- 1Department of Pediatric Hematology and Oncology, IRCCS Bambino Gesù Children’s Hospital, Rome, Italy
- 2Department of “Medicina Clinica e Chirurgia”, University of Naples Federico II, Naples, Italy
- 3Department of Pediatrics, University of Pavia, Pavia, Italy
Hematopoietic stem cell transplantation is standard therapy for numerous hematological diseases. The use of haploidentical donors, sharing half of the HLA alleles with the recipient, has facilitated the use of this procedure as patients can rely on availability of a haploidentical donor within their family. Since HLA disparity increases the risk of graft-versus-host disease, T-cell depletion has been used to remove alloreactive lymphocytes from the graft. Selective removal of αβ T cells, which encompass the alloreactive repertoire, combined with removal of B cells to prevent EBV-related lymphoproliferative disease, proved safe and effective in clinical studies. Depleted αβ T cells and B cells are generally discarded as by-products. Considering the possible use of donor T cells for donor lymphocyte infusions or for generation of pathogen-specific T cells as mediators of graft-versus-infection effect, we tested whether cells in the discarded fractions were functionally intact. Response to alloantigens and to viral antigens comparable to that of unmanipulated cells indicated a functional integrity of αβ T cells, in spite of the manipulation used for their depletion. Furthermore, B cells proved to be efficient antigen-presenting cells, indicating that antigen uptake, processing, and presentation were fully preserved. Therefore, we propose that separated αβ T lymphocytes could be employed for obtaining pathogen-specific T cells, applying available methods for positive selection, which eventually leads to indirect allodepletion. In addition, these functional T cells could undergo additional manipulation, such as direct allodepletion or genetic modification.
Introduction
HLA-haploidentical hematopoietic stem cell transplantation (haplo-HSCT) from a related donor is a suitable option for patients affected by many malignant and non-malignant hematological diseases (1–3). In fact, patients have a high chance of having a haploidentical related donor within their family group, and pediatric patients, in particular, can rely on both parents as potential donors. Nevertheless, due to the incompatible haplotype, the risk of graft-versus-host disease (GvHD) is high in haplo-HSCT (2). Removal of T cells encompassing the alloreactive precursors has been applied to prevent GvHD (4, 5), as recently reviewed (6). This procedure is effective in preventing GvHD, but a concomitant increase of graft rejection (7, 8), loss of T-cell mediated graft-versus-leukemia (GvL) effect, with higher incidence of relapse of the primary malignant disease (9–12), and loss of graft-versus-infection (GvI) effect, with a higher risk of severe opportunistic infections due to delayed reconstitution of pathogen-specific T-cell immunity (13–15), have been also observed. In comparison to a bone marrow-derived graft, the introduction of G-CSF-mobilized HSC (16) made the apheretic product a more convenient cell source for haplo-HSCT. Recently, more selective procedures of graft manipulation have been introduced to remove alloreactive precursors within well-defined T-cell subsets, preserving other mononuclear cells that exert protective immune functions. Selective T-cell depletion can include (i) removal of αβ T cells, which encompass the alloreactive precursors, sparing γδ T cells, and natural killer (NK) cells, which play a protective role against opportunistic infection and regrowth of leukemia cells (17) and (ii) removal of the CD45RA naïve T-cell fraction containing alloreactive precursors, sparing the memory fraction containing T cells responsive to opportunistic pathogens (18–20). The first procedure, based on removal of αβ T cells with anti αβ TCR antibodies bound on paramagnetic microbeads, which are retained by a magnetic column, is now commercially available with certified reagents, protocols, and automated instrumentation (Miltenyi Biotec, Bergish Gladbach, Germany). This procedure includes concomitant removal of B cells with anti-CD19 antibodies with the purpose of reducing the risk of EBV-associated posttransplant lymphoproliferative disease. Clinical results demonstrating the safety and efficacy of this procedure have been recently reported (21–23).
The αβ T-cell and B-cell depleted product (graft) contains, in addition to CD34 HSC, other mononuclear cells such as NK cells, γδ T cells, and monocytes/dendritic cells (MoDC), which exert positive immune functions (21). The labeled αβ T cells and B cells retained by the magnetic column represent the non-target (NT) population. If the magnetic field is withdrawn from the paramagnetic column, the retained cells can be eluted and collected as the NT fraction, but they are generally disposed of.
We considered the NT fractions as an immunological asset worth analyzing for specific functions after the in vitro graft manipulation. NT cells, in fact, could be considered as an alternative source of T cells for unmanipulated donor lymphocyte infusion (DLI) to control/prevent infectious complications (GvI effect) or to prevent/treat relapse of the primary malignancy (GvL effect) (24–27). Additionally, NT cells can be a valuable starting material to obtain antigen-specific T cells able to accelerate immune reconstitution (28), by using direct selection procedures based on multimer technology as described on recent reports (29–31). These reports are of special relevance in this context as they demonstrate that low doses of selected T cells effectively provided a GvI effect and could expand in vivo to reconstitute a protective T-cell response. Furthermore, the same T cells can be considered for further advanced manipulation (32–35) for the introduction of suicide genes or for expression of novel engineered T-cell receptors.
In light of these considerations, in this work we tested the preservation of antigen-specific functions of αβ T cells and the antigen-presenting function of B cells present in the NT fraction after the depletion procedures.
Materials and Methods
Reagents and Media
The kit for αβ T-cell/B-cell depletion includes reagents and disposable bags with interconnecting tubing in addition to the in-line magnetic column (Miltenyi, Bergish Gladbach, Germany). The PBS-EDTA buffer (Miltenyi) was supplemented with human serum albumin (HSA, Grifols, Barcelona, Spain). Ficoll (Lymphoprep) was obtained from Sigma (St. Louis, MO, USA). RPMI 1640 with HEPES buffer, l-glutamine, and antibiotics were from Euroclone (Milan, Italy). PPD was purchased from Statens Seruminstitut (Copenhagen, Denmark). CMV, EBV, and adenovirus antigens were obtained from Microbix Biosystems (Mississauga, ON, Canada) as lysates of infected cells centrifuged to remove cell debris. The CMV pp65 peptide library (pepmix, 15mer peptides overlapping by 11 residues) was purchased from JPT (Berlin, Germany). Reagents for IFNγ ELISA were from Mabtech (Stockholm, Sweden) and 3H-thymidine (specific activity 0.25 TBq/mmole) was from Perkin Elmer (Boston, MA, USA). Monoclonal antibodies (Moab) for cell phenotyping were from Becton Dickinson (San Josè, CA, USA), and they were used in combinations previously described (36).
Collection of NT Cells
Donors of cells for haplo-HSCT underwent an apheretic session after HSC mobilization with G-CSF (22, 36). The cells in the apheresis (Aph) bag were processed to remove αβ T cells and B cells, as described in Ref. (37), following the detailed protocol provided by Miltenyi. Briefly, cells in the Aph bag were tagged with biotin-conjugated anti-αβ TCR Moab. After incubation and washing, the cells were labeled with paramagnetic beads conjugated to anti-biotin and anti-CD19 antibodies. After washing to remove unbound beads, the cell suspension was loaded on the CliniMACS device to automatically process cells through a magnetic column, which retains the labeled cells (αβ T cells and CD19 B cells). The effluent cells in the collection bag (CB) included CD34 HSC, hematopoietic precursors, γδ T cells, NK cells, MoDC, and represented the graft ready for infusion into the recipients. The average performance of 200 procedures, evaluated as depletion efficiency of unwanted cells and as recovery efficiency of desired cells (38), matched the data from other reports (22, 36, 37, 39). The retained population, consisting of αβ T cells and B cells, was designated as the NT fraction. This fraction could be eluted from the column upon withdrawal of the magnetic field. Samples from the different fractions obtained from 20 depletion procedures performed on 20 donors were saved for the experiments described here. HLA typing and serostatus of the donors is reported in Table 1. Positive serostatus was defined according to thresholds provided by the clinical laboratory.
Processing of NT Cells
Samples were obtained from the Aph bag, from the CB, and from the NT fraction. Cells were used for phenotypic characterization to evaluate procedure efficiency and for testing functional activity, as described here. Cells were kept in PBS–EDTA–HSA 0.5% buffer at 4°C for up to 24 h before processing. After washing with PBS, cells were loaded on conventional Ficoll gradients to obtain the mononuclear fraction. This step was not required for NT fractions, which already contained purified αβ T cells and B cells, but at any rate it was used for better parallel comparison with the other cell fractions. Ficoll passage and washing of the NT fractions removed the residual free beads that were eluted from the column along with labeled cells. Cells were resuspended at 2 × 106 cells/ml in RPMI 1640 medium supplemented with l-glutamine 2 mM, 2-mercapto-ethanol 5 × 10−5 M, penicillin 100 U/ml, and streptomycin 100 μg/ml. Heat-inactivated human AB serum (Sigma) was added at 5% final concentration to produce the complete medium. Average cell viability was >90%.
