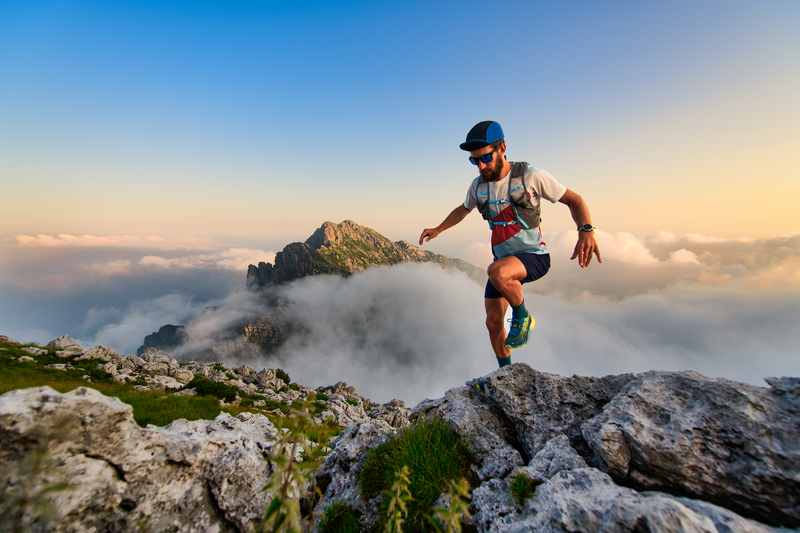
95% of researchers rate our articles as excellent or good
Learn more about the work of our research integrity team to safeguard the quality of each article we publish.
Find out more
ORIGINAL RESEARCH article
Front. Immunol. , 30 June 2017
Sec. Nutritional Immunology
Volume 8 - 2017 | https://doi.org/10.3389/fimmu.2017.00696
Human milk provides a very wide range of nutrients and bioactive components, including immune factors, human milk oligosaccharides, and a commensal microbiota. These factors are essential for interconnected processes including immunity programming and the development of a normal infant gastrointestinal microbiome. Newborn immune protection mostly relies on maternal immune factors provided through milk. However, studies dealing with an in-depth profiling of the different immune compounds present in human milk and with the assessment of their natural variation in healthy women from different populations are scarce. In this context, the objective of this work was the detection and quantification of a wide array of immune compounds, including innate immunity factors (IL1β, IL6, IL12, INFγ, TNFα), acquired immunity factors (IL2, IL4, IL10, IL13, IL17), chemokines (IL8, Groα, MCP1, MIP1β), growth factors [IL5, IL7, epidermal growth factor (EGF), granulocyte colony-stimulating factor, granulocyte–macrophage colony-stimulating factor, TGFβ2], and immunoglobulins (IgA, IgG, IgM), in milk produced by healthy women of different ethnicities living in different geographic, dietary, socioeconomic, and environmental settings. Among the analyzed factors, IgA, IgG, IgM, EGF, TGFβ2, IL7, IL8, Groα, and MIP1β were detected in all or most of the samples collected in each population and, therefore, this specific set of compounds might be considered as the “core” soluble immune factors in milk produced by healthy women worldwide. This approach may help define which immune factors are (or are not) common in milk produced by women living in various conditions, and to identify host, lifestyle, and environmental factors that affect the immunological composition of this complex biological fluid.
Clinical Trial Registration: www.ClinicalTrials.gov, identifier NCT02670278.
Human milk is uniquely suited to the infant’s nutritional needs and is also like a responsive and training substance that protects infants from a wide array of diseases in both developed and developing countries (1, 2). The presence of a wealth of bioactive factors in human milk—including cellular and soluble immune factors (3–5), human milk oligosaccharides (6), and live bacteria (7)—seems to be coordinately responsible for the unparalleled immunological, anti-inflammatory, and anti-infectious properties of this biological fluid. Bioactive components in human milk play key roles in the establishment of an efficient gastrointestinal (GI) barrier and a physiological GI microbiota in infancy, and in the training of the infant immune system, favoring the development of intestinal and systemic immune-homeostasis (8).
Throughout pregnancy, maternal B and T cells are selectively directed from blood and mucosal surfaces, including those of the GI and respiratory tracts, to the mammary gland, where they produce a wide range of immune factors essential to protect the inexperienced, mucosal-associated immune system of the newborn infant (9, 10). Therefore, the lactating mammary gland (and the colostrum and milk it produces) can be truly considered as a relevant part of the infant immune system where breastfeeding provides the postnatal link that promotes maternal–infant immune dialog (11). The effects of such fine programming are long-lasting and, in fact, breastfeeding has been associated to a significant reduction in the rates of allergic and respiratory diseases during adulthood (12–14).
Immunoglobulins (Ig) are the immune factors most studied in human milk. Dimeric IgA or pentameric IgM confer the infant immune protection against antigens to which the maternal mucosal-associated lymphoid tissues (MALTs) have been exposed and, therefore, to which the baby is very likely to be exposed during early life (11). IgA-coated bacteria can be detected in the infant GI tract, providing a mechanistic explanation for the IgA-mediated protection against neonatal infection and sepsis (15). Other immune factors present in human milk, including cytokines, chemokines, and growth factors [e.g., IL6, IL7, IL10, epidermal growth factor (EGF), TGFβ], contribute to differentiation of IgA-producing cells, playing a pivotal role in the maturation of the infant GI-associated immune system and in protecting the newborn against infectious diseases (16).
Maternal environmental factors, such as gestation length, birth mode, diet, time postpartum, or previous antigenic exposures are known to affect the immunological composition of human milk (17–19). Therefore, it is reasonable to assume that the concentrations of these substances in milk produced by healthy women may depend on an individual’s own life circumstances. Previous studies focused on the immunological composition of human milk have assessed a narrow panel of immune factors, have recruited women from a single location, and/or have included a relatively small sample size (20–26). In this context, the objective of this work was the assessment of a wide spectrum of immunological compounds, including innate immunity factors, acquired immunity factors, chemokines, growth factors, and Ig, in milk produced by healthy women of different ethnicities, living in high-, middle-, and low-income countries and, therefore, including very different geographical, dietary, socioeconomic, and environmental settings. International cohort studies, such as this, are fundamental in determining if there is a common set of “core” immune factors naturally present in human milk under various physiological conditions. In addition, studies such as this are needed to identify host, lifestyle, and environmental factors associated with (1) the presence/absence of and (2) variation in the concentration of these (and other) human milk-borne immunomodulatory constituents. Our overarching hypothesis was that “normal” varies in terms of immune components of human milk.
This investigation took place between May 2014 and April 2016 and was carried out as a cross-sectional, observational study involving eight contrasting countries. A total of 410 healthy breastfeeding women initially participated in the study, which was designed primarily to characterize global variation in the milk microbiome and oligosaccharide profiles. Results concerning the latter have been published previously (27). To be eligible for participation, women had to be breastfeeding or expressing milk at least five times daily (to assure adequate milk production); self-reported as healthy and nursing healthy infants; ≥18 years of age; and between 2 weeks and 5 months postpartum. Women did not need to be exclusively breastfeeding. Exclusion criteria included current indication of breast infection or breast pain that the woman did not consider “normal” for lactation; maternal use of antibiotics in the previous 30 days; or nursing a child with signs and/or symptoms of acute illness in the previous 7 days or who had taken antibiotics in the previous 30 days.
Our original sample included two European (Spanish and Swedish), one South American (Peruvian), two North American (USA), and six sub-Saharan African [rural and urban Ethiopian (ETU), rural and urban Gambian, Ghanaian (GN), and Kenyan] cohorts. Samples collected from rural Ethiopian women, however, were not analyzed in this work because they were initially preserved using a chemical preservative (rather than being frozen). Therefore, a total of 370 samples were included in the immunological analysis.
Spanish (SP) subjects (n = 41) were recruited in Madrid, Zaragoza, Huesca, and Vizcaya. Swedish (SW) subjects (n = 24) were recruited in or near Helsingborg and were self-reported as Nordic (both parents and all grandparents self-described as having only Swedish, Finnish, Danish, Icelandic, or Norwegian heritage). Peruvian (PE) subjects (n = 43) resided in a peri-urban area of Lima. The North American subjects were recruited in southeastern Washington and northwestern Idaho [USA/Washington (USW); n = 41] and southern California (USC; n = 19), the former being of unspecified ethnicity and the latter self-identified as Hispanic. ETU (n = 40) subjects self-identified as Sidama and resided in Hawassa, in the Southern Nations, Nationalities, and Peoples’ Region. Rural and urban Gambian (GBR and GBU, respectively) subjects self-identified as Mandinka. Urban Gambian participants (n = 40) were selected from the Bakauarea, while the rural cohort (n = 40) lived in the West Kiang region. Ghanaian subjects (n = 40) were Krobo or Dangme and lived in southeastern Ghana. Kenyan (KE) subjects (n = 42) were recruited from the multiethnic city of Nakuru.
