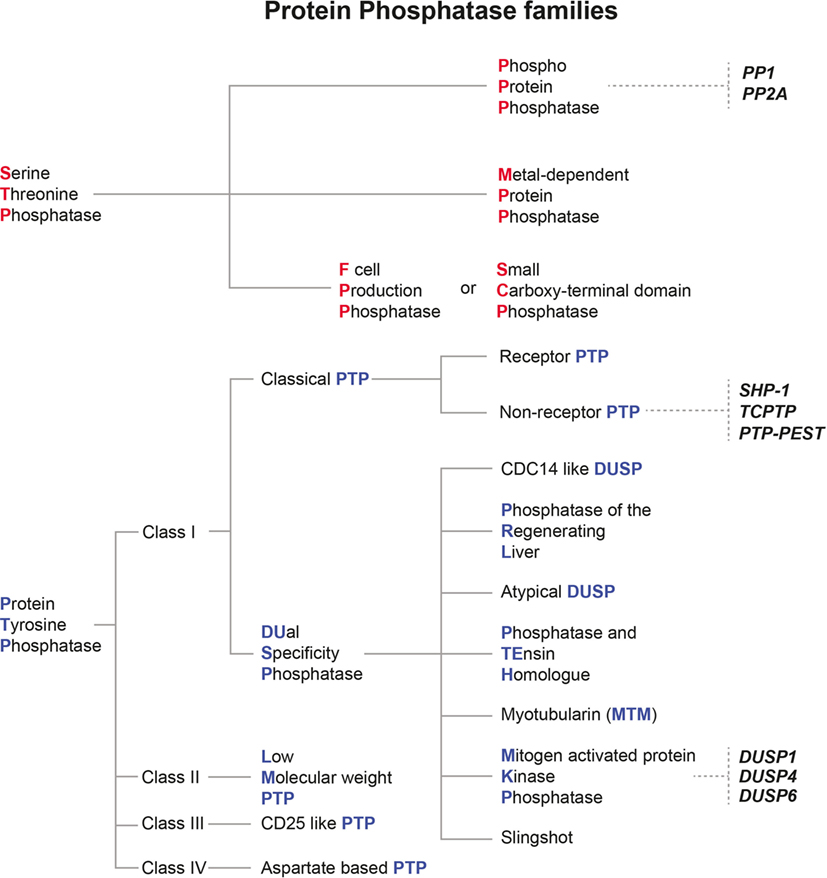
Figure 2. Classification of mammalian protein phosphatase families and their relevance for Leishmania infection. Protein phosphatases are classified depending on their structural features and their substrate specificity. The two main families of protein phosphatases and their respective subfamilies are depicted here. Serine threonine phosphatases are marked with red initials, whereas protein tyrosine phosphatases are given with blue initials. Host protein phosphatases which were already shown to affect the outcome of Leishmania infections are written in bold and italic black letters.
Serine-Threonine Host Phosphatases Regulating the Outcome of Leishmania Infections
Immune cells responding to Leishmania infection rely on efficient signaling pathways to stimulate the production of leishmanicidal molecules or to initiate and support their migration, proliferation, and cytokine production. In general, these pathways are finely balanced between an activating signal transmitted by protein kinases and a stopping signal mediated by phosphatases. From the parasite’s perspective, this balance needs to be shifted toward the phosphatase side to guarantee intracellular survival in host cells. Activation of host phosphatases following Leishmania infection has been described in macrophages (43) and dendritic cells (47) and helps to explain the reduced immune response observed during visceral leishmaniasis. In mice infected with L. donovani, lymphocytes were unresponsive to strong activating and proliferative stimuli like phorbol 12-myristate 13-acetate (PMA) and ionomycin. This defect could be reversed when PKC and ERK phosphorylation were restored by treating the lymphocytes with okadaic acid, an STP inhibitor specific for the heterotrimeric STP protein phosphatase 2A (PP2A) (48, 49). In L. donovani-infected macrophages activation of PP2A was triggered by host production of ceramide after parasite uptake which resulted in the dephosphorylation of AKT and the subsequent decrease of TNF secretion (50). In striking contrast to these findings are observations made with saliva from the sand fly vector Phlebotomus papatasi, which contains a non-proteinaceous inhibitor of the STPs protein phosphatase 1 (PP1) and PP2A. As PP1 and PP2A are required for the induction of the iNOS gene (51), the inhibitor present in the sand fly saliva impeded the production of NO by macrophages (52). Thus, both activation and inhibition of PP1 and PP2A can impair the defense against Leishmania parasites.
The mitochondrial phosphatase, phosphoglycerate mutase 5 (PGAM5), which was recently described as an atypical, histidine-based STP (53), is another example for a host-protective phosphatase. PGAM5, which acts downstream of receptor-interacting serine/threonine-protein kinase 1 (RIPK1), inhibited the intracellular replication of Leishmania (L. infantum, L. major, L. amazonensis) in macrophages, presumably via induction of IL-1β and NO release. Mice deficient for PGAM5 showed a slight, but significant increase of the parasite load in the skin lesions of L. amazonensis-infected mice (54).
Phosphotyrosine Host Phosphatases Regulating the Outcome of Leishmania Infections
A first hint that replication and survival of Leishmania in phagocytes is dependent on this family of phosphatases came from the use of inhibitors. In vitro, macrophages treated with a PTP inhibitor, the peroxovanadium compound bpV(phen), showed an improved control of L. donovani infection, mediated by an increased production of NO (55). Inhibition of ERK2 by a still unknown phosphatase was suggested to account for a similar phenotype seen in a L. major-infected human macrophage-like cell line (56). Treatment of macrophages with a related PTP inhibitor, sodium orthovanadate, enhanced the phagocytosis of Leishmania promastigotes and thereby allowed a safe entry of the parasite into its cellular niche (57, 58). These in vitro results were corroborated by in vivo experiments with L. major-infected BALB/c mice, in which the PTP inhibitors bpV(phen) and pbV(pic) led to partial parasite control via induction of iNOS activity (59). Thus, the activities of PTPs seemed to support intracellular parasite growth.
Dual-Specificity Phosphatases (DUSPs)
The therapeutic effect of PTP inhibitor treatments was in line with observations made during in vivo treatment of L. donovani-infected mice with okadaic acid, a STP PP2A inhibitor (see above). The comparative analysis of both inhibitors in macrophages revealed that PP2A synergistically inhibited the activity of the MAPK ERK1/2 together with a phosphatase belonging to the PTP family, the DUSP6, also known as MAP kinase phosphatase (MKP) 3 (60). The inhibition of MAPKs downstream of PKCζ following Leishmania infection led to a decreased iNOS expression (60). In the same study, the role of DUSP1, also termed MKP1, was analyzed. Activation of DUSP1 downstream of PKCε resulted in the inhibition of p38 MAPK (60). A host-damaging role for these two DUSPs during visceral leishmaniasis was also demonstrated in L. donovani-infected BALB/c mice treated with 18β-glycyrrhetinic acid (GRA), a compound derived from the medicinal plant licorice (Glycyrrhizza glabra). Treatment with GRA caused a shift of the immune response from a detrimental Th2 response to a protective Th1 response (61). At the molecular level, the shift was explained by a decreased DUSP1 and DUSP6 expression and the concomitant activation of mitogen- and stress-activated protein kinase 1 (MSK1), which ultimately led to the production of leishmanicidal NO (62).
Findings with L. major-infected macrophages, however, indicated that the activity of DUSP6 is not always detrimental for the outcome of Leishmania infections. In L. major-infected macrophages activated by anti-CD40, siRNA-mediated inhibition of DUSP6 led to ERK activation, enhanced production of IL-10, and reduced expression of IL-12 and iNOS, whereas inhibition of DUSP1 caused p38 activation and increased generation of IL-12 and iNOS. Consequently, in vivo overexpression of DUSP6 or pharmacological inhibition of DUSP1 both ameliorated the course a cutaneous L. major infection (63). This functional dichotomy between DUSP6 and DUSP1 has also been reported in the context of macrophage coinfections with the immunomodulatory bacterium Mycobacterium indicus pranii and L. donovani. The protective effect of this bacterial infection relied on a toll-like receptor (TLR) 4-dependent increase of DUSP6 and simultaneous decrease of DUSP1. Whereas DUSP1 inhibition led to the activation of p38, the production of NO and the release of IL-12, the activation of DUSP6 blocked the ERK1/2 pathway and the expression of IL-10 and arginase-1 (Arg1). Overall, M. indicus pranii conferred protection against visceral leishmaniasis by reciprocal regulation of DUSP1 and DUSP6 and a shift toward a Th1 response (64).
A phenotype similar to the one of DUSP6 was also observed for DUSP4 (syn. MKP2). Mice deficient for the dusp4 gene were more susceptible to a cutaneous infection with L. mexicana than wild type mice. This was due to an enhanced JNK and p38 phosphorylation in macrophages that increased the expression of Arg1 and was associated with a disease-promoting Th2 immune response (65, 66).
Src Homology Region 2 Domain-Containing Phosphatase-1 (SHP-1)
In macrophages infected with L. donovani, a PTP activity identified as Src homology region 2 domain-containing phosphatase-1 (SHP-1; also known as protein tyrosine phosphatase non-receptor type 6 [PTPN6]) was rapidly activated and induced hypo-responsiveness to IFN-γ by directly interacting with the kinase JAK2 recruited to the IFN-γ receptor (31, 43, 44). The confirmation that SHP-1 played a key role in the pathogenicity of leishmaniasis came from infection experiments with viable motheaten (mev) mice that are deficient for this phosphatase (67). Mev mice were largely protected against L. major infection, most likely because of an increased NO production by their phagocytes (68) and an enhanced secretion of proinflammatory cytokines like TNF, IL-1β, and IL-6 (69). Another group, however, did not observe an impact of the SHP-1 deficiency in mev mice on the course of L. major-infection and questioned a selective role of SHP-1 in macrophages as SHP-1 is also regulating T and B cell responses (70). It is also important to point out that even in the absence of SHP-1 activity different species of Leishmania (L. major, L. braziliensis, L. mexicana, and L. donovani) caused degradation and reduced nuclear translocation of STAT1 in primary mouse macrophages (71). This finding indicates that at least part of the suppression of the JAK2/STAT1 pathway in Leishmania-infected macrophages and the subsequent reduced release of cytokines and NO in response to IFN-γ is SHP-1 independent.
Infection of SHP-1-deficient macrophages also revealed the importance of this phosphatase for the downregulation of MAPK activity after IFN-γ (31, 44). Control of MAPK by SHP-1 was proposed to be partially responsible for the inhibition of iNOS during IFN-γ stimulation (44, 72). However, involvement of individual MAPK seemed to differ depending on the stimuli used to trigger iNOS expression during Leishmania infection. Although SHP-1 negatively regulated ERK1/2 and JNK after IFN-γ stimulation (72), this phosphatase boosted the activity of ERK1/2 after CD40 stimulation, which promoted the secretion of IL-10 and simultaneously inhibited p38 MAPK activation (73).
During Leishmania infection, SHP-1 did not only alter the macrophage responses to proinflammatory stimuli originating from T cell such as IFN-γ and CD40 ligand. Signaling pathways triggered by pathogen recognition receptors (PRRs), e.g., TLR4, were also disturbed. In mouse bone marrow-derived macrophages, infection with different Leishmania species (L. major, L. mexicana, L. donovani) caused rapid activation of SHP-1, which bound to a kinase tyrosyl-based inhibitory motif (KTIM) of the interleukin 1 receptor-associated kinase 1 (IRAK-1) and thereby blocked the intrinsic kinase activity of IRAK-1. The inactivation of IRAK-1 by SHP-1 was associated with the inability of IRAK-1 to detach from the TLR4 adaptor MyD88 and to attach to the downstream signal transducer TRAF6 so that the LPS-induced macrophage responses (e.g., release of NO and TNF) were severely impaired (74). According to the authors of this study KTIMs are also present in other targets of SHP-1, such as ERK1/2, p38, and JNK, and therefore offer a molecular explanation for the multiple effects of SHP-1 (74). A SHP-1 mediated hypo-responsiveness to LPS was also observed in human macrophages infected by L. donovani (75).
Two recent studies demonstrated that SHP-1 also modulates the signaling downstream of PRRs of the lectin receptor family during leishmaniasis. C-type lectin receptor Mincle expressed by dendritic cells recognized a still unknown ligand present on the surface of L. major. Ligation of the Mincle receptor by L. major impaired the activation of dendritic cells due to recruitment of SHP-1, which converted the canonical immunoreceptor tyrosine-based activation motif (ITAM) of the FcRγ-chain signaling component of the receptor into an inhibitory motif (ITAMi). In accordance with these in vitro findings Mincle-deficient mice developed less severe skin lesion after infection with L. major (76). Likewise, I-type lectin receptors Siglec-1 and -5 expressed by macrophages were described to recognize sialylated ligands on the surface of L. donovani parasites. Engagement of Siglec-1 facilitated the uptake of the parasites by the macrophages, whereas ligation of Siglec-5 led to a reduced production of leishmanicidal ROS and NO accompanied by a shift toward a Th2 response. The latter resulted from the recruitment of SHP-1 to the Siglec-5 receptor promoting here again the deactivation of the immune cell (77).
