- 1Internal Medicine III, University Hospital Regensburg, Regensburg, Germany
- 2Regensburg Center for Interventional Immunology, Regensburg, Germany
- 3Department of Surgery, University Hospital Regensburg, Regensburg, Germany
Cytotoxic T lymphocytes and NK cells play an important role in eliminating malignant tumor cells and the number and activity of tumor-infiltrating T cells represent a good marker for tumor prognosis. Based on these findings, immunotherapy, e.g., checkpoint blockade, has received considerable attention during the last couple of years. However, for the majority of patients, immune control of their tumors is gray theory as malignant cells use effective mechanisms to outsmart the immune system. Increasing evidence suggests that changes in tumor metabolism not only ensure an effective energy supply and generation of building blocks for tumor growth but also contribute to inhibition of the antitumor response. Immunosuppression in the tumor microenvironment is often based on the mutual metabolic requirements of immune cells and tumor cells. Cytotoxic T and NK cell activation leads to an increased demand for glucose and amino acids, a well-known feature shown by tumor cells. These close metabolic interdependencies result in metabolic competition, limiting the proliferation, and effector functions of tumor-specific immune cells. Moreover, not only nutrient restriction but also tumor-driven shifts in metabolite abundance and accumulation of metabolic waste products (e.g., lactate) lead to local immunosuppression, thereby facilitating tumor progression and metastasis. In this review, we describe the metabolic interplay between immune cells and tumor cells and discuss tumor cell metabolism as a target structure for cancer therapy. Metabolic (re)education of tumor cells is not only an approach to kill tumor cells directly but could overcome metabolic immunosuppression in the tumor microenvironment and thereby facilitate immunotherapy.
Tumor Metabolism
Accelerated Glucose Metabolism in Tumor Cells—The “Warburg Effect”
Cells need energy to carry out their various functions. Glucose is the primary energy source for most cells and central to cell proliferation and survival. In addition, cells use lipids or amino acids such as glutamine to generate energy in form of ATP and to build biomolecules for cell growth. In non-malignant cells glucose is mainly metabolized via oxidative phosphorylation (OXPHOS), whereas tumor cells primarily use glycolysis for glucose metabolism, a phenomenon first described by Otto Warburg almost a century ago (1). It is clear that this metabolic alteration is important for tumor development and progression and is a hallmark of cancer (2). Vander Heiden and coauthors proposed that highly proliferating cells switch to glycolysis because mitochondria are needed as anabolic organelles for the generation of building blocks (3, 4). Accelerated glycolysis is regulated by hypoxia, oncogenes, and tumor suppressor genes, as well as kinases such as the mammalian target of rapamycin (mTOR).
Hypoxia-inducible factors (HIFs) are stabilized in response to hypoxia and induce transcription of the glucose transporter GLUT-1 and lactate dehydrogenase (LDH) (5, 6). HIF proteins are expressed in the majority of human tumors and can also be induced by the glycolytic end products pyruvate and lactate (7). HIFs also operate in conjunction with oncogenic MYC, an oncogene overexpressed in about 30% of human cancers and known to upregulate glycolytic enzymes such as LDH (8). The mTOR pathway is one of the most dysregulated signaling pathways in human cancer, leading to accelerated glucose metabolism by regulating HIF-1α and MYC (9). It was also shown that the BRAF oncogene causes upregulation of genes involved in glycolysis and its knockdown results in reduced glycolysis (10). Genetic alteration or loss of p53, one of the most frequently mutated genes in cancer, also leads to a decreased oxygen consumption and increased lactate production (11). Accordingly, tumor cells are typically characterized by increased uptake of glucose and positron emission tomography exploits this feature to identify tumors diagnostically.
Glucose is metabolized to lactate, the latter is exported from tumor cells in cotransport with protons by monocarboxylate-transporters (MCT), MCT-1 and MCT-4, which results in an accumulation of lactate lowering the pH in the tumor microenvironment (12). Gatenby and Gillies proposed that the “glycolytic phenotype” of tumor cells confers a growth advantage and is necessary for the evolution of invasive human cancers (13). This hypothesis was confirmed by Walenta et al. who found a correlation between lactate concentration in tumor tissues and the incidence of metastases, as well as a reduced overall survival in cancer patients (14).
Interestingly, tumors can display the Warburg phenotype and possess intact OXPHOS, with some cancer subtypes and cancer stem cells actually depending on mitochondrial respiration (15). Nonetheless, the “Warburg effect” is only one part of the complex tumor metabolome puzzle. Amino acid, lipid, and adenosine metabolism are also adapted to fulfill the metabolic needs of tumor cells.
Alterations in the Key Enzymes of Lipid, Adenosine, and Amino Acid Metabolism
A considerable increase in the extracellular adenosine concentration has been reported for hypoxic tissues. Accordingly, HIF-1α has been shown to regulate the ecto-5′-nucleotidase CD73, which metabolizes adenosine monophosphate to adenosine. CD73 is expressed on the surface of tumor cells and elevated activity is found in many cancer entities (16–18). By contrast, expression of methylthioadenosine phosphorylase (MTAP), which catalyzes the conversion of 5′-deoxy-5′methylthioadenosine (MTA) to adenine and methylthioribose 1-phosphate, is reduced in many tumors including malignant melanoma (19) and hepatocellular carcinoma (20) due to either gene disruption by chromosomal rearrangement or epigenetic silencing. This results in accumulation of MTA in the tumor environment. In case of malignant melanoma, the loss of MTAP expression is linked to a higher invasive potential, leading to the hypothesis that loss of MTAP expression might contribute to metastasis of malignant melanoma (21).
Hypoxia-inducible factor also regulates genes important for lipid metabolism such as cyclooxygenase (COX)-2 (5, 22). COX enzymes are responsible for the synthesis of prostaglandins. While COX-1 is constitutively expressed in almost all tissues, its isoenzyme COX-2 is primarily found in tumors (23) and overexpression is associated with a poor prognosis in breast and ovarian cancer (24, 25). Pharmacological inhibition of COX-2 can block arginase (ARG)-1 induction in mouse lung carcinoma (26, 27) indicating that prostaglandins are important for ARG expression that hydrolyzes arginine to ornithine and urea. ARG is not only expressed in tumor cells but also in tumor-infiltrating myeloid-derived suppressor cells (MDSCs), causing depletion of arginine from the tumor environment (28). Interestingly, in myeloid cells, prostaglandins are not only involved in the regulation of ARG but also upregulate indoleamine 2,3-dioxygenase (IDO) (29), which is the rate-limiting enzyme of tryptophan catabolism through the kynurenine pathway. IDO is overexpressed in many cancers (e.g., melanoma, colon, and renal cell carcinoma) and depletes tryptophan, thus inhibiting T cell proliferation in tumor tissues (30, 31).
