- 1Department of Medicine, Transplantation Centre and Transplantation Immunopathology Laboratory, Service of Immunology, Centre Hospitalier Universitaire Vaudois, University of Lausanne, Lausanne, Switzerland
- 2Department of Surgery, Transplantation Centre, Centre Hospitalier Universitaire Vaudois, University of Lausanne, Lausanne, Switzerland
- 3Département coeur-vaisseaux, Centre Hospitalier Universitaire Vaudois, Lausanne, Switzerland
- 4Fondazione Cardiocentro Ticino, Swiss Institute of Regenerative Medicine (SIRM), Lugano, Switzerland
Dendritic cells (DCs) as highly efficient antigen-presenting cells are at the interface of innate and adaptive immunity. As such, they are key mediators of immunity and antigen-specific immune tolerance. Due to their functional specialization, research efforts have focused on the characterization of DCs subsets involved in the initiation of immunogenic responses and in the maintenance of tissue homeostasis. Tolerogenic DCs (tolDCs)-based therapies have been designed as promising strategies to prevent and control autoimmune diseases as well as allograft rejection after solid organ transplantation (SOT). Despite successful experimental studies and ongoing phase I/II clinical trials using autologous tolDCs in patients with type 1 diabetes, rheumatoid arthritis, multiple sclerosis, and in SOT recipients, additional basic research will be required to determine the optimal DC subset(s) and conditioning regimens for tolDCs-based treatments in vivo. In this review, we discuss the characteristics of human DCs and recent advances in their classification, as well as the role of DCs in immune regulation and their susceptibility to in vitro or in vivo manipulation for the development of tolerogenic therapies, with a focus on the potential of tolDCs for the treatment of autoimmune diseases and the prevention of allograft rejection after SOT.
Introduction
Dendritic cells (DCs) are at the interface of innate and adaptive immunity and, thus, are key mediators of immunity and tolerance. Importantly, DCs constitute a heterogeneous population that comprises multiple subsets exhibiting distinct functional specializations that vary according to their origin, maturation state, location, and environmental conditions (1, 2). In their immature state, DCs mainly traffic and reside in peripheral tissues where they can capture antigens and process them into major histocompatibility complex (MHC):peptide complexes. DCs undergo maturation not only after microbial infection but also in response to damage-associated molecular patterns (DAMPs) and pro-inflammatory cytokines produced as a result of tissue injury. This is the cornerstone for the initiation of effective adaptive immune responses (3, 4). Extensive experimental data over the years have highlighted the possibility of generating “tolerogenic DCs” (tolDCs) that are maturation-resistant in vitro, express low levels of T-cell costimulatory molecules, and a have a reduced capacity to produce pro-inflammatory cytokines. These tolDCs mediate antigen-specific T-cell hyporesponsiveness and promote the expansion and/or induction of regulatory T cells (Treg). Thus, the potential for tolDCs to dampen immune responses may be used clinically, e.g., in autoimmune diseases and after solid organ transplantation (SOT). In this review, we briefly describe human DC subsets and the immune regulatory mechanisms mediated by these cells. We then discuss how DCs may be manipulated in the perspective of tolerogenic immune therapies.
Human DC Subsets and Functional Specialization
Dendritic cells represent a heterogeneous cell population arising from bone marrow-restricted precursors identified in mice and humans (5). In humans, common DC progenitors give rise to plasmacytoid DCs (pDCs) and intermediate precursors of conventional DCs (pre-cDCs) that are pre-committed to become either CD1c+ (BDCA-1) or CD141+ (BDCA-3) conventional DCs (cDCs) (6). The HLA-DR+CD14−CD11b− fraction of human peripheral blood mononuclear cells (PBMCs) comprises the CD1c+ DC subset (characterized by CD172α and IRF4 expression), the CD141high DC subset (characterized by Clec9A, XCR1, IRF8, and TLR3 expression), and the pDC subset (identified by BDCA-2, BDCA-4, and CD123bright expression) (Table 1).
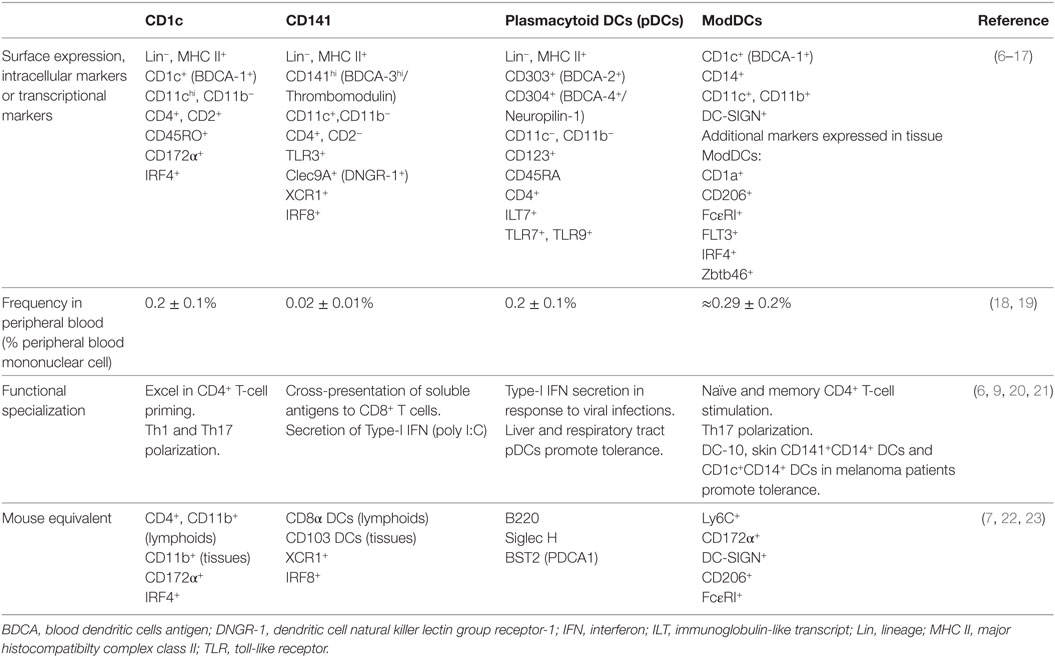
Table 1. Characteristics of blood human dendritic cells (DCs) and monocyte-derived DCs (ModDCs) subsets.
Peripheral Blood DCs
Peripheral blood DCs are likely the precursors of DCs found in peripheral tissues and lymphoid organs. In peripheral tissues, there is evidence for high phenotypic heterogeneity of DCs, contrasting with the well-defined phenotypic expression of blood DCs. In addition to the BDCA population and Langerhans cells, other subpopulations of tissue DCs can be distinguished by the expression of langerin, CD1a, and CD14 (24, 25); however, these markers are promiscuously expressed making it difficult to unambiguously discriminate peripheral tissue DC subpopulations (24). For example, studies in patients with allergic asthma show that most of the lung CD1c+ (BDCA-1+) DCs also express CD141 (BDCA-3) (26). Recently, using gene expression profiling and mass cytometry analysis, a set of lineage-imprinted cell-surface markers, such as CD172α/IRF4 and XCR1/IRF8, were identified, allowing for a better discrimination between CD1c+CD14− DC and CD141+CD14− DC subsets in human tissues (7).
Lymphoid Organs DCs
Multiple subsets of DCs have been found in lymphoid organs; however the distinction between migratory and lymphoid organs-resident DCs still requires further investigation. CD1c+CD14− DC, CD141+CD14− DC, and pDC subsets have been found in the human spleen, tonsils, and axillary and pulmonary lymph nodes (LNs) (8–10, 27). These subsets likely correspond to the resident DC population. In axillary LNs, pDCs have been reported to localize in the paracortex (27). The CD141+CD14− DC subset, characterized by Clec9A expression, is mostly distributed around the LN cortex (inner and outer) (25). By contrast, CD1c+CD14− DCs have been reported to localize within the T-cell zone in close proximity to the B-cell zone (10). Interestingly, in axillary and pulmonary LNs, but not in the spleen and tonsils, a high frequency of a HLA-DR+ cells with the CD141+CD14+DC-SIGN+CD206+CD1c+CCR7int/low phenotype has been reported, suggesting that this subset could be related to a migratory subset. This population is found in the diffuse T-lymphocyte regions of the LN paracortex (10, 27).
Monocyte-Derived DCs (ModDCs)
In vitro experiments have documented that monocytes are important precursors of DCs (28, 29). However, it has been difficult to properly identify ModDCs in vivo due to common features shared by cDCs, monocytes and macrophages. Recent data suggest that a ModDCs subset may exist in humans (10–12, 25, 30). For example, studies in steady-state conditions described a subpopulation of cells expressing CD1c+CD14+HLA-DR+ in both blood and bronchoalveolar lavage fluid (BALF) (10, 18). Although it was demonstrated that blood CD1c+CD14+ cells have monocytic features, these cells have increased antigen-presenting ability and a different gene signature compared to monocytes (18). Interestingly, in non-diseased lung tissue CD1c+CD14+ populations were shown to be enriched for the gene signatures of ModDCs described in the literature, which includes the expression of ZBTB46, IRF4, and FLT3 genes (10). During inflammation, CD1c+CD14+ cells have been reported in the BALF from sarcoidosis patients co-expressing CD141, CD123, and DC-SIGN, or in synovial fluid from rheumatoid arthritis (RA) patients and carcinomatous ascites from untreated cancer patients co-expressing CD1a, FcεRI, CD172a, and CD206 (11, 12). These cells were enriched for the ModDC signature and functionally ModDC from ascites showed an important capacity to polarize naive T cells into Th17 cells as well as to stimulate memory CD4 T cells to produce IL-17 (11).
In the past few years, additional DC subsets were associated with the induction of immune tolerance; however, their precise ontogeny and phenotype remains to be fully established. Gregory and co-workers described a DC subset expressing HLA-DR+CD14+CD16+ receptors in human blood, which was able to induce type 1 regulatory T (Tr1) cells through the release of IL-10; hence, its name DC-10 (31). Furthermore, the presence of a DC subset expressing HLA-DR+CD141+CD14+ was reported in skin dermis. This subset exhibited a potent inhibitory activity on skin inflammation.
Functional Specialization of DCs
In terms of function, DCs can exhibit an immature phenotype at steady-state or a mature phenotype upon exposure to inflammatory stimuli. Immature DCs have a unique immune surveillance function. At this stage, DCs express low levels of MHC and costimulatory molecules such as CD80/B7.1, CD86/B7.2, CD40, OX40L, inducible T-cell costimulatory ligand, as well as low expression of adhesion molecules such as intercellular adhesion molecule-1 (ICAM-1/CD54) (32). Interestingly, at steady-state tissue CD1c+CD14− DCs exhibit a higher activation state, e.g., higher expression levels of CD80, CD83, CD86, and CD40 compared with their blood counterparts (22, 30).
Quiescent immature DCs can mature and become activated in local tissues in the presence of pathogen-associated molecular patterns or DAMPs in the context of sterile injury (e.g., autoimmunity or ischemia/reperfusion) and local inflammatory mediators (IFN-α, IL-1β, IL-6, TNF-α, or CD40L/CD154). Within the context of this maturation process, DC function is regulated by a core set of genes controlled by NF-κB and IFN-mediated signaling (33). In this process, immature DCs evolve from an antigen-capturing mode to an antigen-processing and antigen-presenting mode by upregulating MHC molecules and costimulatory molecules along with chemokine receptors. This allows them to migrate to specialized lymphoid organs, release the corresponding polarizing cytokines, and initiate specific adaptive immune responses.
Regarding the fate and function of human DCs, both unstimulated CD1c+CD14− and CD141+CD14− DCs from blood, non-lymphoid, and lymphoid tissues were shown to be more immunogenic than pDCs, with an increased capacity to process and present soluble foreign antigens, including transplant-derived alloantigens, as immunogenic MHC:peptide complexes to CD4+ T cells (25, 34–36). It has been reported that both blood CD1c+ DCs and CD141+ DCs efficiently induce Th1 polarization in allogeneic co-culture assays, the latter with increased release of IFN-γ upon maturation (9). CD141+ DCs were also shown to be more efficient at inducing Th2 cells compared to CD1c+ DCs (20). By contrast, both CD1c+ and CD141+ DCs derived from lymphoid tissues efficiently induced Th1 and Th2 responses (21). In lung tissues, CD1c+ DCs were shown to have a great capacity to induce Th17 responses following A. fumigatus challenge (37). In addition to their capacity to induce effector CD4+ T cells, all DC subsets isolated from lymphoid tissues were able to efficiently cross-present soluble antigens to CD8+ T lymphocytes (21). CD141+ DCs are referred to as “human cross-presenting DCs” due to their functional homology with mouse CD8α+ DCs (38, 39), in particular with respect to the expression of TLR3 which promotes cross-priming and is required for the production of large amounts of IFN-λ upon TLR3 ligation (13).
In comparison to cDCs, pDCs have a similar distribution in peripheral blood and lymphoid organs, but are present at lower numbers in tissues (30, 40). In the immature state, pDCs express lower levels of costimulatory molecules but multiple pattern recognition receptors that are important for type-I IFN secretion, including intracellular TLR7 and TLR9 (14). In the presence of infectious or inflammatory stimuli, pDCs traffic to lymphoid organs and sites of inflammation. While their role was first described in response to viral infections via the recognition of nucleic acids, tissue-resident gut and airway pDCs have been shown to exert a pivotal role in oral and mucosal tolerance (15, 16, 41). In experimental mouse models of SOT as well as in clinical liver transplantation, pDCs were associated with the generation of alloantigen-specific Treg promoting prolonged allograft survival (42–45). Activated pDCs could also induce CD8+ Treg in in vitro co-cultures (46).
Overall, these studies highlight the diverse responses of DCs depending on their origin. Bona fide cDC were demonstrated to have an inherent capacity to induce immunogenic responses, while pDCs and some immature ModDC subsets participate in tolerance induction. In humans, however, the functional specialization of DCs in the polarization of T cells appears to be less sharply defined compared to mice. The nature and the intensity of the stimuli, as well as the local environment, play an important role in determining the functional specialization of human DCs.
Mechanisms of Immune Regulation by DCs
Dendritic cells play an important role in the maintenance of immune homeostasis and self-tolerance under steady-state conditions. Their significant role in the induction and maintenance of tolerance has been demonstrated in experimental models. Constitutive or conditional depletion of cDCs was shown to break self-tolerance of CD4+ T cells, leading to spontaneous development of lethal autoimmunity manifested by splenomegaly, neutrophilia, autoantibody formation, and an increased frequency of Th1 and Th17 effector cells (47, 48).