Together, these data illustrate that phosphatases are critical regulators of the immune response against Leishmania parasites. Parasite-driven activation of PTPs, notably SHP-1, clearly impairs the effector function of macrophages [reviewed in Ref. (78)]. On the other hand, host cells have developed strategies to counteract the evasion mechanisms of Leishmania parasites. As an example, natural resistance-associated macrophage protein 1 (NRAMP-1) inhibited the function of PTPs, either via a direct interaction between PTPs and the metal cation (Fe2+) transported by NRAMP-1 or by oxidation of the phosphatases resulting from NRAMP-1/iron-dependent ROS-formation (79). However, in the arm race between host and parasite, Leishmania parasites were shown to again antagonize this ROS-mediated inhibition of host PTPs in macrophages by inducing the expression of the uncoupling protein 2 (UCP2), a negative regulator of mitochondrial ROS generation. This led to lower ROS production resulting in an enhanced host PTP activity (80).
Evidence for the Activation of Host Phosphatases by Leishmania Virulence Factors
As summarized above, infection of macrophages with Leishmania leads to the induction of phosphatase activities, especially SHP-1, which help the parasite to create a safe niche for its replication. Phosphatase activation can be the outcome of normal cell signaling pathways triggered by cytokines and/or PRRs. Alternatively it is possible that microbial virulence factors released inside the host cell directly stimulate phosphatases such as SHP-1. The first evidence that Leishmania could do so came from pull-down assays with L. donovani extracts and recombinant SHP-1. In these experiments, the Leishmania elongation factor 1α (EF-1α) was identified as an interactor of the host phosphatase. Leishmania EF-1α secreted by intraphago(lyso)somal parasites reached the cytosol of the infected macrophages. Purified Leishmania EF1α directly bound to SHP-1 and induced its activity (81), whereas mammalian EF-1α was unable to do so, presumably because of an additional hairpin loop at its surface (82). The secretion of EF-1α by intracellular Leishmania amastigotes during macrophage infection was later confirmed and attributed to the exosomes released by the parasites (83).
Production of exosomes by Leishmania appears to be the main secretory pathway used by the parasite to export its virulence factors inside and outside the host cells (83, 84). The important contribution of exosomes to the pathogenicity of Leishmania was supported (a) by their increased release after a temperature shift mimicking the transit from the sand fly vector (26°C) to the human host (37°C) (85) and (b) by their immunomodulatory effects on innate immune cells (86, 87).
In addition to EF-1α, other proteins contained in Leishmania exosomes (83) were found to activate SHP-1. The fructose-1,6-biphosphate aldolase was also secreted by L. donovani during macrophage infection and could directly interact and activate in vitro the phosphatase activity of SHP-1 (88). Unfortunately, the molecular mechanism of SHP-1 activation by both virulence factors, EF-1α and the aldolase, is still unknown.
One of the best studied virulence factors of Leishmania is the zinc metalloprotease GP63. This multifunctional virulence factor is the most abundant C-terminal glycosyl-phosphatidyl-inositol-anchored protein associated with the cell surface of Leishmania promastigotes, but is found in limited quantities also inside the parasite (89). On the surface of Leishmania parasites, GP63 increased the resistance of promastigotes to complement-mediated lysis by rapidly converting C3b into the inactive complement fragment iC3b (90). iC3b opsonized the parasite and facilitated its silent phagocytosis via the CR3 receptor (CD11b/CD18), which synergized with the fibronectin receptor that directly binds GP63 (91). Importantly, GP63, like EF-1α and the aldolase, was also secreted by promastigotes inside exosomes (83, 92). In the cytosol of infected cells, multiple targets of GP63 protease activity have been identified. These include (a) the cytoskeleton adaptors p130Cas (93), cortactin (93) and MARCKS-related protein (94), all of which are degraded by GP63; (b) the translational regulator mTOR (95), which is cleaved by GP63 leading to repression of macrophage translation; (c) PKC, which is also cleaved by GP63 leading to the attenuation of PKC activity (29); (d) transcription factors c-Jun (96) and NF-κB p65/RelA fragment (97), which are inactivated or activated by GP63-mediated proteolysis, respectively; and (e) the host phosphatases SHP-1, PTP1B and TCPTP which are proteolytically activated by GP63 (98). As already discussed before, SHP-1 is a key regulator of many different signaling pathways, including the TLR (74, 75), lectin receptor (76, 77), the MAPK (72, 73, 93) and the IFN-γ-triggered JAK/STAT pathways (44, 68) (Figure 3). It is worth noting that SHP-1 was not the only host phosphatase targeted by GP63 and shown to modulate the activity of JAK2. PTP1B was also proteolytically activated by GP63. Interestingly, whereas under resting conditions both SHP-1 and PTP1B were bound as full-length enzymes to JAK2, in L. major-infected macrophages the GP63-activated fragment of PTP1B and the uncleaved (i.e., inactive) SHP-1 prevailed, indicating that PTP1B might have a predominant role compared to SHP-1 in the deactivation of JAK2 (98). The importance of PTP1B activation for parasite survival was further underlined by the increased resistance to L. major seen in PTP1B-deficient mice (98).
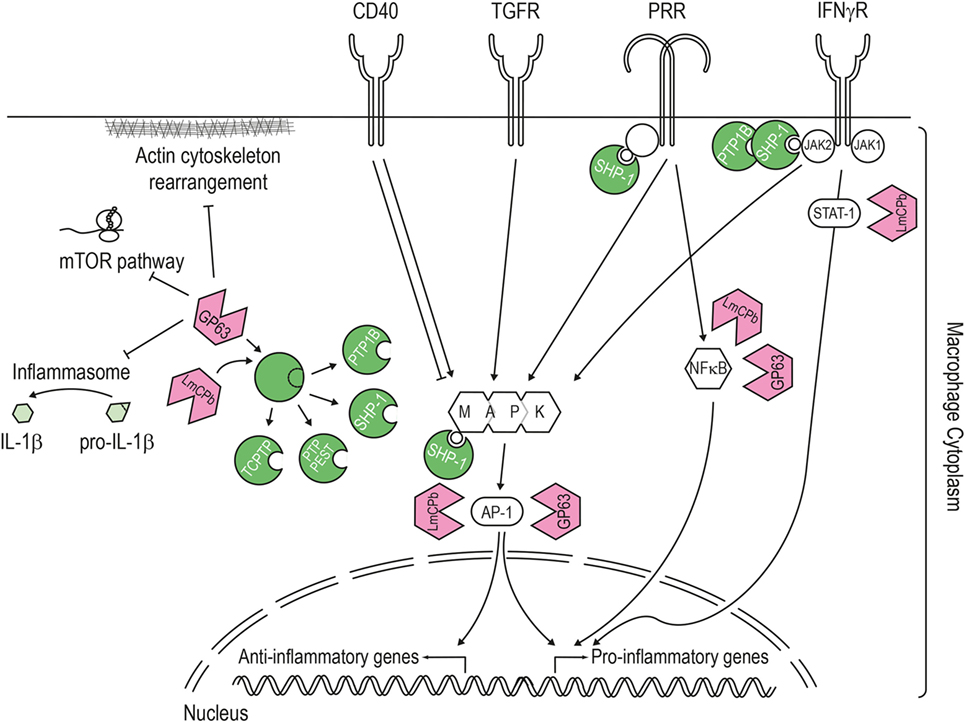
Figure 3. Pathways targeted by the Leishmania virulence factors. LmCPb and GP63 proteases are Leishmania virulence factors secreted via the exosome route and found inside the host cell cytoplasm where they can alter numerous signaling pathways. The best studied Leishmania protease is GP63, which proteolytically cleaves and activates host phosphatases (such as PTP1B, PTP-PEST, TCPTP, and SHP-1) or the NF-κB subunit p65/RelA. Once activated, SHP-1 downregulates the macrophage response to interferon-γ by interacting with the kinase JAK2, modulates the pathways induced by pathogen recognition receptors (e.g., toll-like and lectin receptor) and also targets signaling kinases such as mitogen-activated protein kinase. In addition, Leishmania proteases contribute to this general downregulation of the host cell response by degrading proteins of the actin cytoskeleton, mTOR (leading to repression of host cell translation), transcription factors (like STAT1 or AP-1), and components of the NLPR3 inflammasome (thereby blocking the generation of IL-1β).
Two further host phosphatases have been described to be proteolytically activated by GP63 during Leishmania infection: TCPTP (92, 93, 98) and PTP-PEST (93). However, their functional relevance for the infection has not been tested so far. Moreover, it seemed that the proteolytic activation of these phosphatases by GP63 was species-specific. GP63-mediated proteolysis was only observed after infection with L. major, L. donovani, and L. mexicana, but not with L. tarentolae and L. braziliensis (93).
Another protease, the cysteine proteinase b of L. mexicana (LmCPb) was proposed to synergize with GP63 to cleave PTP1B. This protease seemed to also participate in the degradation of important transcription factors such as NF-κB, AP-1, and STAT-1 (99). Intriguingly, PTP1B activation only occurred during infection of macrophages by promastigotes, but not by amastigotes (99), unlike SHP-1 that is cleaved after infection with either of both parasite stages, and despite the higher expression of LmCPB in amastigote (100). The authors suggested that amastigotes did not need to target PTP1B, whose regulatory functions overlap with SHP-1, because amastigotes were already more adapted to their niche than promastigotes.
Leishmania Phosphatases
The data summarized in the preceding section illustrated that activation of host phosphatases by Leishmania is essential for their infection of mammalian organisms. However, Leishmania phosphatases might be equally relevant for the development of the parasite and its survival during the infection process. In the following, we will review the Leishmania protein phosphatases already characterized in the context of the parasite life cycle. Next, the role of extracellular acid phosphatases (AcPs) of Leishmania will be discussed and compared to a newly described secreted Leishmania phosphatase which appears to contribute to the virulence of the parasite.
Phosphatases Involved in the Life Cycle of Leishmania Parasites
Recent technical advances in mass spectrometry allowed systematic analysis of protein phosphorylation in multiple biological contexts. In case of Leishmania, studies have initially focused on the differentiation of promastigotes into amastigotes, especially because only a few genes are differentially regulated during this adaptive process, making posttranslational modification a good candidate to regulate this transformation (101). Axenic differentiation of L. donovani promastigotes into amastigotes revealed the presence of 627 proteins phosphorylated on 1,614 residues with a majority modified on serine (80%) and threonine (19.4%) and only a few on tyrosine (0.4%). Among them, only 27% of the modifications were present in both developmental stages, whereas 35 and 38% of the phosphorylation were restricted to promastigotes or amastigotes, respectively (102). A refined analysis of the kinetic of differentiation suggested that promastigotes sensed an environmental signal triggering a phosphorylation pathway and resulting in Leishmania transformation (103). Like for the stress response protein A2 expressed by Leishmania species causing VL (11), gene ontology analysis of the phosphoproteome of Leishmania pinpointed the critical role of the heat shock response during differentiation (104). Obviously, parasites need to adapt to the temperature increase experienced during the inoculation into the skin. Indeed, experiments using parasites deficient for heat shock protein (Hsp)70/Hsp90-organizing protein (Hop) (also termed stress-inducible protein 1), a protein belonging to a chaperone complex, proved the importance of phosphorylation in the regulation of the heat shock response (105).
The fine tuning of all these adaptive pathways as well as the metabolic equilibrium of Leishmania parasites requires a balanced activation of phosphatases and kinases. Bioinformatic analyses identified 199 protein kinases (106) and 88 protein phosphatases (107) in the genome of the L. major species Friedlin. Among the kinases, a few have been identified and functionally characterized. Mainly, the role of MAPK was described in different pathways responsible for sensing environmental changes and ranged from flagellar control (108) to arginine deprivation (109). Regarding protein phosphatases, their characterization is still patchy as we will see in the following paragraphs (Tables 1 and 2) [reviewed in Refs. (107, 110)].
Leishmania Protein Serine/Threonine Phosphatases
Leishmania major, the model species used for this review, encodes 58 genes for serine/threonine phosphatases (STP) which are members of the three different groups of enzymes within this family: the PPP group (30 genes), the PPMs (15 genes), and the aspartate-based phosphatases (FCP/SCP; 13 genes) (see Table 1). These enzymes are highly conserved in the different Leishmania species (Table S1 in Supplementary Material).
Within the PPP-group, the eight Leishmania phosphatases that belong to the PP1 subgroup have not yet been biochemically and/or functionally characterized. Surprisingly, five of them are encoded by genes organized in tandem on chromosome 34. This organization suggests a common regulation of the expression of the different isoforms. PP2A phosphatases are also not yet fully characterized, but their increased expression has been linked to resistance against antibiotics such as paromomycin (111). Additional information on their functions in Leishmania parasites could be derived from studies of orthologs in other kinetoplastid. PP1 and PP2A phosphatases of Trypanosoma parasites participate in amastigote transformation (110) and, interestingly, share the highest homology with Leishmania orthologs (Table S1 in Supplementary Material).
Regarding the calcineurin-like phosphatase subgroup (PP2B), a calcium- and calmodulin-dependent phosphatase activity was purified from L. donovani parasites but their respective genes have not yet been sequenced (124). In L. major two genes, LmjF26.2530 and LmjF36.1980, might account for this activity (Table 1) as they encode proteins carrying a calcineurin A catalytic domain and the three canonical regulatory domains: the calcineurin B (CnB) binding domain, the calmodulin (CaM) binding domain and an autoinhibitory domain (possibly missing in LmjF36.1980) (125). Noteworthy, the ortholog found in T. cruzi does not contain the last two domains which likely causes a substantial functional difference (125). Interestingly, Leishmania CnB (LmjF.21.1630), the regulatory subunit of calcineurin A, was shown to control the temperature adaptation of L. major. Promastigotes deficient for CnB failed to differentiate into amastigotes and were completely avirulent in macrophages and during in vivo infection (126).