Glutamine is the most abundant amino acid in the body and tumors act as “glutamine traps” as high rates of glutamine uptake are characteristic for many tumor cells. The increased turnover of glutamine is in part based on the higher activity and expression of glutaminase (GLS), the first enzyme in glutamine metabolism (4, 32, 33). Accordingly, intra-tumoral glutamine levels are low, and cancer patients exhibit lowered plasma glutamine levels and conversely elevated glutamate concentrations (34).
Immune Cell Metabolism
The metabolism of immune cells has gained increasing attention recently since it is now recognized as a sensitive factor influencing immune cell activation and differentiation. Here, we will focus on glycolytic activity and OXPHOS in immune cells.
Glucose Metabolism in Immune Cells
Early on Otto Warburg observed increased glycolytic activity in leukocytes comparable to tumor cells; however, he interestingly attributed this phenomenon to a preparation artifact (35). It is now accepted that immune cell stimulation causes a shift toward increased glucose metabolism. In proliferating cells, the obvious reason for accelerated glycolysis is the generation of nucleotides and building blocks, e.g., via the connected pentose-phosphate pathway. While several studies have shown that T cell proliferation depends on glucose metabolism (36–41), this concept has been challenged recently since T cell proliferation is not affected by Ldha knockout (42). Besides supporting proliferation, glycolysis is crucial for the functional activity of immune cells. Among these effects, glycolysis has been linked to cytokine production in lymphoid and myeloid cells (42–45). While glycolysis guarantees rapid energy provision, it also participates in posttranscriptional and epigenetic control of IFNγ production (42, 43, 46).
The same signaling pathways are essential for the metabolic regulation in immune cells and tumor cells. AKT, rapidly activated upon T cell receptor (TCR) stimulation, is involved in the induction of glycolysis (47, 48) and determines expression of cytokines and adhesion molecules (47, 49). Furthermore, MYC is transiently upregulated in activated T cells (38) and increases the expression of genes related to glucose and glutamine metabolism (50). Another example is the mTOR complex that is a central regulator of immune cell metabolism and consequently differentiation of T cells into effector or regulatory phenotypes (47, 48, 51). Inhibition of T cell mTORC1 via rapamycin results in inhibition of glycolysis (46) and enhances CD8 memory generation (52, 53).
Not only lymphoid cells but also myeloid cells switch to glucose metabolism upon activation by a wide range of stimuli including lipopolysaccharides (54–56). The shift from OXPHOS toward glycolysis sustains ATP production, while oxygen and NADPH are available for reactive oxygen species (ROS) and nitric oxide (NO) production. ROS promotes IL-6 and TNF production and is important for bacterial defense (57–59). As a result of a truncated citrate cycle, elevated levels of citrate and succinate are detected in activated myeloid cells (60, 61). Succinate stabilizes HIF-1, which can trigger IL-1β synthesis, and accumulation of citrate serves as a precursor for lipid biosynthesis (62).
Role of OXPHOS in Immune Cell Activation
In contrast to lipopolysaccharides, IL-4 stimulation of macrophages does not increase the glycolytic activity but rather commits these cells to OXPHOS and to increased mitochondrial biogenesis (61, 62). Besides glycolysis, OXPHOS is also immediately elevated upon anti-CD3/CD28 stimulation in T cells and supports the transition from quiescent to effector cells (41, 43, 63). OXPHOS can compensate for glucose restriction and IFNγ production is maintained (41, 64, 65). Moreover, mitochondrial ROS production synergizes with Ca2+ influx to activate NF-kB and AP-1 (66, 67) and is important for antigen-specific T cell activation (68). With regard to controlling immune responses, OXPHOS is particularly important for regulatory T cells (Tregs) (69) since their suppressive function is linked to glucose and lipid oxidation (70). Consistent with this general effect, FOXP3 stability is increased by acetyl-CoA and inhibition of lipid oxidation reduces FOXP3 expression and its related suppressive capacity (71). FOXP3 itself shifts metabolism toward oxidation by inhibiting AKT activation and thereby GLUT-1 expression (72). However, proliferating Tregs switch to glycolysis (73) and the induction of Tregs depends on glycolysis (74). Differing results have been published regarding the role of OXPHOS on memory T cell formation. Buck et al. have shown that mitochondrial fusion, favoring OXPHOS, is important for the generation of memory T cells (75), whereas Phan et al. have demonstrated that OXPHOS is not essential for memory T cell differentiation. The observed difference may relate to subset variations between effector memory and central memory T cells (76). In summary, it seems that T cells exhibit some metabolic flexibility and do not rely on a single energy providing pathway.
Amino Acid Metabolism in Immune Cells
T cells, like all immune cells, are auxotroph for many amino acids and proliferation as well as activation results in an increased need for amino acids. Glutamine is essential for proliferation (38, 77, 78) during the initial growth phase as well as for protein and lipid biosynthesis in T cells (38) and for inflammasome activity, phagocytosis, and antigen expression in myeloid cells (79, 80). Glutamine supports OXPHOS, protein biosynthesis, and fuels protein glycosylation (81). Other amino acids such as arginine, tryptophan, and cysteine are also essential for T cell proliferation as well as for macrophage and MDSC function. Depletion of those amino acids by myeloid cells leads to cell cycle arrest in T cells (82–84). Arginine deprivation-induced cell cycle arrest is mediated in part by Rictor/mTORC2 which controls an amino acid-sensitive checkpoint that allows T cells to determine whether the microenvironment contains sufficient resources for proliferation (84). Elevating arginine levels induces a shift from glycolysis to OXPHOS in activated T cells and promotes the generation of central memory-like cells with enhanced survival and antitumor activity (85).
Interplay Between Tumor and Immune Cell Metabolism
Tumor stroma consists of diverse cell populations such as T cells, NK cells, macrophages, dendritic cells, fibroblasts, and endothelial cells. Tumor-infiltrating immune cells represent a double-edged sword as they can support or inhibit tumor growth. Activated lymphoid cells can control tumor growth and malignancies, as shown in reports where dense infiltration with T cells correlates with a better prognosis (86). However, tumors often blunt the activity of tumor-infiltrating lymphocytes (87, 88) and support the differentiation of tumor-associated macrophages (TAMs) or MDSCs that promote tumor growth, e.g., by inhibiting T cells or secreting growth factors (89).