Surface Molecules Expressed on tolDCs
In the absence of local inflammation, DCs remain immature with low surface expression of MHC class II and costimulatory molecules, reflecting their participation in the maintenance of peripheral immune tolerance. Indeed, some DC subsets, such as CD103+ DCs in mice and pDCs, blood DC-10, and skin CD141+CD14+ DCs in human, exhibit inherent tolerogenic properties including the ability to induce Treg and/or promote T-cell hyporesponsivness to antigenic stimuli (15, 31, 49, 50). In addition to low expression of MHC class II and costimulatory molecules, tolDCs overexpress inhibitory molecules such as HLA-G, programmed death ligand (PD-L)-1 and PD-L2, and galectins that contribute to their tolerogenic potential. HLA-G is a non-classical MHC class I antigen that plays an important role in materno-fetal tolerance. Through interactions with inhibitory receptors expressed on maternal NK cells (killer cell immunoglobulin-like receptor, KIR) and T cells (ILT2 and ILT4), the expression of HLA-G on fetal cells protects them against maternal alloreactive cytotoxic cells (51). HLA-G engagement of the human inhibitory receptor ILT4 overexpressed on DCs in transgenic mice promoted long-term survival of allogeneic skin grafts, in part as a result of the downregulation of MHC class II and costimulatory molecules, leading to the induction of Treg and hyporesponsiveness of the alloreactive T-cell repertoire (52). Expression levels of PD-L1 and PD-L2 on DCs increase during DC maturation. These ligands can interact with the inhibitory receptor PD-1 expressed on activated T cells and Treg, thus contributing to T-cell homeostasis (53). Galectins have been identified as important regulators of T cells and DCs (54–56). Galectin-1 was shown to inhibit T-cell effector functions by promoting growth arrest and apoptosis of activated T cells (57, 58), and by blocking pro-inflammatory cytokines secretion by DCs (59). Moreover, galectins are overexpressed in the microenvironment of tumors and have been implicated in their immune escape. In in vivo models, DCs constitutively expressing galectin-1 delayed the onset of autoimmune diabetes in mice (60). Conversely, galectin-1-deficient mice experienced accelerated rejection of skin allografts (61).
Immunomodulatory Molecules Secreted by tolDCs
Tolerogenic DCs were shown to secrete molecules, such as transforming growth factor-beta (TGF-β), IL-10, and indoleamine 2,3-dioxygenase (IDO), which favor a tolerogenic environment and the induction and/or expansion of Treg. TGF-β is a pleiotrophic cytokine involved in multiple cellular functions, including growth, differentiation, proliferation, remodeling, apoptosis, and immune homeostasis. TGF-β is secreted in a latent form complexed with latent TGF-β binding protein and latency-associated peptide. tolDCs were shown to play a crucial role in both the release of TGF-β and activation of the latent TGF-β protein complex (62, 63). TGF-β has been also involved in the induction of Foxp3 expression and peripheral conversion of conventional naïve CD4+ T cells into induced Treg (iTreg) in the presence of IL-2 (64, 65). Through their constitutively high expression of the inhibitory receptor cytotoxic T-lymphocyte antigen-4 (CTLA-4/CD152), Foxp3+ Treg ligate B7.1/2 expressed on mature DCs and outcompete costimulatory CD28 unregulated on effector T cells (Teff) (66). The interaction between B7.1/2 and CTLA-4 was shown to promote the expression of IDO by DCs, a potent regulatory molecule that catalyzes the degradation of tryptophan required for Teff functions (67–72). In addition, tryptophan catabolites, such as kynurenine, quinolinic acid, and 3-hydroxyanthranilic acid exhibit direct immunosuppressive properties (73, 74).
Function of tolDCs
Mouse CD103+ DCs as well as both human and mouse pDCs were shown to mediate oral tolerance through an IDO-dependent mechanism (49). In human blood, DC-10 induce and expand Tr1 cells through the release of IL-10 and TGF-β (31, 75). DC-10 identified as CD11b+CD11c+CD14+CD16+CD83+HLA-DR+ cells that do not express CD1a and CD1c. Furthermore, DC-10 expresses the inhibitory receptors ILT2, ILT3, ILT4, and HLA-G. Despite concomitant high surface levels of costimulatory molecules (CD40, CD80, and CD86), DC-10 exhibit potent tolerogenic activity (31). Another DC population characterized by CD1c+CD14+CD16− expression was found in the blood of melanoma patients and exhibited immunosuppressive functions by suppressing T-cell proliferation in an antigen-specific manner. It was suggested that this DC subtype modulated T-cell responses through the expression of PD-L1 (18). A similar phenotype was described in minced lung tissues. The frequency of the CD1c+ subset (including the CD1c+CD14+ fraction) was increased in patients with chronic obstructive pulmonary disease, suggesting that these cells may be involved in the enhanced susceptibility of these patients to infections. Indeed, this subset favored the generation of IL-10-secreting CD4+ T cells and mediated immunosuppression through IL-10, IL-27, and ICOS-L (76). In skin dermis, resident CD141+CD14+ DCs were shown to produce large amounts of IL-10 and were able to induce Treg (50).
Besides their role in controlling peripheral immune responses, DCs play a role in the maintenance of central tolerance, as trafficking peripheral DCs can home to the thymus and promote negative selection of antigen-reactive T cells, thus contributing to a safe peripheral T-cell repertoire (77, 78). By presenting antigens directly within the thymus, DCs and particularly resident pDCs influence the generation of natural Foxp3+ Treg, a process mediated by the IL-7-related molecule, thymic stromal lymphopoietin (TSLP), which is secreted in the thymic medulla (79–81).
Generation of tolDCs
Dendritic cell-based therapeutic approaches are being explored with the aim to reestablish self-tolerance in autoimmune diseases, and to promote alloimmune tolerance after SOT. Several strategies for the generation of tolDCs are being explored (Figure 1). These include treatment with pharmacologic agents or cocktails of immunomodulatory cytokines, genetic engineering, and exposure to apoptotic cells. Research groups have developed protocols to generate and expand antigen-specific tolDCs in vitro. Most of these in vitro conditioning regimens aim to stabilize the immature state of DCs, even in the presence of strong inflammatory challenges [e.g., lipopolysaccharide (LPS)]. The resulting tolDCs also express and/or secrete immunomodulatory molecules that favor the development and expansion of Treg (82, 83). After adoptive transfer in vivo, maturation-resistant tolDCs may, therefore, promote peripheral tolerance mainly by inducing antigen-specific T-cell hyporesponsiveness and an immuno-regulatory microenvironment.
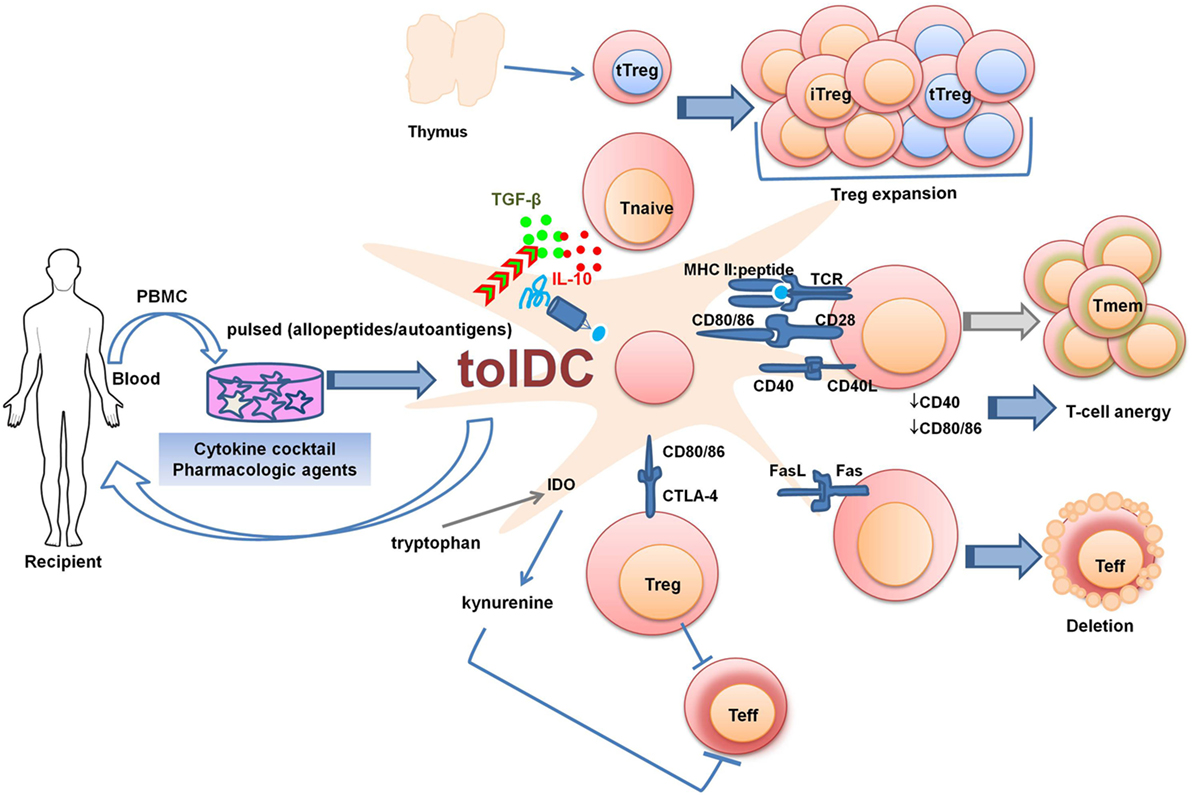
Figure 1. Strategies to generate tolDCs for clinical therapeutics. PBMCs or bone marrow-derived cells can be differentiated into tolDCs in the presence of pharmacologic agents and immunomodulatory cytokines. The generated tolDCs are either donor-derived (in the case of living-donor transplantation) or autologous (in the case of autoimmune diseases or transplantation) and can be further pulsed in vitro with specific antigens (peptides, donor cell lysates, apoptotic cells). tolDCs can regulate Teff responses by various mechanisms: 1. Fas/FasL pathway-mediated deletion; 2. Production of IDO which degrades the essential amino acid tryptophan through kynurenine pathway, causing starvation of Teff. The production of IDO is favored by reversed signaling via interaction between CD80/CD86 on DCs and CTLA-4 on regulatory T cell (Treg). 3. Surface expression of inhibitory molecules and secretion of regulatory mediators. Abbreviations: CTLA-4, cytotoxic T-lymphocyte antigen-4; IDO, indoleamine 2,3-dioxygenase; iTreg, induced regulatory T cell; PBMC, peripheral blood mononuclear cells; tolDC, tolerogenic dendritic cell; Teff, effector T cell; Tmem, memory T cell; Tnaive, naïve T cell; tTreg, thymic-derived regulatory T cell.
Pharmacologic Interventions for tolDC Induction
Various pharmacological agents have been used to generate tolDCs, including immunosuppressive drugs, cyclic AMP inducers, chemicals, cytokines, and growth factors (Table 2) (84, 85). Many of these agents were primarily studied for their inhibitory effects on T-cell activation and proliferation. As such, some of them are currently used in the clinical treatment of autoimmune diseases and in the prevention of allograft rejection after SOT.
Dexamethasone, a potent immunosuppressant, blocks the differentiation and maturation of DCs and enhances their death by apoptosis (92, 109). Using rat bone marrow-derived DCs and human GM-CSF/IL-4-induced ModDCs, we demonstrated that pretreatment with dexamethasone-induced selective expansion of Treg and T-cell alloantigen-specific hyporesponsiveness in re-challenge experiments (110). Dexamethasone was reported to have synergistic effects with other drugs to induce tolDC, in particular 1,25-dihydroxyvitamin D3 (VitD3) (111). While traditionally known for its role in the regulation of calcium and bone homeostasis, VitD3 and its receptor were also described to regulate innate and adaptive immune responses. Exposure to VitD3 inhibited the expression of MHC class II, CD80, and CD86 on DCs with a high ratio of PD-L1/CD86, while reducing the production of pro-inflammatory cytokines, such as IL-12 and IL-23, and increasing that of TGF-β and IL-10. Moreover, VitD3 favored Treg development and blocked B-cell proliferation and differentiation toward antibody-producing plasma cells (112).
Inhibitors of the mammalian target of rapamycine (mTOR) pathway engage FK506-binding protein 12 forming a complex that blocks mTOR, but not calcineurin, resulting in non-specific inhibition of cell cycle progression and, therefore, of T- and B-cell proliferation. In vitro assays, together with experimental and clinical data, suggest that immunosuppression based on mTOR-inhibitors may favor the induction of peripheral tolerance. In rodent models, the adoptive transfer of rapamycin-conditioned alloantigen-pulsed DCs resulted in prolonged cardiac and skin allograft survival (87, 113). Moreover, rapamycin was shown to facilitate peripheral deletion of alloreactive Teff by promoting activation-induced cell death in experimental transplantation models, while selectively expanding human Foxp3+ Treg and Tr1 cells both in vitro and in vivo (114–117).
Various other immunosuppressive drugs and biologic agents were described to generate tolDCs in vitro including mycophenolic acid (MPA) formulations (90, 118), deoxyspergualin (DSG) and its analogs (93), aspirin (98, 99), retinoic acid (117), and prostaglandin E2 (119). These substances mainly interfere with NF-κB pathway-mediated DCs maturation and the capacity of DCs to produce IL-12p70 (84, 85).
Bone marrow-derived DCs exposed in vitro to the immunomodulatory cytokines IL-10, TGF-β, or low-dose GM-CSF in the absence of IL-4 exhibit low expression levels of costimulatory molecules and pro-inflammatory cytokines with minor changes in MHC class-I and -II molecules. These conditioned DCs were less immunogenic when co-cultured with CD4+ and CD8+ T cells, while promoting the expansion of Treg (both natural Treg and iTreg) and antigen-specific T-cell hyporesponsiveness in vivo upon re-challenge (108, 120).
Some of these agents also directly promote the differentiation of tolDCs in vivo. For example, local or systemic presence of cytokines such as IL-10, TGF-β, and even IFN-γ-induced differentiation of monocytes into tolDCs and promoted FoxP3+ Treg (121). The eye is a known locally immune-privileged site and the aqueous humor constitutively contains molecules that maintain DCs in an immature state, such as TGF-β2, IDO, the neuropeptide α-melanocyte stimulating hormone (α-MSH) and FasL (122). The epithelium also plays a critical role in dampening inflammation through the release of epithelial-derived factors, including prostaglandin E2, TSLP, retinoic acid, and TGF-β, which are able to promote tolDC (119, 123).
Cobalt protoporphyrin is an inducer of heme oxygenase (HO)-1, an intracellular enzyme that catalyzes the degradation of heme, resulting in the production of biliverdin and carbon monoxide. HO-1 expression is induced by local oxidative stress. Experimentally, HO-1 upregulation was protective in the context of inflammatory processes and after allogeneic SOT (124). Besides maintaining HO-1 expression on human DC, cobalt protoporphyrin prevents their maturation and promotes the secretion of regulatory cytokines (124, 125). Interestingly, HO-1 is also able to inhibit the activation of T, B, and NK cells (126).
Genetic Engineering of DCs
We and others have used gene transfer technology to generate tolDCs via increased expression of immunomodulatory molecules such as IL-10, TGF-β, CTLA-4, IDO, PD-L1, or ligands for receptors resulting in T-cell deletion such as CD95/Fas (TNF-family related death receptor) and TNF-related apoptosis-inducing ligand (127–129). Using gene therapy approaches, recombinant adenovirus vectors typically achieve great transfection efficiencies but are limited by pro-inflammatory effects leading to DCs maturation (130). Methods using recombinant retrovirus vectors and, recently, non-viral gene transfer methods such as nucleoporation may induce lower degrees of DCs maturation (131). As an example, genetically engineered DCs over-expressing IDO regulated T-cell alloresponses in vitro, while IDO adenovirus-mediated gene transfer into the donor heart attenuated acute rejection of MHC-mismatched cardiac allografts in rats (132). Compared to pharmacological conditioning, genetic engineering of DCs using retroviral or lentiviral vectors offers the advantage of a potentially more stable cell phenotype and function in vivo. Another interesting approach that was described is the in vivo transfer of antigen-encoding bone marrow progenitor cells which, at steady-state, prevent antigen-specific sensitization and promote T-cell tolerance mostly by deletional mechanisms (133–135).