The PP7-like phosphatase, encoded by the gene LmjF.12.0660, showed a strong homology to protein phosphatases with a E-helix/F-helix (EF)-hand (PPEF) that were identified in sensory cells of higher eukaryotes. However, LmPPEF did not bind calcium because its EF-hand domains appeared degenerated and the functionality of its N-terminal calmodulin binding domain was not ascertained (113). Due to a N-myristoylation modification, LmPPEF appears to be membrane-bound and localized in the vicinity of the kinetoplast and the flagellar pocket (113), an active secretory site, which might explain its detection in the exosome content of promastigotes (83, 92).
PP5 phosphatases showed some similarities with PP7 proteins, but instead of calcium-dependent regulatory domains they carry N-terminal tricopeptide repeat (TPR) domains, which can mediate protein–protein interaction and autoinhibition. The PP5 phosphatase encoded by L. major (LmjF18.0150) has been biochemically characterized. Mutation analysis of the recombinant protein confirmed its phosphatase activity and the autoinhibitory role of the TPR domains (112). Functional data on this protein are scarce but suggested its involvement in stress response as also seen with the T. brucei homolog (110). Expression of the unique PP5 gene in L. infantum correlated with increased resistance to antimony (114).
Regarding the eukaryotic-like PP6 phosphatases or the eukaryotic phosphatases homologous to the bacterial enzyme diadenosine tetraphosphate hydrolase ApaH (ApaH-like phosphatases [ALPHs]), only few data exist on their biochemical or physiological role. In Trypanosoma brucei, ALPH1 was shown to have all features of an mRNA decapping enzyme (127).
The last subgroup of the PPPs comprises kinetoplastid-specific proteins (kPPP) that carry a mutation in their catalytic site. These pseudo-phosphatases (kSTP pseudo) show some similarities to plant and fungal PPPs. They likely add an extra level of regulation to the kinase/phosphatase activities by stably binding to phosphorylated substrates and shielding them against other phosphatase activities (110). These adaptations to numerous substrates combined with the absent catalytic activity might explain the higher divergence observed between kPPP from Leishmania and Trypanosoma (Table S1 in Supplementary Material). Expression of such pseudo-enzyme, encoded by the gene LinJ.34.2610, has been negatively correlated with the resistance of L. infantum to antimony treatment (115).
Regarding the 15 phosphatases of the PPM (or PP2C) group, their biological functions have not yet been unraveled, but their dependency on divalent manganese or magnesium cations has been validated in L. donovani and L. infantum/L. chagasi promastigotes (116, 128). In case of L. infantum/chagasi, a 42 kDa PP2C-like phosphatase named LcPP2C, encoded by the gene LinJ.25.0780, was biochemically characterized in promastigotes and amastigotes (116). Interestingly, the ortholog of this protein was secreted by L. mexicana along with 71 other proteins detected (84, 85). However, whether this enzyme contributes to the modulation of the host cell signaling (e.g., via activation of the host phosphatases SHP-1 and PTP-1B) by the L. mexicana secretome, was not investigated (85). Other PP2C-like proteins have been detected in L. mexicana promastigotes but they still lack functional characterization (129). Moreover, expression of two additional PP2C genes, LinJ.34.2310 and LinJ.34.2320, was recently linked to methotrexate resistance of L. infantum (114).
Altogether, only 6 of the 58 STP genes have been partly characterized despite the suggested crucial role in amastigote transformation (Table 1). More research on this phosphatase family could therefore open new avenues toward the development of alternative therapeutic approaches.
Leishmania Protein Tyrosine Phosphatases
In additions to STPs, 31 putative protein tyrosine phosphatases (PTPs) are encoded in the L. major genome (Table 2). All of them share a common catalytic site surrounded by a cysteine and an arginine (CX5R). They are well conserved among Leishmania species and are divided into four classes (Table S1 in Supplementary Material). Class I PTPs, which form the largest group, contain enzymes with either proven PTP activity or a structural organization characteristic for PTPs, whereas classes II and III, represented by a single member each, might not convey such activities. Class IV enzymes were not detected in the genome of Leishmania parasites.
Proteins of the class II PTPs are homologous to low-molecular-weight (LMW) PTPs found in human or bacteria. However, the protein encoded by the gene LmjF.01.0200 carries a mutated catalytic cysteine as revealed during our own sequence analyses, suggesting that it is probably inactive and might therefore act, similar to the kPPPs, as substrate trapping molecule. Another degenerated LMW PTP-like enzyme is encoded by the gene LTRL590_050007600.1, whose expression correlated to antimony resistance in L. tropica field isolates (130).
Regarding the class III PTPs, the enzyme named LmACR2 has been initially characterized in L. major as an arsenate (As)/antimony (Sb) reductase despite containing a canonical PTP catalytic site. The antimony reductase activity of LmACR2 converted Sb(V), present in Sb-containing antileishmanial drugs (e.g., sodium stibogluconate), into Sb(III) which is the active microbicidal molecule. Overexpression of LmACR2 in promastigotes supported this observation as parasites became more sensitive to antimonials (120). However, metalloids like Sb are not commonly encountered by Leishmania parasites in nature. Therefore, the evolutionary pressure to develop such activity should have been low. Thus, the concomitant PTP activity of LmACR2 detected in vitro might reflect the true physiological function of this enzyme (121, 122).
Most of the PTPs expressed in Leishmania parasites belong to the class I PTPs that can be further divided into two subgroups of enzymes. The first subgroup consists of classical PTPs homologous to human PTP1B. However, in Leishmania and other kinetoplastid parasites, this group does not contain any receptor-PTP usually found in higher eukaryotes. Among the three non-receptor-PTPs encoded by Leishmania parasites, only the eukaryotic-like LdPTP1 was functionally characterized. The deletion of the gene encoding for this enzyme, LdBPK_365610.1, resulted in complete loss of amastigote transformation during infection of macrophages with L. donovani promastigotes and in a dramatic decrease of the parasite virulence in vivo (117). The molecular mechanism underlying this drastic phenotype has not yet been elucidated. However, the study of the TbPTP1 ortholog in Trypanosoma brucei revealed that this family of phosphatase could control the integration of environmental differentiation signal (131, 132).
The second subgroup of class I PTPs comprises dual specificity phosphatases (DUSP) (Table 2). These phosphatases are characterized by a substrate spectrum that extends beyond phosphotyrosine residue (e.g., dephosphorylation of serine and threonine residues). Some of these DUSPs are even described as lipid phosphatases:
(a) Myotubularins (MTMs) were shown to target phosphoinositides like PI(3)P or PI(3,5)P2 in mammals, and therefore control endocytosis and membrane trafficking (133). In Leishmania parasites, the MTM group consists of only two genes (instead of the 16 encoded by the human genome making it the largest group of human DUSP). Leishmania MTMs are characterized by their very large size with up to 3,245 amino acid (as in the case of LmjF.12.0320), which is due to long N-terminal extensions. However, their function has not been yet studied in trypanosomatids.
(b) Two Leishmania genes encode still uncharacterized phosphatase and tensin homologs (PTEN) that are well known to target PI(3,4,5,)P3 in mammals. Whereas one gene is homologous to kinetoplastid PTENs, the other one shares homology with eukaryotic phosphoinositide phosphatases. Interestingly, the latter subgroup is not represented in all Trypanosomatidae, as it is missing in T. brucei (Table S1 in Supplementary Material).
(c) A group of four Leishmania DUSPs encodes lipid-like phosphatases that have similarities with bacterial virulence factors. LmjF.22.0250 was identified as a close homolog of the secreted PIP phosphatases MptpB from Mycobacterium tuberculosis (119) and LipA from Listeria monocytogenes (134). Strikingly, LmjF.22.0250 is the only protein phosphatase gene that is not shared by all Leishmania species or strains as it was absent from the genome of L. panamensis MHOM/COL/81/L13 and L. braziliensis MHOM/BR/75/M2903, but present in L. braziliensis MHOM/BR/75/M2904 (Table S1 in Supplementary Material). As this phosphatase was not found to be secreted, the absence of LmjF.220250 might be compensated by the other three members of this group of lipid-like phosphatases encoded in the genome of Leishmania. Nonetheless, lipid phosphatases might contribute to the parasite virulence, because one of them, LbrM.25.21.80 was among the 100 genes most abundantly expressed by L. braziliensis in skin lesions of humans with CL (135).
In addition to these phospholipid phosphatases, DUSPs dephosphorylating proteins on tyrosine, serine or threonine residues were also identified in the Leishmania genomes:
(a) Three Leishmania genes encode eukaryotic-like DUSPs (eDUSPs). These include a homolog of the human CDC14 phosphatase that was described to control mitosis by targeting cyclin dependent kinase (136). However, this crucial function has not been yet documented in trypanosomatids. The two genes homologous to phosphatase of the regenerating liver (PRL) will be discussed later in the context of their importance for the virulence of L. major.
(b) In addition to the lipid-like phosphatases discussed above, the group of atypical DUSPs encodes for (i) a kinetoplastid MAP kinase-like phosphatase (MKP) lacking a functional rhodanese homology domain, (ii) a leucine-rich repeat (LRR) DUSP, (iii) a kinatase having LRR domains and a pseudokinase domain, and (iv) an inactive STYX pseudo-phosphatase. None of these enzymes has yet been characterized in Trypanosomatidae.
(c) The remaining Leishmania DUSPs belong to the group of still uncharacterized kinetoplastid-specific DUSPs.
Although the large majority of the Leishmania DUSPs have so far not been functionally studied in Leishmania, it is interesting to note that two of them (LmjF.16.0230 and LmjF34.2190) were secreted in exosomes by Leishmania parasites (83, 92) (Table 2). These two DUSPs along with the six STPs also detected in exosomes (i.e., LmPPEF, LcPP2C, two PP1, and two kSTPs) represent a small pool of Leishmania phosphatases that might directly interact with phosphorylation cascades inside host cells during infection.
Leishmania Membrane and Secreted AcPs
Until this year, direct modulation of host responses by Leishmania phosphatases had only been documented for AcPs. These phosphatases do not belong to the classical STP or PTP families because of the unique composition of their catalytic site that is based on histidine residues. In the literature, histidine phosphatases are usually not discussed along with other protein phosphatases as their substrate specificity has not been ascertained. AcP activities have been described against various substrates, including glycerophosphate, fructose-1,6-diphosphate (137, 138), phosphoinositides (139), pyrosphosphate (140), and phosphoproteins (138, 141).
A search for histidine phosphatases in the genome of six species of Leishmania pathogenic for humans (L. major, L. mexicana, L. braziliensis, L. panamensis, L. infantum, L. donovani) using their signature motif (Interpro accession reference for the histidine phosphatase superfamily: IPR000560) revealed that each Leishmania genome contained six or seven genes of this family. Extending our search to pathogenic Trypanosoma species (T. brucei and T. cruzi) revealed that this family of phosphatase is well conserved among the Trypanosomatidae. These genes are distributed in two main groups with four to five genes encoding membrane acid phosphatase (MAcP; also known as ectophosphatases) and two to three genes encoding secreted acid phosphatases (SAcP) (Figure 4A; Table S2 in Supplementary Material).