Impact of Rapid Tumor Glucose Metabolism on Immune Cells
The Warburg effect in tumor cells may limit glucose availability and results in lactate accumulation. In renal carcinoma, we showed that accelerated glucose metabolism correlates with low CD8 T cell infiltration (90). Consistent with this finding, high glucose consumption by tumors restricts T cells in a mouse sarcoma model, leading to attenuated mTOR activity, glycolytic capacity, and IFNγ production (91, 92). However, Ho et al. demonstrated that overexpression of phosphoenolpyruvate (PEP) carboxykinase 1 could restore PEP levels and thereby improve T cell function even under glucose restriction (92).
Several studies have demonstrated that (patho)physiologically relevant concentrations of lactate modulate immune cell function in vitro and high levels of lactate correlate with tumor progression and metastatic spread in vivo. Regarding myeloid cells, lactate has been shown to inhibit monocyte activation and dendritic cell differentiation (93, 94). Furthermore, Shime et al. demonstrated that lactate increases the transcription and secretion of IL-23, a tumor-promoting cytokine involved in the generation of Th17 cells, in human monocytes/macrophages (95). Lactic acid also induces M2-polarization in TAMs via HIF-1α stabilization (96). Moreover, reduced Ldha expression in tumor cells resulted in diminished tumor growth and decreased the frequency of splenic MDSCs (97). Furthermore, lactate strongly inhibits the activity of antitumor effector cells such as T cells and NK cells. Husain et al. demonstrated that NK cells from Ldha-depleted tumors showed improved cytolytic function and lactate treatment of NK cells diminished their cytotoxicity. We and others have shown that proliferation and activation of human T cells is suppressed by lactic acid in vitro (98, 99). Treatment of T cells with lactic acid prevented TCR-triggered phosphorylation of JNK, c-Jun, p38, and NFAT activation (100, 101). Recently, our group demonstrated that human melanoma metastases exhibit a “‘Warburg phenotype’” that associated with lactate accumulation. In melanoma patients, LDHA expression correlated with T cell activity and LDHA-associated lactic acid production and acidification impaired IFNγ expression in tumor-infiltrating T cells and NK cells, thereby inhibiting tumor immunosurveillance and promoting tumor growth (101). Increasing evidence supports the view of an immunosuppressive effect of rapid glucose metabolism on development of tumor immunity.
Changes in Tumor Amino Acid and Adenosine Metabolism Suppress T Cell Function
Tumor cells and activated immune cells require a continuous supply of amino acids such as tryptophan, arginine, and glutamine for anabolic macromolecule synthesis. A metabolic competition between tumor cells and immune cells can therefore lead to nutrient deprivation. Regarding availability of tryptophan, IDO is overexpressed in many cancers and IDO-expressing tumor cells are not rejected by specific T cells (30). Accordingly, in colorectal cancer, IDO expression is associated with low T cell infiltration and reduced survival (102). IDO-expressing tumor cells secrete tryptophan metabolites like kynurenines, suppressing cytotoxic effector functions via downregulation of TCR CD3 ζ-chain and induce FOXP3+ Treg differentiation (103). In line, upregulation of IDO is associated with a high infiltration of FOXP3+ cells in thyroid carcinoma (104). Arginine depletion occurs in ARG or NO synthase overexpressing tumors, subsequently leading to unresponsive T cells (105). Furthermore, arginine deprivation by tumor-infiltrating MDSCs impairs T cell function (106). Interestingly, arginine depletion not only blunts the antitumor T cell responses but can also induce MDSC generation in vivo (107). Moreover, MDSCs resembling tumor-associated M2 macrophages rely on glutamine metabolism, whereas M1 macrophages are characterized by increased glycolytic flux (60). Glutamine deprivation promotes Treg generation (108) and often results in glutamate accumulation, which in turn suppresses T cell activity (109, 110). Therefore, the balance of amino acids within a tumor has substantial effects on the development of the local immune response.
CD73 expression on tumor cells results in adenosine accumulation in the tumor microenvironment that inhibits activation and cytotoxic capacity of T and NK cells (111, 112). Besides T cell inhibition, adenosine has a positive impact on myeloid cells. Adenosine-generating mouse Lewis lung carcinoma cells attract myeloid cells that differentiate into TAMs, which promote tumor growth (113). Additionally, we could show that MTA suppresses antigen-specific T cell proliferation, activation, and cytokine production via inhibition of AKT and protein methylation (114). CD73 and COX-2 both are regulated by hypoxia and HIF. Tumor cells frequently display increased COX-2 activity and prostaglandin secretion, thus suppressing T cell function and inducing MDSCs (115). Other lipids such as gangliosides are synthesized and shed by tumor cells, especially under hypoxia. Circulating gangliosides have been shown to suppress T cell function thereby contributing to immunosuppression (116). However, a competition for lipids between tumor cells and immune cells as shown for glucose has not been reported so far, thus immune cell function should not be restricted due to limited fatty acid availability.
Targeting Tumor Metabolism
Alterations in tumor cell metabolism represent attractive targets for the development of anticancer drugs. However, targeting tumor cell metabolism may also harm immune cell functions that contribute to tumor elimination. This influence on targeting overlapping metabolic requirements of tumor and immune cells needs to be considered especially when immunotherapy is combined with antimetabolic drugs.
Targeting the “Warburg Effect” and Mitochondrial Activity
Early after Warburg’s observation that tumor cells show major differences in glucose metabolism compared to non-malignant cells, some attempts focused on the inhibition of tumor glucose metabolism for cancer treatment (117). These studies used 2-deoxyglucose (2-DG), a non-metabolizable glucose analog and inhibitor of hexokinase, the enzyme that catalyzes the initial step of glycolysis. This approach has regained attention during the last years (118, 119) and new drugs have been developed such as the hexokinase inhibitor 3-bromopyruvate (120). We and others have shown that inhibition of glycolysis by 2-DG sensitizes acute lymphoblastic leukemia cells to glucocorticoids (121, 122). 2-DG severely disturbs T cell proliferation and activation, although its effects may reach beyond glycolysis inhibition (41, 46). These results suggest that anti-glycolytic drugs should inhibit T cell function. Surprisingly, deletion of Ldha in T cells did not appreciably affect proliferation and growth, but did reduce IFNγ production (42). Therefore, immunological “side effects” of LDH inhibitors like that of the small-molecule inhibitor FX11 or Galloflavin should be considered when administered for tumor therapy (123, 124).