Upon enrollment, each woman completed several questionnaires including one that ensured eligibility and another related to general maternal and infant health and anthropometry (Table 1). Data analyzed to examine possible influence from environmental, behavioral, and individual characteristics on immune composition of milk included the presence of animals in the home, birth mode, maternal and infant health problems and medication use, anthropometric measurements, dietary intake, maternal and infant age, and time since last feeding. Animals in the home referred to all pets and/or livestock that were kept, at least part of the day, within the home and included any and all types of animals (e.g., dogs and cats in the US and cattle in Ethiopia). Mothers also reported whether the focal infant was born vaginally or via cesarean section. The presence or absence of health problems and illnesses for the mother and focal infant in the postpartum period were collected through maternal reports. Mothers also recounted whether they received medication during the birth and whether they or the focal infants took medications in the postpartum period. Medication was widely defined, including Western pharmaceuticals and traditional, local remedies (e.g., medicinal teas). Our current analysis on dietary intake was limited to whether or not the mother was advised to consume fermented or cultured foods or beverages. Maternal and infant age was calculated to the nearest day. At some sites mothers did not know their or their infants’ birth dates. In such cases, maternal age was estimated based on the mother’s estimate with consideration given to her reproductive history (i.e., number of children both living and deceased). When an infant’s date of birth was not known, age was estimated based on the mother’s recollection and in some cases local events and conversations with nurses who knew the population. The precision of these estimates is likely to be excellent due to the age of the infants enrolled: little time had passed, thus decreasing the likelihood of error in self-reports. Body mass index (BMI) was calculated from mothers’ weights and heights. Time since last feeding was collected through maternal self-report. Mothers reported an estimated amount of time since the infant nursed on the breast of choice for sample collection.
For each country, the human development indexes (HDI) from the United Nations Development Program (28) were also taken into consideration. HDI for each country was classified as low human development (LHD), which includes ETU, GBR, GBU, and KE; medium human development (MHD), which includes GN; high human development (HHD), which includes PE; or very-high human development (VHHD), which includes SP, SW, USC, and USW.
Ethics approvals were obtained for all procedures from each participating institution, with overarching approval from the Washington State University Institutional Review Board (#13264). After being translated from English (when needed), informed, verbal, or written consent (depending on locale and the subject’s literacy level) was acquired from each participating woman.
Using gloved hands, research personnel or the mother (depending on cultural acceptability) cleaned the “study breast” (chosen by subject) twice with prepackaged castile soap towelettes (Professional Disposables International, Inc.; Orangeburg, NY, USA) using a newly opened package each time. When deemed appropriate, this step was preceded by a general cleansing with water (and soap if needed) to remove noticeable soil. In PE, SW, USC, and USW cohorts, at least 20 mL (typically 40–60 mL) of milk samples were then collected into a single-use, sterile polypropylene milk collection container with a polybutylene terephthalate cap (Medela, Inc.; McHenry, IL, USA) using an electric breast pump. In the remaining sites, ~20 mL of milk were collected. In SP, milk samples were collected via manual expression (using a gloved hand) into single-use, sterile polypropylene milk collection containers with polybutylene terephthalate caps (Medela, Inc.; McHenry, IL, USA). In the remaining sites, milk was manually expressed (using a gloved hand) into sterile polypropylene specimen containers with polyethylene caps (VWR International, LLC.; Visalia, CA, USA). To help control for known and unknown biases that might be introduced by using different materials, all milk collection supplies (gloves, wipes, collection containers, etc.) were standardized and provided to study personnel at each site.
Milk was immediately placed in ice or in a cold box (4°C) where it remained until it was partitioned, within 1 h, into aliquots. Milk was immediately frozen (−20°C) and, then, transferred to −80°C for long-term storage. Samples collected outside from Madrid were shipped on dry ice (−78.5°C) to the Complutense University of Madrid where all the immunological determinations were performed. In order to eliminate or minimize potential lab biases, all the samples were submitted to a single freeze–thaw cycle and were analyzed by the same researchers using the same reagents’ batches and equipment.
The concentrations of innate immune factors (IL1β, IL6, IL12, IFNγ, TNFα), acquired immunity factors (IL2, IL4, IL10, IL13, IL17), chemokines (IL8, Groα, MCP1, MIP1β), and growth factors [IL5, IL7, granulocyte colony-stimulating factor (GCSF), granulocyte–macrophage colony-stimulating factor (GMCSF), TGFβ2] were determined by magnetic bead-based multiplex immunoassays, using a Bioplex 200 instrument (Bio-Rad, Hercules, CA, USA) and the Bio-PlexPro Human Cytokine, Chemokine, and Growth Factor Assays (Bio-Rad), according to manufacturer’s instructions. TGFβ2 was acid activated prior to the analysis as recommended by the manufacturer. EGF was determined by ELISA using the RayBio Human EGF ELISA kit (RayBiotech, Norcross, GA, USA). Concentrations of Ig (IgA, total IgG, and IgM) were determined using the Bio-Plex Pro Human Isotyping Assay kit (Bio-Rad) in the Bioplex system instrument.
Prior to their analysis, samples (1 mL) were processed and aliquoted as described previously (29). A fresh aliquot was used for each assay, avoiding defrosting cycles. Every assay was run in duplicate according to manufacturer’s instructions, and standard curves were performed for each analyte on every assay. Cytokine concentrations were expressed as nanograms per liter, Ig concentrations as milligrams per liter, and concentrations of EGF, TGFβ2, and Groα as micrograms per liter. The inter-assay coefficients of variation were below manufacturers’ instructions for all the immune markers, and the detection limits of the assays are shown in Table S1 in Supplementary Material.
Normality of data distribution was interrogated through visual inspection of histograms and Shapiro–Wilk test, both evidencing non-normal distribution for all tested variables (p < 0.05). Accordingly, non-parametric statistical analyses were used. Differences in recorded demographic data and detection frequencies of the immunological compounds were evaluated among locations by Fisher test followed by a post hoc Nemenyi test adjusted to χ2 statistics for pairwise multiple comparisons. Descriptive univariate analysis was performed comparing the concentrations of all parameters analyzed for the 10 different subpopulation groups through unadjusted Kruskal–Wallis test and further post hoc Nemenyi test for pairwise multiple comparisons. For each pairwise combination of the immunological compounds analyzed, the Sørensen–Dice index was calculated, and a co-occurrence matrix was constructed. A heatmap representing the values of co-occurrence indexes was then plotted. To summarize the results of the immunological profiles, exploratory multivariate analyses, such as principal component analysis (PCA) with a variable reduction approach (cos2 > 0.2), were performed using the R package FactoMineR.
Agglomerative hierarchical clustering, using the Euclidean distance and Ward methods (R package: dendextend), was used to study the binary matrix of detection and the measured amount matrix of immune factors included in the study. Subsequently, a heatmap representing the detection of the immune factors with the sample labels replaced by a colored bars vector for HDI classification was plotted. The dendrogram obtained for measured amounts of immune factors was also represented as a circularized tree of the samples colored by location. To investigate potential associations between the immunological variables and the categorical variables describing demographic aspects of the populations, generalized linear models (GLMs) were performed. Significance was declared at p < 0.05 for all analyses. All analyses were performed with the R software version 3.3.2 (R-project, http://www.r-project.org).
Maternal health, infant health, lifestyle, and anthropometric parameters that were analyzed in this study are shown in Table 1. Analysis of the data by the Friedman rank sum test revealed significant differences for all the parameters among all the populations. Notable differences included (a) maternal age, which was the highest in the SP cohort (median: 34.0 years) and the lowest in ETU (median: 20.5 years); (b) postpartum days at the time of sample collection, a period that was the shortest in SW (median: 42 days) and the longest in KE (median: 74 days); and (c) C-section rates, which ranged from 48.8% in Peru to 0% in ETU and GBR. Globally, infant medication rate was highest in KE (88.5%), while maternal medication was more frequent among USW, where 56.1% of the mothers received medication (different from intrapartum antibiotic therapy) during pregnancy or delivery, and 91% of them declared that they had received medication during the postpartum period. ETU and PE mothers presented the lowest rates of postpartum medication as 97.5 and 86.0% of subjects, respectively, reported no postpartum medication.
All the immunological factors could be detected among at least some of the human milk samples analyzed in this study, although at highly variable frequencies and concentrations. Globally, IgA and EGF displayed the highest frequencies of detection (100% of the samples), followed by IgG, IgM, TGFβ2, IL7, IL8, and Groα, which were detected in most of the samples collected from each population (Table 2; Figure 1). The detection frequency of MIP1β was high (>91%) in all populations with the exception of samples from USW (51%). IL1β, TNFα, GCSF, IL6, IL13, and MCP1 were also detected in all the populations, but their frequencies varied depending on the group. Some immune compounds exhibited intermediate frequencies of detection in certain locations but could not be detected among samples collected elsewhere. They included IL2 (detected exclusively in some GN samples), IL4 (not detected in USW, SW, and GBU), IL10 (not detected in SW, USC, and USW), IL17 (not detected in SW and USW), IL5 (not detected in GBU, SW, and USW), IL12 (not detected in USW), and INFγ (not detected in USW). Finally, low frequencies of detection were found for GMCSF, which was detected in less than 10% of the samples within each group; except GN where it was detected in 50% of the samples; and with the exceptions of SW, USC, and USW where this factor could not be detected in any sample. IL2, IL17, and IL4 were also found in very low frequencies: <18, 22, and 33%, respectively, across all locations.
Table 2. Relative frequencies of detection of each immune factor in human milk within each population.
Figure 1. Heatmap representing the detection frequency of the immunoglobulins, cytokines, growth factors, and chemokines quantified in human milk (green: present; grey: absent). Each row represents the detection frequency for an individual specific immune factor as indicated on the y-axis, and each column represents an individual sample/subject. Hierarchical clustering of the 365 samples analyzed is shown in the upper dendrogram. Color-coded bar accompanying the dendrogram represents the level of development of the geographical locations analyzed, according to the human development index [United Nations Development Program; (28)]. LHD, countries with low human development index; MHD, countries with medium human development index; HHD, countries with high human development index; VHHD, countries with very-high human development index.