Functional Assays for Antigen-Specific Responses
Allospecific responses against T-cell depleted allogeneic PBMC from random donors as stimulator cells were tested in one-way mixed lymphocyte reactions (MLR). T-cell depletion was performed using anti-CD3 magnetic beads (Dynal, Thermo Fisher) following the manufacturer’s instructions. T-depleted stimulator cells from four subjects were pooled and frozen in aliquots as an allo-pool in order to maximize responder versus stimulator HLA disparity. When needed, cells were thawed, washed, and irradiated (30 Gy) before use. Responder cells were seeded at 100 μl/well (2 × 105 cells) with an equal number of stimulator cells in 100 μl in 96-well, flat bottom microtiter plates. Responses were tested as IFNγ secretion after 2-day incubation. ELISA assays were run on culture supernatants following manufacturer’s instructions. Results are given as picogram per milliliters IFNγ or as stimulation indexes (SI, picogram per milliliters with antigen: picogram per milliliters background). A positive alloresponse was defined as having an SI ≥ 10. Responses to soluble antigens were tested on 2 × 105 cells in 200 μl medium. Specific antigens included CMV, EBV, adenovirus lysates, CMV pp65 peptide mix, and PPD. Antigens were used at 5 μg/ml final concentration. After 5-day incubation, cultures were pulsed with 18 kBq 3H-thymidine for 18 h and harvested on glass fiber filters. Dry filters were counted on a Microbeta Trilux 1450 counter (Wallac, Perkin Elmer). Results are expressed as SI (cpm with antigen/cpm background). An SI ≥ 3 defined an antigen-specific positive response.
Statistical analyses were performed using the Graph Pad Prism 6.1 version software. Significant differences between sets of data were determined by the Mann–Whitney test. Correlation between paired data sets was determined using the Pearson’s coefficient. Highly significant differences were defined by p < 0.01.
Results
Depletion Efficiency and Enrichment of αβ T and B Cells
Figure 1 shows the composition of the manipulated cell fractions in 20 different procedures from which samples were obtained for this study. Most CD34 HSC, NK cells, and γδ T cells were recovered in the CB fractions. A remarkable depletion was achieved for αβ T cells and B cells, with mean values of around 12,000 × 106 and 3,000 × 106 in the Aph samples versus 1 × 106 and 0.8 × 106 in the CB fractions. These cell subsets were recovered in the NT fractions, with mean values of around 10,000 × 106 and 2,800 × 106, corresponding to a recovery efficiency of 84 and 93%, respectively. Since these figures obtained from 20 procedures overlap with the results obtained from over 200 procedures performed in our laboratory (38), the data reported below can be considered fully representative of the fractions obtained with the standard depletion procedure. In order to better visualize the αβ T- and B-cell depletion from the CB fractions and their recovery in the NT fractions, the graphs in Figure 1B express the results as relative percentages.
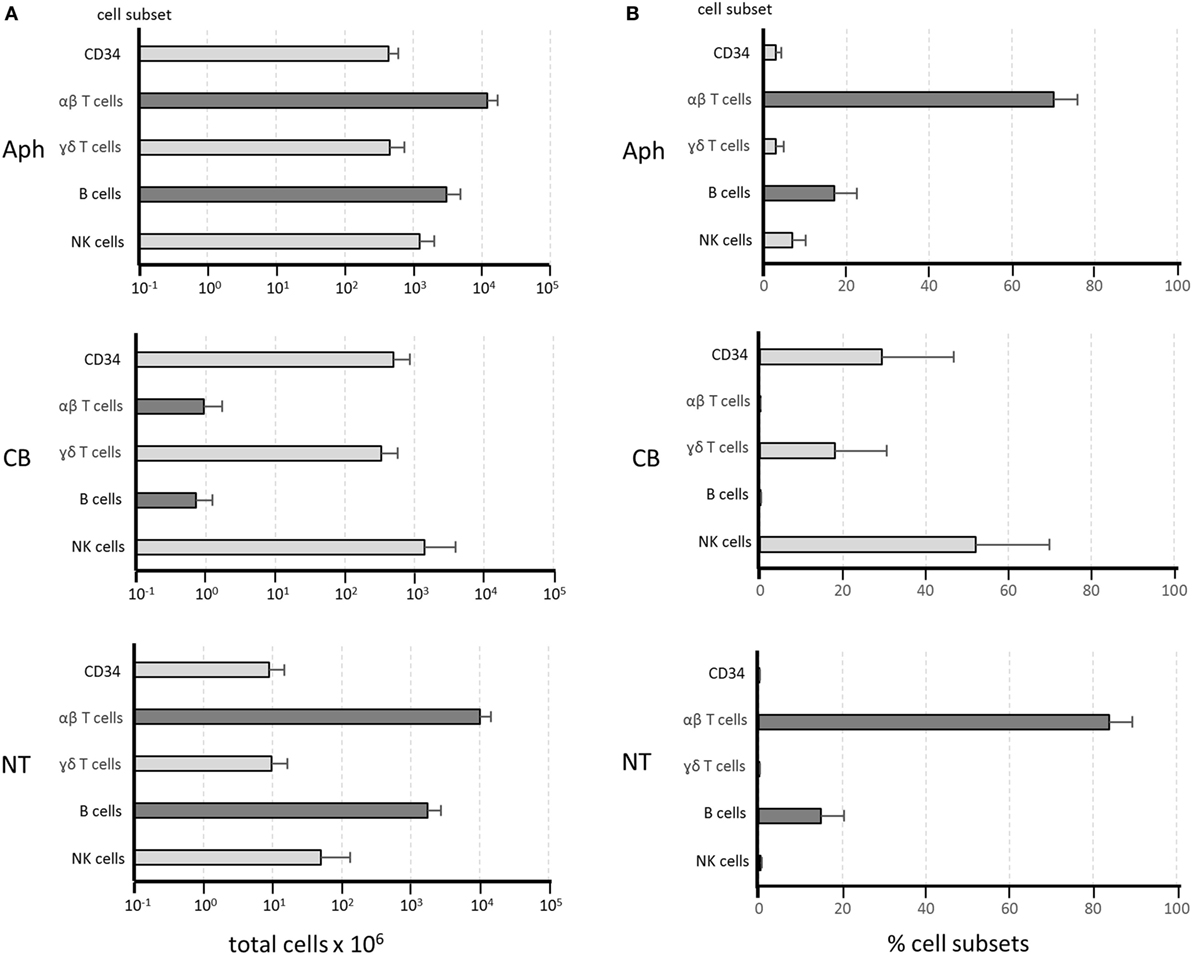
Figure 1. Composition of cellular fractions obtained from αβ T-cell/B-cell depletion procedures. Twenty depletion procedures were used for the reported experiments. Samples of the different cell fractions—apheresis (Aph), collection bag (CB), and non-target (NT) fraction—were evaluated for cellular composition. Dark gray bars indicate αβ T cells and B cells, while light gray bars refer to the other subsets. Results are shown as mean values ± SD of total cell numbers for each subset on a log scale in panel (A). Relative frequency of the same subsets is shown in panel (B) as percentages of the total NC identified by flow cytometry. The Mann–Whitney test was used to define non-significant differences in αβ T-cell and B-cell numbers (A) or frequencies (B) between Aph and NT samples, indicating full recovery of the depleted cells in the NT fractions. By contrast, highly significant depletion for αβ T cells and B cells can be observed in panels (A,B) by comparing the Aph and the CB samples.
Preservation of T-Cell Alloreactive Responses
Representative examples of MLR are shown in Figure 2. Alloreactive responses were tested in Aph samples and in NT samples. Upper panels show results as IFNγ concentration, while middle panels show results as SI. It should be noted that IFNγ concentrations have been normalized and referred to 105 αβ T cells for each donor to take into account the fact that different numbers of αβ T cells were present in the Aph and in the NT fractions. The two graphs on the left are examples of concordant responses, with high alloreactivity in both Aph and NT cells. The two graphs on the right provide examples of discordant responses. The bottom plot shows responses of Aph and of NT cells for each tested donor. Assuming a threshold of SI ≥ 10 for a positive alloresponse, 7/7 NT fractions were positive, while 3/7 Aph samples were positive. No opposite cases of NT negative and Aph positive alloresponses were observed. Overall correlation between alloresponses in NT and in Aph fractions was not statistically significant.