Conflicting results have been reported regarding dichloroacetate (DCA), which induces a shift from glycolysis to OXPHOS and inhibits growth of tumor cells in vitro and in murine tumor models. DCA was found to synergize with 2-DG in complex IV deficient cells (125), whereas other researchers have demonstrated that it suppresses apoptosis induction by cisplatin and doxorubicin. Unfortunately, DCA is not tumor cell specific, therefore, the same shift in glucose metabolism occurs in immune cells, leading to induction of FOXP3+ Tregs (126).
Targeting the lactate transporters MCT-1 to -4 represents another approach to overcome the “Warburg effect” in cancer cells. The second-generation MCT-1/MCT-2 inhibitor (AZD3965) is currently in phase I clinical trials for advanced solid tumors and diffuse large B cell lymphomas (http://www.clinicaltrials.gov/ct2/show/NCT01791595). However, inhibition of MCT-1/-2 also reduces T cell proliferation (127). Recently, Eichner et al. described that thalidomide, lenalidomide, and pomalidomide destabilize the CD147–MCT-1 complex that results in a loss of cell surface expression of MCT-1 (128). However, MCT-1 suppression may be of limited efficacy as many tumor cells express not only MCT-1 but also MCT-4. Lenalidomide has been shown to promote IL-2 expression in T cells (129), raising the possibility that application of lenalidomide could suppress tumor cell proliferation without affecting T cells.
We have investigated the effect of diclofenac, a non-steroidal anti-inflammatory drug, on glucose metabolism and showed that diclofenac is taken up by tumor cells and interferes with lactate secretion (130). Recently, the impact of diclofenac on lactate transport was confirmed in MCT-expressing oocytes (131). In a glioma model, diclofenac lowered lactate levels, decreased tumor growth, and tumor-infiltrating dendritic cells regained their capacity to produce IL-12. Moreover, diclofenac reduced the number of tumor-infiltrating Tregs (132). Application of diclofenac should therefore be feasible even in combination with immunotherapies.
A well-known master regulator of tumor and immune cell metabolism is mTOR. Analogs of rapamycin, an immunosuppressive drug, have been approved for treatment of some cancers based on direct effects on tumor cell proliferation, glycolysis and inhibition of angiogenesis (133, 134). From another perspective, however, treatment with rapamycin reduces the proliferation of effector T cells and stabilizes/expands Tregs (135, 136), but at the same time can increase the presence of antitumor CD8 effector memory cells (137, 138). Therefore, mTOR inhibition has both potentially positive and negative effects on tumor immunity, which are worthy of further investigation. Interestingly, these dual properties of immunosuppression and immune activation may be taken advantage of in the setting of posttransplantation malignancies that plague organ transplant recipients (139).
Besides glycolysis, OXPHOS is also a possible target structure in cancer cells. Several reports have described anticancer effects of biguanides, such as the diabetes therapeutics metformin and phenformin, which are known to inhibit the mitochondrial complex I. Interestingly, those effects seem to be partially immune-mediated as metformin improved T cell function in vivo (140). Furthermore, sorafenib limits respiration in tumor cells and concomitantly decreases Treg numbers in patients (141). Further investigations along this line should prove to be informative.
Direct and Indirect Targeting of Amino Acid Metabolism
The dependency of tumor cells on extracellular arginine led to the development of arginine-depleting drugs, most prominently ADI-PEG20 (142). However, arginine depletion is clearly a double-edged sword in tumor immunology as arginine availability is crucial for proper T cell function. The same holds true for the application of GLS inhibitors. Such drugs might not only affect tumor cells but also impede T cell function. As arginine, tryptophan, and glutamine are essential for T cell function, it might be more appropriate to prevent amino acid depletion by tumor cells or myeloid cells instead of reducing amino acid levels. This approach is currently tested in a clinical trial with CB-1158, an ARG inhibitor, in combination with checkpoint therapy (NCT02903914).
In line, pharmacological inhibition of COX-2 blocks ARG-1 expression in MDSC and prevents the local and systemic expansion of MDSCs, leading to a lymphocyte-mediated antitumor response (26, 27). Immunotherapeutic approaches might therefore benefit from a concurrent blockade of COX-2 activity (143). Moreover, COX is involved in the upregulation of IDO expression in myeloid cells (29). Therefore, pharmacological inhibition of COX could also reduce tryptophan depletion by IDO-expressing tumor and tumor-infiltrating cells. Direct targeting of IDO with siRNA promoted antitumor immunity in vivo in a murine bladder tumor model (144) and IDO-silenced dendritic cells enhanced tumor antigen-specific T cell proliferation, cytotoxic activity, and decreased Treg numbers (145). Drugs targeting this pathway are already in clinical trials with the aim to revert cancer-induced immunosuppression (146).
Combination of Antimetabolic Targeting with Immunotherapy
Clinical benefits from immune-checkpoint inhibition are still modest due to the tumor microenvironment facilitating immune escape. Therefore, an immune- and antimetabolic combination treatment could be a promising strategy. Allard et al. demonstrated that targeted blockade of CD73 significantly enhances the therapeutic activity of anti-PD-1 and anti-CTLA-4 monoclonal antibodies (147). In line, Zelenay and colleagues showed that combination of COX-1 and COX-2 inhibitors with checkpoint blockade immunotherapy can result in melanoma eradication (148). Combining these basic forms of therapy holds real promise for the future.
Summary and Conclusion
The antitumor immune response is not only suppressed by an altered tumor metabolism but also by the metabolism of tumor-associated cell populations. Tumor cells and immunoregulatory myeloid cells such as MDSCs deprive neighboring cells of essential amino acids or sugars thus removing fuels for antitumor immunity. In addition, accumulation of “waste products” such as lactate, glutamate, PGE2, or kynurenines further limit lymphoid antitumor effector functions (Figure 1).