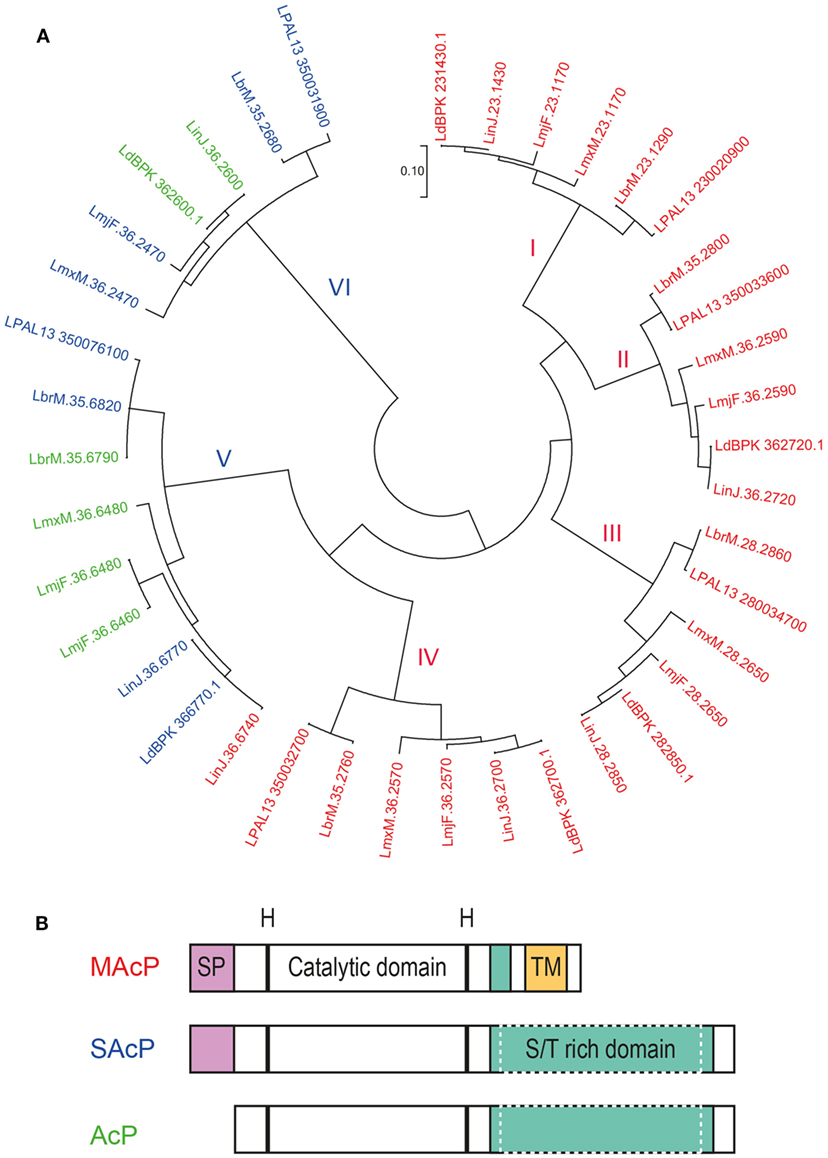
Figure 4. Acid phosphatases (AcPs) of Leishmania parasites. AcP, secreted by Leishmania parasites or expressed on their surface, belong to the family of histidine phosphatases. (A) Phylogenetic comparison between histidine phosphatases of L. major Friedlin, L. infantum JPCM5, L. donovani BPK282A1, L. mexicana MHOM/GT/2001/U1103, L. braziliensis MHOM/BR/75/M2904, L. panamensis MHOM/COL/81/L13. Available gene sequences were extracted from the TriTrypDB Kinetoplastid Genomics Resource database (IPR: 000560) and were used to construct a phylogenetic topology with the minimum evolution method. The evolutionary distance (scale) is measured in number of base substitutions per site. The evolutionary history was inferred using the Minimum Evolution method (142). The evolutionary distances were computed using the Maximum Composite Likelihood method (143) and are in the units of the number of base substitutions per site. The minimum evolution tree was searched using the Close-Neighbor-Interchange (CNI) algorithm (144) at a search level of 1. The Neighbor-Joining algorithm (145) was used to generate the initial tree. The analysis involved 39 nucleotide sequences. All positions containing gaps and missing data were eliminated. There was a total of 409 positions in the final data set. Evolutionary analyses were conducted in MEGA7.0.18 (146). Protein sequences of the respective genes were analyzed for the presence of a putative signal peptide (SP) and transmembrane domain (TM) with the Phobius software (147). Genes are labeled in red for the membrane AcP (MAcP) containing a signal peptide (SP) and transmembrane domain (TM), in blue for the secreted AcP (SAcP) containing only a SP and in green for the phosphatases lacking both domains. (B) Schematic organization of the protein domains of Leishmania AcP. Membrane and secreted AcP have a SP at their N-terminus followed by a catalytic domain based on histidine residues. Next, AcP harbor a serine/threonine(S/T)-rich domain that varies in length and can be significantly larger in SAcPs. Finally, MAcPs harbor a TM close to their C-terminus. Some AcPs (green in panel A) are devoid of SP and TM but can harbor long S/T-rich domains.
Membrane Acid Phosphatases
All MAcPs share the same structural organization starting with a signal peptide at the N-terminus followed by the catalytic domain containing the histidine residues and a transmembrane domain at the C-terminus (Figure 4B). Phylogenetically, MAcPs are organized in four distinct groups (Figure 4A, groups I–IV), all represented by one MAcP gene sequence derived from each of the six analyzed Leishmania genomes. Interestingly, an additional MAcP gene, LinJ.36.6740, could be detected in the genome of L. infantum. Despite being more related to SAcP and being substantially shorter than all other MAcP (only 35 kDa instead of usually around 60 kDa), this phosphatase harbors a signal peptide and a putative transmembrane domain at its end, illustrating the genetic plasticity of Leishmania parasites in which diverging paralogous genes are common. Gene divergence was also seen in the MAcP gene of L. donovani. The MAcP protein of the L. donovani strain MHOM/SD/62/1S-CL2D (148) carried a transmembrane domain, which was found to be replaced by a not-functionally defined C terminal sequence of 91 amino acids in the MAcP gene of the L. donovani strain BPK282A1 (149). Comparing the published sequences, the variation between these Leishmania strains was not related to frameshifts or aberrant stop codons but might result from a more complex evolutionary process.
Numerous studies aimed to characterize the function of MAcPs as they represented the first enzymatic activities identified on the surface of Leishmania promastigotes (150). Having been transported via the classical intracellular secretion pathway, it was an expected finding that the MAcPs were phospho-glycosylated (151). Interestingly, expression of MAcP varied during the different life stages of Leishmania parasites. In L. major, MAcP expression increased during metacyclogenesis of promastigotes and correlated with the translocation of the protein from the cytoplasm to the membrane (152). Increased expression of some of the MAcPs was also detected during metacyclogenesis and differentiation into amastigotes following macrophage infection (153, 154). Most recently, MAcPs were found to be differentially expressed in clinical isolates of the same Leishmania species (155). A MAcP of L. tropica (LTRL590_280034100, annotated as LmjF.28.2650) was described as one of the 30 most diversely expressed genes in a collection of 14 clinical isolates of L. tropica from different geographic areas. This might indicate that the parasite adapts the level of extracellular phosphatase activity depending on the specific infection situation. Functionally, early reports suggested that these ectophosphatases altered the response of neutrophils (156). Partially purified MAcP activity from L. donovani specifically interfered with the production of ROS by neutrophils after stimulation with the tripeptide N-formylmethionine-leucyl-phenylalanine (fMLP), whereas activation by PMA was resistant to MAcP activity (157). Other authors proposed that a phosphoinositide phosphatase activity of MAcP might play a role in this inhibition, because the phagocyte NADPH oxidase (Phox) relies on these signaling molecules for its activation (139). In accordance with this hypothesis, overexpression of LdMAcP by L. donovani promastigotes resulted in an increased survival during in vitro infection of a mouse macrophage cell line (158). However, deletion of the MAcP gene of L. mexicana (LmxM.36.2570 [LmxMBAP]) raised doubts about an essential role of this class of phosphatases for the virulence of L. mexicana parasites; LmxMBAP-deficient parasites were as virulent as wild-type parasites during in vitro infection of macrophages or in vivo infection of susceptible BALB/c mice (159). However, a definitive statement on the function of MAcPs is still not possible because of potential compensatory mechanisms as Leishmania parasites carry a minimum of four MAcP genes.
Secreted Acid Phosphatases
Secreted acid phosphatases are among the most abundant proteins secreted by Leishmania parasites (137, 160). However, a few Leishmania species, such as L. major, were shown to express very little SAcP as promastigotes (161). This difference could be due to the number of genes coding for truly secreted phosphatases. Our in silico analysis of the three L. major histidine phosphatases devoid of transmembrane regions revealed that only one, LmjF.36.2470, might have a functional signal peptide (Table S2 in Supplementary Material), whereas other Leishmania species such as L. panamensis, L. braziliensis, L. mexicana, and L. donovani can express more than one intact SAcP. The phylogenetic analysis also allowed the classification of SAcPs in two different families that are substantially divergent. One group of phosphatases (Figure 4A, group VI) branched out early from the histidine phosphatase family but is highly conserved across species, while the other group (Figure 4A, group V) is more closely related to MAcPs but is also more diverse. In members of group V, a signal peptide is sometimes absent, the enzymes can be significantly shorter than other orthologs and truncation could occur in the catalytic domain. This is the case for the two putatively non-secreted phosphatases of L. major, LmjF.36.6460, and LmjF.36.6480, indicating that the function of these two genes probably differs from their orthologs in case they are expressed.
For parasites expressing large amounts of SAcP, such as L. donovani, soluble phosphatase activity could be detected all along their differentiation from promastigotes to amastigotes (162). Interestingly, SAcP activity was also found in the cytosol of macrophages after 24 h of infection (11). However, the exact molecular identity of the phosphatase was not determined and this family of enzymes was also not detected inside exosomes that represent the main transit route of Leishmania cargo toward host cell cytosol. Like MAcPs, SAcPs are phospho-glycosylated proteins (163, 164). This modification relies on the same family of enzymes responsible for the LPG biosynthesis as a deletion of lpg2 gene, but not lpg1, abolished the phospho-glycosylation of SAcPs in L. donovani (165). Immunoprecipitation of SAcP suggested that the glycosylation pattern might increase during the parasite differentiation into amastigotes (11, 162). In addition to this posttranslational modification, SAcPs can form filamentous polymers. This quaternary structure has been extensively described for L. mexicana, but, surprisingly, was not seen with SAcPs from L. donovani, which remained mono- or oligomeric and non-filamentous (166). In case of L. mexicana, the filament formation occurred in the flagellar pocket of promastigotes (167) to create homo- or hetero-polymers of two phosphatases, LmSAP1 and LmSAP2. The genes encoding these two SAcPs are tandemly arranged and nearly identical, only differing by the addition of a long stretch of serine-threonine repetitions after the catalytic domain of LmSAP2 (168). This stretch is the site of intense glycosylation but does not interfere with the formation of the filament backbone constructed around the catalytic domains of LmSAPs (169). Remarkably, the published sequences of LmSAPs derived from L. mexicana MNYC/BZ/62/M379 could not be fully retrieved from the L. mexicana MHOM/GT/2001/U1103 strain that was sequenced, illustrating again the high plasticity of Leishmania genomes. An ortholog of the LmSAPs, LmxM.36.6480, has even a higher number of serine-threonine repetitions and seems to lack a functional signal peptide. In general, our search of the different Leishmania genomes showed that all SAcPs share a similar architecture but the size of the serine/threonine rich domain varies. This probably reflects the need to adapt the level of phospho-glycosylation to the different environments required by each parasite species.
Comparative genetic analysis of two different isolates of L. major from patients with contrasting severity of disease indicated that the hypo-virulent strain carried a mutated version of SAcP (170), suggesting a role of SAcP in virulence. Interestingly, a similar study looking at the genetic differences between two strains of L. donovani isolated from patients with cutaneous or visceral leishmaniasis, respectively, did not report a link between Leishmania AcPs and virulence (171). The function of SAcP in the virulence Leishmania has been directly addressed with parasites lacking the genomic locus encoding the two LmSAPs. Deletion of this locus abolished the virulence of L. mexicana promastigotes as they failed to transform into amastigotes during macrophage infection (172). However, this phenotype was independent of the LmSAPs because phenotypic complementation was achieved by adding back the MAPK gene that was identified in the intergenic region between the two LmSAP genes (172).
Together, these results demonstrate that the exact contribution of MAcPs and SAcPs to maintain the life cycle of Leishmania parasites is still unknown. First, the lack of a phenotype in a Leishmania mutant deficient for an AcP does not exclude its functional relevance, because compensation between different genes of each group could occur. Second, MAcP could also compensate for SAcP deficiency and vice versa. Finally, a role for these phosphatases also needs to be considered during sand fly infection by promastigotes. To experimentally address these hypotheses, the nature of the AcP substrate needs to be defined. If, however, these enzymes are primarily responsible for providing sufficient inorganic phosphate to the parasite as it has been proposed by Freitas-Mesquita et al. (140), it will be difficult to dissect the functional role of the different classes of AcPs and/or to overcome compensatory mechanisms.
Leishmania Phosphatase LmPRL-1 As a Secreted Virulence Factor
Recently, our laboratory has characterized a new dual specificity phosphatase, LmPRL-1, secreted by L. major during macrophage infection (118). The LmPRL-1 gene, LmjF.16.0230, is organized in tandem with a paralogous gene, LmjF.16.0250, which codes for LmPRL-2. Both PTPs represent the only two members of the family of the phosphatase of the regenerating liver (PRL) within L. major (Table 2). Human PRLs are well studied and are thought to participate in the control of various processes such as cell proliferation, differentiation and motility (173). Remarkably, both LmPRLs are highly conserved in all sequenced Leishmania species indicating that they might play a crucial role in the life of the parasite.
Structurally, we verified that Leishmania PRL-1, like its mammalian homolog, has two important characteristics. First, its cysteine-based catalytic site was shown to be under the control of a neighboring regulatory cysteine. Under oxidative conditions, this cysteine formed a transient inactivating disulfide bridge with the catalytic cysteine. Second, a third cysteine at the C-terminus could be farnesylated. This posttranslational modification controlled the correct subcellular localization inside promastigotes. During metacyclogenesis, LmPRL-1 was constantly expressed by promastigotes. Its level tended to decrease after amastigotes transformation in macrophages and also showed a weaker expression in amastigotes isolated from footpad lesions of L. major-infected BALB/c mice. Strikingly, during the first days of macrophage infection LmPRL-1 reached the cytoplasm of its host cells through the exosome route. There, LmPRL-1 appeared to support the intracellular replication of the parasite in primary mouse macrophages (118). We currently do not know the molecular mechanism by which LmPRL-1 promotes L. major virulence. One possibility is that LmPRL-1 has an intrinsic regulatory effect on parasite development and/or transformation. As secreted LmPRL-1 is detectable in host cell cytosol, it is also plausible to assume that LmPRL-1 modulates host cell signaling pathways by targeting phosphoproteins, interfering with the normal function of host PRLs, or altering the activity of other secreted virulence factors (Figure 5). We currently address these possible mechanisms in studies with LmPRL-1-deficient L. major parasites.