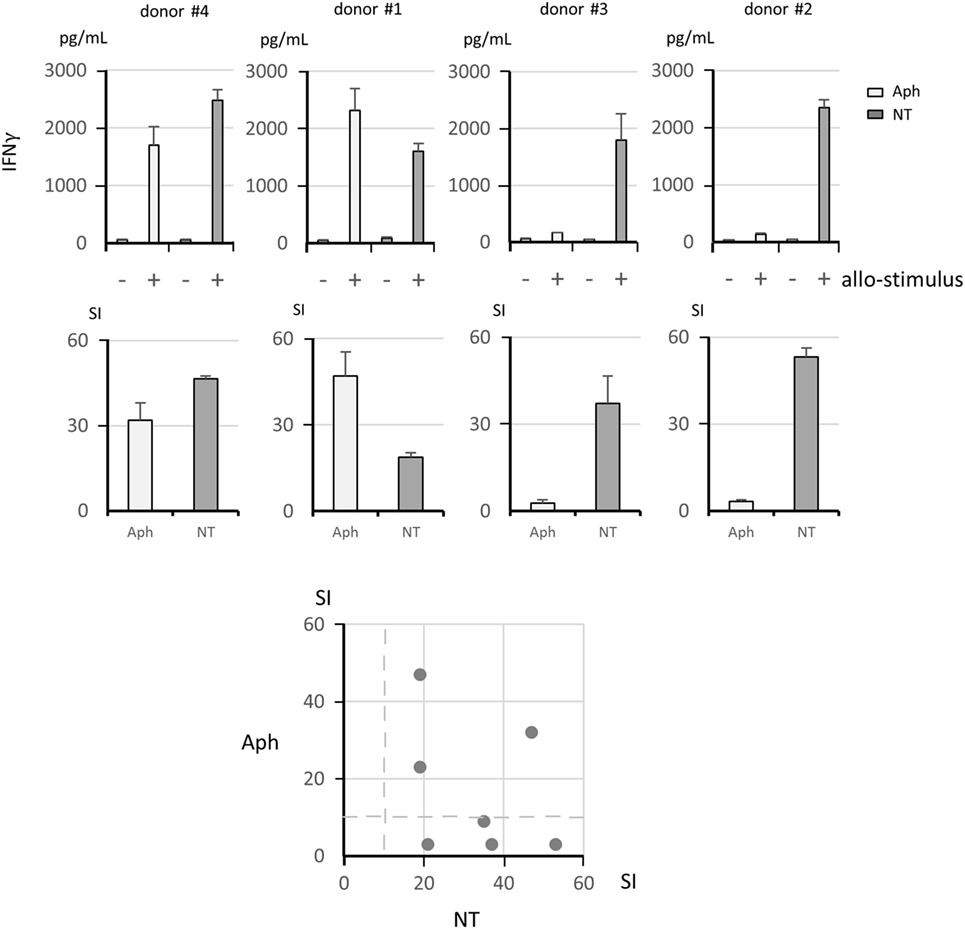
Figure 2. Analysis of alloreactive responses. The upper panels show IFNγ concentration as picogram per milliliters measured by ELISA on culture supernatants from mixed lymphocyte reaction assays (+, allogeneic stimulator cell pool; −, autologous control cells). The IFNγ concentrations have been normalized and referred to 105 αβ T cells for each donor, considering that these cells were present at different concentrations in the Aph and in the non-target (NT) fractions. The middle panels show the corresponding stimulation indexes (SI). Four representative donors are shown, with vigorous responses in both Aph and NT samples in the two left hand panels and vigorous responses in the NT fractions only in the two right hand panels. The bottom panel shows a summary plot of SI for Aph and NT samples for each donor, with no statistically significant correlation between the paired data (Pearson’s coefficient test). Dashed lines indicate SI = 10.
Maintenance of Specific T-Cell Response to Protein Antigens
Since we did not know whether B cells in the NT fractions had reduced APC function due to a possible interference with this function by anti-CD19 beads bound to the cell surface or because of extensive manipulation, we added irradiated cells from the CB fractions (CB*), which contain professional APC represented by MoDC, to ensure an adequate APC function. Four representative examples of antigen-specific responses expressed as SI are shown in Figure 3, upper panels. Lower panels (Figures 3A–E) illustrate the response for each antigen in the Aph fractions and in the NT (+CB*) fractions for all donors. A positive response to one or more antigens seen in NT fractions suggested that αβ T cells were not impaired in their specific capacity to recognize antigens. A summary of responses to the various antigens is shown in Figure 3F. A possible association between serostatus and T-cell response to viral antigens was considered by defining the frequency of concordant responses, either positive or negative. The seropositive status defined using the threshold provided the clinical laboratory and the positive T-cell response was defined by SI ≥ 3 in Aph and/or NT fractions. Concordance was 84% for CMV responses, 89% for EBV responses, and 47% for AdV responses. This suggests that T-cell responses should be defined independently of the donor’s serostatus.
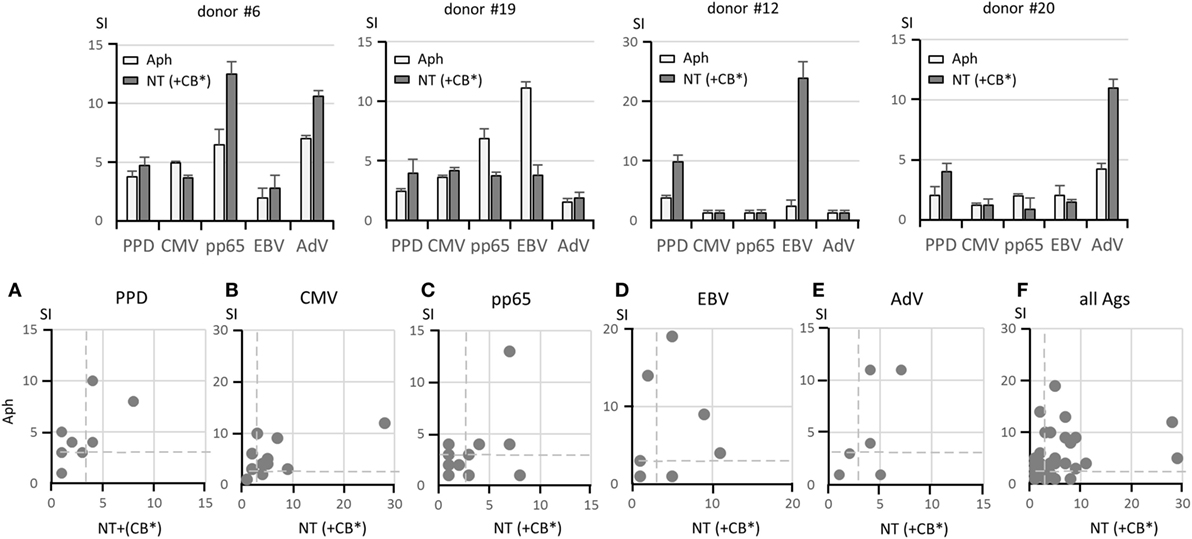
Figure 3. Responses to soluble antigens. The upper panels show representative lymphoproliferation assays of Aph and non-target (NT) (+irradiated CB*) samples from four donors stimulated with the following antigens: PPD, CMV lysate, CMV pp65 pepmix, EBV lysate, and AdV lysate. Different response profiles are shown. Responses to PPD, CMV lysate, CMV pp65 pepmix, EBV lysate, and AdV lysate in 20 pairs of Aph and NT (+CB*) samples are shown in the lower panels (A–E) as dot plots. A comprehensive view of responses to all antigens is given in panel (F). Results are shown as stimulation indexes (SI). Dashed lines indicate SI = 3. Responses with identical SI in the two fractions overlap in the same position, and thus fewer than 20 dots may appear. A statistically significant correlation could not be established between Aph and NT (+CB*) responses (Pearson’s coefficient test).
Ability of B Cells to Process and Present Protein and Peptide Antigens to Specific T Cells
After demonstrating that αβ T cells in the NT fractions are functionally intact, we evaluated whether B cells present in the same fractions were capable of exerting their APC function or whether additional professional APC, such MoDC present in the CB fractions, were necessary for optimal T-cell activation. By using protein antigens, such as viral lysates in addition to the CMV pp65 peptide mixture, it was possible to test the antigen uptake and processing ability and not simply the APC function of B cells. Figure 4 demonstrates that MoDC in the CB fractions irradiated at 30 Gy, which abolishes the function of residual T cells but preserves the function of APC, were of no advantage when added as supplementary APC and, on certain occasions, were even detrimental to specific T-cell responses. This is shown in Figures 4A–D, in which all data are reported for each antigen. It appears that B cells contained in the NT fractions were fully functional as APC and required no additional support. The overall superior responsiveness of NT cells alone is evident also from Figure 4E, in which SI for all antigens have been plotted.
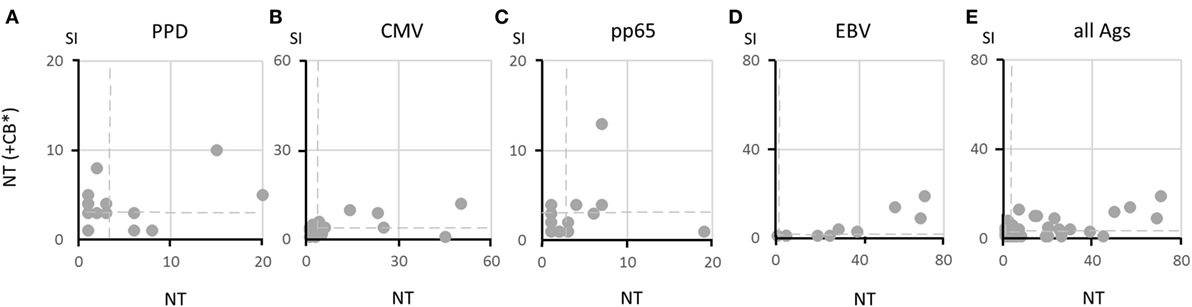
Figure 4. Ability of B cells to present soluble antigens to specific T cells. Responses to various antigens by 20 pairs of non-target (NT) (+irradiated CB*) and NT samples are shown in panels (A–D) as dot plots. Responses with identical stimulation indexes (SI) in the two fractions overlap in the same position, and thus fewer than 20 dots may appear. Dashed lines indicate SI = 3. Responses to all antigens are summarized in panel (E), showing that responses by NT cells are more vigorous than responses by NT (+CB*) cells. A statistically significant correlation could not be established between Aph and NT (+CB*) responses (Pearson’s coefficient test).