Median values of all the immune factors analyzed in this study are recorded in Tables 3–5 and are also summarized as a heatmap in Figure 2A, as described below.
Table 3. Median concentration (in nanograms per liter) and interquartile ranges for the factors in human milk associated to innate immunity.
Table 4. Median and interquartile ranges for the measured concentrations of factors in human milk associated to acquired immunity.
Table 5. Median and interquartile ranges for the measured concentrations of chemokines and growth factors in human milk.
Figure 2. Heatmaps representing the median concentrations of different immune factors in each location where the samples were collected from. (A) Heatmap representing the median concentrations of all the immune factors assayed in this study. (B) Heatmap representing the median concentrations of the 16 immune factors that contributed the most to samples separation according to the multivariate analysis conducted in this work (cos2 > 0.2). SP, Spain; USW, USA/Washington; SW, Sweden; USC, USA/California; GN, Ghana; ETU, urban Ethiopia; GBU, urban Gambia; PE, Peru; GBR, rural Gambia; KE, Kenya.
The concentrations of the innate immunity factors IL1β, IL6, IL12, and TNFα displayed significant differences across geographical locations, in contrast to those of INFγ, which was relatively consistent across cohorts (Table 3). Although IL1β displayed the lowest values in all locations, significant differences were detected among groups: SP samples showed the highest (median: 1.14 ng/L; range: 0.27–2.61 ng/L) and USC the lowest (median: 0.12 ng/L; range: 0.06–0.25 ng/L) concentrations. The lowest IL6 and IL12 concentration values were recorded in USC, USW, and SW, where median concentrations ranged from 2.13 to 3.61 ng/L for IL6, and from below detection to 0.86 ng/L for IL12. On the contrary, the highest concentrations of these two factors were detected in ETU, KE, GBU, and SP for IL6 (range: 12.51–15.37 ng/L) and in KE and GBR for IL12 (range: 4.16–4.46 ng/L). The lowest values of TNFα were detected in GBU (median: 1.27 ng/L; range: 0.91–2.32 ng/L) and the highest in GBR (median: 8.63 ng/L; range: 5.23–13.50 ng/L), followed by KE (median: 6.83 ng/L; range: 2.08–12.47 ng/L). All the other locations showed intermediate TNFα levels ranging from 3.18 to 4.98 ng/L (Table 3; Figure 2A).
The highest IgA concentrations were found in SW, USC, and USW samples with median concentrations ranging from 1,210 to 1,840 mg/L. Interestingly, samples from these same locations contained the lowest IgG and IgM concentrations, ranging from 15.31 to 32.37 mg/L and from 12.27 to 18.95 mg/L, respectively (Table 4). Among the other factors related to acquired immunity, concentrations of IL4, IL10, IL13, and TGFβ2 were different across locations, but no clear patterns were observed except that once again, in SW, USC, and USW concentrations of IL10 were below the assay detection limit (Table 4). Concentrations of IL7 were lower in SW, USC, USW, and GBU (range: 11.10–13.92 ng/L) when compared to other locations (range: 32.14–91.61 ng/L), with the exception of GN (median: 2.39 ng/L). In relation to this location, the levels of acquired immunological factors in GN samples were quite different when compared to those exhibited by the other African locations: GN samples had the lowest IL13 and IL7 concentrations and the highest IgA and IgG concentrations among the African samples (Table 4). It must also be highlighted that, among all the samples analyzed in this study, IL2 was only detected in seven GN samples. No differences were found in IL5 and IL17 concentrations among the locations where these two factors were above the detection limits of the assay (Table 4; Figures 2A,B).
Groα was the most abundant chemokine in the milk samples analyzed in this study and, in fact, its levels were between 100- and 500-fold greater than those obtained for the rest of chemokines (Table 5; Figures 2A,B). Concentrations of all chemokines were significantly different across geographical locations (Table 5). IL8 exhibited the highest concentrations (54–98 ng/L) in SP, PE, and in the African locations with the exception, again, of GN (~7 ng/L). Median IL8 levels in SW, USC, and USW ranged from 5 to 22 ng/L. MCP1 concentrations were also lower in SW, USC, and USW (range: 14.32–52.60 ng/L), as compared to the other locations (126–252 ng/L). No clear patterns were observed in the distribution of Groα and MIP1β concentrations across the locations. Globally, KE samples displayed higher chemokine concentrations than those from other study sites.
In relation to growth factors, no significant differences were found for GMCSF concentrations while GCSF and EGF showed significant variation depending on the location (Table 5; Figures 2A,B). Interestingly, GCSF and EGF showed opposite trends (lower GCSF concentrations, higher EGF concentrations) in the samples from VHHD locations (SP, SW, USC, and USW).
The detection frequencies of the immune compounds were further evaluated by clustering analysis and heatmap plotting (Figure 1). Globally, these analyses suggest that the immune profiles of milk samples from healthy breastfeeding women can be, at least in part, differentiated according to the geographic origin of the samples’ donors. At a linkage distance of three, hierarchical clustering of detection frequencies of the immune factors showed eight different clusters, showing a high consistency with the HDI of the countries where samples were obtained. Some clusters were highly enriched in individuals from a specific geographical location, as it was the case of clusters I, II, and VII, which mainly encompassed samples from VHHD locations (SP, SW, USW, and USC); most of the samples from the MHD location (GN) are included in cluster III, which also contained some samples from LHD (ETU, GBR, GBU, and KE) and HHD (PE) locations. Clusters IV and VIII mostly comprised samples from LHD locations, and clusters V and VI were heterogeneous, including samples from LHD, HHD, and VHHD locations.
IgA, IgG, IgM, TGFβ2, EGF, IL7, IL8, and Groα formed a “core” set of immune factors that were detectable in all or most of the samples analyzed in this work, independent of the location where the samples were collected. Among the immune factors that allowed differentiation of samples in clusters, IL10 and/or IL13 were frequently absent in clusters enriched in samples from HHD locations (I, II, and VII). MIP1β was absent in most samples from cluster I, encompassing a great proportion of the samples from VHD locations. Likewise, IL4, IL17, and GMCSF detection was mostly limited to some samples from cluster III. In addition, the vast majority of samples from LHD and MHD locations (such as those grouping together in clusters III, IV, and VIII) were frequently characterized by detection of MCP1, IL10, IL1β, GCSF, and TNFα. In addition, GMCSF was detectable in many GN samples (MHD), whereas its detection in other samples was limited. Remarkably, the number of immune factors with concentrations below the detection limits was higher in samples from more highly developed locations (median: 11) when compared to those collected in regions with lower development (median 7) (Kruskal–Wallis, p < 0.05). In addition, four of the factors determined in this study (IL10, IL5, IL12, and INFγ) could only be detected in samples from LHD locations and were not detected in any from the highly developed ones. Concentration profiles of the immune factors studied also clustered with location and HDI classification of the location where samples were collected from (Figures S1–S3 in Supplementary Material).
We also performed an analysis to determine the co-occurrence profiles among the 23 immune factors evaluated in this study. For this purpose, the Sørensen–Dice similarity index was calculated individually for developed (USC, USW, SW, SP, PE) and developing (GBR, GBU, GN, KE, ETU) countries; these results are illustrated in a heatmap (Figures 3A,B). Hierarchical clustering evidenced three different clusters (a high co-occurrence cluster, a medium co-occurrence cluster, and a low co-occurrence cluster) in the two analyzed settings. However, the association pattern of immune factors belonging to each cluster was different among locations. Remarkably, the number of immune factors displaying a high co-occurrence was higher in developing locations (n = 15) as opposed to samples from developed settings (n = 11). As an example, while Ig, EGF, and TGFβ2 displayed high co-occurrence in both settings, the high co-occurrence cluster included also MCP1, IL1β, IL10, GCSF, and TNFα in developing locations. In addition, the number of low co-occurrence factors samples was lower in developing locations (n = 3) than in developed locations (n = 6). In this context, some of the immune factors that were predominantly detected in developing locations (e.g., IL5 or INFγ) exhibited medium co-occurrence in such locations but low co-occurrence in the developed regions.
Figure 3. Heatmaps representing the co-occurrence frequency among immune factors analyzed in human milk, as determined per Sorensen–dice index in developed (A) and developing (B) countries. Lighter colors indicate strongest co-occurrence frequency.
Principal component analysis-based clustering of the detected concentrations of immunological factors was performed to summarize and discriminate sample subgroups based on their immune profiles. A total of five independent components with eigen value >1 globally explained 66% of the observed variability. This analysis showed that the immune factors IL1β, IL2, IL5, IL13, IL17, GMCSF, and INFγ exhibited a cos2 < 0.2 and, thus, had little or no contribution to the samples’ positioning along the bidimensional map (they are represented as dashed red arrows in the factor maps). On the other hand, among the variables contributing the most to samples’ positioning in the bidimensional map, high IgA levels seemed to be driving the separation of samples from VHHD locations (Figures 4A,D), while IgG and IL12 seemed to condition the position of the GN samples (Figures 4C,F) and Groα and IL7 that of the Peruvian samples in relation to the rest of the locations (Figures 4B,E). Median values for those immune factors exhibiting a cos2 > 0.2 are summarized as a heatmap in Figure 2B.
Figure 4. Principal component analysis (PCA) for the concentration of various immune factors in human milk. Top panels (A–C) represent separation into bidimensional maps, each one representing different dimension combinations. Arrows indicate variable factors contributing the most to the separation of the samples along the map. Variables with a cos2 > 0.2 are represented in blue and those with a lesser contribution to the graph are indicated in red dashed arrows. Lower panels (D–F) represent the distribution of the samples’ centroids of the different geographical locations (pink letters) along the same dimension combinations displayed in the respective upper panels (A–C). Ellipsoids include at least 50% of the individuals belonging to each of the represented categories, which include VHHD, HHD, MHD, and LHD countries [United Nations Development Program; (28)].