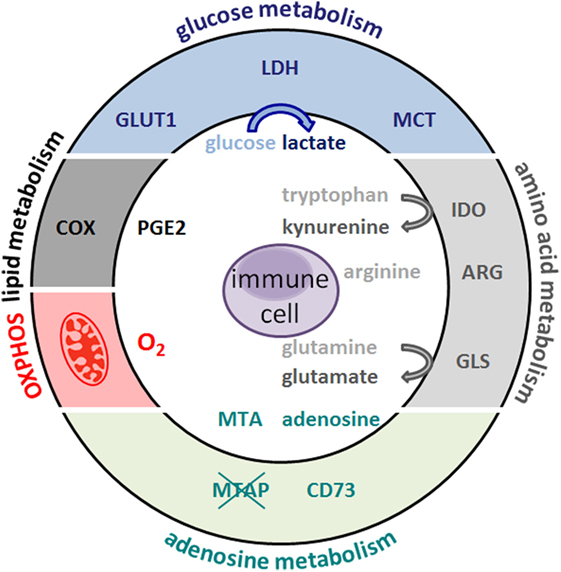
Figure 1. Metabolic hallmarks of tumor cells and the interplay between tumor cells and immune cells. Tumor cells exhibit high expression of glucose transporteres (GLUT), lactate dehydrogenase (LDH), cyclooxygenase (COX), arginase (ARG), indolamine 2,3-dioxygenase (IDO), glutaminase (GLS), and oxidative phosphorylation (OXPHOS). As a consequence, glucose and the amino acids arginine, tryptophan, and glutamine are depleted from the tumor microenvironment and nutrient restriction leads to an anergic status of antitumoral cytotoxic T cells. In addition, accelerated glycolysis by tumor cells results in lactate production and secretion via monocarboxylate-transporters (MCT). Lactate and other metabolites, such as glutamate, prostaglandins (PGE2), and kynurenines, affect immune cells. Overexpression of the ecto-5′-nucleotidase (CD73) leads to adenosine formation; loss of methylthioadenosine phosphorylase (MTAP) results in methylthioadenosine (MTA) accumulation in the tumor environment.
Several therapeutic strategies aim to target tumor metabolism (Figure 2). However, stimulated immune cells with antitumor potential are known to be metabolically active and thus potentially sensitive to metabolic modulation. Therefore, pharmacological strategies should optimally target metabolic pathways that are differently utilized by pro-tumor and antitumor cell populations. This approach is exemplified by the effect of COX inhibitors, where they re-educate MDSCs by decreasing expression of amino acid-depleting enzymes such as IDO or ARG, thus releasing the brake on antitumor responses of T cells that rely on tryptophan and arginine abundance. Another example of a fine-tuned metabolic intervention could be pharmacological reduction of lactate efflux leading to decreased tumor cell proliferation, while supporting antitumor immune responses. Boosting antitumor immune function by antimetabolic treatments could also increase the efficacy of immunotherapies such as checkpoint blockade and may represent future avenues in the treatment of cancer patients.
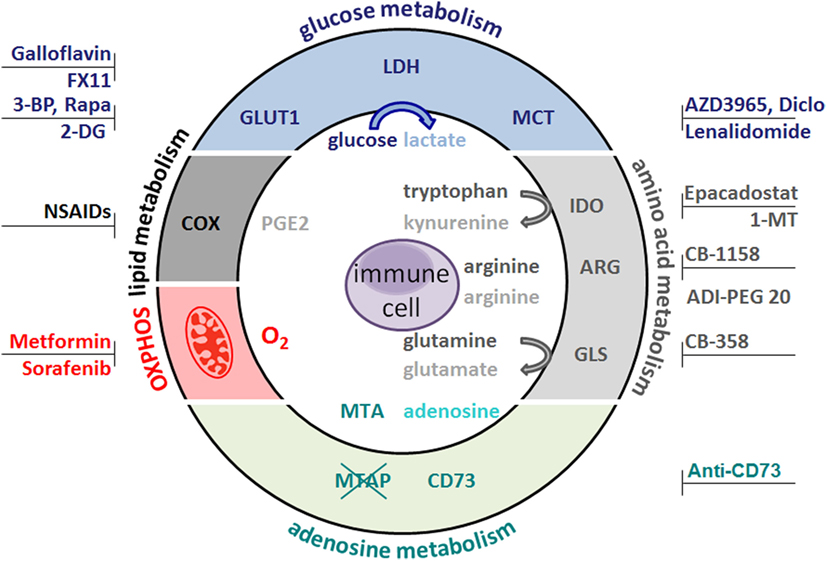
Figure 2. Metabolic target structures in tumor cells and possible inhibitors. Glucose metabolism is an attractive target for cancer therapy. Rapamycin (Rapa) inhibits the mammalian target of rapamycin pathway and glycolysis. 2-Deoxyglucose (2-DG) and bromopyruvate (3-BP) target hexokinase II, the rate-limiting enzyme of the glycolytic pathway. The lactate dehydrogenase (LDH) inhibitors FX11 and galloflavin block lactate production. Diclofenac (Diclo), lenalidomide, and AZD3965 limit lactate secretion via blocking lactate transporters (MCT). Oxidative phosphorylation (OXPHOS) is diminished by metformin and sorafenib. CB-389 is a glutaminase (GLS) inhibitor elevating glutamine levels while concomittantly decreasing glutamate. CB-1158 inhibits arginase (ARG) and thereby increases arginine levels, whereas ADI-PEG20 depletes arginine. Antibodies against the ecto-5′-nucleotidase (CD73) inhibit adenosine formation. Non-steroidal anti-inflammatory drugs (NSAIDs) block cyclooxygenase (COX) activity and decrease prostaglandin (PGE2) production. Indolamine 2,3-dioxygenase (IDO) can be targeted by Epacadostat and 1-methyl-tryptophan (1-MT), which results in lower kynurenine secretion and higher tryptophan levels.
Author Contributions
KR, KS, EG, GK, KP, PS, and MK have designed the figures and wrote the manuscript.
Conflict of Interest Statement
The authors declare that the research was conducted in the absence of any commercial or financial relationships that could be construed as a potential conflict of interest.
The reviewer AZ and handling editor declared their shared affiliation, and the handling editor states that the process nevertheless met the standards of a fair and objective review.
Funding
This work was funded by the German Research Foundation (KFO262) and the Regensburg Center for Interventional Immunology, Regensburg, Germany.