Comparison of Response in Aph and in NT Samples
For a better evaluation of the discrepancies between NT and Aph responses observed on several occasions, Figure 5 shows the percent positive responses defined by an SI ≥ 3 to each tested antigen in all Aph samples and NT samples. The highest frequency of positive responses in Aph and/or NT samples was seen with CMV (55% for Aph and 60% for NT), while the lowest frequency was observed for AdV (24% for Aph and 16% for NT).
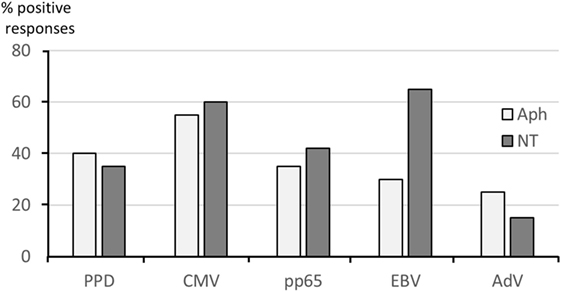
Figure 5. Frequency of antigen-specific responses in paired samples. Positive responses to soluble antigens defined by stimulation indexes (SI) ≥3 are shown by bars referring to Aph (light gray) and to non-target (NT) (dark gray) samples.
Discussion
Recovery of antigen-specific T cells from G-CSF mobilized aphereses has been the subject of some conflicting reports. While G-CSF can increase the number of circulating lymphocytes (40–42), the observation that G-CSF inhibits the production of Th1-type cytokines raised concerns on the use of mobilized T cells with respect to immune reconstitution (43–47). Nevertheless, T cells from mobilized donors proved suitable for specific selection using either multimers (48, 49) or activation markers for enrichment and expansion (50, 51), with effectiveness comparable to that of T cells from non-mobilized samples.
In the perspective of selecting virus-specific T cells from NT fractions, we need to discuss several aspects related to the isolation procedure. Basically, two approaches are to be considered, one based on multimer selection and one based on selection with the cytokine secretion system (CSS).
In the first case, the multimer technology, initially described in Ref. (52), was successfully applied for selection and reinfusion of virus-specific T cells (53, 54). Nevertheless, the introduction of reversible streptamers, which can be eluted from the selected T cells (55), allowed more studies to demonstrate that T cells isolated with these reagents are functionally superior to CTL lines with the same CMV specificity in terms of protective potential (56), provide better protection when expressing high avidity TCR (57), have a remarkable proliferative capacity after infusion (29), can be serially transferred between experimental animals thanks to their stemness (30), and do not need to be specific for immunodominant epitopes (58). Finally, specific T cells can be isolated with minimal manipulation as cellular products for clinical use (31). Therefore, streptamer-enriched T cells can be proposed for immune reconstitution (59), as well as for immunotherapy of selected tumors (59, 60). A variation on the theme of reversible streptamers was introduced to dissociate multimers from CMV-specific selected cells by coupling onto cobalt-based magnetic beads histidine-tagged MHC molecules, which monomerize in the presence of histidine as specific inhibitor (61).
In the second case, the CSS, described in Ref. (62, 63), was applied for selection of CMV-specific T cells in in vitro studies (64, 65). In vivo studies demonstrated successful immune reconstitution with T cells specific for CMV (66), adenovirus (67), and EBV (68, 69).
Both these approaches are appealing as they allow positive selection of specific and functional T cells in a period of time much shorter than that needed to generate after rounds of antigen stimulation-specific T-cell lines, as it has been used in the past (70–72). We should keep in mind that multimers allow selection to be performed in a few hours with a simple procedure, without the requirement of an activation step, but they can be used only with selected epitopes on a limited array of HLA alleles and mostly for selection of CD8 T cells. In contrast, the CSS procedure requires an activation phase and more complex steps of cell processing, but it can activate both CD4 and CD8 cells simultaneously, is not limited by the available recombinant HLA alleles, and mixtures of peptides from different proteins and viruses can be used.
The use of multimer-selected CMV-specific T cells for immune reconstitution has been described in the past (53, 54), proving to be a rapid and highly effective procedure. This approach has been recently reviewed and advocated (73), but some limitations, intrinsic in the multimer strategy, should be considered. Multimer selection for immune reconstitution is limited to CD8 CTL and restricted to the HLA alleles available at present for multimer construction. Immunodominant epitopes must be known for each pathogen, and different multimers must be constructed with each peptide and each HLA allele. These aspects also apply to multimer-based selection of specific T cells from G-CSF mobilized aphereses (48). In addition, multimer-selected T cells from mobilized samples showed a reduction of antiviral function when compared to non-mobilized products (49).
Several conclusions can be drawn from the experiments presented in our study. First, antigen-specific responses by αβ T cells in the NT fractions were well preserved, in spite of manipulation steps, which included labeling, washing, column passage, and elution. In several cases, responses by NT cells were even higher than those obtained with unprocessed T cells from the Aph fractions. Second, antigen processing and presenting capacity was exerted by B cells in full, as additional APC, such as MoDC from the CB population, did not increase specific T-cell responses. The preserved T-cell function indicates that anti-αβ TCR–bead complexes on T cells did not interfere with cell functions (antigen recognition, proliferation, and cytokine secretion), as demonstrated by vigorous responses of NT cells in comparison with unmanipulated Aph cells. To explain this observation, we propose that only a fraction of TCR molecules are engaged by anti αβ TCR Mab on the cell surface or that antibody–bead complexes are rapidly internalized after cell incubation in culture (Miltenyi, personal communication). Similar to the T-cell hypothesis, anti-CD19–bead complexes on B cells, even if persisting on the cell surface, did not interfere with antigen uptake, processing, and presentation, suggesting that either engagement of CD19 does not affect the APC function or not all CD19 molecules are bound or complexes are promptly recycled from the B cell surface as an active process during cell incubation.
With these considerations in mind, preservation of T-cell function suggests that the discarded NT fractions could be considered as an alternative product for DLI, saving the donor the cost and the discomfort of a new apheretic session. In particular, in case a second Aph cannot be performed for whatever reason, NT cells represent a feasible alternative.
A mean number of 1010 αβ T cells were present in the NT fractions. This number largely exceeds the number of T cells theoretically encompassing a full human repertoire (74, 75). In particular, memory T cells, which represent the subset most relevant for protection from opportunistic infections, is represented by approximately 106 different clonotypes with a frequency of each pathogen-specific T cell (including CD4 and CD8 cells) ranging between 0.05 and 5% in peripheral blood (76). An alternative estimate has been proposed by assessing the diversity of the TCR β chains only by high throughput sequencing (74). In this case, the diversity of the memory repertoire was calculated at around 106 different clonotypes, but, considering combinatorial diversity contributed by TCR α chains, the figure can be remarkably higher. These evaluations imply that each clonal specificity is represented by at least one T cell in a sample containing a few million T cells. Therefore, the discarded NT fractions contain thousands of cells from each memory T-cell clone specific for pathogen antigens, redundantly reflecting scores of replicates of the same memory repertoire. Even if far from being accurate, these figures underline how valuable the NT fractions can be from an immunological point of view.
With respect to the observation that specific responses were occasionally higher in NT cells than in Aph cells (or in NT plus CB cells), we should keep in mind that the simplest functional unit for cellular immune response is represented by one specific T cell interacting with one APC. Other neighboring cells may interfere with this interaction, and a third party may not be welcome. Since NT fractions are enriched in T and B cells, antigen-driven cellular interactions may be facilitated. This may account for better responses in NT fractions than in Aph samples, or than in NT fractions supplemented with CB cells. CB fractions, in fact, in addition to MoDC, contain other cell types, such as NK cells and γδ T cells, which may physically interfere with antigen-driven T–B interactions. On the contrary, it is hard to explain why we occasionally observed specific responses higher in Aph than in NT samples. In this case, it is possible that the column elution procedure for NT fractions, which follows the collection of CB fractions to be transplanted, might occasionally affect T-cell functions in NT samples. The observed variability suggests that T-cell functions should be checked in each preparation.
In case large numbers of T cells are required, an aliquot from the mobilized apheretic donation (48) may not suffice. On the other side, a second Aph is hard to propose in case a matched unrelated donor is used for the allograft, due to donor or registry denial, or it may sometimes not be feasible in the haploidentical donor setting for personal or health reasons. Altogether, these considerations make the use of NT cells particularly appealing.
Our view on recovered NT cells is not limited to their exploitation as a substitute for DLI. Thanks to their intact functions, these cells can be candidates for additional in vitro manipulation before reinfusion. A note of caution should be introduced, though, considering that GCSF has been recently shown to affect some functions of CD8 T cells, without impairing their in vitro expansion (77, 78). In this regard, it will be interesting to test whether this effect is reversible upon in vitro culture. Cell manipulation may include specific allodepletion, selection, and expansion of specific T cells, genetic manipulations to introduce suicide genes, or novel chimeric receptors for their retargeting. These possibilities, which need to be experimentally validated on NT cells, are outlined in Figure 6, with relevant references provided in the legend.