Furthermore, three major sample groups were detected; one included samples from SW and USC/USW, and located separately from all the rest of the samples. A second independent group of samples included those from GH, the only MHD country participating in this study. A third group contained the samples from PE and the LHD locations. Samples from Spain, a VHHD country, were positioned between the first and the third group of samples (Figures 4D–F).
Since TNFα/IL10 and IL10/IL12 ratios have been associated with pro-inflammatory and anti-inflammatory states, respectively, they were further studied (Figure 5) (30). Overall, the highest anti-inflammatory ratios were found in samples from developing countries; among them, the KE samples exhibited the most pronounced ratio; and samples from USC, USW, and SW exhibited the lower ratios (p < 0.05). On the contrary, samples from VHHD countries, and particularly those from USC, USW, and SW, exhibited the highest TNFα to IL10 ratio as opposed to the GBU ones, which showed the lowest ratio.
Figure 5. The ratio of TNFα/IL10 and IL10/IL12 in human milk ratios per geographical location. ETU, urban Ethiopia; GBR, rural Gambia; GBU, urban Gambia; GN, Ghana; KE, Kenya; PE, Peru; SP, Spain; SW, Sweden; USC, USA/California; USW, USA/Washington. Letters on top of each bar indicate significant differences (p < 0.05) on pairwise post hoc comparisons for each group (Kruskal–Wallis, Nemenyi post hoc test).
The effect of potential associations between the participants’ characteristics and the immunological variables was evaluated using a GLM. Those that were found to be statistically significant are summarized below, while p-values of the GLMs are shown in Table S2 in Supplementary Material. Among the participant characteristics, only delivery type, maternal age, time postpartum, and time since last feeding showed a significant covariation with location for some of the immunological factors evaluated. Groα covaried simultaneously with type of delivery, maternal age, and time postpartum. EGF and IL7 covaried with time from last feeding and type of delivery, respectively, while MIP1β and TNFα covaried with time postpartum.
Results from this study strongly support the concept that there is a common, but relatively small, “core” set of immunological soluble compounds present in mature milk produced by relatively healthy women, independent of their geographical location. We posit that these compounds are fundamentally important to infant (and/or mammary) health, regardless of contextual situation. Conversely, presence and/or concentration of many other immunological compounds vary geographically, which is in agreement with companion study conducted by us that focused on human milk oligosaccharides profiling within the same cohort (27). Perhaps these more “variable” substances are differently important, depending on location, environmental pathogen stress, hygiene, cultural norms, etc.
In the past, human milk was mainly considered as a source of nutrients for the developing infant. However, repetitive observations that breastfeeding significantly reduces morbidity and mortality rates associated with common diseases in both developed and developing countries have led to the recognition of additional key roles of human milk for infant health and homeostasis (31). From an immunological point of view, human milk contains a large number of immune elements (immune cells, cytokines, chemokines, growth factors, Ig, etc.) that provide passive protection during this period of host defense vulnerability (32). In addition, such elements contribute to the active maturation and shaping of the infant’s immune system and mucosal barriers (33, 34). Indeed, the lactating human mammary glands are a fundamentally relevant part of the MALT system during this crucial period of life (35). The mother’s mature immune system reacts efficiently to microorganisms and allergens to which she and her infant are exposed. Breastfeeding provides an ingenious immunologic integration between the mother’s and the infant’s immune systems (11).
Despite the recognized importance of milk’s immune factors (together with other bioactive factors) for the protection and development of the breastfed infant, studies on natural variations of the immunological composition of human milk among healthy women living in different geographical, dietary, and socioeconomical settings are scarce (25). To shed some light on this research gap, the present work investigated the presence and concentration of 23 soluble immune factors in a relatively large number of milk samples collected using matched protocols from healthy mothers living in high-, middle-, and low-income countries. Globally, our results provide considerable evidence that human milk immune factors exhibit high inter- and intra-variability across different populations, in agreement with previous observations (36, 37). Among the analyzed factors, only IgA, IgG, IgM, EGF, TGFβ2, IL7, IL8, Groα, and MIP1β were detected in all or most of the samples collected in each population at variable, yet biologically relevant, concentrations. TGFβ2, EGF, Groα, and IL8 were also detected in all the milk samples in previous studies (36, 37). Therefore, this specific set of compounds might be considered as the “core” soluble immune factors in milk produced by healthy women worldwide. Each of these factors has key roles in the barrier and immunological functions of the breastfed infant. They might also be important in protecting the mammary gland from disease during lactation.
Passively acquired maternal antibodies are important for protection against some pathogens in the neonatal period and promote long-term intestinal homeostasis by regulating the GI microbiota and host gene expression (38). Secretory IgA (sIgA) is the predominant Ig class found in human milk, compensating for the IgA deficiency of the infant and strongly contributing to the prevention of infant respiratory and GI infectious diseases (11). Both human milk IgA and IgM are active against a wide spectrum of viruses, bacteria, protozoa, yeast, and molds, inhibiting pathogens colonization and invasion (15, 33). Immune exclusion of antigens is performed mainly by sIgA in cooperation with innate defenses, but secretory IgM is also very relevant for neonatal health, being required for inactivating some Gram-negative pathogens (34, 39). In addition, sIgA seem to exert a role in the regulation of the immune response to dietary antigens since some studies have described an inverse relation between milk IgA levels and the development of allergy (40, 41).
Similar to IgA, the amount and repertoire of IgG produced by infants are clearly deficient because antigen-exposed memory T cells have not yet been generated. Transplacental transfer of IgG only partially corrects this deficiency since passively acquired IgG decrease rapidly after birth. The infant begins to actively produce IgG on exposure to antigens, but the complete antibody response is not achieved until 4–5 years of age, making infants particularly sensitive to encapsulated organisms (34), thus highlighting the protective value of breastfeeding in relation to mucosal infections.
Cytokines, chemokines, and growth factors are pluripotent polypeptides that operate in networks and coordinate the development and functions of the immune system. In the past, the study of such soluble factors in human milk has been difficult because of their complexity, their relatively low concentrations, and the lack of specific procedures and reagents to quantify such agents in this biological fluid. However, the number of such compounds that have been detected in human milk is growing rapidly. Although the actual physiologic effects of each of these factors in the infant have not been elucidated completely, their presence seems to be extraordinarily relevant for infant and mammary health (42, 43). Therefore, there is a growing interest in their roles and complex interactions, not only among them but also with other immunological and defense factors present in milk and/or the infant GI tract (lysozyme, lactoferrin, HMO, mucins, functional lipids, antimicrobial peptides and proteins, polyamines, microorganisms, etc.) (44).
In agreement with the results of our work, previous studies have shown that presence of variable (but usually high) concentrations of TGF-β2 is a common feature of human milk under physiological conditions (45, 46). TGF-β is considered as a key immunomodulatory factor in human milk (47, 48), and its importance is highlighted by the fact that endogenous GI TGF-β synthesis is defective in the neonate (49). TGF-β is critical for oral tolerance induction and global regulation of intestinal immune responses after food ingestion (50, 51). Epidemiologic studies have shown a positive correlation between levels of TGF-β in human milk and protection against wheeze and atopic dermatitis in breastfed children (52, 53), while animal studies have demonstrated the ability of TGF-β to prevent allergy in allergic-prone rats (54) and intestinal mucosa inflammation (55). In addition, TGF-β2 specifically attenuates IL1β-induced inflammatory responses in the immature human intestine via an SMAD6- and ERK-dependent mechanism (56). More recently, it has been observed that TGF-β2 and endotoxin interact to regulate homeostasis via IL8 levels in the immature intestine (57).
Chemokines are well known for their classic leukocyte chemoattractant activity, which is critical for directing the immune response to sites of infection and injury (58). Our work suggests that chemokines Groα (or CXCL1), IL8 and, to a lesser extent MIP1β, are included in the immunological core of human milk. Groα plays a role in spinal cord development by inhibiting the migration of oligodendrocyte precursors (59). This chemokine decreased the severity of multiple sclerosis in a mouse model and may provide a neuroprotective function (60). Additionally, Groα is involved in some processes that are essential in early life, such as angiogenesis and wound healing (61, 62).
Previous work investigating the presence of some chemoattractant factors (IL8, RANTES, eotaxin, IL16, MIP1α) in human milk revealed that only IL8 was present in 100% of the samples (63). Therefore, this chemokine may be particularly relevant for the trafficking of leukocytes from maternal circulation to the mammary gland and into milk. The production of IL8 by neonatal cells is reduced compared with adult cells (64) but, as with other immune factors, this developmental delay may be compensated for by ingesting human milk. A recent study reported that IL8 levels decline with stage of lactation (65). This is in contrast with our data since the mean concentration of this chemokine in KE samples (median: 74-day postpartum) was greater than in SW samples (median: 42-day postpartum). This suggests that there may be other factors influencing or determining IL8 levels in human milk.