References
1. Warburg O, Wind F, Negelein E. The metabolism of tumors in the body. J Gen Physiol (1927) 8(6):519–30.
2. Hanahan D, Weinberg RA. Hallmarks of cancer: the next generation. Cell (2011) 144(5):646–74. doi:10.1016/j.cell.2011.02.013
3. Vander Heiden MG, Cantley LC, Thompson CB. Understanding the Warburg effect: the metabolic requirements of cell proliferation. Science (2009) 324(5930):1029–33. doi:10.1126/science.1160809
4. Keibler MA, Wasylenko TM, Kelleher JK, Iliopoulos O, Vander Heiden MG, Stephanopoulos G. Metabolic requirements for cancer cell proliferation. Cancer Metab (2016) 4:16. doi:10.1186/s40170-016-0156-6
5. Brahimi-Horn MC, Pouyssegur J. HIF at a glance. J Cell Sci (2009) 122(Pt 8):1055–7. doi:10.1242/jcs.035022
6. Soga T. Cancer metabolism: key players in metabolic reprogramming. Cancer Sci (2013) 104(3):275–81. doi:10.1111/cas.12085
7. Talks KL, Turley H, Gatter KC, Maxwell PH, Pugh CW, Ratcliffe PJ, et al. The expression and distribution of the hypoxia-inducible factors HIF-1alpha and HIF-2alpha in normal human tissues, cancers, and tumor-associated macrophages. Am J Pathol (2000) 157(2):411–21. doi:10.1016/S0002-9440(10)64554-3
8. Shim H, Dolde C, Lewis BC, Wu CS, Dang G, Jungmann RA, et al. c-Myc transactivation of LDH-A: implications for tumor metabolism and growth. Proc Natl Acad Sci U S A (1997) 94(13):6658–63. doi:10.1073/pnas.94.13.6658
9. Iurlaro R, Leon-Annicchiarico CL, Munoz-Pinedo C. Regulation of cancer metabolism by oncogenes and tumor suppressors. Methods Enzymol (2014) 542:59–80. doi:10.1016/B978-0-12-416618-9.00003-0
10. Hall A, Meyle KD, Lange MK, Klima M, Sanderhoff M, Dahl C, et al. Dysfunctional oxidative phosphorylation makes malignant melanoma cells addicted to glycolysis driven by the (V600E)BRAF oncogene. Oncotarget (2013) 4(4):584–99. doi:10.18632/oncotarget.965
11. Matoba S, Kang JG, Patino WD, Wragg A, Boehm M, Gavrilova O, et al. p53 regulates mitochondrial respiration. Science (2006) 312(5780):1650–3. doi:10.1126/science.1126863
12. Halestrap AP. The monocarboxylate transporter family – structure and functional characterization. IUBMB Life (2012) 64(1):1–9. doi:10.1002/iub.573
13. Gatenby RA, Gillies RJ. Why do cancers have high aerobic glycolysis? Nat Rev Cancer (2004) 4(11):891–9. doi:10.1038/nrc1478
14. Walenta S, Wetterling M, Lehrke M, Schwickert G, Sundfor K, Rofstad EK, et al. High lactate levels predict likelihood of metastases, tumor recurrence, and restricted patient survival in human cervical cancers. Cancer Res (2000) 60(4):916–21.
15. Obre E, Rossignol R. Emerging concepts in bioenergetics and cancer research: metabolic flexibility, coupling, symbiosis, switch, oxidative tumors, metabolic remodeling, signaling and bioenergetic therapy. Int J Biochem Cell Biol (2015) 59:167–81. doi:10.1016/j.biocel.2014.12.008
16. Bardot V, Dutrillaux AM, Delattre JY, Vega F, Poisson M, Dutrillaux B, et al. Purine and pyrimidine metabolism in human gliomas: relation to chromosomal aberrations. Br J Cancer (1994) 70(2):212–8. doi:10.1038/bjc.1994.282
17. Durak I, Cetin R, Canbolat O, Cetin D, Yurtarslani Z, Unal A. Adenosine deaminase, 5’-nucleotidase, guanase and cytidine deaminase activities in gastric tissues from patients with gastric cancer. Cancer Lett (1994) 84(2):199–202. doi:10.1016/0304-3835(94)90376-X
18. Canbolat O, Durak I, Cetin R, Kavutcu M, Demirci S, Ozturk S. Activities of adenosine deaminase, 5’-nucleotidase, guanase, and cytidine deaminase enzymes in cancerous and non-cancerous human breast tissues. Breast Cancer Res Treat (1996) 37(2):189–93. doi:10.1007/BF01806500
19. Stevens AP, Spangler B, Wallner S, Kreutz M, Dettmer K, Oefner PJ, et al. Direct and tumor microenvironment mediated influences of 5’-deoxy-5’-(methylthio)adenosine on tumor progression of malignant melanoma. J Cell Biochem (2009) 106(2):210–9. doi:10.1002/jcb.21984
20. Hellerbrand C, Muhlbauer M, Wallner S, Schuierer M, Behrmann I, Bataille F, et al. Promoter-hypermethylation is causing functional relevant downregulation of methylthioadenosine phosphorylase (MTAP) expression in hepatocellular carcinoma. Carcinogenesis (2006) 27(1):64–72. doi:10.1093/carcin/bgi201
21. Behrmann I, Wallner S, Komyod W, Heinrich PC, Schuierer M, Buettner R, et al. Characterization of methylthioadenosin phosphorylase (MTAP) expression in malignant melanoma. Am J Pathol (2003) 163(2):683–90. doi:10.1016/S0002-9440(10)63695-4
22. Kaidi A, Qualtrough D, Williams AC, Paraskeva C. Direct transcriptional up-regulation of cyclooxygenase-2 by hypoxia-inducible factor (HIF)-1 promotes colorectal tumor cell survival and enhances HIF-1 transcriptional activity during hypoxia. Cancer Res (2006) 66(13):6683–91. doi:10.1158/0008-5472.CAN-06-0425
23. Warner TD, Mitchell JA. Cyclooxygenases: new forms, new inhibitors, and lessons from the clinic. FASEB J (2004) 18(7):790–804. doi:10.1096/fj.03-0645rev
24. Denkert C, Weichert W, Winzer KJ, Muller BM, Noske A, Niesporek S, et al. Expression of the ELAV-like protein HuR is associated with higher tumor grade and increased cyclooxygenase-2 expression in human breast carcinoma. Clin Cancer Res (2004) 10(16):5580–6. doi:10.1158/1078-0432.CCR-04-0070
25. Denkert C, Winzer KJ, Hauptmann S. Prognostic impact of cyclooxygenase-2 in breast cancer. Clin Breast Cancer (2004) 4(6):428–33. doi:10.3816/CBC.2004.n.006
26. Rodriguez PC, Hernandez CP, Quiceno D, Dubinett S, Zabaleta J, Ochoa AC. COX-2 inhibition in tumor cells blocks arginase I induction in myeloid suppressor cells. J Immunother (2005) 28(6):652–652. doi:10.1097/01.cji.0000191079.36286.90
27. Rodriguez PC, Hernandez CP, Quiceno D, Dubinett SM, Zabaleta J, Ochoa JB, et al. Arginase I in myeloid suppressor cells is induced by COX-2 in lung carcinoma. J Exp Med (2005) 202(7):931–9. doi:10.1084/jem.20050715
28. Rodriguez PC, Ernstoff MS, Hernandez C, Atkins M, Zabaleta J, Sierra R, et al. Arginase I-producing myeloid-derived suppressor cells in renal cell carcinoma are a subpopulation of activated granulocytes. Cancer Res (2009) 69(4):1553–60. doi:10.1158/0008-5472.CAN-08-1921
29. Braun D, Longman RS, Albert ML. A two-step induction of indoleamine 2,3 dioxygenase (IDO) activity during dendritic-cell maturation. Blood (2005) 106(7):2375–81. doi:10.1182/blood-2005-03-0979
30. Uyttenhove C, Pilotte L, Theate I, Stroobant V, Colau D, Parmentier N, et al. Evidence for a tumoral immune resistance mechanism based on tryptophan degradation by indoleamine 2,3-dioxygenase. Nat Med (2003) 9(10):1269–74. doi:10.1038/nm934
31. Lob S, Konigsrainer A, Zieker D, Brucher BL, Rammensee HG, Opelz G, et al. IDO1 and IDO2 are expressed in human tumors: levo- but not dextro-1-methyl tryptophan inhibits tryptophan catabolism. Cancer Immunol Immunother (2009) 58(1):153–7. doi:10.1007/s00262-008-0513-6
32. Aledo JC, Segura JA, Medina MA, Alonso FJ, Nunez de Castro I, Marquez J. Phosphate-activated glutaminase expression during tumor development. FEBS Lett (1994) 341(1):39–42. doi:10.1016/0014-5793(94)80236-X
34. Droge W, Eck HP, Betzler M, Naher H. Elevated plasma glutamate levels in colorectal carcinoma patients and in patients with acquired immunodeficiency syndrome (AIDS). Immunobiology (1987) 174(4–5):473–9. doi:10.1016/S0171-2985(87)80019-0