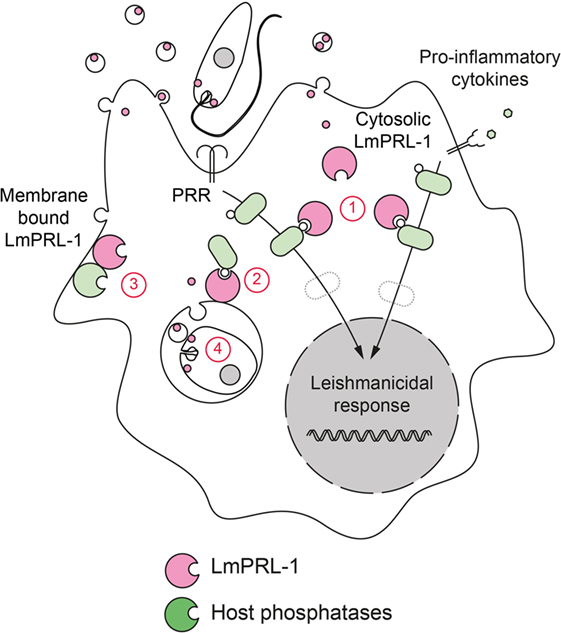
Figure 5. Hypotheses on the virulence mechanism of LmPRL-1. The phosphatase LmPRL-1 is secreted by Leishmania major promastigotes and amastigotes during macrophages infection. Once secreted, LmPRL-1 is localized at the membrane of the parasitophorous vacuole (PV) and/or in the cytosol of the infected macrophage. The observed support of intracellular parasite survival by LmPRL-1 could result from an inhibitory effect on important signaling pathways that otherwise promote the microbicidal response of the host macrophage (1). Other pathways not directly related to the innate immune response, but contributing to the general fitness of the macrophage and its function as host cell niche for the parasite could also be targeted by LmPRL-1 (2). Putative targets of LmPRL-1 are the host PRL proteins. Mammalian PRL activity relies on the formation of PRL trimers. LmPRL-1 might modulate the activity and function of its mammalian orthologs by physically interacting with them (3). Finally, a parasite-autonomous, intrinsic prosurvival effect of LmPRL-1 also needs to be envisaged (4).
Concluding Remarks
Host–pathogen interactions taking place during leishmaniasis rely on complex antagonistic and synergistic molecular interplays some of which we have summarized in this review.
Leishmania parasite survival and disease development depend on the fitness of the host immune reaction. To counteract the generation of a microbicidal host response, Leishmania parasites have developed efficient strategies to extinguish or impede the proinflammatory signaling pathways triggered by their detection. Proteases secreted by the parasite, such GP63 and LmCPb, represent important components to achieve this goal and finally escape the deadly production of leishmanicidal compounds. Among the targets of these virulence factors, host protein phosphatases, especially SHP-1, are of particular relevance. Once proteolytically activated, these host phosphatases can directly inhibit the signaling pathways that are required for an effective anti-Leishmania host response (Figure 3).
While the parasite-driven activation of host phosphatases appears to be the modus operandi of choice for Leishmania to impede host immune responses, an additional strategy has been postulated. Already in the 1980s, the discovery of SAcP activities suggested that Leishmania parasites could use its own secreted enzymes to manipulate its host. However, until now a link between SAcP and Leishmania virulence has not yet been ascertained, probably because of the redundancy between the different orthologous gene encoded by each Leishmania genome. Only recently, our study of the Leishmania dual specificity phosphatase of the PRL family, LmPRL-1, provided the first evidence for a phosphatase secreted by Leishmania that promotes the virulence of the parasite during macrophage infection (Figure 5). As LmPRL-1 is secreted within exosomes, it is accompanied by a miscellaneous pool of proteins and small RNAs (174). Among these exosomal proteins, five other phosphatases have been detected that require further characterization with respect to their possible role as virulence factors of the parasite and their participation in the modulation of phosphorylation events in host cells.
In the past, the mechanism of activation of host phosphatases by Leishmania has been extensively studied by several research groups and thereby led to the identification of some of the signaling pathways that are targeted by the parasite. The efforts should now focus on the biochemical and functional characterization of new Leishmania virulence factors that directly interfere with phosphorylation events in host cells. In addition, our knowledge of host and Leishmania phosphatases that was mainly acquired in mouse models, still requires to be tested in clinical settings in order to establish whether differential expression of Leishmania phosphatases contributes to the severe courses of infection seen in mucocutaneous or visceral leishmaniasis.
Author Contributions
All authors listed, have made a substantial, direct and intellectual contribution to the work, and approved it for publication.
Conflict of Interest Statement
The authors declare that the research was conducted in the absence of any commercial or financial relationships that could be construed as a potential conflict of interest.
Acknowledgments
We apologize to all authors, whose work could only be acknowledged by referring to previous review articles because of space limitations.
Funding
The preparation of this manuscript and some of the work reviewed was supported by the Deutsche Forschungsgemeinschaft (grants BO 996/5-1 [SPP1937] and SFB 1181, project C04, to CB; grant SO 1149/1-1 to DS), the Interdisciplinary Center for Clinical Research (IZKF) of the Universitätsklinikum Erlangen (project grants A61 and A63), and by the Dr. Robert Pfleger-Stiftung, Bamberg (project grant to CB).
Supplementary Material
The Supplementary Material for this article can be found online at http://www.frontiersin.org/articles/10.3389/fimmu.2017.01838/full#supplementary-material.
Footnotes
References
1. GBD 2015 DALYs and HALE Collaborators. Global, regional, and national disability-adjusted life-years (DALYs) for 315 diseases and injuries and healthy life expectancy (HALE), 1990–2015: a systematic analysis for the Global Burden of Disease Study 2015. Lancet (2016) 388:1603–58. doi:10.1016/S0140-6736(16)31460-X
2. Laskay T, van Zandbergen G, Solbach W. Neutrophil granulocytes as host cells and transport vehicles for intracellular pathogens: apoptosis as infection-promoting factor. Immunobiology (2008) 213:183–91. doi:10.1016/j.imbio.2007.11.010
3. Ribeiro-Gomes FL, Sacks D. The influence of early neutrophil-Leishmania interactions on the host immune response to infection. Front Cell Infect Microbiol (2012) 2:59. doi:10.3389/fcimb.2012.00059
4. Rittig MG, Bogdan C. Leishmania-host-cell interaction: complexities and alternative views. Parasitol Today (2000) 16:292–7. doi:10.1016/S0169-4758(00)01692-6
5. Cecílio P, Pérez-Cabezas B, Santarém N, Maciel J, Rodrigues V, Cordeiro da Silva A. Deception and manipulation: the arms of Leishmania, a successful parasite. Front Immunol (2014) 5:480. doi:10.3389/fimmu.2014.00480
7. David CV, Craft N. Cutaneous and mucocutaneous leishmaniasis. Dermatol Ther (2009) 22:491–502. doi:10.1111/j.1529-8019.2009.01272.x
8. Murray HW, Berman JD, Davies CR, Saravia NG. Advances in leishmaniasis. Lancet (2005) 366:1561–77. doi:10.1016/S0140-6736(05)67629-5
9. Bogdan C. Leishmaniasis in rheumatology, haematology and oncology: epidemiological, immunological and clinical aspects and caveats. Ann Rheum Dis (2012) 71(Suppl 2):i60–6. doi:10.1136/annrheumdis-2011-200596
10. Kaye P, Scott P. Leishmaniasis: complexity at the host-pathogen interface. Nat Rev Microbiol (2011) 9:604–15. doi:10.1038/nrmicro2608
11. McCall L-I, Matlashewski G. Localization and induction of the A2 virulence factor in Leishmania: evidence that A2 is a stress response protein. Mol Microbiol (2010) 77:518–30. doi:10.1111/j.1365-2958.2010.07229.x
12. Bogdan C, Röllinghoff M. The immune response to Leishmania: mechanisms of parasite control and evasion. Int J Parasitol (1998) 28:121–34. doi:10.1016/S0020-7519(97)00169-0
13. Kima PE. The amastigote forms of Leishmania are experts at exploiting host cell processes to establish infection and persist. Int J Parasitol (2007) 37:1087–96. doi:10.1016/j.ijpara.2007.04.007
14. Atayde VD, Hassani K, da Silva Lira Filho A, Borges AR, Adhikari A, Martel C, et al. Leishmania exosomes and other virulence factors: impact on innate immune response and macrophage functions. Cell Immunol (2016) 309:7–18. doi:10.1016/j.cellimm.2016.07.013
15. Peters NC, Sacks DL. The impact of vector-mediated neutrophil recruitment on cutaneous leishmaniasis. Cell Microbiol (2009) 11:1290–6. doi:10.1111/j.1462-5822.2009.01348.x
16. Carlsen ED, Liang Y, Shelite TR, Walker DH, Melby PC, Soong L. Permissive and protective roles for neutrophils in leishmaniasis. Clin Exp Immunol (2015) 182:109–18. doi:10.1111/cei.12674
17. Bogdan C. Natural killer cells in experimental and human leishmaniasis. Front Cell Infect Microbiol (2012) 2:69. doi:10.3389/fcimb.2012.00069
18. Bogdan C, Röllinghoff M, Diefenbach A. The role of nitric oxide in innate immunity. Immunol Rev (2000) 173:17–26. doi:10.1034/j.1600-065X.2000.917307.x
19. Müller AJ, Aeschlimann S, Olekhnovitch R, Dacher M, Späth GF, Bousso P. Photoconvertible pathogen labeling reveals nitric oxide control of Leishmania major infection in vivo via dampening of parasite metabolism. Cell Host Microbe (2013) 14:460–7. doi:10.1016/j.chom.2013.09.008
20. Olekhnovitch R, Ryffel B, Müller AJ, Bousso P. Collective nitric oxide production provides tissue-wide immunity during Leishmania infection. J Clin Invest (2014) 124:1711–22. doi:10.1172/JCI72058
21. Schleicher U, Paduch K, Debus A, Obermeyer S, König T, Kling JC, et al. TNF-mediated restriction of arginase 1 expression in myeloid cells triggers type 2 NO synthase activity at the site of infection. Cell Rep (2016) 15:1062–75. doi:10.1016/j.celrep.2016.04.001
22. Bogdan C. Nitric oxide synthase in innate and adaptive immunity: an update. Trends Immunol (2015) 36:161–78. doi:10.1016/j.it.2015.01.003
23. Vinet AF, Fukuda M, Turco SJ, Descoteaux A. The Leishmania donovani lipophosphoglycan excludes the vesicular proton-ATPase from phagosomes by impairing the recruitment of synaptotagmin V. PLoS Pathog (2009) 5:e1000628. doi:10.1371/journal.ppat.1000628
24. Holm A, Tejle K, Magnusson KE, Descoteaux A, Rasmusson B. Leishmania donovani lipophosphoglycan causes periphagosomal actin accumulation: correlation with impaired translocation of PKCalpha and defective phagosome maturation. Cell Microbiol (2001) 3:439–47. doi:10.1046/j.1462-5822.2001.00127.x
25. Olivier M, Brownsey RW, Reiner NE. Defective stimulus-response coupling in human monocytes infected with Leishmania donovani is associated with altered activation and translocation of protein kinase C. Proc Natl Acad Sci U S A (1992) 89:7481–5. doi:10.1073/pnas.89.16.7481
26. Pingel S, Wang ZE, Locksley RM. Distribution of protein kinase C isoforms after infection of macrophages with Leishmania major. Infect Immun (1998) 66:1795–9.
27. Salei N, Hellberg L, Köhl J, Laskay T. Enhanced survival of Leishmania major in neutrophil granulocytes in the presence of apoptotic cells. PLoS One (2017) 12:e0171850. doi:10.1371/journal.pone.0171850
28. Bhattacharyya S, Ghosh S, Sen P, Roy S, Majumdar S. Selective impairment of protein kinase C isotypes in murine macrophage by Leishmania donovani. Mol Cell Biochem (2001) 216:47–57. doi:10.1023/A:1011048210691
29. Shio MT, Christian JG, Jung JY, Chang K-P, Olivier M. PKC/ROS-mediated NLRP3 inflammasome activation is attenuated by Leishmania zinc-metalloprotease during infection. PLoS Negl Trop Dis (2015) 9:e0003868. doi:10.1371/journal.pntd.0003868
30. Martiny A, Meyer-Fernandes JR, de Souza W, Vannier-Santos MA. Altered tyrosine phosphorylation of ERK1 MAP kinase and other macrophage molecules caused by Leishmania amastigotes. Mol Biochem Parasitol (1999) 102:1–12. doi:10.1016/S0166-6851(99)00067-5
31. Nandan D, Lo R, Reiner NE. Activation of phosphotyrosine phosphatase activity attenuates mitogen-activated protein kinase signaling and inhibits c-FOS and nitric oxide synthase expression in macrophages infected with Leishmania donovani. Infect Immun (1999) 67:4055–63.