In relation to growth factors, EGF was present in all the samples analyzed in this study. EGF enhances proliferation and differentiation of epithelial cells in the GI tract (16) and has significant effects on healing of damaged mucosa after injury (66, 67). The major sources of EGF for the infant GI tract are human colostrum and mature milk (68, 69). EGF in human milk has a protective effect against neonatal intestinal diseases, such as necrotizing enterocolitis (NEC) (70). This EGF-mediated protection against NEC has been associated to the well-known role of this growth factor in altering the balance of pro-apoptotic and anti-apoptotic proteins (71). Oral administration of EGF to rats with NEC-like symptoms decreased intestinal permeability, increased mucin production by goblet cells, and improved intestinal structure (72). All these changes improved GI integrity and enhanced intestinal barrier function. EGF may also contribute to the increased thymus size of breastfed (compared to formula-fed) infants (73). This might lead to a more advanced T lymphocyte differentiation and maturation, and consequently reduced risk of self-induced autoimmune disease. In this context, levels of IL7, a cytokine recently described in human milk and a common feature of the samples analyzed in this study, may correlate with improved thymus function in children (74).
The remaining cytokines, chemokines, and growth factors were found at variable detection frequencies and concentrations depending not only on the locations but also from one mother to another. Therefore, they could be considered as the “variable” set of soluble immune factors in human milk. Such physiological changes in the profile of cytokines may reflect individual patterns in the immune system of the mammary gland or the evolving needs of the recipient infants (75).
Despite this otherwise expected variability, the immune profiles obtained in this study allowed the clustering of the samples into groups highly concordant with the geographical origin of the samples and/or the HDI of the corresponding locations. Globally, the profiles in developing locations were consistent with a greater immune response plasticity, capable to exert protection against a broad range of stimuli, as supported by the higher number of high co-occurrence factors, including immune modulators (IL10 and GCSF) and acute response mediators (IL1β and MCP1), and the higher anti-inflammatory IL10/IL12 ratio. In the frame of the “Hygiene Hypothesis” this may reflect a higher level of maternal exposure to microorganisms and other antigens, which have been traditionally associated with developing countries. On the contrary, the immune profile of samples from developed locations was characterized by the low number of detected immune factors and the higher levels of IgA and EGF. This is consistent with a dominance of B cell activity as opposed to T cell-mediated immunity, suggesting a role for some practices that are generalized in Western lifestyle countries (e.g., hygienic birth practices, reduced contact with animals, safe food, water sanitation, sewage treatment, vaccination, use of antibiotic, anti-inflammatory or corticoids drugs, etc.). In this context, it is worth noting that the two populations from Gambia included in this study, with the same ethnic origin but living in two different environmental settings (rural and urban), exhibited significant differences in some immune factors. Additional studies will be needed to understand the drivers of these differences. Nonetheless, globally, the detection frequency and the concentration of pro-inflammatory TNFα and the detection frequency of some factors related to acquired immunity (IL17 and IL5), known to develop through life as a result of antigenic exposures and, therefore, tightly related to environmental pressures, were greater among the women living in the rural environment. In addition GCSF, known to participate in dendritic cells maturation and macrophage activity, exhibited detection frequencies much higher in the rural (75%) than in the urban population (25%). It is also worth remarking that TNFα to IL10 ratios were generally higher in the rural population. The factors that may explain, at least partly, the differences between these two genetically related populations include (a) less contact with animals, which is associated to a reduced contact with microorganisms (76) and (b) a higher BMI in GBU women, a factor generally associated with a state of inflammation and a negative impact on host immunity (77).
In this study, significant differences were found in several demographic, anthropometric, and mother/infant health-related factors but, as determined by GLMs, few significant associations could be established between these factors and immune profiles. This is presumably due to the high heterogeneity of the studied populations, which makes necessary a very high number of participants in order to elucidate the influence of different host and environmental factors on the immune composition of human milk. Recent work highlighted that, even within more homogeneous populations, the high variability in both milk immune profiles and environmental characteristics of the subjects might hinder the establishment of robust correlations (25). In fact, our results showed the existence of a significant covariation between some of the immune factors and certain demographic characteristics, such as including postpartum time or maternal age (Table S2 in Supplementary Material); unfortunately, no clear patterns could be identified, probably due to the high degree of variation in the demographic characteristics among the different populations analyzed in this study. Nonetheless, multivariate analyses revealed that samples from similar socioeconomic environments tend to cluster together, suggesting that common pressures might drive the presence of specific immune factors in human milk which, eventually, might be evolutionary fixed (78).
As stated above, several factors have been suggested to affect the immune composition of human milk, including the health status of the mother-infant dyad (18, 20, 79–81). Increased exposure to pathogens, as those occurring in clinical and subclinical mastitis (82, 83) and during infectious disease of the breastfed infant (84–86), might relate to increased pro-inflammatory factors in human milk. Although all the subjects recruited for the study were self-identified as healthy, the study could be biased as we cannot exclude the possibility that some of the participants were incubating or suffered from non-diagnosed or subclinical infections.
Time postpartum was another characteristic exhibiting variation among some of the population groups analyzed in this work. Some studies have reported that the immunological composition of human milk changes over lactation (87, 88). Although the most dramatic changes occur in the transition from colostrum to mature milk, this variable may be responsible, at least partly, for some of the differences observed in mature milk. In fact, postpartum time was the demographic characteristic that significantly covaried with a higher number of immune factors (Groα, MIP1β, and TNFα) in this work. Variation in human milk immune factors with lactational age might reflect fine-tuning of milk bioactive compounds according to the changing infant needs.
Dietary and nutritional differences among the compared populations might also account for, at least, a part of the variability observed among the human milk immune factors analyzed in this study. On the one hand, recruited women might suffer from food limitation and nutritional deficiencies/excesses that were not taken into consideration in this study. Therefore, it would be highly recommended to include a detailed nutritional assessment in further studies addressing human milk composition. On the other hand, certain foods or supplements, such as fish oil (17), black currant seed oil (80), or probiotic bacteria, more commonly used in certain population groups (79, 89–91), might modify the immunological composition of human milk. In our study, declaration of consumption of probiotic supplements and/or fermented foods by the participants significantly varied among locations, with the highest consumption rates (30%) in VHHD countries. However, since studies with different probiotic strains administered either during pregnancy and/or lactation led to different milk immune outcomes (91–93), no conclusion can be made regarding the impact of probiotic intake on the data of our study.
In summary, our study provides evidence that there is no one-size-fits-all immunological composition of milk produced by healthy women. Instead, there is substantial variation within and, particularly among, human subpopulations in this regard. Nonetheless, our data suggest the existence of a common “core” set of Ig, cytokines, chemokines, and growth factors that are present in mature milk produced by all women, independent of their origin. Other “variable” components may be differentially important to infant health due to location, culture, breastfeeding norms, etc. Additional studies are required to further elucidate relationships among specific host, geographical, environmental, lifestyle, and health variables and the immune composition of colostrum, transient milk, and mature human milk.
As a global conclusion, human milk is a complex and dynamic fluid that provides nutrients, antigens, passive immunity, GI growth factors, and bioactive compounds that can actively shape and educate the infant immune system. The immunological potential of milk differs from one mother to another and likely depends on a mother’s exposure to antigens, her immune responses to them, and the dose in milk of the wide array of cells and compounds with immunological activities. A better understanding of how the levels of these compounds in milk are controlled and the identification of the key promoters of anti-infectious and tolerance-induction properties in neonates should help in the establishment of new strategies to prevent infant diseases (45, 46). This clearly represents a major challenge in the frontiers of immunology.
This study was carried out in accordance with the recommendations of the Washington State University Institutional Review Board guidelines. Ethics approvals were obtained for all procedures from each participating institution, with overarching approval from the Washington State University Institutional Review Board (#13264). After being translated from English (when needed), informed, verbal, or written consent (depending on locale and the subject’s literacy level) was acquired from each participating subject.
LR, IE-M, CG-C, SM, MKM, CM, MAM, JW, EK-M, EK, SM, LK, GO, KL, and KF conducted the research; MKM, CM, MAM, JF, DS, SEM, LK, GO, JR, RP, and LB designed the research; LR, IE-M, CG-C, and JR wrote the manuscript; LR, IE-M, and JR had primary responsibility for the final content of the manuscript; LR, IE-M, and CG-C analyzed the data; and all authors read and approved the final manuscript. None of the authors reported a conflict of interest related to the study.
The authors declare that the research was conducted in the absence of any commercial or financial relationships that could be construed as a potential conflict of interest.
We sincerely thank Andrew Doel (Medical Research Council Unit, The Gambia) for logistics planning and field supervision and Alansan Sey for questionnaire administration and taking anthropometric measurements in The Gambia; Jane Odei (University of Ghana) for supervising data collection in Ghana; Debela Gindola (Hawassa University), Dubale Gebeyehu (Hawassa University), Haile Belachew (Hawassa University), and Birhanu Sintayehu for planning, logistics, recruiting, and data collection and the administration and staff at Adare Hospital in Hawassa for assistance with logistics in Ethiopia; Catherine O Sarange (Egerton University) for field supervision and logistics planning and Milka W. Churuge and Minne M. Gachau for recruiting, questionnaire administration, and taking anthropometric measurements in Kenya; Gisella Barbagelatta (Instituto de Investigación Nutricional) for field supervision and logistics planning, Patricia Calderon (Instituto de Investigación Nutricional) for recruiting, questionnaire administration, and taking anthropometric measurements, and Roxana Barrutia (Instituto de Investigación Nutricional) for the management and shipping of samples in Peru; Leónides Fernández and Esther Jiménez (Complutense University of Madrid) for technical assistance, expertise, and review of the manuscript and M. Ángeles Checa (Zaragoza, Spain), Katalina Legarra (Guernica, Spain), and Julia Mínguez (Huesca, Spain) for participation in the collection of samples in Spain; Kirsti Kaski and Maije Sjöstrand (both Helsingborg Hospital) for participation in the collection of samples, questionnaire administration, and anthropometric measurements in Sweden; Renee Bridge and Kara Sunderland (both from University of California, San Diego, CA, USA) and Janae Carrothers (Washington State University) for logistics planning, recruiting, questionnaire administration, sample collection, and taking anthropometric measurements in California and Washington; and Glenn Miller (Washington State University) for his expertise and critical logistic help that were needed for shipping samples and supplies worldwide.