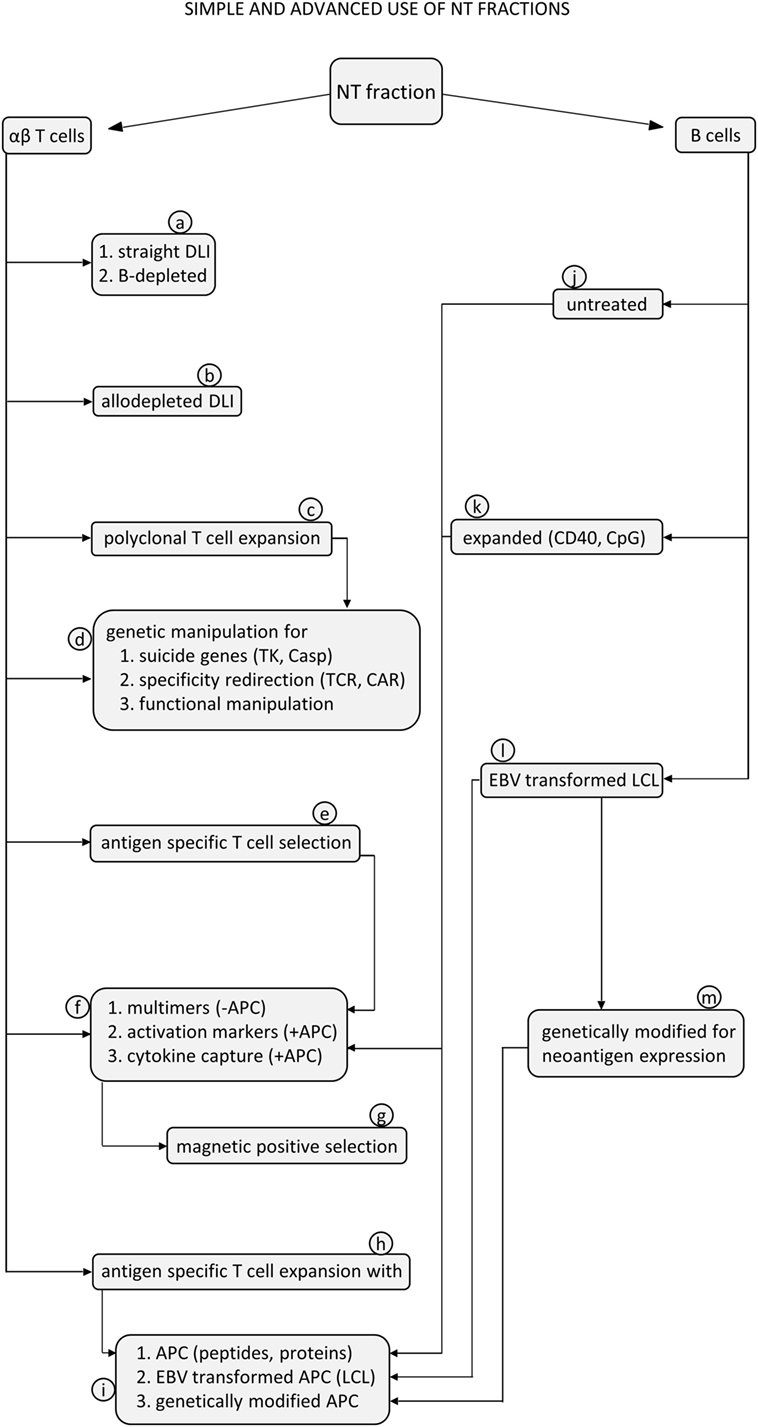
Figure 6. Flow diagram for proposed exploitation of αβ T cells and B cells from non-target (NT) samples using simple or advanced procedures. T cells contained in the NT fractions can be used for unmanipulated donor lymphocyte infusion (DLI) (a1) or after B cells have been removed (a2). This can be achieved by cell preincubation to allow internalization and degradation of the paramagnetic beads, followed by a depletion step with anti-CD19 beads only. T cells can be manipulated to reduce the frequency of alloreactive cells (b) for graft-versus-host disease prevention by using one of the methods already proposed in previous studies, as recently reviewed (75). T cells can be polyclonally expanded with anti-CD3 antibodies (c) (33) to produce large numbers of activated cells ready for subsequent genetic manipulations, such as introduction of suicide genes, like thymidine kinase (TK) or inducible caspase-9 (iCasp9) (d1) (32, 79), for redirection of specificity by introducing a chimeric antigen receptor (d2) (35) or for functional manipulation by adding genes for accessory molecules or cytokines (d3) (35, 80). T cells can be selected based on specificity for antigens of relevant pathogens (e) by using several strategies (f). Multimer selection does not require previous antigen stimulation in the presence of APC (f1), while selection based on expression of activation markers (f2) or on cytokine capture (f3) does require antigen activation with APC. APC can be untreated autologous B cells (j), expanded (k), or modified B cells (l, m). These procedures are followed by positive selection of activated T cells usually based on paramagnetic microbeads (g) to select T cells specific for adenovirus (67), CMV (81), or EBV (68). Finally, T cells can be stimulated and expanded in vitro to generate established T cell lines specific for antigens of relevant pathogens, using different protocols (h) (31). As an example, peptides and proteins can be used in the presence of APC as autologous B cells (i1). EBV-transformed autologous B cells can be used to activate EBV-specific T cells (i2). Genetically modified APC can express antigens of different viruses (i3) (82) to obtain multi-virus-specific T-cell lines. On the other side, B cells can be considered adequate APC under different conditions. B cells can be used untreated (j) or simply irradiated, terminally expanded in vitro with CpG (83), or long-term expanded with anti-CD40 antibodies (k) (84, 85). B cells can be transformed with EBV to generate lymphoblastoid cell lines (LCL) (l), which can directly present EBV antigens to T cells or can be genetically modified to express new viral antigens (m) (82).
With respect to B cells present in the NT fractions, in addition to their intact APC function demonstrated here, three functional features are being tested in experiments in progress, as illustrated in Figure 6. The first one is in vitro expansion with CpG or with anti-CD40 antibodies in order to produce large numbers of APC to be used in restimulation cycles for the generation and expansion of antigen-specific T-cell lines. The second one is related to EBV transformation of these B cells available in large numbers for production of lymphoblastoid cell lines (LCL) to be used for generation of EBV-specific CTL (86, 87) using a faster approach as compared with direct LCL generation from peripheral blood samples. This may permit generation of EBV-specific CTL lines with a procedure swifter than advocated in standard protocols. The third one relates to B-cell removal from the T-cell products. In fact, if multimers are used for T-cell selection on a magnetic column right after the αβ T-cell/B-cell depletion procedure, B cells, which are still coated with anti-CD19 magnetic beads, might be retained by the magnetic column and copurify together with multimer-labeled, antigen-specific T cells. Therefore, a preincubation step is required to allow internalization and degradation of the magnetic beads used for B-cell depletion. In case specific T cells are selected with other methods, based on the expression of activation markers (76) or on the cytokine capture system (62, 63), prior antigen stimulation, which depends on the presenting function of B cells, is needed. In this case, beads used for the first depletion are internalized and degraded during the incubation phase and do not interfere with the second depletion phase. Since specific T cells are positively selected, B cells are excluded from the final product. On the other side, if T cells are stimulated and repeatedly expanded to produce established T-cell lines, B cells present among the cells used as APC can be physically removed by negative selection with anti-CD19 beads or can be neutralized by previous irradiation.
In conclusion, our data are in agreement with other reports testing the possible recovery of cellular products obtained after manipulation procedures (48–51, 88–90), suggesting that fractions comprising αβ T cells and B cells obtained as by-products of ex vivo manipulation (38) of apheretic or bone marrow grafts are a valuable source of cells, which may be useful for adoptive immunotherapy after HSCT.
Ethics Statement
The work described in this study was approved by the institutional Ethics Committee, IRCCS Bambino Gesù Children’s Hospital, Rome, Italy (approval #938/LB), and a written informed consent was obtained from the donors.
Author Contributions
GLP, SDC, MM, and FL conceived and designed the experiments. GLP, SDC, SB, EG, EC, VB, CQ, and IC performed the experiments. BL, PM, DP, LB, and AB selected the donors and contributed with ideas for experimental design and performance. GLP, SDC, and FL wrote the manuscript with edits from AB and MM. All the authors revised and approved the contents of the manuscript.
Conflict of Interest Statement
The authors declare that the research was conducted in the absence of any commercial or financial relationships that could be construed as a potential conflict of interest.
Funding
This work was partially supported by grants from AIRC (Associazione Italiana Ricerca sul Cancro, progetto 5xmille) and IRCCS Bambino Gesù Children’s Hospital to FL (# 201602P003745), by a grant from Bambino Gesù Children’s Hospital to GLP (# 201602T003704), and by a grant from AIRC (MFAG 15925) to AB.
References
1. Aversa F, Tabilio A, Velardi A, Cunningham I, Terenzi A, Falzetti F, et al. Treatment of high-risk acute leukemia with T-cell-depleted stem cells from related donors with one fully mismatched HLA haplotype. N Engl J Med (1998) 339(17):1186–93. doi: 10.1056/NEJM199810223391702
2. Anasetti C. Hematopoietic cell transplantation from HLA partially matched related donors. 2nd ed. In: Thomas ED, Blume KG, Forman SJ, editors. Hematopoietic Cell Transplantation. Malden, MA: Blackwell Science Inc. (1999). p. 904–14.