32. Privé C, Descoteaux A. Leishmania donovani promastigotes evade the activation of mitogen-activated protein kinases p38, c-Jun N-terminal kinase, and extracellular signal-regulated kinase-1/2 during infection of naive macrophages. Eur J Immunol (2000) 30:2235–44. doi:10.1002/1521-4141(2000)30:8<2235::AID-IMMU2235>3.0.CO;2-9
33. Guler R, Afshar M, Arendse B, Parihar SP, Revaz-Breton M, Leitges M, et al. PKCδ regulates IL-12p40/p70 production by macrophages and dendritic cells, driving a type 1 healer phenotype in cutaneous leishmaniasis. Eur J Immunol (2011) 41:706–15. doi:10.1002/eji.201040985
34. Fukao T, Tanabe M, Terauchi Y, Ota T, Matsuda S, Asano T, et al. PI3K-mediated negative feedback regulation of IL-12 production in DCs. Nat Immunol (2002) 3:875–81. doi:10.1038/ni825
35. Pereira RMS, Teixeira KLD, Barreto-de-Souza V, Calegari-Silva TC, De-Melo LDB, Soares DC, et al. Novel role for the double-stranded RNA-activated protein kinase PKR: modulation of macrophage infection by the protozoan parasite Leishmania. FASEB J (2010) 24:617–26. doi:10.1096/fj.09-140053
36. Faria MS, Calegari-Silva TC, de Carvalho Vivarini A, Mottram JC, Lopes UG, Lima APCA. Role of protein kinase R in the killing of Leishmania major by macrophages in response to neutrophil elastase and TLR4 via TNFα and IFNβ. FASEB J (2014) 28:3050–63. doi:10.1096/fj.13-245126
37. Murray HW, Lu CM, Mauze S, Freeman S, Moreira AL, Kaplan G, et al. Interleukin-10 (IL-10) in experimental visceral leishmaniasis and IL-10 receptor blockade as immunotherapy. Infect Immun (2002) 70:6284–93. doi:10.1128/IAI.70.11.6284-6293.2002
38. Murphy ML, Wille U, Villegas EN, Hunter CA, Farrell JP. IL-10 mediates susceptibility to Leishmania donovani infection. Eur J Immunol (2001) 31:2848–56. doi:10.1002/1521-4141(2001010)31:10<2848::AID-IMMU2848>3.0.CO;2-T
39. Kane MM, Mosser DM. The role of IL-10 in promoting disease progression in leishmaniasis. J Immunol (2001) 166:1141–7. doi:10.4049/jimmunol.166.2.1141
40. Buxbaum LU, Scott P. Interleukin 10- and Fcgamma receptor-deficient mice resolve Leishmania mexicana lesions. Infect Immun (2005) 73:2101–8. doi:10.1128/IAI.73.4.2101-2108.2005
41. Schwarz T, Remer KA, Nahrendorf W, Masic A, Siewe L, Müller W, et al. T cell-derived IL-10 determines leishmaniasis disease outcome and is suppressed by a dendritic cell based vaccine. PLoS Pathog (2013) 9:e1003476. doi:10.1371/journal.ppat.1003476
42. Nandan D, Camargo de Oliveira C, Moeenrezakhanlou A, Lopez M, Silverman JM, Subek J, et al. Myeloid cell IL-10 production in response to Leishmania involves inactivation of glycogen synthase kinase-3β downstream of phosphatidylinositol-3 kinase. J Immunol (2012) 188:367–78. doi:10.4049/jimmunol.1100076
43. Blanchette J, Racette N, Faure R, Siminovitch KA, Olivier M. Leishmania-induced increases in activation of macrophage SHP-1 tyrosine phosphatase are associated with impaired IFN-gamma-triggered JAK2 activation. Eur J Immunol (1999) 29:3737–44. doi:10.1002/(SICI)1521-4141(199911)29:11<3737::AID-IMMU3737>3.0.CO;2-S
44. Forget G, Gregory DJ, Whitcombe LA, Olivier M. Role of host protein tyrosine phosphatase SHP-1 in Leishmania donovani-induced inhibition of nitric oxide production. Infect Immun (2006) 74:6272–9. doi:10.1128/IAI.00853-05
45. Brautigan DL. Protein Ser/Thr phosphatases – the ugly ducklings of cell signalling. FEBS J (2013) 280:324–45. doi:10.1111/j.1742-4658.2012.08609.x
46. Alonso A, Sasin J, Bottini N, Friedberg I, Friedberg I, Osterman A, et al. Protein tyrosine phosphatases in the human genome. Cell (2004) 117:699–711. doi:10.1016/j.cell.2004.05.018
47. Contreras I, Estrada JA, Guak H, Martel C, Borjian A, Ralph B, et al. Impact of Leishmania mexicana infection on dendritic cell signaling and functions. PLoS Negl Trop Dis (2014) 8:e3202. doi:10.1371/journal.pntd.0003202
48. Mookerjee A, Sen PC, Ghose AC. Immunosuppression in hamsters with progressive visceral leishmaniasis is associated with an impairment of protein kinase C activity in their lymphocytes that can be partially reversed by okadaic acid or anti-transforming growth factor beta antibody. Infect Immun (2003) 71:2439–46. doi:10.1128/IAI.71.5.2439-2446.2003
49. Mukherjee P, Sen PC, Ghose AC. Lymph node cells from BALB/c mice with chronic visceral leishmaniasis exhibiting cellular anergy and apoptosis: involvement of Ser/Thr phosphatase. Apoptosis (2006) 11:2013–29. doi:10.1007/s10495-006-0088-7
50. Dey R, Majumder N, Bhattacharjee S, Majumdar SB, Banerjee R, Ganguly S, et al. Leishmania donovani-induced ceramide as the key mediator of Akt dephosphorylation in murine macrophages: role of protein kinase Czeta and phosphatase. Infect Immun (2007) 75:2136–42. doi:10.1128/IAI.01589-06
51. Dong Z, Yang X, Xie K, Juang SH, Llansa N, Fidler IJ. Activation of inducible nitric oxide synthase gene in murine macrophages requires protein phosphatases 1 and 2A activities. J Leukoc Biol (1995) 58:725–32.
52. Waitumbi J, Warburg A. Phlebotomus papatasi saliva inhibits protein phosphatase activity and nitric oxide production by murine macrophages. Infect Immun (1998) 66:1534–7.
53. Takeda K, Komuro Y, Hayakawa T, Oguchi H, Ishida Y, Murakami S, et al. Mitochondrial phosphoglycerate mutase 5 uses alternate catalytic activity as a protein serine/threonine phosphatase to activate ASK1. Proc Natl Acad Sci U S A (2009) 106:12301–5. doi:10.1073/pnas.0901823106
54. Farias Luz N, Balaji S, Okuda K, Barreto AS, Bertin J, Gough PJ, et al. RIPK1 and PGAM5 control Leishmania replication through distinct mechanisms. J Immunol (2016) 196:5056–63. doi:10.4049/jimmunol.1502492
55. Olivier M, Romero-Gallo BJ, Matte C, Blanchette J, Posner BI, Tremblay MJ, et al. Modulation of interferon-gamma-induced macrophage activation by phosphotyrosine phosphatases inhibition. Effect on murine leishmaniasis progression. J Biol Chem (1998) 273:13944–9. doi:10.1074/jbc.273.22.13944
56. Guizani-Tabbane L, Ouni I, Sassi A, Dellagi K. Leishmania major induces deactivation of extracellular signal regulated kinases 2 in human U937 macrophage like cells. Arch Inst Pasteur Tunis (2000) 77:45–50.
57. Martiny A, Vannier-Santos MA, Borges VM, Meyer-Fernandes JR, Assreuy J, Cunha e Silva NL, et al. Leishmania-induced tyrosine phosphorylation in the host macrophage and its implication to infection. Eur J Cell Biol (1996) 71:206–15.
58. Ghosh D, Chakraborty P. Involvement of protein tyrosine kinases and phosphatases in uptake and intracellular replication of virulent and avirulent Leishmania donovani promastigotes in mouse macrophage cells. Biosci Rep (2002) 22:395–406. doi:10.1023/A:1020914024544
59. Matte C, Marquis JF, Blanchette J, Gros P, Faure R, Posner BI, et al. Peroxovanadium-mediated protection against murine leishmaniasis: role of the modulation of nitric oxide. Eur J Immunol (2000) 30:2555–64. doi:10.1002/1521-4141(200009)30:9<2555:AID-IMMU2555>3.0.CO;2-X
60. Kar S, Ukil A, Sharma G, Das PK. MAPK-directed phosphatases preferentially regulate pro- and anti-inflammatory cytokines in experimental visceral leishmaniasis: involvement of distinct protein kinase C isoforms. J Leukoc Biol (2010) 88:9–20. doi:10.1189/jlb.0909644
61. Ukil A, Biswas A, Das T, Das PK. 18-beta-glycyrrhetinic acid triggers curative Th1 response and nitric oxide up-regulation in experimental visceral leishmaniasis associated with the activation of NF-kappa B. J Immunol (2005) 175:1161–9. doi:10.4049/jimmunol.175.2.1161
62. Ukil A, Kar S, Srivastav S, Ghosh K, Das PK. Curative effect of 18β-glycyrrhetinic acid in experimental visceral leishmaniasis depends on phosphatase-dependent modulation of cellular MAP kinases. PLoS One (2011) 6:e29062. doi:10.1371/journal.pone.0029062
63. Srivastava N, Sudan R, Saha B. CD40-modulated dual-specificity phosphatases MAPK phosphatase (MKP)-1 and MKP-3 reciprocally regulate Leishmania major infection. J Immunol (2011) 186:5863–72. doi:10.4049/jimmunol.1003957
64. Parveen S, Bandhyopadhyay S, Das S, Majumdar SB, Jawed JJ, Chowdhury BP, et al. Mycobacterium indicus pranii (Mw)-mediated protection against visceral leishmaniasis by reciprocal regulation of host dual-specificity phosphatases. Int Immunol (2016) 28:585–95. doi:10.1093/intimm/dxw049
65. Al-Mutairi MS, Cadalbert LC, McGachy HA, Shweash M, Schroeder J, Kurnik M, et al. MAP kinase phosphatase-2 plays a critical role in response to infection by Leishmania mexicana. PLoS Pathog (2010) 6:e1001192. doi:10.1371/journal.ppat.1001192
66. Schroeder J, McGachy HA, Woods S, Plevin R, Alexander J. T cell hypo-responsiveness against Leishmania major in MAP kinase phosphatase (MKP) 2 deficient C57BL/6 mice does not alter the healer disease phenotype. PLoS Negl Trop Dis (2013) 7:e2064. doi:10.1371/journal.pntd.0002064
67. Shultz LD, Schweitzer PA, Rajan TV, Yi T, Ihle JN, Matthews RJ, et al. Mutations at the murine motheaten locus are within the hematopoietic cell protein-tyrosine phosphatase (Hcph) gene. Cell (1993) 73:1445–54. doi:10.1016/0092-8674(93)90369-2
68. Forget G, Siminovitch KA, Brochu S, Rivest S, Radzioch D, Olivier M. Role of host phosphotyrosine phosphatase SHP-1 in the development of murine leishmaniasis. Eur J Immunol (2001) 31:3185–96. doi:10.1002/1521-4141(200111)31:11<3185::AID-IMMU3185>3.0.CO;2-J
69. Forget G, Matte C, Siminovitch KA, Rivest S, Pouliot P, Olivier M. Regulation of the Leishmania-induced innate inflammatory response by the protein tyrosine phosphatase SHP-1. Eur J Immunol (2005) 35:1906–17. doi:10.1002/eji.200526037
70. Späth GF, McDowell MA, Beverley SM. Leishmania major intracellular survival is not altered in SHP-1 deficient mev or CD45-/- mice. Exp Parasitol (2008) 120:275–9. doi:10.1016/j.exppara.2008.07.003
71. Forget G, Gregory DJ, Olivier M. Proteasome-mediated degradation of STAT1alpha following infection of macrophages with Leishmania donovani. J Biol Chem (2005) 280:30542–9. doi:10.1074/jbc.M414126200
72. Blanchette J, Abu-Dayyeh I, Hassani K, Whitcombe L, Olivier M. Regulation of macrophage nitric oxide production by the protein tyrosine phosphatase Src homology 2 domain phosphotyrosine phosphatase 1 (SHP-1). Immunology (2009) 127:123–33. doi:10.1111/j.1365-2567.2008.02929.x
73. Khan TH, Srivastava N, Srivastava A, Sareen A, Mathur RK, Chande AG, et al. SHP-1 plays a crucial role in CD40 signaling reciprocity. J Immunol (2014) 193:3644–53. doi:10.4049/jimmunol.1400620
74. Abu-Dayyeh I, Shio MT, Sato S, Akira S, Cousineau B, Olivier M. Leishmania-induced IRAK-1 inactivation is mediated by SHP-1 interacting with an evolutionarily conserved KTIM motif. PLoS Negl Trop Dis (2008) 2:e305. doi:10.1371/journal.pntd.0000305
75. Das S, Pandey K, Kumar A, Sardar AH, Purkait B, Kumar M, et al. TGF-β1 re-programs TLR4 signaling in L. donovani infection: enhancement of SHP-1 and ubiquitin-editing enzyme A20. Immunol Cell Biol (2012) 90:640–54. doi:10.1038/icb.2011.80
76. Iborra S, Martínez-López M, Cueto FJ, Conde-Garrosa R, Del Fresno C, Izquierdo HM, et al. Leishmania uses mincle to target an inhibitory ITAM signaling pathway in dendritic cells that dampens adaptive immunity to infection. Immunity (2016) 45:788–801. doi:10.1016/j.immuni.2016.09.012
77. Roy S, Mandal C. Leishmania donovani utilize sialic acids for binding and phagocytosis in the macrophages through selective utilization of siglecs and impair the innate immune arm. PLoS Negl Trop Dis (2016) 10:e0004904. doi:10.1371/journal.pntd.0004904
78. Shio MT, Hassani K, Isnard A, Ralph B, Contreras I, Gomez MA, et al. Host cell signalling and Leishmania mechanisms of evasion. Re Dai Yi Xue Za Zhi (2012) 2012:1–14. doi:10.1155/2012/819512
79. Gomez MA, Li S, Tremblay ML, Olivier M. NRAMP-1 expression modulates protein-tyrosine phosphatase activity in macrophages: impact on host cell signaling and functions. J Biol Chem (2007) 282:36190–8. doi:10.1074/jbc.M703140200
80. Basu Ball W, Kar S, Mukherjee M, Chande AG, Mukhopadhyaya R, Das PK. Uncoupling protein 2 negatively regulates mitochondrial reactive oxygen species generation and induces phosphatase-mediated anti-inflammatory response in experimental visceral leishmaniasis. J Immunol (2011) 187:1322–32. doi:10.4049/jimmunol.1004237
81. Nandan D, Yi T, Lopez M, Lai C, Reiner NE. Leishmania EF-1alpha activates the Src homology 2 domain containing tyrosine phosphatase SHP-1 leading to macrophage deactivation. J Biol Chem (2002) 277:50190–7. doi:10.1074/jbc.M209210200
82. Nandan D, Cherkasov A, Sabouti R, Yi T, Reiner NE. Molecular cloning, biochemical and structural analysis of elongation factor-1 alpha from Leishmania donovani: comparison with the mammalian homologue. Biochem Biophys Res Commun (2003) 302:646–52. doi:10.1016/S0006-291X(03)00216-X
83. Silverman JM, Clos J, de’Oliveira CC, Shirvani O, Fang Y, Wang C, et al. An exosome-based secretion pathway is responsible for protein export from Leishmania and communication with macrophages. J Cell Sci (2010) 123:842–52. doi:10.1242/jcs.056465
84. Silverman JM, Chan SK, Robinson DP, Dwyer DM, Nandan D, Foster LJ, et al. Proteomic analysis of the secretome of Leishmania donovani. Genome Biol (2008) 9:R35. doi:10.1186/gb-2008-9-2-r35
85. Hassani K, Antoniak E, Jardim A, Olivier M. Temperature-induced protein secretion by Leishmania mexicana modulates macrophage signalling and function. PLoS One (2011) 6:e18724. doi:10.1371/journal.pone.0018724
86. Silverman JM, Clos J, Horakova E, Wang AY, Wiesgigl M, Kelly I, et al. Leishmania exosomes modulate innate and adaptive immune responses through effects on monocytes and dendritic cells. J Immunol (2010) 185:5011–22. doi:10.4049/jimmunol.1000541
87. Atayde VD, Aslan H, Townsend S, Hassani K, Kamhawi S, Olivier M. Exosome secretion by the parasitic protozoan Leishmania within the sand fly midgut. Cell Rep (2015) 13:957–67. doi:10.1016/j.celrep.2015.09.058
88. Nandan D, Tran T, Trinh E, Silverman JM, Lopez M. Identification of Leishmania fructose-1,6-bisphosphate aldolase as a novel activator of host macrophage Src homology 2 domain containing protein tyrosine phosphatase SHP-1. Biochem Biophys Res Commun (2007) 364:601–7. doi:10.1016/j.bbrc.2007.10.065
89. Yao C, Donelson JE, Wilson ME. Internal and surface-localized major surface proteases of Leishmania spp. and their differential release from promastigotes. Eukaryot Cell (2007) 6:1905–12. doi:10.1128/EC.00073-07
90. Brittingham A, Morrison CJ, McMaster WR, McGwire BS, Chang KP, Mosser DM. Role of the Leishmania surface protease gp63 in complement fixation, cell adhesion, and resistance to complement-mediated lysis. J Immunol (1995) 155:3102–11.