35. Warburg O, Gawehn K, Geissler AW. [Metabolism of leukocytes]. Z Naturforsch B (1958) 13B(8):515–6.
36. Newsholme EA, Crabtree B, Ardawi MS. The role of high rates of glycolysis and glutamine utilization in rapidly dividing cells. Biosci Rep (1985) 5(5):393–400. doi:10.1007/BF01116556
37. Jacobs SR, Herman CE, Maciver NJ, Wofford JA, Wieman HL, Hammen JJ, et al. Glucose uptake is limiting in T cell activation and requires CD28-mediated Akt-dependent and independent pathways. J Immunol (2008) 180(7):4476–86. doi:10.4049/jimmunol.180.7.4476
38. Wang R, Dillon CP, Shi LZ, Milasta S, Carter R, Finkelstein D, et al. The transcription factor Myc controls metabolic reprogramming upon T lymphocyte activation. Immunity (2011) 35(6):871–82. doi:10.1016/j.immuni.2011.09.021
39. van der Windt GJ, Pearce EL. Metabolic switching and fuel choice during T-cell differentiation and memory development. Immunol Rev (2012) 249(1):27–42. doi:10.1111/j.1600-065X.2012.01150.x
40. Macintyre AN, Gerriets VA, Nichols AG, Michalek RD, Rudolph MC, Deoliveira D, et al. The glucose transporter Glut1 is selectively essential for CD4 T cell activation and effector function. Cell Metab (2014) 20(1):61–72. doi:10.1016/j.cmet.2014.05.004
41. Renner K, Geiselhoringer AL, Fante M, Bruss C, Farber S, Schonhammer G, et al. Metabolic plasticity of human T cells: preserved cytokine production under glucose deprivation or mitochondrial restriction, but 2-deoxy-glucose affects effector functions. Eur J Immunol (2015) 45(9):2504–16. doi:10.1002/eji.201545473
42. Peng M, Yin N, Chhangawala S, Xu K, Leslie CS, Li MO. Aerobic glycolysis promotes T helper 1 cell differentiation through an epigenetic mechanism. Science (2016) 354(6311):481–4. doi:10.1126/science.aaf6284
43. Chang CH, Curtis JD, Maggi LB Jr, Faubert B, Villarino AV, O’Sullivan D, et al. Posttranscriptional control of T cell effector function by aerobic glycolysis. Cell (2013) 153(6):1239–51. doi:10.1016/j.cell.2013.05.016
44. Tannahill GM, Curtis AM, Adamik J, Palsson-McDermott EM, McGettrick AF, Goel G, et al. Succinate is an inflammatory signal that induces IL-1beta through HIF-1alpha. Nature (2013) 496(7444):238–42. doi:10.1038/nature11986
45. Donnelly RP, Loftus RM, Keating SE, Liou KT, Biron CA, Gardiner CM, et al. mTORC1-dependent metabolic reprogramming is a prerequisite for NK cell effector function. J Immunol (2014) 193(9):4477–84. doi:10.4049/jimmunol.1401558
46. Gubser PM, Bantug GR, Razik L, Fischer M, Dimeloe S, Hoenger G, et al. Rapid effector function of memory CD8+ T cells requires an immediate-early glycolytic switch. Nat Immunol (2013) 14(10):1064–72. doi:10.1038/ni.2687
47. Finlay D, Cantrell DA. Metabolism, migration and memory in cytotoxic T cells. Nat Rev Immunol (2011) 11(2):109–17. doi:10.1038/nri2888
48. Byles V, Covarrubias AJ, Ben-Sahra I, Lamming DW, Sabatini DM, Manning BD, et al. The TSC-mTOR pathway regulates macrophage polarization. Nat Commun (2013) 4:2834. doi:10.1038/ncomms3834
49. Navarro MN, Cantrell DA. Serine-threonine kinases in TCR signaling. Nat Immunol (2014) 15(9):808–14. doi:10.1038/ni.2941
50. Chou C, Pinto AK, Curtis JD, Persaud SP, Cella M, Lin CC, et al. c-Myc-induced transcription factor AP4 is required for host protection mediated by CD8+ T cells. Nat Immunol (2014) 15(9):884–93. doi:10.1038/ni.2943
51. Araki K, Ellebedy AH, Ahmed R. TOR in the immune system. Curr Opin Cell Biol (2011) 23(6):707–15. doi:10.1016/j.ceb.2011.08.006
52. Araki K, Turner AP, Shaffer VO, Gangappa S, Keller SA, Bachmann MF, et al. mTOR regulates memory CD8 T-cell differentiation. Nature (2009) 460(7251):108–12. doi:10.1038/nature08155
53. Pearce EL, Walsh MC, Cejas PJ, Harms GM, Shen H, Wang LS, et al. Enhancing CD8 T-cell memory by modulating fatty acid metabolism. Nature (2009) 460(7251):103–7. doi:10.1038/nature08097
54. Krawczyk CM, Holowka T, Sun J, Blagih J, Amiel E, DeBerardinis RJ, et al. Toll-like receptor-induced changes in glycolytic metabolism regulate dendritic cell activation. Blood (2010) 115(23):4742–9. doi:10.1182/blood-2009-10-249540
55. Freemerman AJ, Johnson AR, Sacks GN, Milner JJ, Kirk EL, Troester MA, et al. Metabolic reprogramming of macrophages: glucose transporter 1 (GLUT1)-mediated glucose metabolism drives a proinflammatory phenotype. J Biol Chem (2014) 289(11):7884–96. doi:10.1074/jbc.M113.522037
56. Palsson-McDermott EM, Curtis AM, Goel G, Lauterbach MA, Sheedy FJ, Gleeson LE, et al. Pyruvate kinase M2 regulates Hif-1alpha activity and IL-1beta induction and is a critical determinant of the Warburg effect in LPS-activated macrophages. Cell Metab (2015) 21(1):65–80. doi:10.1016/j.cmet.2014.12.005