This project was primarily funded by the National Science Foundation (award #1344288); in addition, sterile, single-use milk collection kits were kindly provided by Medela, Inc. (McHenry, IL, USA). LR (Spain) was funded by grant 624773 (FP-7-PEOPLE-2013-IEF, European Commission) and by project AGL2013-4190-P (Ministry of Economy and Competitiveness, Spain).
The Supplementary Material for this article can be found online at http://journal.frontiersin.org/article/10.3389/fimmu.2017.00696/full#supplementary-material.
BMI, body mass index; ETU, urban Ethiopia; GBR, rural Gambia; GBU, urban Gambia; GCSF, granulocyte colony-stimulating factor; GMCSF, granulocyte–macrophage colony-stimulating factor; GI, gastrointestinal; GN, Ghana; GLM, general linear model; Groα, growth-related oncogene-α; HDI, human development index; HHD, high human development; IPAb, intrapartum antibiotherapy; IFNγ, interferon-γ; Ig, immunoglobulin; IL, interleukin; KE, Kenya; LHD, low human development; MALT, mucosa-associated lymphoid tissue; MCP1, macrophage–monocyte chemoattractant protein-1; MHD, medium human development; MIP1β, macrophage inflammatory protein-1β; PCA, principal component analysis; PE, Peru; sIg, secretory immunoglobulin; SP, Spain; SW, Sweden; TGFβ2, transforming growth factor-β2; TNFα, tumor necrosis factor-α; USW, USA/Washington; USC, USA/California (Hispanic); VHHD, very-high human development.
1. Gartner LM, Morton J, Lawrence RA, Naylor AJ, O’Hare D, Schanler RJ, et al. American Academy of Pediatrics Section on Breastfeeding. Breastfeeding and the use of human milk. Pediatrics (2005) 115:496–506. doi:10.1542/peds.2004-2491
2. U.S. Department of Health and Human Services. The Surgeon General’s Call to Action to Support Breastfeeding. (2011). Available from: http://www.ncbi.nlm.nih.gov/books/NBK52682/
3. Newburg DS, Walker WA. Protection of the neonate by the innate immune system of developing gut and of human milk. Pediatr Res (2007) 61(1):2–8. doi:10.1203/01.pdr.0000250274.68571.18
4. Gregory KE, Walker WA. Immunologic factors in human milk and disease prevention in the preterm infant. Curr Pediatr Rep (2013) 1(4):1–11. doi:10.1007/s40124-013-0028-2
5. Ismail IH, Licciardi PV, Oppedisano F, Boyle RJ, Tang ML. Relationship between breast milk sCD14, TGF-B1 and total IgA in the first month and development of eczema during infancy. Pediatr Allergy Immunol (2013) 24(4):352–60. doi:10.1111/pai.12075
6. Bode L. Human milk oligosaccharides: every baby needs a sugar mama. Glycobiology (2012) 22(9):1147–62. doi:10.1093/glycob/cws074
7. Fernández L, Langa S, Martín V, Maldonado A, Jiménez E, Martín R, et al. The human milk microbiota: origin and potential roles in health and disease. Pharmacol Res (2013) 69(1):1–10. doi:10.1016/j.phrs.2012.09.001
8. Kainonen E, Rautava S, Isolauri E. Immunological programming by breast milk creates an anti-inflammatory cytokine milieu in breast-fed infants compared to formula-fed infants. Br J Nutr (2013) 109(11):1962–70. doi:10.1017/S0007114512004229
9. Bourges D, Meurens F, Berri M, Chevaleyre C, Zanello G, Levast B, et al. New insights into the dual recruitment of IgA+ B cells in the developing mammary gland. Mol Immunol (2008) 45(12):3354–62. doi:10.1016/j.molimm.2008.04.017
10. Dill R, Walker AM. Role of prolactin in promotion of immune cell migration into the mammary gland. J Mammary Gland Biol Neoplasia (2017) 22(1):13–26. doi:10.1007/s10911-016-9369-0
11. Brandtzaeg P. Mucosal immunity: integration between mother and the breast-fed infant. Vaccine (2003) 21(24):3382–8. doi:10.1016/S0264-410X(03)00338-4
12. Ip S, Chung M, Raman G, Trikalinos TA, Lau J. A summary of the agency for healthcare research and quality’s evidence report on breastfeeding in developed countries. Breastfeed Med (2009) 4(Suppl 1):S17–30. doi:10.1089/bfm.2009.0050
13. Lodge CJ, Tan DJ, Lau MX, Dai X, Tham R, Lowe AJ, et al. Breastfeeding and asthma and allergies: a systematic review and meta-analysis. Acta Paediatr (2015) 104(467):38–53. doi:10.1111/apa.13132
14. Binns C, Lee M, Low WY. The long-term public health benefits of breastfeeding. Asia Pac J Public Health (2016) 28(1):7–14. doi:10.1177/1010539515624964
15. Mantis NJ, Rol N, Corthésy B. Secretory IgA’s complex roles in immunity and mucosal homeostasis in the gut. Mucosal Immunol (2011) 4(6):603–11. doi:10.1038/mi.2011.41
16. Dvorak B. Milk epidermal growth factor and gut protection. J Pediatr (2010) 156(2 Suppl):S31–5. doi:10.1016/j.jpeds.2009.11.018
17. Dunstan JA, Roper J, Mitoulas L, Hartmann PE, Simmer K, Prescott SL. The effect of supplementation with fish oil during pregnancy on breast milk immunoglobulin A, soluble CD14, cytokine levels and fatty acid composition. Clin Exp Allergy (2004) 34(8):1237–42. doi:10.1111/j.1365-2222.2004.02028.x
18. Kondo N, Suda Y, Nakao A, Oh-Oka K, Suzuki K, Ishimaru K, et al. Maternal psychosocial factors determining the concentrations of transforming growth factor-beta in breast milk. Pediatr Allergy Immunol (2011) 22(8):853–61. doi:10.1111/j.1399-3038.2011.01194.x
19. Groer M, Davis M, Steele K. Associations between human milk SIgA and maternal immune, infectious, endocrine, and stress variables. J Hum Lact (2004) 20(2):153–8. doi:10.1177/0890334404264104
20. Ciardelli L, Garofoli F, Avanzini MA, De Silvestri A, Gasparoni A, Sabatino G, et al. Escherichia coli specific secretory IgA and cytokines in human milk from mothers of different ethnic groups resident in northern Italy. Int J Immunopathol Pharmacol (2007) 20(2):335–40. doi:10.1177/039463200702000213
21. Holmlund U, Amoudruz P, Johansson MA, Haileselassie Y, Ongoiba A, Kayentao K, et al. Maternal country of origin, breast milk characteristics and potential influences on immunity in offspring. Clin Exp Immunol (2010) 162(3):500–9. doi:10.1111/j.1365-2249.2010.04275.x
22. Tomicić S, Johansson G, Voor T, Björkstén B, Böttcher MF, Jenmalm MC. Breast milk cytokine and IgA composition differ in Estonian and Swedish mothers-relationship to microbial pressure and infant allergy. Pediatr Res (2010) 68(4):330–4. doi:10.1203/00006450-201011001-00646
23. Urwin HJ, Zhang J, Gao Y, Wang C, Li L, Song P, et al. Immune factors and fatty acid composition in human milk from river/lake, coastal and inland regions of China. Br J Nutr (2013) 109(11):1949–61. doi:10.1017/S0007114512004084
24. Olivares M, Albrecht S, De Palma G, Ferrer MD, Castillejo G, Schols HA, et al. Human milk composition differs in healthy mothers and mothers with celiac disease. Eur J Nutr (2015) 54(1):119–28. doi:10.1007/s00394-014-0692-1
25. Munblit D, Treneva M, Peroni DG, Colicino S, Chow LY, Dissanayeke S, et al. Colostrum and mature human milk of women from London, Moscow and Verona: determinants of immune composition. Nutrients (2016) 8:695. doi:10.3390/nu8110695
26. Trend S, Strunk T, Lloyd ML, Kok CH, Metcalfe J, Geddes DT, et al. Levels of innate immune factors in preterm and term mother’s breast milk during the 1st month postpartum. Br J Nutr (2016) 115(7):1178–93. doi:10.1017/S0007114516000234
27. McGuire MK, Meehan CL, McGuire MA, Williams JE, Foster J, Sellen DW, et al. What’s normal? Oligosaccharide concentrations and profiles in milk produced by healthy women vary geographically. Am J Clin Nutr (2017) 105(5):1086–100. doi:10.3945/ajcn.116.139980