DC Exposure to Apoptotic Cells
Exposure of DCs to early apoptotic cells down-modulates their stimulatory functions (136). DC internalization of apoptotic-cells-associated molecular patterns selectively leads to decreased production of pro-inflammatory cytokines (e.g., IL-1, TNF-α, IL-6, and IL-12), while enhancing the secretion of IL-10 and TGF-β (137). This process also limits upregulation of MHC class II and costimulatory molecules such as CD40, CD80 and CD86, hence maintaining DCs in an immature state (138). This mechanism contributes to self-tolerance and was exploited to induce tolerance to alloantigens in SOT. Following intravenous injection, donor allogeneic apoptotic cells were rapidly internalized in the spleen by red pulp macrophages and marginal zone DCs and were able to prolong cardiac allograft survival in rodents (139). Interestingly, T-cell depleting therapies used in clinical SOT, such as anti-CD3 or anti-CD52 monoclonal antibodies, were shown to induce T-cell apoptosis in vivo. This effect was associated with TGF-β secretion by DCs and subsequent expansion of Treg (140). Thus, administration of apoptotic cells or direct induction of apoptosis in vivo could be used to promote the generation of tolDCs in vivo. Of note, the induction of apoptosis in vivo must be carefully controlled to prevent simultaneous activation of the necrotic cell death mechanism and subsequent release of necrotic cell-associated antigens. These antigens were demonstrated to be able to stimulate CD141+CD14− DCs through the Clec9A receptor favoring antigen cross-presentation and, hence, CD8+ T-cell responses (8).
Therapeutic Use of tolDCs in Autoimmune Diseases
In Europe and North America, 5% of adults, of whom two-thirds are females, suffer from autoimmune diseases, the most prevalent pathologies being type 1 diabetes (T1D), psoriasis, RA, inflammatory bowel disease (IBD), and multiple sclerosis (MS).
Type 1 Diabetes
Type 1 diabetes is due to a breakdown of self-tolerance and is mainly orchestrated by CD4+ and CD8+ autoreactive T cells that activate B cells, resulting in the production of autoantibodies specific for pancreatic islets β-cell antigens with progressive immune-mediated destruction of the β-cell mass and insulin insufficiency (141). Insulin treatment increases the life expectancy of T1D patients; however, it often fails to prevent T1D-associated cardiovascular and renal complications with increased morbidity and mortality. This opens the way to immune-based interventions aiming at restoring immune tolerance and preventing early T1D either by targeting autoreactive T cells (142) or by modifying the immunogenicity of DC. As T1D is a progressive and not a relapsing-remitting autoimmune disease, there is only a window of opportunity treatment period at the onset of the disease, in order to reinstitute self-tolerance and preserve the function of the existing β-cells mass.
In non-obese diabetic (NOD) mice, GM-CSF treatment prevented the development of diabetes primarily by inducing tolDCs and Treg (143). Another therapeutic option for T1D consists of transplantation of pancreatic islets or whole pancreas; however, such approaches require long-term immunosuppression to prevent allograft rejection and reoccurrence of autoimmunity. In an experimental model of syngeneic pancreatic islets transplantation in NOD mice, both transient TGF-β expression within islets and transplantation of islets grafts containing TGF-β-conditioned tolDCs reduced the activation of islet autoantigen-specific T cells in graft-draining LNs, resulting in prolonged graft survival (144). These results support the notion that TGF-β-induced tolDCs could be an effective strategy to restore peripheral tolerance within the context of an established autoimmune disease. Aside from generating tolDC, TGF-β promoted the survival of thymic-derived Treg and the differentiation of iTreg (64, 145, 146).
Other strategies using tolDCs for the prevention or treatment of T1D currently are being explored in experimental models. Antisense oligonucleotides have been used to specifically downregulate costimulatory molecules, resulting in DCs with an immature phenotype. A single injection of bone marrow-derived DCs engineered ex vivo with a mixture of antisense oligonucleotides targeting the CD40, CD80, and CD86 primary transcripts significantly delayed the onset of diabetes in syngeneic NOD recipients. The beneficial effect of these tolDCs was partly mediated by an increase in Treg (147). Based on these encouraging experimental data, efforts have been made at translating the use of autologous tolDCs in patients with new-onset T1D, aiming to prevent disease progression, or even to revert established disease (148, 149). A phase I clinical study showed that intradermal injection of autologous monocyte-derived costimulation-impaired tolDCs (10 × 106 cells every 2 weeks for a total of 4 administrations), treated ex vivo with antisense oligonucleotides, was safe and well tolerated in patients with established T1D (150). A phase II follow-up clinical trial (ClinicalTrials.gov identifier NCT02354911) using DCs isolated from patients with recent-onset T1D is ongoing. Potential therapeutic success will be evaluated through the improvement of the glycemic control as evidence for a preserved β-cell mass. A similar clinical study using costimulation-impaired tolDCs is currently registered (identifier NCT01947569). This clinical trial includes a sequential open-label, phase-IB safety assessment and a randomized, double-blind, phase-IIA efficacy trial aiming at maintaining and improving residual β-cell function in new-onset T1D patients.
Psoriasis
Psoriasis is a chronic inflammatory skin disease mainly characterized by abnormal keratinocyte proliferation and differentiation causing thickening of the epidermis (151). The psoriatic skin shows a prominent infiltration of neutrophils in the epidermis, together with macrophages, DCs, and T cells in the dermis. The successful use of cyclosporine A, a drug that inhibits early T-cell activation by blocking the TCR-downstream calcium–calcineurin pathway, highlights the role of T cells in the pathogenesis of the disease (152). Psoriasis has been associated with impaired Treg suppressive functions, resulting in overproduction of pro-inflammatory cytokines such as TNF-α and IFN-γ, as well as IL-17 and IL-22 produced by Th1 and Th17 effector cells, respectively (151, 153–155). The neuropeptide α-MSH is a well known mediator of skin pigmentation and has recently been shown to exert anti-inflammatory and immunomodulatory activities (106). Treatment with α-MSH ameliorated psoriasis-like skin inflammation, in part by suppressing the proliferation and effector function of Th17 cells. The beneficial effect of α-MSH was shown to be mediated by tolDCs and functional iTreg (107).
Rheumatoid Arthritis
Rheumatoid arthritis, an autoimmune disease associated with chronic joint inflammation and destruction, is characterized by infiltration of innate immune cells (neutrophils, monocytes, NK cells and DCs) as well as T and B cells in the synovial compartment (156). The current treatment of RA includes immunosuppressive drugs such as corticosteroids, cytokine antagonists (anti-TNF-α), costimulation blockade (CTLA-4 Ig), and B-cell depleting monoclonal antibodies. More recently, the potential of DC-mediated immunomodulation for the treatment of RA was investigated. Clinical-grade tolDCs have been generated from monocytes of patients with RA by conditioning them ex vivo with VitD3 and dexamethasone. The resulting tolDCs exhibited reduced costimulatory molecules expression, low production of pro-inflammatory cytokines, and impaired capacity to stimulate antigen-specific T cells. Importantly, the phenotype and functional characteristics of tolDCs generated from RA patients were comparable to those generated from healthy individuals. These tolDCs remained stable in the absence of immunosuppressive drugs even after further challenge with pro-inflammatory mediators (157). Interestingly, the tolDCs exhibited high cell-surface expression of TLR2 compared to mature immunogenic DC. The use of this marker should be considered in future immunotherapeutic protocols to assess the quality and stability of tolDCs produced ex vivo. An ongoing registered phase I randomized, placebo-controlled trial (identifier NCT01352858, AutoDECRA) aims to generate autologous tolDCs to be injected (single dose) into the knee joint of patients suffering from RA. This clinical study will evaluate the effect of injected tolDCs on both the local and the systemic disease activity.
Inflammatory Bowel Disease
The gut mucosa is constantly exposed to food antigens, pathogens, and commensal microorganisms, and holds the largest mass of lymphoid tissues in the body. The interplay between the intestinal epithelium and the local innate and adaptive immune system is crucial to the maintenance of immune homeostasis and oral tolerance. IBD (Crohn’s disease and ulcerative colitis) is mainly a consequence of loss of peripheral tolerance to otherwise harmless bacterial flora with dysregulated T-cell function in response to local intestinal antigens (158). Current treatments include corticosteroids, azathioprine, and 6-mercaptopurine, as well as anti-TNF-α and anti-α4β7 integrin therapies in severe cases. T-cell activation and effector function is dependent on the microenvironment in which antigen presentation occurs; hence, tolDCs may have a potential for reestablishing intestinal immune regulation (159). CD103+ DCs are found in the human gut under normal conditions (160). These cells express low levels of CD40, TLR2 and TLR4, secrete IL-10 but not IL-12, and produce retinoic acid and IDO that promote the differentiation of iTreg and local immune regulation (49, 161). Peripheral tissue-resident pDCs exert a pivotal role in oral and mucosal tolerance (15, 16). An aberrant pDC distribution and effector function was described in the mesenteric LNs and inflamed mucosa of patients with Crohn’s disease compared to healthy individuals (162). Moreover, immature peripheral blood pDCs and cDCs were reduced during flares in IBD patients (163). A phase I randomized clinical study (identifier NCT02622763 TolDecCDintra) currently evaluates the safety and clinical efficacy of autologous tolerogenic ModDCs injected into the intestinal lesions identified by endoscopy in patients with refractory Crohn’s disease.
G-CSF therapy has been shown to be beneficial in Crohn’s disease patients. The benefit was associated with increased numbers of pDCs in the gut mucosa and induction of IL-10 production (164). IL-10 is a crucial immunoregulatory cytokine in the gut, as documented by severe spontaneous IBD in IL-10-knockout mice (165). Protocols are under development to produce tolDCs under clinical-grade conditions for IBD patients by conditioning ModDCs with IL-10, together with a cocktail of other cytokines and prostaglandin E2 (166). The generated tolDCs display a semi-mature phenotype (intermediate expression of CD80 and CD86, MHC class II low), produce IL-10 with low levels of IL-12p70, IL-23, and TNF-α, and remain stable even in pro-inflammatory conditions. These data suggest that the strategy based on using autologous DCs (derived from patients with autoimmune diseases) may indeed be feasible for future immune therapies. Although the initial monocyte population is selected from patients with an overt inflammatory disease, the cells can be conditioned to acquire beneficial tolerogenic properties ex vivo.
Multiple Sclerosis
Multiple sclerosis, a chronic inflammatory disease of the central nervous system (CNS), is predominantly a T-cell-mediated autoimmune disease characterized by leukocyte infiltration into the CNS, demyelination, and axonal loss. Besides current strategies targeting T and/or B cells, tolDCs may represent a potential therapeutic approach. Myelin peptide-loaded tolDCs were generated ex vivo, exhibiting a stable semi-mature phenotype and an anti-inflammatory cytokine profile. These tolDCs induced antigen-specific hyporesponsiveness in myelin-reactive T cells isolated from relapsing-remitting MS patients (167). IFN-β is an immunomodulatory agent used in the treatment of MS (168). Using healthy donors as well as MS patients PBMCs, it was shown that in vitro treatment with IFN-β enhanced PD-L1 expression on monocytes and DCs, inhibited antigen-specific CD4+ T-cell activation, and increased Treg numbers. In addition, serial in vivo measurements in MS patients before and 6 months after initiation of IFN-β therapy revealed a significant increase in PD-L1 mRNA (169). Sex hormones such as estrogens can modulate immune responses, contributing to the observed difference in the incidence of autoimmune diseases between males and females. Although the overall incidence of autoimmune diseases is higher in women compared with men, estrogens were protective in the experimental autoimmune encephalomyelitis (EAE) animal model of MS, even during pregnancy (170, 171). In vivo exposure to estriol (E3), a pregnancy-specific estrogen, induced tolDCs (E3 tolDC) characterized by increased expression of the inhibitory molecules PD-L1, PD-L2, B7-H3, and B7-H4, as well as mediators such as IL-10 and TGF-β, along with decreased expression of IL-12, IL-23, and IL-6. The transfer of E3 tolDCs to mice prior to active induction of EAE prevented the development of the disease. The protective effect was associated with immune deviation from pathogenic Th1/Th17 cells to a Th2 response (172). Two phase I clinical trials are currently registered on the ClinicalTrials.gov website, assessing the feasibility and safety of tolDCs loaded with myelin peptide in patients with MS (identifier NCT02618902 and NCT02903537). The TOLERVIT-MS (NCT02903537) trial involves VitD3-induced tolDCs used at increasing doses, starting from 5 × 106 cells. Interestingly, different routes of administration will be explored for optimal efficacy, such as intravenous, intradermal or direct intranodal cell injection into cervical LNs.
Therapeutic Potential of tolDCs in SOT
In the absence of adequate immunosuppression after SOT, the recognition of donor alloantigens by recipient T cells initiates a strong immune response leading to alloimmunization and allograft rejection. As T cells play a central role in alloresponses, immunosuppressive regimens have been historically developed to target T cells, whereas recent attention has also been devoted to the roles of B cells and alloantibodies (173). The development of immunosuppressive drugs has led to decreased rates of acute rejection after SOT, but their long-term administration is associated with side-effects including cardiovascular and renal toxicities, as well as infections and tumors (174, 175). Moreover, current regimens have limited effects on T- and B-cell memory responses and may interfere with the induction and expansion of donor-specific Treg (83, 176). Therefore, inducing sustained donor-specific tolerance with minimal drug exposure remains an important goal to improve long-term outcomes in transplantation medicine.
Compared to immune responses to autoantigens or pathogens, SOT constitutes a unique situation whereby DCs present antigens to alloreactive T cells through three different mechanisms: the direct, indirect and semi-direct pathways (177). Recipient T cells can be activated by donor antigens either as intact allogeneic MHC:peptide complexes presented by donor mature DCs that have migrated out of the allograft (direct allorecognition) or as donor-derived MHC:peptide complexes that have been processed and presented by recipient DCs (indirect allorecognition) (178, 179). The semi-direct pathway involves the presentation by recipient DCs of intact donor MHC:peptide complexes that have been captured from donor cell membranes or exosomes (177). Therefore, DCs from either donor or recipient origin can be considered for the development of immunotherapeutic protocols in SOT.