91. Brittingham A, Chen G, McGwire BS, Chang KP, Mosser DM. Interaction of Leishmania gp63 with cellular receptors for fibronectin. Infect Immun (1999) 67:4477–84.
92. Hassani K, Shio MT, Martel C, Faubert D, Olivier M. Absence of metalloprotease GP63 alters the protein content of Leishmania exosomes. PLoS One (2014) 9:e95007. doi:10.1371/journal.pone.0095007
93. Hallé M, Gomez MA, Stuible M, Shimizu H, McMaster WR, Olivier M, et al. The Leishmania surface protease GP63 cleaves multiple intracellular proteins and actively participates in p38 mitogen-activated protein kinase inactivation. J Biol Chem (2009) 284:6893–908. doi:10.1074/jbc.M805861200
94. Corradin S, Ransijn A, Corradin G, Roggero MA, Schmitz AA, Schneider P, et al. MARCKS-related protein (MRP) is a substrate for the Leishmania major surface protease leishmanolysin (gp63). J Biol Chem (1999) 274:25411–8. doi:10.1074/jbc.274.36.25411
95. Jaramillo M, Gomez MA, Larsson O, Shio MT, Topisirovic I, Contreras I, et al. Leishmania repression of host translation through mTOR cleavage is required for parasite survival and infection. Cell Host Microbe (2011) 9:331–41. doi:10.1016/j.chom.2011.03.008
96. Contreras I, Gomez MA, Nguyen O, Shio MT, McMaster RW, Olivier M. Leishmania-induced inactivation of the macrophage transcription factor AP-1 is mediated by the parasite metalloprotease GP63. PLoS Pathog (2010) 6:e1001148. doi:10.1371/journal.ppat.1001148
97. Gregory DJ, Godbout M, Contreras I, Forget G, Olivier M. A novel form of NF-kappaB is induced by Leishmania infection: involvement in macrophage gene expression. Eur J Immunol (2008) 38:1071–81. doi:10.1002/eji.200737586
98. Gomez MA, Contreras I, Hallé M, Tremblay ML, McMaster RW, Olivier M. Leishmania GP63 alters host signaling through cleavage-activated protein tyrosine phosphatases. Sci Signal (2009) 2:ra58–58. doi:10.1126/scisignal.2000213
99. Abu-Dayyeh I, Hassani K, Westra ER, Mottram JC, Olivier M. Comparative study of the ability of Leishmania mexicana promastigotes and amastigotes to alter macrophage signaling and functions. Infect Immun (2010) 78:2438–45. doi:10.1128/IAI.00812-09
100. Souza AE, Waugh S, Coombs GH, Mottram JC. Characterization of a multi-copy gene for a major stage-specific cysteine proteinase of Leishmania mexicana. FEBS Lett (1992) 311:124–7. doi:10.1016/0014-5793(92)81382-V
101. Depledge DP, Evans KJ, Ivens AC, Aziz N, Maroof A, Kaye PM, et al. Comparative expression profiling of Leishmania: modulation in gene expression between species and in different host genetic backgrounds. PLoS Negl Trop Dis (2009) 3:e476. doi:10.1371/journal.pntd.0000476
102. Tsigankov P, Gherardini PF, Helmer-Citterich M, Späth GF, Zilberstein D. Phosphoproteomic analysis of differentiating Leishmania parasites reveals a unique stage-specific phosphorylation motif. J Proteome Res (2013) 12:3405–12. doi:10.1021/pr4002492
103. Tsigankov P, Gherardini PF, Helmer-Citterich M, Späth GF, Myler PJ, Zilberstein D. Regulation dynamics of Leishmania differentiation: deconvoluting signals and identifying phosphorylation trends. Mol Cell Proteomics (2014) 13:1787–99. doi:10.1074/mcp.M114.037705
104. Morales MA, Watanabe R, Laurent C, Lenormand P, Rousselle J-C, Namane A, et al. Phosphoproteomic analysis of Leishmania donovani pro- and amastigote stages. Proteomics (2008) 8:350–63. doi:10.1002/pmic.200700697
105. Morales MA, Watanabe R, Dacher M, Chafey P, Osorio y Fortéa J, Scott DA, et al. Phosphoproteome dynamics reveal heat-shock protein complexes specific to the Leishmania donovani infectious stage. Proc Natl Acad Sci U S A (2010) 107:8381–6. doi:10.1073/pnas.0914768107
106. Parsons M, Worthey EA, Ward PN, Mottram JC. Comparative analysis of the kinomes of three pathogenic trypanosomatids: Leishmania major, Trypanosoma brucei and Trypanosoma cruzi. BMC Genomics (2005) 6:127. doi:10.1186/1471-2164-6-127
107. Brenchley R, Tariq H, McElhinney H, Szöőr B, Huxley-Jones J, Stevens R, et al. The TriTryp phosphatome: analysis of the protein phosphatase catalytic domains. BMC Genomics (2007) 8:434. doi:10.1186/1471-2164-8-434
108. Rotureau B, Morales MA, Bastin P, Späth GF. The flagellum-mitogen-activated protein kinase connection in trypanosomatids: a key sensory role in parasite signalling and development? Cell Microbiol (2009) 11:710–8. doi:10.1111/j.1462-5822.2009.01295.x
109. Goldman-Pinkovich A, Balno C, Strasser R, Zeituni-Molad M, Bendelak K, Rentsch D, et al. An arginine deprivation response pathway is induced in Leishmania during macrophage invasion. PLoS Pathog (2016) 12:e1005494. doi:10.1371/journal.ppat.1005494
110. Szöör B. Trypanosomatid protein phosphatases. Mol Biochem Parasitol (2010) 173:53–63. doi:10.1016/j.molbiopara.2010.05.017
111. Bhandari V, Sundar S, Dujardin JC, Salotra P. Elucidation of cellular mechanisms involved in experimental paromomycin resistance in Leishmania donovani. Antimicrob Agents Chemother (2014) 58:2580–5. doi:10.1128/AAC.01574-13
112. Norris-Mullins B, Vacchina P, Morales MA. Catalytic activity of a novel serine/threonine protein phosphatase PP5 from Leishmania major. Parasite (2014) 21:25. doi:10.1051/parasite/2014027
113. Mills E, Price HP, Johner A, Emerson JE, Smith DF. Kinetoplastid PPEF phosphatases: dual acylated proteins expressed in the endomembrane system of Leishmania. Mol Biochem Parasitol (2007) 152:22–34. doi:10.1016/j.molbiopara.2006.11.008
114. Gazanion É, Fernández-Prada C, Papadopoulou B, Leprohon P, Ouellette M. Cos-Seq for high-throughput identification of drug target and resistance mechanisms in the protozoan parasite Leishmania. Proc Natl Acad Sci U S A (2016) 113:E3012–21. doi:10.1073/pnas.1520693113
115. Bagher Khadem Erfan M, Mohebali M, Kazemi-Rad E, Hajjaran H, Edrissian G, Mamishi S, et al. Downregulation of calcineurin gene is associated with Glucantime(®) resistance in Leishmania infantum. Iran J Parasitol (2013) 8:359–66.
116. Burns JM, Parsons M, Rosman DE, Reed SG. Molecular cloning and characterization of a 42-kDa protein phosphatase of Leishmania chagasi. J Biol Chem (1993) 268:17155–61.
117. Nascimento M, Zhang W-W, Ghosh A, Houston DR, Berghuis AM, Olivier M, et al. Identification and characterization of a protein-tyrosine phosphatase in Leishmania: involvement in virulence. J Biol Chem (2006) 281:36257–68. doi:10.1074/jbc.M606256200
118. Leitherer S, Clos J, Liebler-Tenorio EM, Schleicher U, Bogdan C, Soulat D. Characterization of the protein tyrosine phosphatase LmPRL-1 secreted by Leishmania major via the exosome pathway. Infect Immun (2017) 85. doi:10.1128/IAI.00084-17
119. Beresford NJ, Saville C, Bennett HJ, Roberts IS, Tabernero L. A new family of phosphoinositide phosphatases in microorganisms: identification and biochemical analysis. BMC Genomics (2010) 11:457. doi:10.1186/1471-2164-11-457
120. Zhou Y, Messier N, Ouellette M, Rosen BP, Mukhopadhyay R. Leishmania major LmACR2 is a pentavalent antimony reductase that confers sensitivity to the drug pentostam. J Biol Chem (2004) 279:37445–51. doi:10.1074/jbc.M404383200
121. Zhou Y, Bhattacharjee H, Mukhopadhyay R. Bifunctional role of the leishmanial antimonate reductase LmACR2 as a protein tyrosine phosphatase. Mol Biochem Parasitol (2006) 148:161–8. doi:10.1016/j.molbiopara.2006.03.009
122. Mukhopadhyay R, Bisacchi D, Zhou Y, Armirotti A, Bordo D. Structural characterization of the As/Sb reductase LmACR2 from Leishmania major. J Mol Biol (2009) 386:1229–39. doi:10.1016/j.jmb.2008.07.056
123. Bisacchi D, Zhou Y, Rosen BP, Mukhopadhyay R, Bordo D. Crystallization and preliminary crystallographic characterization of LmACR2, an arsenate/antimonate reductase from Leishmania major. Acta Crystallogr Sect F Struct Biol Cryst Commun (2006) 62:976–9. doi:10.1107/S1744309106033537
124. Banerjee C, Sarkar D, Bhaduri A. Ca2+ and calmodulin-dependent protein phosphatase from Leishmania donovani. Parasitology (1999) 118(Pt 6):567–73. doi:10.1017/S0031182099004308
125. Moreno VR, Agüero F, Tekiel V, Sánchez DO. The calcineurin A homologue from Trypanosoma cruzi lacks two important regulatory domains. Acta Trop (2007) 101:80–9. doi:10.1016/j.actatropica.2006.11.008
126. Naderer T, Dandash O, McConville MJ. Calcineurin is required for Leishmania major stress response pathways and for virulence in the mammalian host. Mol Microbiol (2011) 80:471–80. doi:10.1111/j.1365-2958.2011.07584.x
127. Kramer S. The ApaH-like phosphatase TbALPH1 is the major mRNA decapping enzyme of trypanosomes. PLoS Pathog (2017) 13:e1006456. doi:10.1371/journal.ppat.1006456
128. Nandi S, Sarkar D. Partial purification and characterization of a soluble protein phosphatase from Leishmania donovani promastigotes. Mol Cell Biochem (1995) 148:191–8. doi:10.1007/BF00928156
129. Escalona-Montaño AR, Ortiz-Lozano DM, Rojas-Bernabé A, Wilkins-Rodriguez AA, Torres-Guerrero H, Mondragón-Flores R, et al. Leishmania mexicana: promastigotes and amastigotes secrete protein phosphatases and this correlates with the production of inflammatory cytokines in macrophages. Parasitology (2016) 143:1409–20. doi:10.1017/S0031182016000949
130. Kazemi-Rad E, Mohebali M, Khadem-Erfan MB, Saffari M, Raoofian R, Hajjaran H, et al. Identification of antimony resistance markers in Leishmania tropica field isolates through a cDNA-AFLP approach. Exp Parasitol (2013) 135:344–9. doi:10.1016/j.exppara.2013.07.018
131. Szöőr B, Ruberto I, Burchmore R, Matthews KR. A novel phosphatase cascade regulates differentiation in Trypanosoma brucei via a glycosomal signaling pathway. Genes Dev (2010) 24:1306–16. doi:10.1101/gad.570310
132. Szöőr B, Dyer NA, Ruberto I, Acosta-Serrano A, Matthews KR. Independent pathways can transduce the life-cycle differentiation signal in Trypanosoma brucei. PLoS Pathog (2013) 9:e1003689. doi:10.1371/journal.ppat.1003689
133. Hnia K, Vaccari I, Bolino A, Laporte J. Myotubularin phosphoinositide phosphatases: cellular functions and disease pathophysiology. Trends Mol Med (2012) 18:317–27. doi:10.1016/j.molmed.2012.04.004
134. Kastner R, Dussurget O, Archambaud C, Kernbauer E, Soulat D, Cossart P, et al. LipA, a tyrosine and lipid phosphatase involved in the virulence of Listeria monocytogenes. Infect Immun (2011) 79:2489–98. doi:10.1128/IAI.05073-11
135. Christensen SM, Dillon LAL, Carvalho LP, Passos S, Novais FO, Hughitt VK, et al. Meta-transcriptome profiling of the human-Leishmania braziliensis cutaneous lesion. PLoS Negl Trop Dis (2016) 10:e0004992. doi:10.1371/journal.pntd.0004992
136. Machín F, Quevedo O, Ramos-Pérez C, García-Luis J. Cdc14 phosphatase: warning, no delay allowed for chromosome segregation! Curr Genet (2016) 62:7–13. doi:10.1007/s00294-015-0502-1
137. Gottlieb M, Dwyer DM. Identification and partial characterization of an extracellular acid phosphatase activity of Leishmania donovani promastigotes. Mol Cell Biol (1982) 2:76–81. doi:10.1128/MCB.2.1.76
138. Remaley AT, Das S, Campbell PI, LaRocca GM, Pope MT, Glew RH. Characterization of Leishmania donovani acid phosphatases. J Biol Chem (1985) 260:880–6.