28. United Nations Development Program. Work for Human Development. New York: Human Development Report (2015).
29. Espinosa-Martos I, Jiménez E, de Andrés J, Rodríguez-Alcalá LM, Tavárez S, Manzano S, et al. Milk and blood biomarkers associated to the clinical efficacy of a probiotic for the treatment of infectious mastitis. Benef Microbes (2016) 7(3):305–18. doi:10.3920/BM2015.0134
30. Tsurumi A, Que YA, Ryan CM, Tompkins RG, Rahme LG. TNF-α/IL-10 ratio correlates with burn severity and may serve as a risk predictor of increased susceptibility to infections. Front Public Health (2016) 4:216. doi:10.3389/fpubh.2016.00216
31. Labbok MH, Clark D, Goldman AS. Breastfeeding: maintaining an irreplaceable immunological resource. Nat Rev Immunol (2004) 4:565–72. doi:10.1038/nri1393
32. Ballard O, Morrow AL. Human milk composition: nutrients and bioactive factors. Pediatr Clin North Am (2013) 60(1):49–74. doi:10.1016/j.pcl.2012.10.002
33. Goldman AS. The immune system of human milk: antimicrobial, anti-inflammatory and immunomodulating properties. Pediatr Infect Dis J (1993) 12:664–71. doi:10.1007/978-1-4614-2251-8_9
34. Lawrence RM, Pane CA. Human breast milk: current concepts of immunology and infectious diseases. Curr Probl Pediatr Adolesc Health Care (2007) 37(1):7–36. doi:10.1016/j.cppeds.2006.10.002
35. Brandtzaeg P. The mucosal immune system and its integration with the mammary glands. J Pediatr (2010) 156(2 Suppl):S8–15. doi:10.1016/j.jpeds.2009.11.014
36. Srivastava MD, Srivastava A, Brouhard B, Saneto R, Groh-Wargo S, Kubit J. Cytokines in human milk. Res Commun Mol Pathol Pharmacol (1996) 93(3):263–87. doi:10.1016/j.jpeds.2009.11.019
37. Kverka M, Burianova J, Lodinova-Zadnikova R, Kocourkova I, Cinova J, Tuckova L, et al. Cytokine profiling in human colostrum and milk by protein array. Clin Chem (2007) 53:955–62. doi:10.1373/clinchem.2006.077107
38. Rogier EW, Frantz AL, Bruno ME, Wedlund L, Cohen DA, Stromberg AJ, et al. Secretory antibodies in breast milk promote long-term intestinal homeostasis by regulating the gut microbiota and host gene expression. Proc Natl Acad Sci U S A (2014) 111(8):3074–9. doi:10.1073/pnas.1315792111
39. Brandtzaeg P, Johansen F-E. IgA and intestinal homeostasis. In: Kaetzel CS, editor. Mucosal Immune Defense: Immunoglobulin A. New York: Springer Science + Business Media LLC (2007). p. 221–68.
40. Järvinen KM, Laine ST, Järvenpää AL, Suomalainen HK. Does low IgA in human milk predispose the infant to development of cow’s milk allergy? Pediatr Res (2000) 48:457–62. doi:10.1203/00006450-200010000-00007
41. Savilahti E, Siltanen M, Kajosaari M, Vaarala O, Saarinen KM. IgA antibodies, TGF-beta1 and -beta2, and soluble CD14 in the colostrum and development of atopy by age 4. Pediatr Res (2005) 58:1300–5. doi:10.1203/01.pdr.0000183784.87452.c6
42. Garofalo RP, Goldman AS. Cytokines, chemokines, and colony-stimulating factors in human milk: the 1997 update. Biol Neonate (1998) 74(2):134–42. doi:10.1159/000014019
43. Garofalo RP. Cytokines in human milk. J Pediatr (2010) 156:S36–40. doi:10.1016/j.jpeds.2009.11.019
44. Jakaitis BM, Denning PW. Human breast milk and the gastrointestinal innate immune system. Clin Perinatol (2014) 41(2):423–35. doi:10.1016/j.clp.2014.02.011
45. Verhasselt V. Neonatal tolerance under breastfeeding influence: the presence of allergen and transforming growth factor-β in breast milk protects the progeny from allergic asthma. J Pediatr (2010) 156:S16–20. doi:10.1016/j.jpeds.2009.11.015
46. Verhassel V. Neonatal tolerance under breastfeeding influence. Curr Opin Immunol (2010) 22:623–30. doi:10.1016/j.coi.2010.08.008
47. Smith KM, Eaton AD, Finlayson LM, Garside P. Oral tolerance. Am J Respir Crit Care Med (2000) 162:S175–8. doi:10.1164/ajrccm.162.supplement_3.15tac7
48. Veldhoen M, Hocking RJ, Atkins CJ, Locksley RM, Stockinger B. TGFbeta in the context of an inflammatory cytokine milieu supports de novo differentiation of IL-17-producing T cells. Immunity (2006) 24:179–89. doi:10.1016/j.immuni.2006.01.001
49. Penttila IA, van Spriel AB, Zhang MF, Xian CJ, Steeb CB, Cummins AG, et al. Transforming growth factor-beta levels in maternal milk and expression in postnatal rat duodenum and ileum. Pediatr Res (1998) 44:524–31. doi:10.1203/00006450-199810000-00010
50. Faria AM, Weiner HL. Oral tolerance and TGF-beta-producing cells. Inflamm Allergy Drug Targets (2006) 5:179–90. doi:10.2174/187152806778256034
51. Penttila IA. Milk-derived transforming growth factor-β and the infant immune response. J Pediatr (2010) 156:S21–5. doi:10.1016/j.jpeds.2009.11.016
52. Kalliomaki M, Ouwehand A, Arvilommi H, Kero P, Isolauri E. Transforming growth factor-beta in breast milk: a potential regulator of atopic disease at an early age. J Allergy Clin Immunol (1999) 104:1251–7. doi:10.1016/S0091-6749(99)70021-7
53. Oddy WH, Halonen M, Martinez FD, Lohman IC, Stern DA, Kurzius-Spencer M, et al. TGF-beta in human milk is associated with wheeze in infancy. J Allergy Clin Immunol (2003) 112:723–8. doi:10.1016/S0091
54. Penttila I. Effects of transforming growth factor-beta and formula feeding on systemic immune responses to dietary β-lactoglobulin in allergy-prone rats. Pediatr Res (2006) 59:650–5. doi:10.1203/01.pdr.0000203149.75465.74
55. Penttila IA, Flesch IE, McCue AL, Powell BC, Zhou FH, Read LC, et al. Maternal milk regulation of cell infiltration and interleukin 18 in the intestine of suckling rat pups. Gut (2003) 52:1579–86. doi:10.1136/gut.52.11.1579
56. Rautava S, Nanthakumar NN, Dubert-Ferrandon A, Lu L, Rautava J, Walker WA. Breast milk-transforming growth factor-β specifically attenuates IL-1β-induced inflammatory responses in the immature human intestine via an SMAD6- and ERK-dependent mechanism. Neonatology (2011) 99(3):192–201. doi:10.1159/000314109
57. Nguyen DN, Sangild PT, Ostergaard MV, Bering SB, Chatterton DE. Transforming growth factor-β2 and endotoxin interact to regulate homeostasis via interleukin-8 levels in the immature intestine. Am J Physiol Gastrointest Liver Physiol (2014) 307(7):G689–99. doi:10.1152/ajpgi.00193.2014
58. Yung SC, Murphy PM. Antimicrobial chemokines. Front Immunol (2012) 3:276. doi:10.3389/fimmu.2012.00276
59. Tsai HH, Frost E, To V, Robinson S, Ffrench-Constant C, Geertman R, et al. The chemokine receptor CXCR2 controls positioning of oligodendrocyte precursors in developing spinal cord by arresting their migration. Cell (2002) 110(3):373–83. doi:10.1016/S0092-8674(02)00838-3
60. Omari KM, Lutz SE, Santambrogio L, Lira SA, Raine CS. Neuroprotection and remyelination after autoimmune demyelination in mice that inducibly overexpress CXCL1. Am J Pathol (2009) 174(1):164–76. doi:10.2353/ajpath.2009.080350
61. Devalaraja RM, Nanney LB, Du J, Qian Q, Yu Y, Devalaraja MN, et al. Delayed wound healing in CXCR2 knockout mice. J Invest Dermatol (2000) 115(2):234–44. doi:10.1046/j.1523-1747.2000.00034.x
62. Vries MH, Wagenaar A, Verbruggen SE, Molin DG, Dijkgraaf I, Hackeng TH, et al. CXCL1 promotes arteriogenesis through enhanced monocyte recruitment into the peri-collateral space. Angiogenesis (2015) 18(2):163–71. doi:10.1007/s10456-014-9454-1
63. Böttcher MF, Jenmalm MC, Björkstén B, Garofalo RP. Chemoattractant factors in breast milk from allergic and nonallergic mothers. Pediatr Res (2000) 47(5):592–7. doi:10.1203/00006450-200005000-00006
64. Ebert EC. Human intestinal intraepithelial lymphocytes have potent chemotactic activity. Gastroenterology (1995) 109:1154–9. doi:10.1016/0016-5085(95)90573-1
65. Polat A, Tunc T, Erdem G, Yerebasmaz N, Tas A, Beken S, et al. Interleukin-8 and its receptors in human milk from mothers of full-term and premature infants. Breastfeed Med (2016) 11(5):247–51. doi:10.1089/bfm.2015.0186
66. Jones MK, Tomikawa M, Mohajer B, Tarnawski AS. Gastrointestinal mucosal regeneration: role of growth factors. Front Biosci (1999) 4:D303–9. doi:10.2741/A428
67. Warner BW, Warner BB. Role of epidermal growth factor in the pathogenesis of neonatal necrotizing enterocolitis. Semin Pediatr Surg (2005) 14(3):175–80. doi:10.1053/j.sempedsurg.2005.05.006
68. Connolly JM, Rose DP. Epidermal growth factor-like proteins in breast fluid and human milk. Life Sci (1988) 42(18):1751–6. doi:10.1016/0024-3205(88)90041-0
69. Moran JR, Courtney ME, Orth DN, Vaughan R, Coy S, Mount CD, et al. Epidermal growth factor in human milk: daily production and diurnal variation during early lactation in mothers delivering at term and at premature gestation. J Pediatr (1983) 103(3):402–5. doi:10.1016/S0022-3476(83)80412-0
70. Sullivan PB, Brueton MJ, Tabara ZB, Goodlad RA, Lee CY, Wright NA. Epidermal growth factor in necrotising enteritis. Lancet (1991) 338(8758):53–4. doi:10.1016/0140-6736(91)90042-N
71. Clark JA, Lane RH, Maclennan NK, Holubec H, Dvorakova K, Halpern MD, et al. Epidermal growth factor reduces intestinal apoptosis in an experimental model of necrotizing enterocolitis. Am J Physiol Gastrointest Liver Physiol (2005) 288(4):G755–62. doi:10.1152/ajpgi.00172.2004
72. Clark JA, Doelle SM, Halpern MD, Saunders TA, Holubec H, Dvorak K, et al. Intestinal barrier failure during experimental necrotizing enterocolitis: protective effect of EGF treatment. Am J Physiol Gastrointest Liver Physiol (2006) 291(5):G938–49. doi:10.1152/ajpgi.00090.2006
73. Hasselbalch H, Engelmann MD, Ersboll AK, Jeppesen DL, Fleischer-Michaelsen K. Breast-feeding influences thymic size in late infancy. Eur J Pediatr (1996) 158:964–7. doi:10.1007/s004310051258
74. Ngom PT, Collinson AC, Pido-Lopez J, Henson SM, Prentice AM, Aspinall R. Improved thymic function in exclusively breastfed infants is associated with higher interleukin 7 concentrations in their mothers’ breast milk. Am J Clin Nutr (2004) 80:722–8.