Donor-Derived tolDC
Experimental models have illustrated the potential of donor-derived tolDCs to promote peripheral transplantation tolerance through the induction of donor-specific T-cell hyporesponsiveness, deletion, and/or regulation of alloreactive T cells (180–182). Costimulation-deficient tolDCs generated from donor bone marrow in the presence of GM-CSF, without IL-4, significantly prolonged the survival of MHC-mismatched heart allografts in mice when delivered (2 × 106 cells intravenously) 1 week before transplantation. The effect was only partially antigen-specific, as third-party tolDCs also prolonged graft survival, albeit to a lesser extent (median graft survival time 22 vs. 16.5 days, respectively; vs. 9.5 days in control non-treated mice) (180). However, in vivo maturation of the injected tolDC occurred, as evidenced by upregulation of CD80 and CD86, partially explaining the limited efficacy of tolDCs in vivo. Based on these results, a newer approach evaluated donor-derived tolDCs generated in the presence of GM-CSF and TGF-β, delivered in conjunction with CD40–CD40L costimulation blockade 1 week before transplantation. This strategy resulted in extended allograft survival (181). In a preclinical non-human primate (NHP) model, the infusion of donor-derived tolerogenic ModDCs (3.5–10 × 106 cells/kg) in combination with B7-CD28 costimulation blockade (CTLA-4 Ig given at day −7 and up to 8 weeks after transplantation) and rapamycin (started on day −2 and tapered over 6 months) significantly prolonged renal allograft survival (median graft survival time 113.5 vs. 39.5 days in controls) (86). In this study, tolDCs generated in vitro with VitD3 and IL-10 expressed low MHC class II and costimulatory molecules, high levels of PD-L1, and were resistant to inflammation-induced maturation. Ex vivo immune monitoring demonstrated regulation of donor-reactive memory T cells in tolDC-treated NHP compared to controls. Importantly, no adverse events, and particularly no significant allosensitization, were observed in the recipients.
Donor-derived tolDCs could also contribute to the induction of donor-specific central tolerance after SOT. A recent study evaluated the thymus-homing ability of DCs and their tolerogenic function. FMS-related tyrosine kinase 3 ligand (Flt3L) is a cytokine that synergizes with other growth factors to stimulate the proliferation and differentiation of hematopoietic progenitor cells. Flt3L-induced bone marrow-derived DCs (FLDCs), but not GM-CSF-induced DCs, were able to traffic to recipient thymus after systemic injection. Infusion of allogeneic donor-derived FLDCs induced clonal deletion of both CD4 and CD8 single-positive alloreactive thymocytes, leading to donor-specific central tolerance and prolonged survival of donor skin grafts (183).
Recipient-Derived tolDCs
It should be emphasized that the use of recipient autologous DCs appears to be more feasible than that of donor DCs, as tolDCs can be prepared from peripheral blood of the recipient before SOT. Recipient tolDCs could be generated and stored while the patient is on the waiting list, and loaded with donor-derived antigens (HLA peptides, donor cell lysates) at the time of transplantation. Taking advantage of linked suppression mechanisms, it could be sufficient to generate recipient tolDCs expressing some but not all donor alloantigens (184). Importantly, the use of recipient DCs allows for the generation of tolDCs with the potential to induce indirect pathway CD4+ T-cell hyporesponsiveness and donor-specific Treg, while also controlling anti-donor humoral responses, which could have protective effects against chronic rejection (185–188). Recipient DCs pulsed with donor allopeptides and injected into the thymus in combination with one dose of anti-lymphocyte serum 7 days before transplantation induced donor-specific tolerance to cardiac (189) and pancreatic islets (190) allografts in rats. In an experimental model, the infusion of recipient DCs expressing donor intact MHC class I antigens selectively prevented indirect pathway alloreactive CD4+ T cells activation and the generation of alloantibodies, resulting in long-term survival of MHC-mismatched skin allografts (191). Interestingly, recipient bone marrow-derived tolDCs (generated in the presence of low-dose GM-CSF) infused one day before transplantation significantly prolonged cardiac allograft survival, even in the absence of prior in vitro pulsing with donor antigens. Although recipients showed reduced anti-donor cellular and humoral responses ex vivo, the effect of these tolDCs was not antigen-specific (192). This study suggests that, while alloantigen-specific immune regulation is desirable in the setting of SOT, recipient autologous tolDCs may also modulate the microenvironment in a way that favors allograft survival. The ONE study consortium aims at promoting clinical tolerance in living-donor renal transplant recipients (193). Within this consortium, a multicenter phase I/II safety/efficacy trial (ClinicalTrials.gov identifier NCT02252055) is currently evaluating the administration of autologous tolDCs on top of the standard immunosuppressive regimen (tacrolimus-MPA-corticosteroids).
Feasibility and Safety of DC-Based Immunotherapy
Phenotype and Function of Generated tolDCs
In recent years, an improved understanding of the mechanisms that govern central and peripheral immune tolerance, along with a more precise characterization of DC subsets, has opened the door to their therapeutic use in autoimmune diseases and SOT (Table 3). There are, however, some caveats that need to be addressed in order to safely translate and evaluate DC-based immunotherapy to the clinical arena. One major hurdle is the generation of high amounts of tolDCs of reproducible quality using clinical-grade procedures. Cells isolation, culture, and preconditioning protocols need to be optimized to generate tolDCs with stable phenotypes and functions. A better identification of subset-specific markers of human tolDCs would be valuable for safe delivery to patients. Another major concern is the stability of ex vivo generated tolDCs in a pro-inflammatory environment in vivo. While many studies support a maturation-resistant state of human tolDCs produced in vitro using various conditioning regimens, the in vivo environment may still induce maturation toward undesired immunogenic DCs (180, 194, 195). In the context of SOT, infused donor tolDCs have been shown to be short-lived, being eliminated mainly by recipient NK cells. Autologous recipient DCs could then process and present donor-derived MHC:peptide alloantigens in an immunogenic context, leading to allosensitization and accelerated allograft rejection (196). However, this aspect remains controversial as there is evidence suggesting that, while donor tolDCs indeed rapidly die after infusion and are re-processed by splenic recipient DC, this may not lead to allograft rejection because alloantigens are delivered in the context of tolDCs (197). Nonetheless, tolDC-based “negative vaccination” most likely will need to be combined with immunomodulatory drugs in vivo to harness strong immune activation, as demonstrated in NHP transplantation models (86, 198). Overall, additional preclinical and clinical trials are now needed to demonstrate the feasibility and safety of tolDC-based immunotherapy in humans. For instance, in a clinical study antigen-loaded autologous tolDCs were injected intradermic in healthy volunteers. While the treatment was well tolerated, it also resulted in antigen-specific regulation of Teff (199, 200).
Source of DCs and Administration Protocols
The source of DCs is a central aspect of tolDC-based strategies. In the context of autoimmunity or end-stage organ disease, a possible effect of the disease on DCs population and function needs to be addressed. Specifically, it must be demonstrated that autologous tolDCs from patients are as stable as tolDCs derived from healthy individuals (157). The number of cells to be delivered, the most appropriate timing of injection, and the route of administration also need to be carefully evaluated. Antigen-specificity of DC-based immunomodulation is another open issue with respect to clinical applications. Targeted regulation of antigen-specific T-cell responses would avoid generalized immunosuppression and impaired immune surveillance leading to infections or the development of malignancies. However, specific autoantigens that are responsible for T-cell priming have not been identified in some autoimmune diseases such as IBD, and peripheral DCs used for immunotherapy may not present some tissue-specific antigens. It also should be considered that, in deceased donor SOT, donor alloantigens are not known until the day of transplantation.
In an attempt to limit the workload and costs of ex vivo generation of tolDCs, while also circumventing the uncertainty regarding their stability, these cells could be directly generated in vivo. As discussed, the administration of apoptotic cells or in vivo induction of apoptosis could be a first option in this regard (136, 201). Another possibility may involve the administration of specific immunomodulatory cytokines or drugs. Infliximab, a chimeric monoclonal antibody that neutralizes both soluble and membrane-bound TNF-α, is an effective treatment in autoimmune diseases such as psoriasis, RA and IBD, which can lead to long-term remission. Besides its inhibitory effects on T-cell activation and homing, infliximab was shown to impair the differentiation and antigen-presenting capacity of ModDCs (202) and to restore the suppressive function of previously compromised Treg in patients with RA (203). CTLA-4 Ig, a fusion protein that blocks B7-CD28 costimulation and is currently used in RA patients as well as in SOT, can also promote tolDC (204, 205). The combination of adoptive transfer of tolDCs and CTLA-4 Ig resulted in extended survival of MHC-mismatched heart allografts in mice (206). In an experimental model, CTLA-4 Ig suppressed collagen-induced arthritis by inducing tolDCs and expanding Treg. This effect was abrogated by anti-TGF-β treatment (207). Other drugs that can be used to modulate DCs functions are listed in Table 2. Finally, antigens could be directly delivered to steady-state quiescent DCs in vivo by specifically targeting DC-restricted endocytic receptors (DEC-205) with monoclonal antibodies (208), aiming at inducing antigen-specific T-cell hyporesponsiveness. Altogether, it will be important to take advantage of some currently used drugs or biological agents in order to promote an environment that favors tolDCs.
Overcoming Memory Responses
Based on their central role in immune activation, it is very tempting to consider immunotherapy using tolDCs for tolerance induction to specific antigens. Indeed, the type of DC and the cytokine microenvironment at the time of antigen presentation are major components in the regulation of T-cell responsiveness. While many in vitro and in vivo experimental data support the potential of tolDCs in regulating the priming of naïve T cells, less evidence exist regarding memory T cells. This is an important issue as the human immune repertoire harbors antigen-specific as well as cross-reactive long-lived memory T cells and antibodies which could represent an obstacle for clinical translational of tolDCs-based immunotherapy in chronic autoimmune diseases as well as in SOT (209, 210). There are, however, some experimental and preclinical studies that illustrate the potential of tolDCs in controlling memory T cells (108, 133, 134), suggesting that tolDCs would be advantageous compared to Treg-based immunotherapy or more conventional immunosuppression that are less efficient in the presence of pre-existing memory responses (211). In an experimental allergic airway disease model, tolDCs were shown to inhibit allergen-specific memory Th2 responses and airway inflammation in sensitized hosts (135). In a clinically relevant NHP model of MHC-mismatched renal transplantation with minimal immunosuppression, infusion of donor-derived tolDCs 1 week before transplantation prolonged allograft survival, with no evidence of host sensitization. This therapeutic effect was associated with selective attenuation of donor-reactive memory T-cell responses (86, 198).
Migration of tolDCs
Although significant progress has been made in the generation of tolDCs, the capacity of DCs to access LNs and encounter T cells, as well as the survival of treated DCs remains a critical concern. It is known that immature DCs traffic poorly to LNs. Different approaches have been made to overcome this issue. Genetically engineered DCs that express CCR7 and IL-10 showed improved migration ability to T-cell zones of secondary lymphoid organs and promoted prolonged heart allograft survival in a mouse model (212). Interestingly, in the setting of cancer vaccines development, the direct intra-lymphatic delivery approach is being evaluated in a clinical setting. DCs injected into a lymphatic vessel showed a prolonged half-life compared to DCs injected intravenously and were rapidly detected in LNs where they elicited a strong T-cell response (213, 214). The technique proved to be feasible and safe for short-term delivery of DCs. However, research is still needed to assess the efficacy of this route and the clinical relevance in the context of tolerance induction.
Conclusion and Perspectives
The field of immune regulation has become increasingly complex with the identification of both lymphoid and non-lymphoid cells that are involved in the induction and maintenance of immune tolerance. DCs are at the cornerstone of adaptive immune responses and, therefore, represent an appealing tool for immunoregulatory therapies in autoimmune diseases as well as in SOT. While extensive data have been generated in animal models, questions remain regarding the distribution and function of human DCs subsets in vivo. Clinical protocols need to be optimized for the safe generation of tolDCs, ensuring stable phenotypes and immunomodulatory functions. Interestingly, although both exogenously transferred or in vivo induced tolDCs may have a short half-life, they were shown to induce a regulatory environment (regulatory cell-subsets and cytokines) that could promote a more prolonged maintenance of peripheral tolerance (215). Finally, tolDCs immunotherapy has a unique potential for inducing antigen-specific central tolerance.
Whether or not tolDCs can maintain their function and regulate memory T-cell and B-cell responses in a strong inflammatory environment still remains to be demonstrated in more stringent preclinical models (209, 216, 217). In the clinical setting, tolDC-based therapy, therefore, may not be sufficient per se to promote tolerance and may need to be used in conjunction with immunomodulatory drugs. While further preclinical and clinical studies are needed before tolDC-based immunotherapy can be successfully translated to the clinic, the quest for modalities to induce immune tolerance has clearly improved our understanding of human DC biology. The production and use of tolDCs in the upcoming years will continue to represent a challenging and exciting road, which, ultimately, may improve various immune-mediated clinical pathologies.
Author Contributions
All authors contributed to writing the article. The final version of the manuscript was reviewed and approved by all authors.
Conflict of Interest Statement
The authors declare that the research was conducted in the absence of any commercial or financial relationships that could be construed as a potential conflict of interest.
Acknowledgments
This work was supported by the Fondation Pierre Mercier pour la Science, the Fondation Medi-CAL Futur, the Fondation Lausannoise pour la Transplantation d’Organes (to DG), the Fondation Vaudoise de Cardiologie (to GV), as well as an unrestricted grant from Astellas (to DG and MP).