3. Ciurea SO, Bayraktar UD. “No donor”? Consider a haploidentical transplant. Blood Rev (2015) 29(2):63–70. doi:10.1016/j.blre.2014.09.009
4. Hale G, Cobbold S, Waldmann H. T cell depletion with CAMPATH-1 in allogeneic bone marrow transplantation. Transplantation (1988) 45(4):753–9. doi:10.1097/00007890-198804000-00018
5. Reisner Y, Kapoor N, Kirkpatrick D, Pollack MS, Dupont B, Good RA, et al. Transplantation for acute leukaemia with HLA-A and B nonidentical parental marrow cells fractionated with soybean agglutinin and sheep red blood cells. Lancet (1981) 2(2):327–31. doi:10.1016/S0140-6736(81)90647-4
6. Or-Geva N, Reisner Y. The evolution of T-cell depletion in haploidentical transplantation. Br J Hematol (2015) 172(5):667–84. doi:10.1111/bjh.13868
7. Patterson J, Prentice HG, Brenner MK, Gilmore M, Janossy G, Ivory K, et al. Graft rejection following HLA matched T-lymphocyte depleted bone marrow transplantation. Br J Haematol (1986) 63(2):221–30. doi:10.1111/j.1365-2141.1986.tb05544.x
8. Martin PJ, Hansen JA, Torok-Storb B, Durnam D, Przepiorka D, O’Quigley J, et al. Graft failure in patients receiving T cell-depleted HLA-identical allogeneic marrow transplants. Bone Marrow Transplant (1988) 3(5):445–56.
9. Goldman JM, Szydlo R, Horowitz MM, Gale RP, Ash RC, Atkinson K, et al. Choice of pretransplant treatment and timing of transplants for chronic myelogenous leukemia in chronic phase. Blood (1993) 82(7):2235–8.
10. Horowitz MM, Gale RP, Sondel PM, Goldman JM, Kersey J, Kolb HJ, et al. Graft-versus-leukemia reactions after bone marrow transplantation. Blood (1990) 75(3):555–62.
11. Truitt RL, Johnson B. Principles of graft-vs-leukemia reactivity. Biol Blood Marrow Transplant (1995) 1(2):61–8.
12. Barrett AJ. Mechanisms of the graft-versus-leukemia reaction. Stem Cells (1997) 15(4):248–58. doi:10.1002/stem.150248
13. Appelbaum FR. The current status of hematopoietic cell transplantation. Annu Rev Med (2003) 54:491–512. doi:10.1146/annurev.med.54.101601.152456
14. Gratama JW, van Esser JW, Lamers CH, Tournay C, Löwenberg B, Bolhuis RL, et al. Tetramer-based quantification of cytomegalovirus (CMV)-specific CD8+ T lymphocytes in T-cell-depleted stem cell grafts and after transplantation may identify patients at risk for progressive CMV infection. Blood (2001) 98(5):1358–64. doi:10.1182/blood.V98.5.1358
15. van Esser JW, van der Holt B, Meijer E, Niesters HG, Trenschel R, Thijsen SF, et al. Epstein-Barr virus (EBV) reactivation is a frequent event after allogeneic stem cell transplantation (SCT) and quantitatively predicts EBV-lymphoproliferative disease following T-cell-depleted SCT. Blood (2001) 98(4):972–8. doi:10.1182/blood.V98.4.972
16. Aversa F, Tabilio A, Terenzi A, Velardi A, Falzetti F, Giannoni C, et al. Successful engraftment of T-cell-depleted haploidentical “three-loci” incompatible transplants in leukemia patients by addition of recombinant human granulocyte colony-stimulating factor-mobilized peripheral blood progenitor cells to bone marrow inoculum. Blood (1994) 84(11):3948–55.
17. Vantourout P, Hayday A. Six-of-the-best: unique contributions of γd T cells to immunology. Nat Rev Immunol (2013) 13(2):88–100. doi:10.1038/nri3384
18. Bleakley M, Heimfeld S, Jones LA, Turtle C, Krause D, Riddell SR, et al. Engineering human peripheral blood stem cell grafts that are depleted of naïve T cells and retain functional pathogen-specific memory T cells. Biol Blood Marrow Transplant (2014) 20(5):705–16. doi:10.1016/j.bbmt.2014.01.032
19. Bleakley M, Heimfeld S, Loeb KR, Jones LA, Chaney C, Seropian S, et al. Outcomes of acute leukemia patients transplanted with naive T cell-depleted stem cell grafts. J Clin Invest (2015) 125(7):2677–89. doi:10.1172/JCI81229
20. Teschner D, Distler E, Wehler D, Frey M, Marandiuc D, Langeveld K, et al. Depletion of naive T cells using clinical grade magnetic CD45RA beads: a new approach for GVHD prophylaxis. Bone Marrow Transplant (2014) 49(1):138–44. doi:10.1038/bmt.2013.114
21. Handgretinger R. New approaches to graft engineering for haploidentical bone marrow transplantation. Semin Oncol (2012) 39:664–73. doi:10.1053/j.seminoncol.2012.09.007
22. Bertaina A, Merli P, Rutella S, Pagliara D, Bernardo ME, Masetti R, et al. HLA-haploidentical stem cell transplantation after removal of αβ+ T and B cells in children with nonmalignant disorders. Blood (2014) 124(5):822–6. doi:10.1182/blood-2014-03-563817
23. Maschan M, Shelikhova L, Ilushina M, Kurnikova E, Boyakova E, Balashov D, et al. TCR-alpha/beta and CD19 depletion and treosulfan-based conditioning regimen in unrelated and haploidentical transplantation in children with acute myeloid leukemia. Bone Marrow Transplant (2016) 51(5):668–74. doi:10.1038/bmt.2015.343
24. Kolb HJ, Mittermüller J, Clemm C, Holler E, Ledderose G, Brehm G, et al. Donor leukocyte transfusions for treatment of recurrent chronic myelogenous leukemia in marrow transplant patients. Blood (1990) 76(12):2462–5.
25. Alyea E, Weller E, Schlossman R, Canning C, Webb I, Doss D, et al. T-cell-depleted allogeneic bone marrow transplantation followed by donor lymphocyte infusion in patients with multiple myeloma: induction of graft-versus-myeloma effect. Blood (2001) 98(4):934–9. doi:10.1182/blood.V98.4.934
26. Simula MP, Marktel S, Fozza C, Kaeda J, Szydlo RM, Nadal E, et al. Response to donor lymphocyte infusions for chronic myeloid leukemia is dose-dependent: the importance of escalating the cell dose to maximize therapeutic efficacy. Leukemia (2007) 21(5):943–8. doi:10.1038/sj.leu.2404641
27. Bloor AJ, Thomson K, Chowdhry N, Verfuerth S, Ings SJ, Chakraverty R, et al. High response rate to donor lymphocyte infusion after allogeneic stem cell transplantation for indolent non-Hodgkin lymphoma. Biol Blood Marrow Transplant (2008) 14(1):50–8. doi:10.1016/j.bbmt.2007.04.013
28. Li Pira G, Kapp M, Manca F, Einsele H. Pathogen specific T-lymphocytes for reconstitution of the immunocompromised host. Curr Opin Immunol (2009) 21(5):549–56. doi:10.1016/j.coi.2009.08.006
29. Stemberger C, Graef P, Odendahl M, Albrecht J, Dössinger G, Anderl F, et al. Lowest numbers of primary CD8(+) T cells can reconstitute protective immunity upon adoptive immunotherapy. Blood (2014) 124(4):628–37. doi:10.1182/blood-2013-12-547349
30. Graef P, Buchholz VR, Stemberger C, Flossdorf M, Henkel L, Schiemann M, et al. Serial transfer of single-cell-derived immunocompetence reveals stemness of CD8(+) central memory T cells. Immunity (2014) 41(1):116–26. doi:10.1016/j.immuni.2014.05.018
31. Odendahl M, Grigoleit GU, Bönig H, Neuenhahn M, Albrecht J, Anderl F, et al. Clinical-scale isolation of ’minimally manipulated’ cytomegalovirus-specific donor lymphocytes for the treatment of refractory cytomegalovirus disease. Cytotherapy (2014) 16(9):1245–56. doi:10.1016/j.jcyt.2014.05.023
32. Di Stasi A, Tey SK, Dotti G, Fujita Y, Kennedy-Nasser A, Martinez C, et al. Inducible apoptosis as a safety switch for adoptive cell therapy. N Engl J Med (2011) 365(18):1673–83. doi:10.1056/NEJMoa1106152
33. Levine BL, June CH. Perspective: assembly line immunotherapy. Nature (2013) 498(7455):s17. doi:10.1038/498S17a
34. Tzannou I, Leen AM. Accelerating immune reconstitution after hematopoietic stem cell transplantation. Clin Transl Immunology (2014) 3(2):e11. doi:10.1038/cti.2014.2
35. Gill S, June CH. Going viral: chimeric antigen receptor T-cell therapy for hematological malignancies. Immunol Rev (2015) 263:68–89. doi:10.1111/imr.12243
36. Rutella S, Filippini P, Bertaina V, Li Pira G, Altomare L, Ceccarelli S, et al. Mobilization of healthy donors with plerixafor affects the cellular composition of T-cell receptor (TCR)-αβ/CD19-depleted haploidentical stem cell grafts. J Transl Med (2014) 12(9):240–54. doi:10.1186/s12967-014-0240-z
37. Chaleff S, Otto M, Barfield RC, Leimig T, Iyengar R, Martin J, et al. A large-scale method for the selective depletion of alphabeta T lymphocytes from PBSC for allogeneic transplantation. Cytotherapy (2007) 9(8):746–54. doi:10.1080/14653240701644000
38. Li Pira G, Malaspina D, Girolami E, Biagini S, Cicchetti E, Conflitti G, et al. Selective depletion of αβ T cells and B cells for haploidentical hematopoietic stem cell transplantation. A three-year follow-up of procedure efficiency. Biol Blood Marrow Transpl (2016) 22(11):2056–64. doi:10.1016/j.bbmt.2016.08.006
39. Schumm M, Lang P, Bethge W, Faul C, Feuchtinger T, Pfeiffer M, et al. Depletion of T-cell receptor alpha/beta and CD19 positive cells from apheresis products with the CliniMACS device. Cytotherapy (2013) 15(10):1253–8. doi:10.1016/j.jcyt.2013.05.014
40. Sica S, Rutella S, Di Mario A, Salutari P, Rumi C, Ortu la Barbera E, et al. rhG-CSF in healthy donors: mobilization of peripheral hemopoietic progenitors and effect on peripheral blood leukocytes. J Hematother (1996) 5(4):391–7. doi:10.1089/scd.1.1996.5.391
41. Rutella S, Rumi C, Testa U, Sica S, Teofili L, Martucci R, et al. Inhibition of lymphocyte blastogenic response in healthy donors treated with recombinant human granulocyte colony-stimulating factor (rhG-CSF): possible role of lactoferrin and interleukin-1 receptor antagonist. Bone Marrow Transplant (1997) 20(5):355–64. doi:10.1038/sj.bmt.1700899
42. Hartung T, Doecke WD, Bundschuh D, Foote MA, Gantner F, Hermann C, et al. Effect of filgrastim treatment on inflammatory cytokines and lymphocyte functions. Clin Pharmacol Ther (1999) 66(4):415–24. doi:10.1053/cp.1999.v66.a101210
43. Pan L, Teshima T, Hill GR, Bungard D, Brinson YS, Reddy VS, et al. Granulocyte colony-stimulating factor-mobilized allogeneic stem cell transplantation maintains graft-versus-leukemia effects through a perforin-dependent pathway while preventing graft-versus-host disease. Blood (1999) 93(12):4071–8.