75. Ustundag B, Yilmaz E, Dogan Y, Akarsu S, Canatan H, Haelifeoglu I, et al. Levels of cytokines (IL-1β, IL-2, IL-6, IL-8, TNF-α) and trace elements (Zn, Cu) in breast milk from mothers of preterm and term infants. Mediators Inflamm (2005) 2005(6):331–6. doi:10.1155/MI.2005.331
76. Bloomfield S, Stanwell-Smith R, Crevel R, Pickup J. Too clean, or not too clean: the hygiene hypothesis and home hygiene. Clin Exp Allergy (2006) 36(4):402–25. doi:10.1111/j.1365-2222.2006.02463.x
77. Andersen CJ, Murphy KE, Fernandez ML. Impact of obesity and metabolic syndrome on immunity. Adv Nutr (2016) 7(1):66–75. doi:10.3945/an.115.010207
78. Quach H, Maxime R, Pothlichet J, Loh Y-HE, Dannemann M, Zidane N, et al. Genetic adaptation and neandertal admixture shaped the immune system of human populations. Cell (2016) 167(3):643–56. doi:10.1016/j.cell.2016.09.024
79. Hoppu U, Isolauri E, Laakso P, Matomäki J, Laitinen K. Probiotics and dietary counselling targeting maternal dietary fat intake modifies breast milk fatty acids and cytokines. Eur J Nutr (2012) 51(2):211–9. doi:10.1007/s00394-011-0209-0
80. Linnamaa P, Nieminen K, Koulu L, Tuomasjukka S, Kallio H, Yang B, et al. Black currant seed oil supplementation of mothers enhances IFN-γ and suppresses IL-4 production in breast milk. Pediatr Allergy Immunol (2013) 24(6):562–6. doi:10.1111/pai.12110
81. Groer M, Ashmeade T, Duffy A, Morse S, Zaritt J. Changes in the immune components of preterm human milk and associations with maternal and infant characteristics. J Obstet Gynecol Neonatal Nurs (2016) 45(5):639–48. doi:10.1016/j.jogn.2016.04.009
82. Mizuno K, Hatsuno M, Aikawa K, Takeichi H, Himi T, Kaneko A, et al. Mastitis is associated with IL-6 levels and milk fat globule size in breast milk. J Hum Lact (2012) 28(4):529–34. doi:10.1177/0890334412455946
83. Tuaillon E, Viljoen J, Dujols P, Cambonie G, Rubbo PA, Nagot N, et al. Subclinical mastitis occurs frequently in association with dramatic changes in inflammatory/anti-inflammatory breast milk components. Pediatr Res (2016) 81(4):556–64. doi:10.1038/pr.2016.220
84. Bryan DL, Hart PH, Forsyth KD, Gibson RA. Immunomodulatory constituents of human milk change in response to infant bronchiolitis. Pediatr Allergy Immunol (2007) 18(6):495–502. doi:10.1111/j.1399-3038.2007.00565.x
85. Riskin A, Almog M, Peri R, Halasz K, Srugo I, Kessel A. Changes in immunomodulatory constituents of human milk in response to active infection in the nursing infant. Pediatr Res (2012) 71(2):220–5. doi:10.1038/pr.2011.34
86. Hassiotou F, Hepworth AR, Metzger P, Tat Lai C, Trengove N, Hartmann PE, et al. Maternal and infant infections stimulate a rapid leukocyte response in breastmilk. Clin Transl Immunol (2013) 2(4):e3. doi:10.1038/cti.2013.1
87. Chollet-Hinton LS, Stuebe AM, Casbas-Hernandez P, Chetwynd E, Troester MA. Temporal trends in the inflammatory cytokine profile of human breastmilk. Breastfeed Med (2014) 9(10):530–7. doi:10.1089/bfm.2014.0043
88. Collado MC, Santaella M, Mira-Pascual L, Martínez-Arias E, Khodayar-Pardo P, Ros G, et al. Longitudinal study of cytokine expression, lipid profile and neuronal growth factors in human breast milk from term and preterm deliveries. Nutrients (2015) 7(10):8577–91. doi:10.3390/nu7105415
89. Ortiz-Andrellucchi A, Sánchez-Villegas A, Rodríguez-Gallego C, Lemes A, Molero T, Soria A, et al. Immunomodulatory effects of the intake of fermented milk with Lactobacillus casei DN114001 in lactating mothers and their children. Br J Nutr (2008) 100(4):834–834. doi:10.1017/S0007114508959183
90. Nikniaz L, Ostadrahimi A, Mahdavi R, Hejazi MA, Salekdeh GH. Effects of synbiotic supplementation on breast milk levels of IgA, TGFβ1 and TGFβ2. J Hum Lact (2013) 29(4):591–6. doi:10.1177/0890334413490833
91. Prescott SL, Wickens K, Westcott L, Jung W, Currie H, Black PN, et al. Supplementation with Lactobacillus rhamnosus or Bifidobacterium lactis probiotics in pregnancy increases cord blood interferon-γ and breast milk transforming growth factor-β and immunoglobulin A detection. Clin Exp Allergy (2008) 38(10):1606–14. doi:10.1111/j.1365-2222.2008.03061.x
92. Baldassarre ME, Di Mauro A, Mastromarino P, Fanelli M, Martinelli D, Urbano F, et al. Administration of a multi-strain probiotic product to women in the perinatal period differentially affects the breast milk cytokine profile and may have beneficial effects on neonatal gastrointestinal functional symptoms. A randomized clinical trial. Nutrients (2016) 8(11):E677. doi:10.3390/nu8110677
Keywords: breastfeeding, human milk, lactation, immunoglobulins, cytokines, chemokines, growth factors
Citation: Ruiz L, Espinosa-Martos I, García-Carral C, Manzano S, McGuire MK, Meehan CL, McGuire MA, Williams JE, Foster J, Sellen DW, Kamau-Mbuthia EW, Kamundia EW, Mbugua S, Moore SE, Kvist LJ, Otoo GE, Lackey KA, Flores K, Pareja RG, Bode L and Rodríguez JM (2017) What’s Normal? Immune Profiling of Human Milk from Healthy Women Living in Different Geographical and Socioeconomic Settings. Front. Immunol. 8:696. doi: 10.3389/fimmu.2017.00696
Received: 31 March 2017; Accepted: 29 May 2017;
Published: 30 June 2017
Edited by:
Caroline Elizabeth Childs, University of Southampton, United KingdomReviewed by:
Alberto Finamore, Council for Agricultural Research and Economics-Food and Nutrition Research Center, ItalyCopyright: © 2017 Ruiz, Espinosa-Martos, García-Carral, Manzano, McGuire, Meehan, McGuire, Williams, Foster, Sellen, Kamau-Mbuthia, Kamundia, Mbugua, Moore, Kvist, Otoo, Lackey, Flores, Pareja, Bode and Rodríguez. This is an open-access article distributed under the terms of the Creative Commons Attribution License (CC BY). The use, distribution or reproduction in other forums is permitted, provided the original author(s) or licensor are credited and that the original publication in this journal is cited, in accordance with accepted academic practice. No use, distribution or reproduction is permitted which does not comply with these terms.
*Correspondence: Lorena Ruiz, bG9yZW5hLnJ1aXpAaXBsYS5jc2ljLmVz;
Irene Espinosa-Martos, aXJlbmVlNzBAZ21haWwuY29t;
Juan M. Rodríguez, am1yb2RyaWdAdmV0LnVjbS5lcw==
†These authors have contributed equally to this work.
Disclaimer: All claims expressed in this article are solely those of the authors and do not necessarily represent those of their affiliated organizations, or those of the publisher, the editors and the reviewers. Any product that may be evaluated in this article or claim that may be made by its manufacturer is not guaranteed or endorsed by the publisher.
Research integrity at Frontiers
Learn more about the work of our research integrity team to safeguard the quality of each article we publish.