References
1. Steinman RM, Hawiger D, Liu K, Bonifaz L, Bonnyay D, Mahnke K, et al. Dendritic cell function in vivo during the steady state: a role in peripheral tolerance. Ann N Y Acad Sci (2003) 987:15–25. doi:10.1111/j.1749-6632.2003.tb06029.x
2. Boltjes A, van Wijk F. Human dendritic cell functional specialization in steady-state and inflammation. Front Immunol (2014) 5:131. doi:10.3389/fimmu.2014.00131
3. Baldwin WM III, Larsen CP, Fairchild RL. Innate immune responses to transplants: a significant variable with cadaver donors. Immunity (2001) 14(4):369–76. doi:10.1016/S1074-7613(01)00117-0
4. Mellman I, Steinman RM. Dendritic cells: specialized and regulated antigen processing machines. Cell (2001) 106(3):255–8. doi:10.1016/S0092-8674(01)00449-4
5. Lee J, Breton G, Oliveira TY, Zhou YJ, Aljoufi A, Puhr S, et al. Restricted dendritic cell and monocyte progenitors in human cord blood and bone marrow. J Exp Med (2015) 212(3):385–99. doi:10.1084/jem.20141442
6. Breton G, Zheng S, Valieris R, Tojal da Silva I, Satija R, Nussenzweig MC. Human dendritic cells (DCs) are derived from distinct circulating precursors that are precommitted to become CD1c+ or CD141+ DCs. J Exp Med (2016) 213(13):2861–70. doi:10.1084/jem.20161135
7. Guilliams M, Dutertre CA, Scott CL, McGovern N, Sichien D, Chakarov S, et al. Unsupervised high-dimensional analysis aligns dendritic cells across tissues and species. Immunity (2016) 45(3):669–84. doi:10.1016/j.immuni.2016.08.015
8. Poulin LF, Salio M, Griessinger E, Anjos-Afonso F, Craciun L, Chen JL, et al. Characterization of human DNGR-1+ BDCA3+ leukocytes as putative equivalents of mouse CD8alpha+ dendritic cells. J Exp Med (2010) 207(6):1261–71. doi:10.1084/jem.20092618
9. Jongbloed SL, Kassianos AJ, McDonald KJ, Clark GJ, Ju X, Angel CE, et al. Human CD141+ (BDCA-3)+ dendritic cells (DCs) represent a unique myeloid DC subset that cross-presents necrotic cell antigens. J Exp Med (2010) 207(6):1247–60. doi:10.1084/jem.20092140
10. Desch AN, Gibbings SL, Goyal R, Kolde R, Bednarek J, Bruno T, et al. Flow cytometric analysis of mononuclear phagocytes in nondiseased human lung and lung-draining lymph nodes. Am J Respir Crit Care Med (2016) 193(6):614–26. doi:10.1164/rccm.201507-1376OC
11. Segura E, Touzot M, Bohineust A, Cappuccio A, Chiocchia G, Hosmalin A, et al. Human inflammatory dendritic cells induce Th17 cell differentiation. Immunity (2013) 38(2):336–48. doi:10.1016/j.immuni.2012.10.018
12. Gazdhar A, Blank F, Cesson V, Lovis A, Aubert JD, Lazor R, et al. Human bronchial epithelial cells induce CD141/CD123/DC-SIGN/FLT3 monocytes that promote allogeneic Th17 differentiation. Front Immunol (2017) 8:447. doi:10.3389/fimmu.2017.00447
13. Lauterbach H, Bathke B, Gilles S, Traidl-Hoffmann C, Luber CA, Fejer G, et al. Mouse CD8alpha+ DCs and human BDCA3+ DCs are major producers of IFN-lambda in response to poly IC. J Exp Med (2010) 207(12):2703–17. doi:10.1084/jem.20092720
14. Cao W, Bover L, Cho M, Wen X, Hanabuchi S, Bao M, et al. Regulation of TLR7/9 responses in plasmacytoid dendritic cells by BST2 and ILT7 receptor interaction. J Exp Med (2009) 206(7):1603–14. doi:10.1084/jem.20090547
15. Matta BM, Castellaneta A, Thomson AW. Tolerogenic plasmacytoid DC. Eur J Immunol (2010) 40(10):2667–76. doi:10.1002/eji.201040839
16. Swiecki M, Colonna M. Unraveling the functions of plasmacytoid dendritic cells during viral infections, autoimmunity, and tolerance. Immunol Rev (2010) 234(1):142–62. doi:10.1111/j.0105-2896.2009.00881.x
17. Dzionek A, Fuchs A, Schmidt P, Cremer S, Zysk M, Miltenyi S, et al. BDCA-2, BDCA-3, and BDCA-4: three markers for distinct subsets of dendritic cells in human peripheral blood. J Immunol (2000) 165(11):6037–46. doi:10.4049/jimmunol.165.11.6037
18. Bakdash G, Buschow SI, Gorris MA, Halilovic A, Hato SV, Sköld AE, et al. Expansion of a BDCA1+CD14+ myeloid cell population in melanoma patients may attenuate the efficacy of dendritic cell vaccines. Cancer Res (2016) 76(15):4332–46. doi:10.1158/0008-5472.CAN-15-1695
19. Rovati B, Mariucci S, Manzoni M, Bencardino K, Danova M. Flow cytometric detection of circulating dendritic cells in healthy subjects. Eur J Histochem (2008) 52(1):45–52. doi:10.4081/1185
20. Yu CI, Becker C, Metang P, Marches F, Wang Y, Toshiyuki H, et al. Human CD141+ dendritic cells induce CD4+ T cells to produce type 2 cytokines. J Immunol (2014) 193(9):4335–43. doi:10.4049/jimmunol.1401159
21. Segura E, Durand M, Amigorena S. Similar antigen cross-presentation capacity and phagocytic functions in all freshly isolated human lymphoid organ-resident dendritic cells. J Exp Med (2013) 210(5):1035–47. doi:10.1084/jem.20121103
22. Collin M, McGovern N, Haniffa M. Human dendritic cell subsets. Immunology (2013) 140(1):22–30. doi:10.1111/imm.12117
23. Lambrecht BN, Hammad H. Lung dendritic cells in respiratory viral infection and asthma: from protection to immunopathology. Annu Rev Immunol (2012) 30:243–70. doi:10.1146/annurev-immunol-020711-075021
24. Van Pottelberge GR, Bracke KR, Demedts IK, De Rijck K, Reinartz SM, van Drunen CM, et al. Selective accumulation of Langerhans-type dendritic cells in small airways of patients with COPD. Respir Res (2010) 11:35. doi:10.1186/1465-9921-11-35
25. Segura E, Valladeau-Guilemond J, Donnadieu MH, Sastre-Garau X, Soumelis V, Amigorena S. Characterization of resident and migratory dendritic cells in human lymph nodes. J Exp Med (2012) 209(4):653–60. doi:10.1084/jem.20111457
26. Bratke K, Lommatzsch M, Julius P, Kuepper M, Kleine HD, Luttmann W, et al. Dendritic cell subsets in human bronchoalveolar lavage fluid after segmental allergen challenge. Thorax (2007) 62(2):168–75. doi:10.1136/thx.2006.067793
27. Angel CE, Chen CJ, Horlacher OC, Winkler S, John T, Browning J, et al. Distinctive localization of antigen-presenting cells in human lymph nodes. Blood (2009) 113(6):1257–67. doi:10.1182/blood-2008-06-165266
28. Sallusto F, Lanzavecchia A. Efficient presentation of soluble antigen by cultured human dendritic cells is maintained by granulocyte/macrophage colony-stimulating factor plus interleukin 4 and downregulated by tumor necrosis factor alpha. J Exp Med (1994) 179(4):1109–18. doi:10.1084/jem.179.4.1109
29. Ebner S, Hofer S, Nguyen VA, Fürhapter C, Herold M, Fritsch P, et al. A novel role for IL-3: human monocytes cultured in the presence of IL-3 and IL-4 differentiate into dendritic cells that produce less IL-12 and shift Th cell responses toward a Th2 cytokine pattern. J Immunol (2002) 168(12):6199–207. doi:10.4049/jimmunol.168.12.6199
30. Baharom F, Thomas S, Rankin G, Lepzien R, Pourazar J, Behndig AF, et al. Dendritic cells and monocytes with distinct inflammatory responses reside in lung mucosa of healthy humans. J Immunol (2016) 196(11):4498–509. doi:10.4049/jimmunol.1600071
31. Gregori S, Tomasoni D, Pacciani V, Scirpoli M, Battaglia M, Magnani CF, et al. Differentiation of type 1 T regulatory cells (Tr1) by tolerogenic DC-10 requires the IL-10-dependent ILT4/HLA-G pathway. Blood (2010) 116(6):935–44. doi:10.1182/blood-2009-07-234872
32. Hill M, Cuturi MC. Negative vaccination by tolerogenic dendritic cells in organ transplantation. Curr Opin Organ Transplant (2010) 15(6):738–43. doi:10.1097/MOT.0b013e32833f7114
33. Manh TP, Alexandre Y, Baranek T, Crozat K, Dalod M. Plasmacytoid, conventional, and monocyte-derived dendritic cells undergo a profound and convergent genetic reprogramming during their maturation. Eur J Immunol (2013) 43(7):1706–15. doi:10.1002/eji.201243106
34. Demedts IK, Bracke KR, Maes T, Joos GF, Brusselle GG. Different roles for human lung dendritic cell subsets in pulmonary immune defense mechanisms. Am J Respir Cell Mol Biol (2006) 35(3):387–93. doi:10.1165/rcmb.2005-0382OC
35. MacDonald KP, Munster DJ, Clark GJ, Dzionek A, Schmitz J, Hart DN. Characterization of human blood dendritic cell subsets. Blood (2002) 100(13):4512–20. doi:10.1182/blood-2001-11-0097
36. Schlitzer A, McGovern N, Teo P, Zelante T, Atarashi K, Low D, et al. IRF4 transcription factor-dependent CD11b+ dendritic cells in human and mouse control mucosal IL-17 cytokine responses. Immunity (2013) 38(5):970–83. doi:10.1016/j.immuni.2013.04.011
37. Shan M, Cheng HF, Song LZ, Roberts L, Green L, Hacken-Bitar J, et al. Lung myeloid dendritic cells coordinately induce TH1 and TH17 responses in human emphysema. Sci Transl Med (2009) 1(4):4ra10. doi:10.1126/scitranlsmed.3000154
38. Haniffa M, Shin A, Bigley V, McGovern N, Teo P, See P, et al. Human tissues contain CD141hi cross-presenting dendritic cells with functional homology to mouse CD103+ nonlymphoid dendritic cells. Immunity (2012) 37(1):60–73. doi:10.1016/j.immuni.2012.04.012
39. Crozat K, Guiton R, Contreras V, Feuillet V, Dutertre CA, Ventre E, et al. The XC chemokine receptor 1 is a conserved selective marker of mammalian cells homologous to mouse CD8alpha+ dendritic cells. J Exp Med (2010) 207(6):1283–92. doi:10.1084/jem.20100223
40. Merad M, Sathe P, Helft J, Miller J, Mortha A. The dendritic cell lineage: ontogeny and function of dendritic cells and their subsets in the steady state and the inflamed setting. Annu Rev Immunol (2013) 31:563–604. doi:10.1146/annurev-immunol-020711-074950
41. Kool M, van Nimwegen M, Willart MA, Muskens F, Boon L, Smit JJ, et al. An anti-inflammatory role for plasmacytoid dendritic cells in allergic airway inflammation. J Immunol (2009) 183(2):1074–82. doi:10.4049/jimmunol.0900471
42. Abe M, Wang Z, de Creus A, Thomson AW. Plasmacytoid dendritic cell precursors induce allogeneic T-cell hyporesponsiveness and prolong heart graft survival. Am J Transplant (2005) 5(8):1808–19. doi:10.1111/j.1600-6143.2005.00954.x
43. Ochando JC, Homma C, Yang Y, Hidalgo A, Garin A, Tacke F, et al. Alloantigen-presenting plasmacytoid dendritic cells mediate tolerance to vascularized grafts. Nat Immunol (2006) 7(6):652–62. doi:10.1038/ni1333
44. Tokita D, Mazariegos GV, Zahorchak AF, Chien N, Abe M, Raimondi G, et al. High PD-L1/CD86 ratio on plasmacytoid dendritic cells correlates with elevated T-regulatory cells in liver transplant tolerance. Transplantation (2008) 85(3):369–77. doi:10.1097/TP.0b013e3181612ded
45. Tang Q, Bluestone JA. Plasmacytoid DCs and T(reg) cells: casual acquaintance or monogamous relationship? Nat Immunol (2006) 7(6):551–3. doi:10.1038/ni0606-551
46. Gilliet M, Boonstra A, Paturel C, Antonenko S, Xu XL, Trinchieri G, et al. The development of murine plasmacytoid dendritic cell precursors is differentially regulated by FLT3-ligand and granulocyte/macrophage colony-stimulating factor. J Exp Med (2002) 195(7):953–8. doi:10.1084/jem.20020045
47. Ohnmacht C, Pullner A, King SB, Drexler I, Meier S, Brocker T, et al. Constitutive ablation of dendritic cells breaks self-tolerance of CD4 T cells and results in spontaneous fatal autoimmunity. J Exp Med (2009) 206(3):549–59. doi:10.1084/jem.20082394
48. Bar-On L, Jung S. Defining dendritic cells by conditional and constitutive cell ablation. Immunol Rev (2010) 234(1):76–89. doi:10.1111/j.0105-2896.2009.00875.x
49. Matteoli G, Mazzini E, Iliev ID, Mileti E, Fallarino F, Puccetti P, et al. Gut CD103+ dendritic cells express indoleamine 2,3-dioxygenase which influences T regulatory/T effector cell balance and oral tolerance induction. Gut (2010) 59(5):595–604. doi:10.1136/gut.2009.185108
50. Chu CC, Ali N, Karagiannis P, Di Meglio P, Skowera A, Napolitano L, et al. Resident CD141 (BDCA3)+ dendritic cells in human skin produce IL-10 and induce regulatory T cells that suppress skin inflammation. J Exp Med (2012) 209(5):935–45. doi:10.1084/jem.20112583
51. Laskarin G, Kämmerer U, Rukavina D, Thomson AW, Fernandez N, Blois SM. Antigen-presenting cells and materno-fetal tolerance: an emerging role for dendritic cells. Am J Reprod Immunol (2007) 58(3):255–67. doi:10.1111/j.1600-0897.2007.00511.x