44. Kitabayashi A, Hirokawa M, Hatano Y, Lee M, Kuroki J, Niitsu H, et al. Granulocyte colony-stimulating factor downregulates allogeneic immune responses by posttranscriptional inhibition of tumor necrosis factor-alpha production. Blood (1995) 86(6):2220–7.
45. Arpinati M, Green CL, Heimfeld S, Heuser JE, Anasetti C. Granulocyte-colony stimulating factor mobilizes T helper 2-inducing dendritic cells. Blood (2000) 95(8):2484–90.
46. Sloand EM, Kim S, Maciejewski JP, Van Rhee F, Chaudhuri A, Barrett J, et al. Pharmacologic doses of granulocyte colony-stimulating factor affect cytokine production by lymphocytes in vitro and in vivo. Blood (2000) 95(7):2269–74.
47. Tayebi H, Kuttler F, Saas P, Lienard A, Petracca B, Lapierre V, et al. Effect of granulocyte colony-stimulating factor mobilization on phenotypical and functional properties of immune cells. Exp Hematol (2001) 29(4):458–70. doi:10.1016/S0301-472X(01)00613-0
48. Beloki L, Ramírez N, Olavarría E, Samuel ER, Lowdell MW. Manufacturing of highly functional and specific T cells for adoptive immunotherapy against virus from granulocyte colony-stimulating factor-mobilized donors. Cytotherapy (2014) 16(10):1390–408. doi:10.1016/j.jcyt.2014.05.009
49. Beloki L, Ciaurriz M, Mansilla C, Zabalza A, Perez-Valderrama E, Samuel ER, et al. Assessment of the effector function of CMV-specific CTLs isolated using MHC-multimers from granulocyte-colony stimulating factor mobilized peripheral blood. J Transl Med (2015) 13:165. doi:10.1186/s12967-015-0515-z
50. Samuel ER, Newton K, Mackinnon S, Lowdell MW. Successful isolation and expansion of CMV-reactive T cells from G-CSF mobilized donors that retain a strong cytotoxic effector function. Br J Haematol (2013) 160(1):87–100. doi:10.1111/bjh.12082
51. Clancy LE, Blyth E, Simms RM, Micklethwaite KP, Ma CK, Burgess JS, et al. Cytomegalovirus-specific cytotoxic T lymphocytes can be efficiently expanded from granulocyte colony-stimulating factor-mobilized hemopoietic progenitor cell products ex vivo and safely transferred to stem cell transplantation recipients to facilitate immune reconstitution. Biol Blood Marrow Transplant (2013) 19(5):725–34. doi:10.1016/j.bbmt.2013.01.021
52. Altman JD, Moss PA, Goulder PJ, Barouch DH, McHeyzer MG, Bell JI, et al. Phenotypic analysis of antigen-specific T lymphocytes. Science (1996) 274:94–6. doi:10.1126/science.274.5284.94
53. Cobbold M, Khan N, Pourgheysari B, Tauro S, McDonald D, Osman H, et al. Adoptive transfer of cytomegalovirus-specific CTL to stem cell transplant patients after selection by HLA-peptide tetramers. J Exp Med (2005) 202(3):379–86. doi:10.1084/jem.20040613
54. Uhlin M, Gertow J, Uzunel M, Okas M, Berglund S, Watz E, et al. Rapid salvage treatment with virus-specific T cells for therapy-resistant disease. Clin Infect Dis (2012) 55(8):1064–73. doi:10.1093/cid/cis625
55. Knabel M, Franz TJ, Schiemann M, Wulf A, Villmow B, Schmidt B, et al. Reversible MHC multimer staining for functional isolation of T-cell populations and effective adoptive transfer. Nat Med (2002) 8(6):631–7. doi:10.1038/nm0602-631
56. Pahl-Seibert MF, Juelch M, Podlech J, Thomas D, Deegen P, Reddehase MJ, et al. Highly protective in vivo function of cytomegalovirus IE1 epitope-specific memory CD8 T cells purified by T-cell receptor-based cell sorting. J Virol (2005) 79(9):5400–13. doi:10.1128/JVI.79.9.5400-5413.2005
57. Nauerth M, Weißbrich B, Knall R, Franz T, Dössinger G, Bet J, et al. TCR-ligand koff rate correlates with the protective capacity of antigen-specific CD8+ T cells for adoptive transfer. Sci Transl Med (2013) 5(192):192ra87. doi:10.1126/scitranslmed.3005958
58. Holtappels R, Lemmermann NA, Podlech J, Ebert S, Reddehase MJ. Reconstitution of CD8 T cells protective against cytomegalovirus in a mouse model of hematopoietic cell transplantation: dynamics and inessentiality of epitope immunodominance. Front Immunol (2016) 14(7):232. doi:10.3389/fimmu.2016.00232
59. Schober K, Busch DH. TIL 2.0: more effective and predictive T-cell products by enrichment for defined antigen specificities. Eur J Immunol (2016) 46(6):1335–9. doi:10.1002/eji.201646436
60. Kelderman S, Heemskerk B, Fanchi L, Philips D, Toebes M, Kvistborg P, et al. Antigen-specific TIL therapy for melanoma: a flexible platform for personalized cancer immunotherapy. Eur J Immunol (2016) 46(6):1351–60. doi:10.1002/eji.201545849
61. Tischer S, Kaireit T, Figueiredo C, Hiller O, Maecker-Kolhoff B, Geyeregger R, et al. Establishment of the reversible peptide-major histocompatibility complex (pMHC) class I histamer technology: tool for visualization and selection of functionally active antigen-specific CD8(+) T lymphocytes. Int Immunol (2012) 24(9):561–72. doi:10.1093/intimm/dxs059
62. Manz R, Assenmacher M, Pflüger E, Miltenyi S, Radbruch A. Analysis and sorting of live cells according to secreted molecules, relocated to a cell-surface affinity matrix. Proc Natl Acad Sci U S A (1995) 92(6):1921–5. doi:10.1073/pnas.92.6.1921
63. Brosterhus H, Brings S, Leyendeckers H, Manz RA, Miltenyi S, Radbruch A, et al. Enrichment and detection of live antigen-specific CD4(+) and CD8(+) T cells based on cytokine secretion. Eur J Immunol (1999) 29(12):4053–9. doi:10.1002/(SICI)1521-4141(199912)29:12<4053::AID-IMMU4053>3.0.CO;2-C
64. Feuchtinger T, Lang P, Hamprecht K, Schumm M, Greil J, Jahn G, et al. Isolation and expansion of human adenovirus-specific CD4+ and CD8+ T cells according to IFN-gamma secretion for adjuvant immunotherapy. Exp Hematol (2004) 32(3):282–9. doi:10.1016/j.exphem.2003.12.009
65. Rauser G, Einsele H, Sinzger C, Wernet D, Kuntz G, Assenmacher M, et al. Rapid generation of combined CMV-specific CD4+ and CD8+ T-cell lines for adoptive transfer into recipients of allogeneic stem cell transplants. Blood (2004) 103(9):3565–72. doi:10.1182/blood-2003-09-3056
66. Mackinnon S, Thomson K, Verfuerth S, Peggs K, Lowdell M. Adoptive cellular therapy for cytomegalovirus infection following allogeneic stem cell transplantation using virus-specific T cells. Blood Cells Mol Dis (2008) 40(1):63–7. doi:10.1016/j.bcmd.2007.07.003
67. Feuchtinger T, Matthes-Martin S, Richard C, Lion T, Fuhrer M, Hamprecht K, et al. Safe adoptive transfer of virus-specific T-cell immunity for the treatment of systemic adenovirus infection after allogeneic stem cell transplantation. Br J Haematol (2006) 134(1):64–76. doi:10.1111/j.1365-2141.2006.06108.x
68. Moosmann A, Bigalke I, Tischer J, Schirrmann L, Kasten J, Tippmer S, et al. Effective and long-term control of EBV PTLD after transfer of peptide-selected T cells. Blood (2010) 15(14):2960–70. doi:10.1182/blood-2009-08-236356
69. Bollard CM, Gottschalk S, Torrano V, Diouf O, Ku S, Hazrat Y, et al. Sustained complete responses in patients with lymphoma receiving autologous cytotoxic T lymphocytes targeting Epstein-Barr virus latent membrane proteins. J Clin Oncol (2014) 32:798–808. doi:10.1200/JCO.2013.51.5304