139. Das S, Saha AK, Remaley AT, Glew RH, Dowling JN, Kajiyoshi M, et al. Hydrolysis of phosphoproteins and inositol phosphates by cell surface phosphatase of Leishmania donovani. Mol Biochem Parasitol (1986) 20:143–53. doi:10.1016/0166-6851(86)90026-5
140. Freitas-Mesquita AL, Fonseca-de-Souza AL, Meyer-Fernandes JR. Leishmania amazonensis: characterization of an ecto-pyrophosphatase activity. Exp Parasitol (2014) 137:8–13. doi:10.1016/j.exppara.2013.11.008
141. de Almeida-Amaral EE, Belmont-Firpo R, Vannier-Santos MA, Meyer-Fernandes JR. Leishmania amazonensis: characterization of an ecto-phosphatase activity. Exp Parasitol (2006) 114:334–40. doi:10.1016/j.exppara.2006.04.011
142. Rzhetsky A, Nei M. A simple method for evaluating and testing minimum-evolution trees. Mol Biol Evol (1992) 9:945–67.
143. Tamura K, Nei M, Kumar S. Prospects for inferring very large phylogenies by using the neighbor-joining method. Proc Natl Acad Sci U S A (2004) 101:11030–5. doi:10.1073/pnas.0404206101
144. Nei M, Kumar S. Molecular Evolution and Phylogenetics. New York: Oxford University Press (2000).
145. Saitou N, Nei M. The neighbor-joining method: a new method for reconstructing phylogenetic trees. Mol Biol Evol (1987) 4:406–25.
146. Kumar S, Stecher G, Tamura K. MEGA7: molecular evolutionary genetics analysis version 7.0 for bigger datasets. Mol Biol Evol (2016) 33:1870–4. doi:10.1093/molbev/msw054
147. Käll L, Krogh A, Sonnhammer ELL. A combined transmembrane topology and signal peptide prediction method. J Mol Biol (2004) 338:1027–36. doi:10.1016/j.jmb.2004.03.016
148. Shakarian A, Joshi M, Ghedin E, Dwyer D. Molecular dissection of the functional domains of a unique, tartrate-resistant, surface membrane acid phosphatase in the primitive human pathogen Leishmania donovani. J Biol Chem (2002) 277:17994–8001. doi:10.1074/jbc.M200114200
149. Aslett M, Aurrecoechea C, Berriman M, Brestelli J, Brunk BP, Carrington M, et al. TriTrypDB: a functional genomic resource for the trypanosomatidae. Nucleic Acids Res (2010) 38:D457–62. doi:10.1093/nar/gkp851
150. Gottlieb M, Dwyer DM. Protozoan parasite of humans: surface membrane with externally disposed acid phosphatase. Science (1981) 212:939–41. doi:10.1126/science.7233189
151. Menz B, Winter G, Ilg T, Lottspeich F, Overath P. Purification and characterization of a membrane-bound acid phosphatase of Leishmania mexicana. Mol Biochem Parasitol (1991) 47:101–8. doi:10.1016/0166-6851(91)90152-V
152. Aguirre-García MM, Escalona-Montaño AR, Bakalara N, Pérez-Torres A, Gutiérrez-Kobeh L, Becker I. Leishmania major: detection of membrane-bound protein tyrosine phosphatase. Parasitology (2006) 132:641–9. doi:10.1017/S0031182005009595
153. Dillon LAL, Suresh R, Okrah K, Corrada Bravo H, Mosser DM, El-Sayed NM. Simultaneous transcriptional profiling of Leishmania major and its murine macrophage host cell reveals insights into host-pathogen interactions. BMC Genomics (2015) 16:1108. doi:10.1186/s12864-015-2237-2
154. Dillon LAL, Okrah K, Hughitt VK, Suresh R, Li Y, Fernandes MC, et al. Transcriptomic profiling of gene expression and RNA processing during Leishmania major differentiation. Nucleic Acids Res (2015) 43:6799–813. doi:10.1093/nar/gkv656
155. Iantorno SA, Durrant C, Khan A, Sanders MJ, Beverley SM, Warren WC, et al. Gene expression in Leishmania is regulated predominantly by gene dosage. MBio (2017) 8:e1393–1317. doi:10.1128/mBio.01393-17
156. Remaley AT, Kuhns DB, Basford RE, Glew RH, Kaplan SS. Leishmanial phosphatase blocks neutrophil O-2 production. J Biol Chem (1984) 259:11173–5.
157. Remaley AT, Glew RH, Kuhns DB, Basford RE, Waggoner AS, Ernst LA, et al. Leishmania donovani: surface membrane acid phosphatase blocks neutrophil oxidative metabolite production. Exp Parasitol (1985) 60:331–41. doi:10.1016/0014-4894(85)90039-6
158. Papadaki A, Politou AS, Smirlis D, Kotini MP, Kourou K, Papamarcaki T, et al. The Leishmania donovani histidine acid ecto-phosphatase LdMAcP: insight into its structure and function. Biochem J (2015) 467:473–86. doi:10.1042/BJ20141371
159. Benzel I, Weise F, Wiese M. Deletion of the gene for the membrane-bound acid phosphatase of Leishmania mexicana. Mol Biochem Parasitol (2000) 111:77–86. doi:10.1016/S0166-6851(00)00306-6
160. Fernandes ACS, Soares DC, Saraiva EM, Meyer-Fernandes JR, Souto-Padrón T. Different secreted phosphatase activities in Leishmania amazonensis. FEMS Microbiol Lett (2013) 340:117–28. doi:10.1111/1574-6968.12080
161. Doyle PS, Dwyer DM. Leishmania: immunochemical comparison of the secretory (extracellular) acid phosphatases from various species. Exp Parasitol (1993) 77:435–44. doi:10.1006/expr.1993.1103
162. Debrabant A, Joshi MB, Pimenta PFP, Dwyer DM. Generation of Leishmania donovani axenic amastigotes: their growth and biological characteristics. Int J Parasitol (2004) 34:205–17. doi:10.1016/j.ijpara.2003.10.011
163. Lovelace JK, Gottlieb M. Evidence for phosphorylation of the extracellular acid phosphatase of Leishmania donovani. J Protozool (1987) 34:78–9. doi:10.1111/j.1550-7408.1987.tb03136.x
164. Bates PA, Dwyer DM. Biosynthesis and secretion of acid phosphatase by Leishmania donovani promastigotes. Mol Biochem Parasitol (1987) 26:289–96. doi:10.1016/0166-6851(87)90081-8
165. Dermine JF, Scianimanico S, Privé C, Descoteaux A, Desjardins M. Leishmania promastigotes require lipophosphoglycan to actively modulate the fusion properties of phagosomes at an early step of phagocytosis. Cell Microbiol (2000) 2:115–26. doi:10.1046/j.1462-5822.2000.00037.x
166. Ilg T, Stierhof YD, Etges R, Adrian M, Harbecke D, Overath P. Secreted acid phosphatase of Leishmania mexicana: a filamentous phosphoglycoprotein polymer. Proc Natl Acad Sci U S A (1991) 88:8774–8. doi:10.1073/pnas.88.19.8774
167. Stierhof YD, Ilg T, Russell DG, Hohenberg H, Overath P. Characterization of polymer release from the flagellar pocket of Leishmania mexicana promastigotes. J Cell Biol (1994) 125:321–31. doi:10.1083/jcb.125.2.321
168. Wiese M, Ilg T, Lottspeich F, Overath P. Ser/Thr-rich repetitive motifs as targets for phosphoglycan modifications in Leishmania mexicana secreted acid phosphatase. EMBO J (1995) 14:1067–74.
169. Stierhof YD, Wiese M, Ilg T, Overath P, Häner M, Aebi U. Structure of a filamentous phosphoglycoprotein polymer: the secreted acid phosphatase of Leishmania mexicana. J Mol Biol (1998) 282:137–48. doi:10.1006/jmbi.1998.2012
170. Ghouila A, Guerfali FZ, Atri C, Bali A, Attia H, Sghaier RM, et al. Comparative genomics of Tunisian Leishmania major isolates causing human cutaneous leishmaniasis with contrasting clinical severity. Infect Genet Evol (2017) 50:110–20. doi:10.1016/j.meegid.2016.10.029
171. Zhang W-W, Ramasamy G, McCall L-I, Haydock A, Ranasinghe S, Abeygunasekara P, et al. Genetic analysis of Leishmania donovani tropism using a naturally attenuated cutaneous strain. PLoS Pathog (2014) 10:e1004244. doi:10.1371/journal.ppat.1004244
172. Wiese M. A mitogen-activated protein (MAP) kinase homologue of Leishmania mexicana is essential for parasite survival in the infected host. EMBO J (1998) 17:2619–28. doi:10.1093/emboj/17.9.2619
173. Rios P, Li X, Köhn M. Molecular mechanisms of the PRL phosphatases. FEBS J (2013) 280:505–24. doi:10.1111/j.1742-4658.2012.08565.x
174. Lambertz U, Oviedo Ovando ME, Vasconcelos EJR, Unrau PJ, Myler PJ, Reiner NE. Small RNAs derived from tRNAs and rRNAs are highly enriched in exosomes from both old and new world Leishmania providing evidence for conserved exosomal RNA Packaging. BMC Genomics (2015) 16:151. doi:10.1186/s12864-015-1260-7
Keywords: Leishmania, macrophages, protein tyrosine phosphatase, signaling, exosome
Citation: Soulat D and Bogdan C (2017) Function of Macrophage and Parasite Phosphatases in Leishmaniasis. Front. Immunol. 8:1838. doi: 10.3389/fimmu.2017.01838
Received: 23 September 2017; Accepted: 05 December 2017;
Published: 22 December 2017
Edited by:
Uwe Ritter, University of Regensburg, GermanyReviewed by:
Robert Adam Harris, Karolinska Institute (KI), SwedenDaniel Ferreira Feijó, Instituto Gonçalo Moniz (IGM), Brazil
Copyright: © 2017 Soulat and Bogdan. This is an open-access article distributed under the terms of the Creative Commons Attribution License (CC BY). The use, distribution or reproduction in other forums is permitted, provided the original author(s) or licensor are credited and that the original publication in this journal is cited, in accordance with accepted academic practice. No use, distribution or reproduction is permitted which does not comply with these terms.
*Correspondence: Didier Soulat, ZGlkaWVyLnNvdWxhdEB1ay1lcmxhbmdlbi5kZQ==;
Christian Bogdan, Y2hyaXN0aWFuLmJvZ2RhbkB1ay1lcmxhbmdlbi5kZQ==