52. Ristich V, Zhang W, Liang S, Horuzsko A. Mechanisms of prolongation of allograft survival by HLA-G/ILT4-modified dendritic cells. Hum Immunol (2007) 68(4):264–71. doi:10.1016/j.humimm.2006.11.008
53. Fife BT, Pauken KE, Eagar TN, Obu T, Wu J, Tang Q, et al. Interactions between PD-1 and PD-L1 promote tolerance by blocking the TCR-induced stop signal. Nat Immunol (2009) 10(11):1185–92. doi:10.1038/ni.1790
54. Rabinovich GA, Baum LG, Tinari N, Paganelli R, Natoli C, Liu FT, et al. Galectins and their ligands: amplifiers, silencers or tuners of the inflammatory response? Trends Immunol (2002) 23(6):313–20. doi:10.1016/S1471-4906(02)02232-9
55. Garín MI, Chu CC, Golshayan D, Cernuda-Morollón E, Wait R, Lechler RI. Galectin-1: a key effector of regulation mediated by CD4+CD25+ T cells. Blood (2007) 109(5):2058–65. doi:10.1182/blood-2006-04-016451
56. Ilarregui JM, Croci DO, Bianco GA, Toscano MA, Salatino M, Vermeulen ME, et al. Tolerogenic signals delivered by dendritic cells to T cells through a galectin-1-driven immunoregulatory circuit involving interleukin 27 and interleukin 10. Nat Immunol (2009) 10(9):981–91. doi:10.1038/ni.1772
57. Perillo NL, Pace KE, Seilhamer JJ, Baum LG. Apoptosis of T cells mediated by galectin-1. Nature (1995) 378(6558):736–9. doi:10.1038/378736a0
58. Rabinovich GA, Daly G, Dreja H, Tailor H, Riera CM, Hirabayashi J, et al. Recombinant galectin-1 and its genetic delivery suppress collagen-induced arthritis via T cell apoptosis. J Exp Med (1999) 190(3):385–98. doi:10.1084/jem.190.3.385
59. Mobergslien A, Sioud M. Galectin-1 and -3 gene silencing in immature and mature dendritic cells enhances T cell activation and interferon-gamma production. J Leukoc Biol (2012) 91(3):461–7. doi:10.1189/jlb.0711361
60. Perone MJ, Bertera S, Tawadrous ZS, Shufesky WJ, Piganelli JD, Baum LG, et al. Dendritic cells expressing transgenic galectin-1 delay onset of autoimmune diabetes in mice. J Immunol (2006) 177(8):5278–89. doi:10.4049/jimmunol.177.8.5278
61. Moreau A, Noble A, Ratnasothy K, Chai JG, Deltour L, Cuturi MC, et al. Absence of galectin-1 accelerates CD8(+) T cell-mediated graft rejection. Eur J Immunol (2012) 42(11):2881–8. doi:10.1002/eji.201142325
62. Seeger P, Musso T, Sozzani S. The TGF-beta superfamily in dendritic cell biology. Cytokine Growth Factor Rev (2015) 26(6):647–57. doi:10.1016/j.cytogfr.2015.06.002
63. Worthington JJ, Czajkowska BI, Melton AC, Travis MA. Intestinal dendritic cells specialize to activate transforming growth factor-beta and induce Foxp3+ regulatory T cells via integrin alphavbeta8. Gastroenterology (2011) 141(5):1802–12. doi:10.1053/j.gastro.2011.06.057
64. Chen W, Jin W, Hardegen N, Lei KJ, Li L, Marinos N, et al. Conversion of peripheral CD4+CD25- naive T cells to CD4+CD25+ regulatory T cells by TGF-beta induction of transcription factor Foxp3. J Exp Med (2003) 198(12):1875–86. doi:10.1084/jem.20030152
65. Chen Q, Kim YC, Laurence A, Punkosdy GA, Shevach EM. IL-2 controls the stability of Foxp3 expression in TGF-beta-induced Foxp3+ T cells in vivo. J Immunol (2011) 186(11):6329–37. doi:10.4049/jimmunol.1100061
66. Qureshi OS, Zheng Y, Nakamura K, Attridge K, Manzotti C, Schmidt EM, et al. Trans-endocytosis of CD80 and CD86: a molecular basis for the cell-extrinsic function of CTLA-4. Science (2011) 332(6029):600–3. doi:10.1126/science.1202947
67. Munn DH, Sharma MD, Lee JR, Jhaver KG, Johnson TS, Keskin DB, et al. Potential regulatory function of human dendritic cells expressing indoleamine 2,3-dioxygenase. Science (2002) 297(5588):1867–70. doi:10.1126/science.1073514
68. Grohmann U, Orabona C, Fallarino F, Vacca C, Calcinaro F, Falorni A, et al. CTLA-4-Ig regulates tryptophan catabolism in vivo. Nat Immunol (2002) 3(11):1097–101. doi:10.1038/ni846
69. Mellor AL, Chandler P, Baban B, Hansen AM, Marshall B, Pihkala J, et al. Specific subsets of murine dendritic cells acquire potent T cell regulatory functions following CTLA4-mediated induction of indoleamine 2,3 dioxygenase. Int Immunol (2004) 16(10):1391–401. doi:10.1093/intimm/dxh140
70. Puccetti P, Grohmann U. IDO and regulatory T cells: a role for reverse signalling and non-canonical NF-kappaB activation. Nat Rev Immunol (2007) 7(10):817–23. doi:10.1038/nri2163
71. Chung DJ, Rossi M, Romano E, Ghith J, Yuan J, Munn DH, et al. Indoleamine 2,3-dioxygenase-expressing mature human monocyte-derived dendritic cells expand potent autologous regulatory T cells. Blood (2009) 114(3):555–63. doi:10.1182/blood-2008-11-191197
72. Brenk M, Scheler M, Koch S, Neumann J, Takikawa O, Häcker G, et al. Tryptophan deprivation induces inhibitory receptors ILT3 and ILT4 on dendritic cells favoring the induction of human CD4+CD25+ Foxp3+ T regulatory cells. J Immunol (2009) 183(1):145–54. doi:10.4049/jimmunol.0803277
73. Fallarino F, Grohmann U, Vacca C, Bianchi R, Orabona C, Spreca A, et al. T cell apoptosis by tryptophan catabolism. Cell Death Differ (2002) 9(10):1069–77. doi:10.1038/sj.cdd.4401073
74. Fallarino F, Grohmann U, You S, McGrath BC, Cavener DR, Vacca C, et al. The combined effects of tryptophan starvation and tryptophan catabolites down-regulate T cell receptor zeta-chain and induce a regulatory phenotype in naive T cells. J Immunol (2006) 176(11):6752–61. doi:10.4049/jimmunol.176.11.6752
75. Naranjo-Gómez M, Raïch-Regué D, Oñate C, Grau-López L, Ramo-Tello C, Pujol-Borrell R, et al. Comparative study of clinical grade human tolerogenic dendritic cells. J Transl Med (2011) 9:89. doi:10.1186/1479-5876-9-89
76. Tsoumakidou M, Tousa S, Semitekolou M, Panagiotou P, Panagiotou A, Morianos I, et al. Tolerogenic signaling by pulmonary CD1c+ dendritic cells induces regulatory T cells in patients with chronic obstructive pulmonary disease by IL-27/IL-10/inducible costimulator ligand. J Allergy Clin Immunol (2014) 134(4):944–54.e8. doi:10.1016/j.jaci.2014.05.045
77. Bonasio R, Scimone ML, Schaerli P, Grabie N, Lichtman AH, von Andrian UH. Clonal deletion of thymocytes by circulating dendritic cells homing to the thymus. Nat Immunol (2006) 7(10):1092–100. doi:10.1038/ni1385
78. Proietto AI, van Dommelen S, Zhou P, Rizzitelli A, D’Amico A, Steptoe RJ, et al. Dendritic cells in the thymus contribute to T-regulatory cell induction. Proc Natl Acad Sci U S A (2008) 105(50):19869–74. doi:10.1073/pnas.0810268105
79. Watanabe N, Wang YH, Lee HK, Ito T, Wang YH, Cao W, et al. Hassall’s corpuscles instruct dendritic cells to induce CD4+CD25+ regulatory T cells in human thymus. Nature (2005) 436(7054):1181–5. doi:10.1038/nature03886
80. Besin G, Gaudreau S, Ménard M, Guindi C, Dupuis G, Amrani A. Thymic stromal lymphopoietin and thymic stromal lymphopoietin-conditioned dendritic cells induce regulatory T-cell differentiation and protection of NOD mice against diabetes. Diabetes (2008) 57(8):2107–17. doi:10.2337/db08-0171
81. Martín-Gayo E, Sierra-Filardi E, Corbí AL, Toribio ML. Plasmacytoid dendritic cells resident in human thymus drive natural Treg cell development. Blood (2010) 115(26):5366–75. doi:10.1182/blood-2009-10-248260
82. Chen ZM, O’Shaughnessy MJ, Gramaglia I, Panoskaltsis-Mortari A, Murphy WJ, Narula S, et al. IL-10 and TGF-beta induce alloreactive CD4+CD25- T cells to acquire regulatory cell function. Blood (2003) 101(12):5076–83. doi:10.1182/blood-2002-09-2798
83. Govender L, Pascual M, Golshayan D. Potential and limitations of regulatory T-cell therapy in solid organ transplantation. Expert Rev Clin Immunol (2014) 10(9):1197–212. doi:10.1586/1744666X.2014.943191
84. Hackstein H, Thomson AW. Dendritic cells: emerging pharmacological targets of immunosuppressive drugs. Nat Rev Immunol (2004) 4(1):24–34. doi:10.1038/nri1256
85. Morelli AE, Thomson AW. Tolerogenic dendritic cells and the quest for transplant tolerance. Nat Rev Immunol (2007) 7(8):610–21. doi:10.1038/nri2132
86. Ezzelarab MB, Zahorchak AF, Lu L, Morelli AE, Chalasani G, Demetris AJ, et al. Regulatory dendritic cell infusion prolongs kidney allograft survival in nonhuman primates. Am J Transplant (2013) 13(8):1989–2005. doi:10.1111/ajt.12310
87. Horibe EK, Sacks J, Unadkat J, Raimondi G, Wang Z, Ikeguchi R, et al. Rapamycin-conditioned, alloantigen-pulsed dendritic cells promote indefinite survival of vascularized skin allografts in association with T regulatory cell expansion. Transpl Immunol (2008) 18(4):307–18. doi:10.1016/j.trim.2007.10.007
88. Lee JI, Ganster RW, Geller DA, Burckart GJ, Thomson AW, Lu L. Cyclosporine A inhibits the expression of costimulatory molecules on in vitro-generated dendritic cells: association with reduced nuclear translocation of nuclear factor kappa B. Transplantation (1999) 68(9):1255–63. doi:10.1097/00007890-199911150-00007
89. Lee YR, Yang IH, Lee YH, Im SA, Song S, Li H, et al. Cyclosporin A and tacrolimus, but not rapamycin, inhibit MHC-restricted antigen presentation pathways in dendritic cells. Blood (2005) 105(10):3951–5. doi:10.1182/blood-2004-10-3927
90. Lagaraine C, Lemoine R, Baron C, Nivet H, Velge-Roussel F, Lebranchu Y. Induction of human CD4+ regulatory T cells by mycophenolic acid-treated dendritic cells. J Leukoc Biol (2008) 84(4):1057–64. doi:10.1189/jlb.1007716
91. Gregori S, Casorati M, Amuchastegui S, Smiroldo S, Davalli AM, Adorini L. Regulatory T cells induced by 1 alpha,25-dihydroxyvitamin D3 and mycophenolate mofetil treatment mediate transplantation tolerance. J Immunol (2001) 167(4):1945–53. doi:10.4049/jimmunol.167.4.1945
92. Piemonti L, Monti P, Allavena P, Sironi M, Soldini L, Leone BE, et al. Glucocorticoids affect human dendritic cell differentiation and maturation. J Immunol (1999) 162(11):6473–81.
93. Thomas JM, Eckhoff DE, Contreras JL, Lobashevsky AL, Hubbard WJ, Moore JK, et al. Durable donor-specific T and B cell tolerance in rhesus macaques induced with peritransplantation anti-CD3 immunotoxin and deoxyspergualin: absence of chronic allograft nephropathy. Transplantation (2000) 69(12):2497–503. doi:10.1097/00007890-200006270-00007
94. Min WP, Zhou D, Ichim TE, Strejan GH, Xia X, Yang J, et al. Inhibitory feedback loop between tolerogenic dendritic cells and regulatory T cells in transplant tolerance. J Immunol (2003) 170(3):1304–12. doi:10.4049/jimmunol.170.3.1304
95. Zeng M, Guinet E, Nouri-Shirazi M. Comparative analysis of dendritic cells and anti-CD3/CD28 expanded regulatory T cells for application in transplantation. Transpl Immunol (2009) 22(1–2):82–92. doi:10.1016/j.trim.2009.07.004
96. Szeberényi JB, Pállinger E, Zsinkó M, Pós Z, Rothe G, Orsó E, et al. Inhibition of effects of endogenously synthesized histamine disturbs in vitro human dendritic cell differentiation. Immunol Lett (2001) 76(3):175–82. doi:10.1016/S0165-2478(01)00184-5
97. Penna G, Adorini L. 1 Alpha,25-dihydroxyvitamin D3 inhibits differentiation, maturation, activation, and survival of dendritic cells leading to impaired alloreactive T cell activation. J Immunol (2000) 164(5):2405–11. doi:10.4049/jimmunol.164.5.2405
98. Buckland M, Jago CB, Fazekasova H, Scott K, Tan PH, George AJ, et al. Aspirin-treated human DCs up-regulate ILT-3 and induce hyporesponsiveness and regulatory activity in responder T cells. Am J Transplant (2006) 6(9):2046–59. doi:10.1111/j.1600-6143.2006.01450.x
99. Roehrich ME, Wyss JC, Kumar R, Pascual M, Golshayan D, Vassalli G. Additive effects of rapamycin and aspirin on dendritic cell allostimulatory capacity. Immunopharmacol Immunotoxicol (2015) 37(5):434–41. doi:10.3109/08923973.2015.1081606
100. Buckland M, Lombardi G. Aspirin and the induction of tolerance by dendritic cells. In: Lombardi G, Riffo-Vasquez Y, editors. Dendritic Cells. Handbook of Experimental Pharmacology, vol 188. Berlin, Heidelberg: Springer. (2009). p. 197–213.