70. Saglio F, Hanley PJ, Bollard CM. The time is now: moving toward virus-specific T cells after allogeneic hematopoietic stem cell transplantation. Cytotherapy (2014) 16:149–59. doi:10.1016/j.jcyt.2013.11.010
71. Naik S, Nicholas SK, Martinez CA, Leen AM, Hanley PJ, Gottschalk SM, et al. Immunotherapy for immunodeficiency disorders with virus-specific T cells. J Allergy Clin Immunol (2016) 137(5):1498–505.e1. doi:10.1016/j.jaci.2015.12.1311
72. Bollard CM, Heslop HE. T cells for viral infections after allogeneic hematopoietic stem cell transplant. Blood (2016) 127(26):3331–40. doi:10.1182/blood-2016-01-628982
73. Ramírez N, Olavarría E. Viral-specific adoptive immunotherapy after allo-SCT: the role of multimer-based selection strategies. Bone Marrow Transplant (2013) 48(10):1265–70. doi:10.1038/bmt.2012.262
74. Robins HS, Campregher PV, Srivastava SK, Wacher A, Turtle CJ, Kahsai O, et al. Comprehensive assessment of T-cell receptor beta-chain diversity in alphabeta T cells. Blood (2009) 114(19):4099–107. doi:10.1182/blood-2009-04-217604
75. Li Pira G, Di Cecca S, Montanari M, Moretta L, Manca F. Specific removal of alloreactive T cells to prevent GvHD in hemopoietic stem cell transplantation: rationale, strategies and perspectives. Blood Rev (2016) 30(4):297–307. doi:10.1016/j.blre.2016.03.001
76. Kern F, Li Pira G, Gratama JW, Manca F, Roederer M. Measuring Ag-specific immune responses: understanding immunopathogenesis and improving diagnostics in infectious disease, autoimmunity and cancer. Trends Immunol (2005) 26:477–84. doi:10.1016/j.it.2005.07.005
77. Bunse CE, Borchers S, Varanasi PR, Tischer S, Figueiredo C, Immenschuh S, et al. Impaired functionality of antiviral T cells in G-CSF mobilized stem cell donors: implications for the selection of CTL donor. PLoS One (2013) 8(12):e77925. doi:10.1371/journal.pone.0077925
78. Bunse CE, Tischer S, Lahrberg J, Oelke M, Figueiredo C, Blasczyk R, et al. Granulocyte colony-stimulating factor impairs CD8(+) T cell functionality by interfering with central activation elements. Clin Exp Immunol (2016) 185(1):107–18. doi:10.1111/cei.12794
79. Marktel S, Magnani Z, Ciceri F, Cazzaniga S, Riddell SR, Traversari C, et al. Immunologic potential of donor lymphocytes expressing a suicide gene for early immune reconstitution after hematopoietic T-cell-depleted stem cell transplantation. Blood (2003) 101(4):1290–8. doi:10.1182/blood-2002-08-2351
80. Heslop HE, Bollard CM, Gottschalk S, Kuehnle I, Huls MH, Gee AP, et al. Immune therapy for EBV infections after hemopoietic stem-cell transplant. Cytotherapy (2002) 4(5):433–4. doi:10.1080/146532402320776071
81. Peggs KS, Thomson K, Samuel E, Dyer G, Armoogum J, Chakraverty R, et al. Directly selected cytomegalovirus-reactive donor T cells confer rapid and safe systemic reconstitution of virus-specific immunity following stem cell transplantation. Clin Infect Dis (2011) 52(1):49–57. doi:10.1093/cid/ciq042
82. Leen AM, Myers GD, Sili U, Huls MH, Weiss H, Leung KS, et al. Monoculture-derived T lymphocytes specific for multiple viruses expand and produce clinically relevant effects in immunocompromised individuals. Nat Med (2006) 12(10):1160–6. doi:10.1038/nm1475
83. Capolunghi F, Cascioli S, Giorda E, Rosaldo MM, Plebani A, Auriti C, et al. CpG drives human transitional B cells to terminally differentiation and production of natural antibodies. J Immunol (2008) 180(2):800–8. doi:10.4049/jimmunol.180.2.800
84. Schultze JL, Michalak S, Seamon MJ, Dranoff G, Jung K, Daley J, et al. CD40-activated human B cells: an alternative source of highly efficient antigen presenting cells to generate autologous antigen-specific T cells for adoptive immunotherapy. J Clin Invest (1997) 100(11):2757–65. doi:10.1172/JCI119822
85. Zand MS, Bose A, Vo T, Coppage M, Pellegrin T, Arend L, et al. A renewable source of donor cells for repetitive monitoring of T- and B-cell alloreactivity. Am J Transplant (2005) 5(1):76–86. doi:10.1111/j.1600-6143.2003.00637.x
86. Roskrow MA, Suzuki N, Gan YJ, Sixbey JW, Ng CY, Kimbrough S, et al. Epstein-Barr virus (EBV)-specific cytotoxic T lymphocytes for the treatment of patients with EBV-positive relapsed Hodgkin’s disease. Blood (1998) 91(8):2925–34.
87. Comoli P, Labirio M, Basso S, Baldanti F, Grossi P, Furione M, et al. Infusion of autologous Epstein-Barr virus (EBV)-specific cytotoxic T cells for prevention of EBV-related lymphoproliferative disorder in solid organ transplant recipients with evidence of active virus replication. Blood (2002) 99(7):2592–8. doi:10.1182/blood.V99.7.2592
88. Bernardo ME, Ingo D, Aiuti A. Bone marrow derived CD34 negative fraction: an alternative source to isolate large numbers of mesenchymal stromal cells. EBMT (2016) abstr P010.
89. Montoro J, Gurreiro M, O’Reilly RJ, Koehne G. CD34 negative fractions of leukapheresies collections permit generation of highly functional donor-derived CMV-specific cytotoxic T lymphocytes. EBMT (2016) abstr P018.
90. Samuel ER, Beloki L, Newton K, Mackinnon S, Lowdell MW. Isolation of highly suppressive CD25+FoxP3+ T regulatory cells from G-CSF-mobilized donors with retention of cytotoxic anti-viral CTLs: application for multi-functional immunotherapy post stem cell transplantation. PLoS One (2014) 9(1):e85911. doi:10.1371/journal.pone.0085911
Keywords: HLA-haploidentical transplantation, graft manipulation, αβ T cells, alloreactivity, antigen-induced activation, B-cell presentation
Citation: Li Pira G, Di Cecca S, Biagini S, Girolami E, Cicchetti E, Bertaina V, Quintarelli C, Caruana I, Lucarelli B, Merli P, Pagliara D, Brescia LP, Bertaina A, Montanari M and Locatelli F (2017) Preservation of Antigen-Specific Functions of αβ T Cells and B Cells Removed from Hematopoietic Stem Cell Transplants Suggests Their Use As an Alternative Cell Source for Advanced Manipulation and Adoptive Immunotherapy. Front. Immunol. 8:332. doi: 10.3389/fimmu.2017.00332
Received: 20 October 2016; Accepted: 08 March 2017;
Published: 23 March 2017
Edited by:
Christopher M. Snyder, Thomas Jefferson University, USAReviewed by:
Ignacio Anegon, University of Nantes, FranceMatthias J. Reddehase, Johannes Gutenberg-Universität Mainz, Germany
Copyright: © 2017 Li Pira, Di Cecca, Biagini, Girolami, Cicchetti, Bertaina, Quintarelli, Caruana, Lucarelli, Merli, Pagliara, Brescia, Bertaina, Montanari and Locatelli. This is an open-access article distributed under the terms of the Creative Commons Attribution License (CC BY). The use, distribution or reproduction in other forums is permitted, provided the original author(s) or licensor are credited and that the original publication in this journal is cited, in accordance with accepted academic practice. No use, distribution or reproduction is permitted which does not comply with these terms.
*Correspondence: Giuseppina Li Pira, Z2l1c2VwcGluYS5saXBpcmEmI3gwMDA0MDtvcGJnLm5ldA==