101. Hauben E, Gregori S, Draghici E, Migliavacca B, Olivieri S, Woisetschläger M, et al. Activation of the aryl hydrocarbon receptor promotes allograft-specific tolerance through direct and dendritic cell-mediated effects on regulatory T cells. Blood (2008) 112(4):1214–22. doi:10.1182/blood-2007-08-109843
102. Olivar R, Luque A, Naranjo-Gómez M, Quer J, García de Frutos P, Borràs FE, et al. The alpha7beta0 isoform of the complement regulator C4b-binding protein induces a semimature, anti-inflammatory state in dendritic cells. J Immunol (2013) 190(6):2857–72. doi:10.4049/jimmunol.1200503
103. Delgado M. Generating tolerogenic dendritic cells with neuropeptides. Hum Immunol (2009) 70(5):300–7. doi:10.1016/j.humimm.2009.01.020
104. Gonzalez-Rey E, Delgado M. Therapeutic treatment of experimental colitis with regulatory dendritic cells generated with vasoactive intestinal peptide. Gastroenterology (2006) 131(6):1799–811. doi:10.1053/j.gastro.2006.10.023
105. Gonzalez-Rey E, Chorny A, Fernandez-Martin A, Ganea D, Delgado M. Vasoactive intestinal peptide generates human tolerogenic dendritic cells that induce CD4 and CD8 regulatory T cells. Blood (2006) 107(9):3632–8. doi:10.1182/blood-2005-11-4497
106. Brzoska T, Luger TA, Maaser C, Abels C, Böhm M. Alpha-melanocyte-stimulating hormone and related tripeptides: biochemistry, antiinflammatory and protective effects in vitro and in vivo, and future perspectives for the treatment of immune-mediated inflammatory diseases. Endocr Rev (2008) 29(5):581–602. doi:10.1210/er.2007-0027
107. Auriemma M, Brzoska T, Klenner L, Kupas V, Goerge T, Voskort M, et al. alpha-MSH-stimulated tolerogenic dendritic cells induce functional regulatory T cells and ameliorate ongoing skin inflammation. J Invest Dermatol (2012) 132(7):1814–24. doi:10.1038/jid.2012.59
108. Torres-Aguilar H, Aguilar-Ruiz SR, González-Pérez G, Munguía R, Bajaña S, Meraz-Ríos MA, et al. Tolerogenic dendritic cells generated with different immunosuppressive cytokines induce antigen-specific anergy and regulatory properties in memory CD4+ T cells. J Immunol (2010) 184(4):1765–75. doi:10.4049/jimmunol.0902133
109. Rozkova D, Horvath R, Bartunkova J, Spisek R. Glucocorticoids severely impair differentiation and antigen presenting function of dendritic cells despite upregulation of Toll-like receptors. Clin Immunol (2006) 120(3):260–71. doi:10.1016/j.clim.2006.04.567
110. Fazekasova H, Golshayan D, Read J, Tsallios A, Tsang JY, Dorling A, et al. Regulation of rat and human T-cell immune response by pharmacologically modified dendritic cells. Transplantation (2009) 87(11):1617–28. doi:10.1097/TP.0b013e3181a5504c
111. Pedersen AE, Schmidt EG, Gad M, Poulsen SS, Claesson MH. Dexamethasone/1alpha-25-dihydroxyvitamin D3-treated dendritic cells suppress colitis in the SCID T-cell transfer model. Immunology (2009) 127(3):354–64. doi:10.1111/j.1365-2567.2008.02996.x
112. Ferreira GB, Gysemans CA, Demengeot J, da Cunha JP, Vanherwegen AS, Overbergh L, et al. 1,25-Dihydroxyvitamin D3 promotes tolerogenic dendritic cells with functional migratory properties in NOD mice. J Immunol (2014) 192(9):4210–20. doi:10.4049/jimmunol.1302350
113. Raimondi G, Sumpter TL, Matta BM, Pillai M, Corbitt N, Vodovotz Y, et al. Mammalian target of rapamycin inhibition and alloantigen-specific regulatory T cells synergize to promote long-term graft survival in immunocompetent recipients. J Immunol (2010) 184(2):624–36. doi:10.4049/jimmunol.0900936
114. Battaglia M, Stabilini A, Roncarolo MG. Rapamycin selectively expands CD4+CD25+FoxP3+ regulatory T cells. Blood (2005) 105(12):4743–8. doi:10.1182/blood-2004-10-3932
115. Coenen JJ, Koenen HJ, van Rijssen E, Hilbrands LB, Joosten I. Rapamycin, and not cyclosporin A, preserves the highly suppressive CD27+ subset of human CD4+CD25+ regulatory T cells. Blood (2006) 107(3):1018–23. doi:10.1182/blood-2005-07-3032
116. Zeiser R, Negrin RS. Interleukin-2 receptor downstream events in regulatory T cells: implications for the choice of immunosuppressive drug therapy. Cell Cycle (2008) 7(4):458–62. doi:10.4161/cc.7.4.5454
117. Scottà C, Esposito M, Fazekasova H, Fanelli G, Edozie FC, Ali N, et al. Differential effects of rapamycin and retinoic acid on expansion, stability and suppressive qualities of human CD4(+)CD25(+)FOXP3(+) T regulatory cell subpopulations. Haematologica (2013) 98(8):1291–9. doi:10.3324/haematol.2012.074088
118. Lagaraine C, Hoarau C, Chabot V, Velge-Roussel F, Lebranchu Y. Mycophenolic acid-treated human dendritic cells have a mature migratory phenotype and inhibit allogeneic responses via direct and indirect pathways. Int Immunol (2005) 17(4):351–63. doi:10.1093/intimm/dxh215
119. Schmidt LM, Belvisi MG, Bode KA, Bauer J, Schmidt C, Suchy MT, et al. Bronchial epithelial cell-derived prostaglandin E2 dampens the reactivity of dendritic cells. J Immunol (2011) 186(4):2095–105. doi:10.4049/jimmunol.1002414
120. Hawiger D, Wan YY, Eynon EE, Flavell RA. The transcription cofactor Hopx is required for regulatory T cell function in dendritic cell-mediated peripheral T cell unresponsiveness. Nat Immunol (2010) 11(10):962–8. doi:10.1038/ni.1929
121. Eljaafari A, Li YP, Miossec P. IFN-gamma, as secreted during an alloresponse, induces differentiation of monocytes into tolerogenic dendritic cells, resulting in FoxP3+ regulatory T cell promotion. J Immunol (2009) 183(5):2932–45. doi:10.4049/jimmunol.0804352
122. Streilein JW, Masli S, Takeuchi M, Kezuka T. The eye’s view of antigen presentation. Hum Immunol (2002) 63(6):435–43. doi:10.1016/S0198-8859(02)00393-2
123. Iliev ID, Spadoni I, Mileti E, Matteoli G, Sonzogni A, Sampietro GM, et al. Human intestinal epithelial cells promote the differentiation of tolerogenic dendritic cells. Gut (2009) 58(11):1481–9. doi:10.1136/gut.2008.175166
124. Moreau A, Hill M, Thébault P, Deschamps JY, Chiffoleau E, Chauveau C, et al. Tolerogenic dendritic cells actively inhibit T cells through heme oxygenase-1 in rodents and in nonhuman primates. FASEB J (2009) 23(9):3070–7. doi:10.1096/fj.08-128173
125. Chauveau C, Rémy S, Royer PJ, Hill M, Tanguy-Royer S, Hubert FX, et al. Heme oxygenase-1 expression inhibits dendritic cell maturation and proinflammatory function but conserves IL-10 expression. Blood (2005) 106(5):1694–702. doi:10.1182/blood-2005-02-0494
126. Blancou P, Anegon I. Editorial: heme oxygenase-1 and dendritic cells: what else? J Leukoc Biol (2010) 87(2):185–7. doi:10.1189/jlb.0909636
127. Dudler J, Li J, Pagnotta M, Pascual M, von Segesser LK, Vassalli G. Gene transfer of programmed death ligand-1.Ig prolongs cardiac allograft survival. Transplantation (2006) 82(12):1733–7. doi:10.1097/01.tp.0000250757.69384.79
128. Li J, Meinhardt A, Roehrich ME, Golshayan D, Dudler J, Pagnotta M, et al. Indoleamine 2,3-dioxygenase gene transfer prolongs cardiac allograft survival. Am J Physiol Heart Circ Physiol (2007) 293(6):H3415–23. doi:10.1152/ajpheart.00532.2007
129. Vassalli G. Dendritic cell-based approaches for therapeutic immune regulation in solid-organ transplantation. J Transplant (2013) 2013:761429. doi:10.1155/2013/761429
130. Morelli AE, Larregina AT, Ganster RW, Zahorchak AF, Plowey JM, Takayama T, et al. Recombinant adenovirus induces maturation of dendritic cells via an NF-kappaB-dependent pathway. J Virol (2000) 74(20):9617–28. doi:10.1128/JVI.74.20.9617-9628.2000
131. Humbert JM, Halary F. Viral and non-viral methods to genetically modify dendritic cells. Curr Gene Ther (2012) 12(2):127–36. doi:10.2174/156652312800099580
132. Cravens PD, Hayashida K, Davis LS, Nanki T, Lipsky PE. Human peripheral blood dendritic cells and monocyte subsets display similar chemokine receptor expression profiles with differential migratory responses. Scand J Immunol (2007) 65(6):514–24. doi:10.1111/j.1365-3083.2007.01933.x
133. Kenna TJ, Waldie T, McNally A, Thomson M, Yagita H, Thomas R, et al. Targeting antigen to diverse APCs inactivates memory CD8+ T cells without eliciting tissue-destructive effector function. J Immunol (2010) 184(2):598–606. doi:10.4049/jimmunol.0900032
134. Coleman MA, Jessup CF, Bridge JA, Overgaard NH, Penko D, Walters S, et al. Antigen-encoding bone marrow terminates islet-directed memory CD8+ T-cell responses to alleviate islet transplant rejection. Diabetes (2016) 65(5):1328–40. doi:10.2337/db15-1418
135. Al-Kouba J, Wilkinson AN, Starkey MR, Rudraraju R, Werder RB, Liu X, et al. Allergen-encoding bone marrow transfer inactivates allergic T cell responses, alleviating airway inflammation. JCI Insight (2017) 2(11):e85742. doi:10.1172/jci.insight.85742
136. Morelli AE, Larregina AT. Apoptotic cell-based therapies against transplant rejection: role of recipient’s dendritic cells. Apoptosis (2010) 15(9):1083–97. doi:10.1007/s10495-010-0469-9
137. Morelli AE, Larregina AT, Shufesky WJ, Zahorchak AF, Logar AJ, Papworth GD, et al. Internalization of circulating apoptotic cells by splenic marginal zone dendritic cells: dependence on complement receptors and effect on cytokine production. Blood (2003) 101(2):611–20. doi:10.1182/blood-2002-06-1769
138. Sauter B, Albert ML, Francisco L, Larsson M, Somersan S, Bhardwaj N. Consequences of cell death: exposure to necrotic tumor cells, but not primary tissue cells or apoptotic cells, induces the maturation of immunostimulatory dendritic cells. J Exp Med (2000) 191(3):423–34. doi:10.1084/jem.191.3.423
139. Wang Z, Larregina AT, Shufesky WJ, Perone MJ, Montecalvo A, Zahorchak AF, et al. Use of the inhibitory effect of apoptotic cells on dendritic cells for graft survival via T-cell deletion and regulatory T cells. Am J Transplant (2006) 6(6):1297–311. doi:10.1111/j.1600-6143.2006.01308.x
140. Perruche S, Zhang P, Liu Y, Saas P, Bluestone JA, Chen W. CD3-specific antibody-induced immune tolerance involves transforming growth factor-beta from phagocytes digesting apoptotic T cells. Nat Med (2008) 14(5):528–35. doi:10.1038/nm1749
141. Di Lorenzo TP, Peakman M, Roep BO. Translational mini-review series on type 1 diabetes: systematic analysis of T cell epitopes in autoimmune diabetes. Clin Exp Immunol (2007) 148(1):1–16. doi:10.1111/j.1365-2249.2006.03244.x
142. Chatenoud L, Bluestone JA. CD3-specific antibodies: a portal to the treatment of autoimmunity. Nat Rev Immunol (2007) 7(8):622–32. doi:10.1038/nri2134
143. Gaudreau S, Guindi C, Ménard M, Besin G, Dupuis G, Amrani A. Granulocyte-macrophage colony-stimulating factor prevents diabetes development in NOD mice by inducing tolerogenic dendritic cells that sustain the suppressive function of CD4+CD25+ regulatory T cells. J Immunol (2007) 179(6):3638–47. doi:10.4049/jimmunol.179.6.3638
144. Thomas DC, Wong FS, Zaccone P, Green EA, Wållberg M. Protection of islet grafts through transforming growth factor-beta-induced tolerogenic dendritic cells. Diabetes (2013) 62(9):3132–42. doi:10.2337/db12-1740
145. Fantini MC, Becker C, Monteleone G, Pallone F, Galle PR, Neurath MF. Cutting edge: TGF-beta induces a regulatory phenotype in CD4+CD25- T cells through Foxp3 induction and down-regulation of Smad7. J Immunol (2004) 172(9):5149–53. doi:10.4049/jimmunol.172.9.5149
146. Grewal IS, Grewal KD, Wong FS, Wang H, Picarella DE, Janeway CA Jr, et al. Expression of transgene encoded TGF-beta in islets prevents autoimmune diabetes in NOD mice by a local mechanism. J Autoimmun (2002) 19(1–2):9–22. doi:10.1006/jaut.2002.0599
147. Machen J, Harnaha J, Lakomy R, Styche A, Trucco M, Giannoukakis N. Antisense oligonucleotides down-regulating costimulation confer diabetes-preventive properties to nonobese diabetic mouse dendritic cells. J Immunol (2004) 173(7):4331–41. doi:10.4049/jimmunol.173.7.4331
148. Giannoukakis N, Phillips B, Trucco M. Toward a cure for type 1 diabetes mellitus: diabetes-suppressive dendritic cells and beyond. Pediatr Diabetes (2008) 9(3 Pt 2):4–13. doi:10.1111/j.1399-5448.2008.00401.x
149. Mukherjee G, Dilorenzo TP. The immunotherapeutic potential of dendritic cells in type 1 diabetes. Clin Exp Immunol (2010) 161(2):197–207. doi:10.1111/j.1365-2249.2010.04157.x
150. Giannoukakis N, Phillips B, Finegold D, Harnaha J, Trucco M. Phase I (safety) study of autologous tolerogenic dendritic cells in type 1 diabetic patients. Diabetes Care (2011) 34(9):2026–32. doi:10.2337/dc11-0472
151. Nestle FO, Kaplan DH, Barker J. Psoriasis. N Engl J Med (2009) 361(5):496–509. doi:10.1056/NEJMra0804595
152. Mueller W, Herrmann B. Cyclosporin A for psoriasis. N Engl J Med (1979) 301(10):555. doi:10.1056/NEJM197909063011015
153. Sugiyama H, Gyulai R, Toichi E, Garaczi E, Shimada S, Stevens SR, et al. Dysfunctional blood and target tissue CD4+CD25high regulatory T cells in psoriasis: mechanism underlying unrestrained pathogenic effector T cell proliferation. J Immunol (2005) 174(1):164–73. doi:10.4049/jimmunol.174.1.164
154. Yun WJ, Lee DW, Chang SE, Yoon GS, Huh JR, Won CH, et al. Role of CD4CD25FOXP3 regulatory T cells in psoriasis. Ann Dermatol (2010) 22(4):397–403. doi:10.5021/ad.2010.22.4.397
155. Elliott M, Benson J, Blank M, Brodmerkel C, Baker D, Sharples KR, et al. Ustekinumab: lessons learned from targeting interleukin-12/23p40 in immune-mediated diseases. Ann N Y Acad Sci (2009) 1182:97–110. doi:10.1111/j.1749-6632.2009.05070.x
156. Wenink MH, Han W, Toes RE, Radstake TR. Dendritic cells and their potential implication in pathology and treatment of rheumatoid arthritis. Handb Exp Pharmacol (2009) (188):81–98. doi:10.1007/978-3-540-71029-5_4
157. Harry RA, Anderson AE, Isaacs JD, Hilkens CM. Generation and characterisation of therapeutic tolerogenic dendritic cells for rheumatoid arthritis. Ann Rheum Dis (2010) 69(11):2042–50. doi:10.1136/ard.2009.126383
158. Mottet C, Uhlig HH, Powrie F. Cutting edge: cure of colitis by CD4+CD25+ regulatory T cells. J Immunol (2003) 170(8):3939–43. doi:10.4049/jimmunol.170.8.3939
159. Cabezon R, Benitez-Ribas D. Therapeutic potential of tolerogenic dendritic cells in IBD: from animal models to clinical application. Clin Dev Immunol (2013) 2013:789814. doi:10.1155/2013/789814
160. Coombes JL, Powrie F. Dendritic cells in intestinal immune regulation. Nat Rev Immunol (2008) 8(6):435–46. doi:10.1038/nri2335
161. Coombes JL, Siddiqui KR, Arancibia-Cárcamo CV, Hall J, Sun CM, Belkaid Y, et al. A functionally specialized population of mucosal CD103+ DCs induces Foxp3+ regulatory T cells via a TGF-beta and retinoic acid-dependent mechanism. J Exp Med (2007) 204(8):1757–64. doi:10.1084/jem.20070590
162. Baumgart DC, Metzke D, Guckelberger O, Pascher A, Grötzinger C, Przesdzing I, et al. Aberrant plasmacytoid dendritic cell distribution and function in patients with Crohn’s disease and ulcerative colitis. Clin Exp Immunol (2011) 166(1):46–54. doi:10.1111/j.1365-2249.2011.04439.x
163. Baumgart DC, Metzke D, Schmitz J, Scheffold A, Sturm A, Wiedenmann B, et al. Patients with active inflammatory bowel disease lack immature peripheral blood plasmacytoid and myeloid dendritic cells. Gut (2005) 54(2):228–36. doi:10.1136/gut.2004.040360
164. Mannon PJ, Leon F, Fuss IJ, Walter BA, Begnami M, Quezado M, et al. Successful granulocyte-colony stimulating factor treatment of Crohn’s disease is associated with the appearance of circulating interleukin-10-producing T cells and increased lamina propria plasmacytoid dendritic cells. Clin Exp Immunol (2009) 155(3):447–56. doi:10.1111/j.1365-2249.2008.03799.x
165. Kühn R, Löhler J, Rennick D, Rajewsky K, Müller W. Interleukin-10-deficient mice develop chronic enterocolitis. Cell (1993) 75(2):263–74. doi:10.1016/0092-8674(93)80068-P
166. Boks MA, Kager-Groenland JR, Haasjes MS, Zwaginga JJ, van Ham SM, ten Brinke A. IL-10-generated tolerogenic dendritic cells are optimal for functional regulatory T cell induction – a comparative study of human clinical-applicable DC. Clin Immunol (2012) 142(3):332–42. doi:10.1016/j.clim.2011.11.011
167. Raïch-Regué D, Grau-López L, Naranjo-Gómez M, Ramo-Tello C, Pujol-Borrell R, Martínez-Cáceres E, et al. Stable antigen-specific T-cell hyporesponsiveness induced by tolerogenic dendritic cells from multiple sclerosis patients. Eur J Immunol (2012) 42(3):771–82. doi:10.1002/eji.201141835
168. Freedman MS. Efficacy and safety of subcutaneous interferon-beta-1a in patients with a first demyelinating event and early multiple sclerosis. Expert Opin Biol Ther (2014) 14(8):1207–14. doi:10.1517/14712598.2014.924496
169. Schreiner B, Mitsdoerffer M, Kieseier BC, Chen L, Hartung HP, Weller M, et al. Interferon-beta enhances monocyte and dendritic cell expression of B7-H1 (PD-L1), a strong inhibitor of autologous T-cell activation: relevance for the immune modulatory effect in multiple sclerosis. J Neuroimmunol (2004) 155(1–2):172–82. doi:10.1016/j.jneuroim.2004.06.013
170. Offner H, Polanczyk M. A potential role for estrogen in experimental autoimmune encephalomyelitis and multiple sclerosis. Ann N Y Acad Sci (2006) 1089:343–72. doi:10.1196/annals.1386.021
171. McClain MA, Gatson NN, Powell ND, Papenfuss TL, Gienapp IE, Song F, et al. Pregnancy suppresses experimental autoimmune encephalomyelitis through immunoregulatory cytokine production. J Immunol (2007) 179(12):8146–52. doi:10.4049/jimmunol.179.12.8146
172. Papenfuss TL, Powell ND, McClain MA, Bedarf A, Singh A, Gienapp IE, et al. Estriol generates tolerogenic dendritic cells in vivo that protect against autoimmunity. J Immunol (2011) 186(6):3346–55. doi:10.4049/jimmunol.1001322
173. Golshayan D, Pascual M. Tolerance-inducing immunosuppressive strategies in clinical transplantation: an overview. Drugs (2008) 68(15):2113–30. doi:10.2165/00003495-200868150-00004
174. Pascual M, Theruvath T, Kawai T, Tolkoff-Rubin N, Cosimi AB. Strategies to improve long-term outcomes after renal transplantation. N Engl J Med (2002) 346(8):580–90. doi:10.1056/NEJMra011295
175. Golshayan D, Pascual M. Minimization of calcineurin inhibitors to improve long-term outcomes in kidney transplantation. Transpl Immunol (2008) 20(1–2):21–8. doi:10.1016/j.trim.2008.08.006
176. De Serres SA, Sayegh MH, Najafian N. Immunosuppressive drugs and Tregs: a critical evaluation! Clin J Am Soc Nephrol (2009) 4(10):1661–9. doi:10.2215/CJN.03180509
177. Smyth LA, Herrera OB, Golshayan D, Lombardi G, Lechler RI. A novel pathway of antigen presentation by dendritic and endothelial cells: implications for allorecognition and infectious diseases. Transplantation (2006) 82(1 Suppl):S15–8. doi:10.1097/01.tp.0000231347.06149.ca
178. Lechler RI, Batchelor JR. Restoration of immunogenicity to passenger cell-depleted kidney allografts by the addition of donor strain dendritic cells. J Exp Med (1982) 155(1):31–41. doi:10.1084/jem.155.1.31
179. Celli S, Albert ML, Bousso P. Visualizing the innate and adaptive immune responses underlying allograft rejection by two-photon microscopy. Nat Med (2011) 17(6):744–9. doi:10.1038/nm.2376
180. Fu F, Li Y, Qian S, Lu L, Chambers F, Starzl TE, et al. Costimulatory molecule-deficient dendritic cell progenitors (MHC class II+, CD80dim, CD86-) prolong cardiac allograft survival in nonimmunosuppressed recipients. Transplantation (1996) 62(5):659–65. doi:10.1097/00007890-199609150-00021
181. Lu L, Li W, Fu F, Chambers FG, Qian S, Fung JJ, et al. Blockade of the CD40-CD40 ligand pathway potentiates the capacity of donor-derived dendritic cell progenitors to induce long-term cardiac allograft survival. Transplantation (1997) 64(12):1808–15. doi:10.1097/00007890-199712270-00031
182. Lutz MB, Suri RM, Niimi M, Ogilvie AL, Kukutsch NA, Rössner S, et al. Immature dendritic cells generated with low doses of GM-CSF in the absence of IL-4 are maturation resistant and prolong allograft survival in vivo. Eur J Immunol (2000) 30(7):1813–22. doi:10.1002/1521-4141(200007)30:7<1813::AID-IMMU1813>3.0.CO;2-8
183. Yamano T, Watanabe S, Hasegawa H, Suzuki T, Abe R, Tahara H, et al. Ex vivo-expanded DCs induce donor-specific central and peripheral tolerance and prolong the acceptance of donor skin grafts. Blood (2011) 117(9):2640–8. doi:10.1182/blood-2010-07-293860
184. Wise MP, Bemelman F, Cobbold SP, Waldmann H. Linked suppression of skin graft rejection can operate through indirect recognition. J Immunol (1998) 161(11):5813–6.
185. Lee RS, Grusby MJ, Glimcher LH, Winn HJ, Auchincloss H Jr. Indirect recognition by helper cells can induce donor-specific cytotoxic T lymphocytes in vivo. J Exp Med (1994) 179(3):865–72. doi:10.1084/jem.179.3.865
186. Steele DJ, Laufer TM, Smiley ST, Ando Y, Grusby MJ, Glimcher LH, et al. Two levels of help for B cell alloantibody production. J Exp Med (1996) 183(2):699–703. doi:10.1084/jem.183.2.699
187. Hornick PI, Mason PD, Baker RJ, Hernandez-Fuentes M, Frasca L, Lombardi G, et al. Significant frequencies of T cells with indirect anti-donor specificity in heart graft recipients with chronic rejection. Circulation (2000) 101(20):2405–10. doi:10.1161/01.CIR.101.20.2405
188. Baker RJ, Hernandez-Fuentes MP, Brookes PA, Chaudhry AN, Cook HT, Lechler RI. Loss of direct and maintenance of indirect alloresponses in renal allograft recipients: implications for the pathogenesis of chronic allograft nephropathy. J Immunol (2001) 167(12):7199–206. doi:10.4049/jimmunol.167.12.7199
189. Garrovillo M, Ali A, Oluwole SF. Indirect allorecognition in acquired thymic tolerance: induction of donor-specific tolerance to rat cardiac allografts by allopeptide-pulsed host dendritic cells. Transplantation (1999) 68(12):1827–34. doi:10.1097/00007890-199912270-00001
190. Ali A, Garrovillo M, Jin MX, Hardy MA, Oluwole SF. Major histocompatibility complex class I peptide-pulsed host dendritic cells induce antigen-specific acquired thymic tolerance to islet cells. Transplantation (2000) 69(2):221–6. doi:10.1097/00007890-200001270-00005
191. Tanriver Y, Ratnasothy K, Bucy RP, Lombardi G, Lechler R. Targeting MHC class I monomers to dendritic cells inhibits the indirect pathway of allorecognition and the production of IgG alloantibodies leading to long-term allograft survival. J Immunol (2010) 184(4):1757–64. doi:10.4049/jimmunol.0902987
192. Pêche H, Trinité B, Martinet B, Cuturi MC. Prolongation of heart allograft survival by immature dendritic cells generated from recipient type bone marrow progenitors. Am J Transplant (2005) 5(2):255–67. doi:10.1111/j.1600-6143.2004.00683.x
193. Geissler EK. The ONE Study compares cell therapy products in organ transplantation: introduction to a review series on suppressive monocyte-derived cells. Transplant Res (2012) 1(1):11. doi:10.1186/2047-1440-1-10
194. Voigtländer C, Rössner S, Cierpka E, Theiner G, Wiethe C, Menges M, et al. Dendritic cells matured with TNF can be further activated in vitro and after subcutaneous injection in vivo which converts their tolerogenicity into immunogenicity. J Immunother (2006) 29(4):407–15. doi:10.1097/01.cji.0000210081.60178.b4
195. Lim DS, Kang MS, Jeong JA, Bae YS. Semi-mature DC are immunogenic and not tolerogenic when inoculated at a high dose in collagen-induced arthritis mice. Eur J Immunol (2009) 39(5):1334–43. doi:10.1002/eji.200838987
196. Smyth LA, Ratnasothy K, Moreau A, Alcock S, Sagoo P, Meader L, et al. Tolerogenic donor-derived dendritic cells risk sensitization in vivo owing to processing and presentation by recipient APCs. J Immunol (2013) 190(9):4848–60. doi:10.4049/jimmunol.1200870
197. Divito SJ, Wang Z, Shufesky WJ, Liu Q, Tkacheva OA, Montecalvo A, et al. Endogenous dendritic cells mediate the effects of intravenously injected therapeutic immunosuppressive dendritic cells in transplantation. Blood (2010) 116(15):2694–705. doi:10.1182/blood-2009-10-251058
198. Ezzelarab MB, Raich-Regue D, Lu L, Zahorchak AF, Perez-Gutierrez A, Humar A, et al. Renal allograft survival in nonhuman primates infused with donor antigen-pulsed autologous regulatory dendritic cells. Am J Transplant (2017) 17(6):1476–89. doi:10.1111/ajt.14182
199. Dhodapkar MV, Steinman RM, Krasovsky J, Munz C, Bhardwaj N. Antigen-specific inhibition of effector T cell function in humans after injection of immature dendritic cells. J Exp Med (2001) 193(2):233–8. doi:10.1084/jem.193.2.233
200. Dhodapkar MV, Steinman RM. Antigen-bearing immature dendritic cells induce peptide-specific CD8(+) regulatory T cells in vivo in humans. Blood (2002) 100(1):174–7. doi:10.1182/blood.V100.1.174
201. Morelli AE. The immune regulatory effect of apoptotic cells and exosomes on dendritic cells: its impact on transplantation. Am J Transplant (2006) 6(2):254–61. doi:10.1111/j.1600-6143.2005.01197.x
202. Bedini C, Nasorri F, Girolomoni G, Pità Od, Cavani A. Antitumour necrosis factor-alpha chimeric antibody (infliximab) inhibits activation of skin-homing CD4+ and CD8+ T lymphocytes and impairs dendritic cell function. Br J Dermatol (2007) 157(2):249–58. doi:10.1111/j.1365-2133.2007.07945.x
203. Ehrenstein MR, Evans JG, Singh A, Moore S, Warnes G, Isenberg DA, et al. Compromised function of regulatory T cells in rheumatoid arthritis and reversal by anti-TNFalpha therapy. J Exp Med (2004) 200(3):277–85. doi:10.1084/jem.20040165
204. Ruderman EM, Pope RM. The evolving clinical profile of abatacept (CTLA4-Ig): a novel co-stimulatory modulator for the treatment of rheumatoid arthritis. Arthritis Res Ther (2005) 7(Suppl 2):S21–5. doi:10.1186/ar1688
205. Bluestone JA, St Clair EW, Turka LA. CTLA4Ig: bridging the basic immunology with clinical application. Immunity (2006) 24(3):233–8. doi:10.1016/j.immuni.2006.03.001
206. Lan YY, Wang Z, Raimondi G, Wu W, Colvin BL, de Creus A, et al. “Alternatively activated” dendritic cells preferentially secrete IL-10, expand Foxp3+CD4+ T cells, and induce long-term organ allograft survival in combination with CTLA4-Ig. J Immunol (2006) 177(9):5868–77. doi:10.4049/jimmunol.177.9.5868
207. Ko HJ, Cho ML, Lee SY, Oh HJ, Heo YJ, Moon YM, et al. CTLA4-Ig modifies dendritic cells from mice with collagen-induced arthritis to increase the CD4+CD25+Foxp3+ regulatory T cell population. J Autoimmun (2010) 34(2):111–20. doi:10.1016/j.jaut.2009.07.006
208. Hawiger D, Inaba K, Dorsett Y, Guo M, Mahnke K, Rivera M, et al. Dendritic cells induce peripheral T cell unresponsiveness under steady state conditions in vivo. J Exp Med (2001) 194(6):769–79. doi:10.1084/jem.194.6.769
209. Yang J, Brook MO, Carvalho-Gaspar M, Zhang J, Ramon HE, Sayegh MH, et al. Allograft rejection mediated by memory T cells is resistant to regulation. Proc Natl Acad Sci U S A (2007) 104(50):19954–9. doi:10.1073/pnas.0704397104
210. Ehlers MR, Rigby MR. Targeting memory T cells in type 1 diabetes. Curr Diab Rep (2015) 15(11):84. doi:10.1007/s11892-015-0659-5
211. Afzali B, Mitchell PJ, Scottà C, Canavan J, Edozie FC, Fazekasova H, et al. Relative resistance of human CD4(+) memory T cells to suppression by CD4(+) CD25(+) regulatory T cells. Am J Transplant (2011) 11(8):1734–42. doi:10.1111/j.1600-6143.2011.03635.x
212. Garrod KR, Chang CK, Liu FC, Brennan TV, Foster RD, Kang SM. Targeted lymphoid homing of dendritic cells is required for prolongation of allograft survival. J Immunol (2006) 177(2):863–8. doi:10.4049/jimmunol.177.2.863
213. Grover A, Kim GJ, Lizée G, Tschoi M, Wang G, Wunderlich JR, et al. Intralymphatic dendritic cell vaccination induces tumor antigen-specific, skin-homing T lymphocytes. Clin Cancer Res (2006) 12(19):5801–8. doi:10.1158/1078-0432.CCR-05-2421
214. Radomski M, Zeh HJ, Edington HD, Pingpank JF, Butterfield LH, Whiteside TL, et al. Prolonged intralymphatic delivery of dendritic cells through implantable lymphatic ports in patients with advanced cancer. J Immunother Cancer (2016) 4:24. doi:10.1186/s40425-016-0128-y
215. Waldmann H, Adams E, Fairchild P, Cobbold S. Infectious tolerance and the long-term acceptance of transplanted tissue. Immunol Rev (2006) 212:301–13. doi:10.1111/j.0105-2896.2006.00406.x
216. Zhai Y, Meng L, Gao F, Busuttil RW, Kupiec-Weglinski JW. Allograft rejection by primed/memory CD8+ T cells is CD154 blockade resistant: therapeutic implications for sensitized transplant recipients. J Immunol (2002) 169(8):4667–73. doi:10.4049/jimmunol.169.8.4667
Keywords: tolerogenic dendritic cells, autoimmune diseases, immunotherapy, solid organ transplantation, tolerance
Citation: Obregon C, Kumar R, Pascual MA, Vassalli G and Golshayan D (2017) Update on Dendritic Cell-Induced Immunological and Clinical Tolerance. Front. Immunol. 8:1514. doi: 10.3389/fimmu.2017.01514
Received: 12 July 2017; Accepted: 26 October 2017;
Published: 20 November 2017
Edited by:
John Isaacs, Newcastle University, United KingdomReviewed by:
Raymond John Steptoe, The University of Queensland, AustraliaHans Acha-Orbea, University of Lausanne, Switzerland
Copyright: © 2017 Obregon, Kumar, Pascual, Vassalli and Golshayan. This is an open-access article distributed under the terms of the Creative Commons Attribution License (CC BY). The use, distribution or reproduction in other forums is permitted, provided the original author(s) or licensor are credited and that the original publication in this journal is cited, in accordance with accepted academic practice. No use, distribution or reproduction is permitted which does not comply with these terms.
*Correspondence: Déla Golshayan, ZGVsYS5nb2xzaGF5YW5AY2h1di5jaA==
†Present address: Rajesh Kumar, Surgical Oncology Research Laboratory, Massachusetts General Hospital, Harvard Medical School, Boston, MA, United States
‡These authors have contributed equally to this work.