57. Chandel NS, Trzyna WC, McClintock DS, Schumacker PT. Role of oxidants in NF-kappa B activation and TNF-alpha gene transcription induced by hypoxia and endotoxin. J Immunol (2000) 165(2):1013–21. doi:10.4049/jimmunol.165.2.1013
58. Bulua AC, Simon A, Maddipati R, Pelletier M, Park H, Kim KY, et al. Mitochondrial reactive oxygen species promote production of proinflammatory cytokines and are elevated in TNFR1-associated periodic syndrome (TRAPS). J Exp Med (2011) 208(3):519–33. doi:10.1084/jem.20102049
59. West AP, Brodsky IE, Rahner C, Woo DK, Erdjument-Bromage H, Tempst P, et al. TLR signalling augments macrophage bactericidal activity through mitochondrial ROS. Nature (2011) 472(7344):476–80. doi:10.1038/nature09973
60. Jha AK, Huang SC, Sergushichev A, Lampropoulou V, Ivanova Y, Loginicheva E, et al. Network integration of parallel metabolic and transcriptional data reveals metabolic modules that regulate macrophage polarization. Immunity (2015) 42(3):419–30. doi:10.1016/j.immuni.2015.02.005
61. O’Neill LA, Pearce EJ. Immunometabolism governs dendritic cell and macrophage function. J Exp Med (2016) 213(1):15–23. doi:10.1084/jem.20151570
62. O’Neill LA. A broken krebs cycle in macrophages. Immunity (2015) 42(3):393–4. doi:10.1016/j.immuni.2015.02.017
63. Christie DA, Mitsopoulos P, Blagih J, Dunn SD, St-Pierre J, Jones RG, et al. Stomatin-like protein 2 deficiency in T cells is associated with altered mitochondrial respiration and defective CD4+ T cell responses. J Immunol (2012) 189(9):4349–60. doi:10.4049/jimmunol.1103829
64. Dziurla R, Gaber T, Fangradt M, Hahne M, Tripmacher R, Kolar P, et al. Effects of hypoxia and/or lack of glucose on cellular energy metabolism and cytokine production in stimulated human CD4+ T lymphocytes. Immunol Lett (2010) 131(1):97–105. doi:10.1016/j.imlet.2010.02.008
65. Salerno F, Guislain A, Cansever D, Wolkers MC. TLR-mediated innate production of IFN-gamma by CD8+ T cells is independent of glycolysis. J Immunol (2016) 196(9):3695–705. doi:10.4049/jimmunol.1501997
66. Devadas S, Zaritskaya L, Rhee SG, Oberley L, Williams MS. Discrete generation of superoxide and hydrogen peroxide by T cell receptor stimulation: selective regulation of mitogen-activated protein kinase activation and fas ligand expression. J Exp Med (2002) 195(1):59–70. doi:10.1084/jem.20010659
67. Kaminski MM, Sauer SW, Klemke CD, Suss D, Okun JG, Krammer PH, et al. Mitochondrial reactive oxygen species control T cell activation by regulating IL-2 and IL-4 expression: mechanism of ciprofloxacin-mediated immunosuppression. J Immunol (2010) 184(9):4827–41. doi:10.4049/jimmunol.0901662
68. Sena LA, Li S, Jairaman A, Prakriya M, Ezponda T, Hildeman DA, et al. Mitochondria are required for antigen-specific T cell activation through reactive oxygen species signaling. Immunity (2013) 38(2):225–36. doi:10.1016/j.immuni.2012.10.020
69. Beier UH, Angelin A, Akimova T, Wang L, Liu Y, Xiao H, et al. Essential role of mitochondrial energy metabolism in Foxp3(+) T-regulatory cell function and allograft survival. FASEB J (2015) 29(6):2315–26. doi:10.1096/fj.14-268409
70. Lochner M, Berod L, Sparwasser T. Fatty acid metabolism in the regulation of T cell function. Trends Immunol (2015) 36(2):81–91. doi:10.1016/j.it.2014.12.005
71. Michalek RD, Gerriets VA, Jacobs SR, Macintyre AN, MacIver NJ, Mason EF, et al. Cutting edge: distinct glycolytic and lipid oxidative metabolic programs are essential for effector and regulatory CD4+ T cell subsets. J Immunol (2011) 186(6):3299–303. doi:10.4049/jimmunol.1003613
72. Basu S, Hubbard B, Shevach EM. Foxp3-mediated inhibition of Akt inhibits Glut1 (glucose transporter 1) expression in human T regulatory cells. J Leukoc Biol (2015) 97(2):279–83. doi:10.1189/jlb.2AB0514-273RR
73. Gerriets VA, Kishton RJ, Johnson MO, Cohen S, Siska PJ, Nichols AG, et al. Foxp3 and toll-like receptor signaling balance Treg cell anabolic metabolism for suppression. Nat Immunol (2016) 17(12):1459–66. doi:10.1038/ni.3577
74. De Rosa V, Galgani M, Porcellini A, Colamatteo A, Santopaolo M, Zuchegna C, et al. Glycolysis controls the induction of human regulatory T cells by modulating the expression of FOXP3 exon 2 splicing variants. Nat Immunol (2015) 16(11):1174–84. doi:10.1038/ni.3269
75. Buck MD, O’Sullivan D, Klein Geltink RI, Curtis JD, Chang CH, Sanin DE, et al. Mitochondrial dynamics controls T cell fate through metabolic programming. Cell (2016) 166(1):63–76. doi:10.1016/j.cell.2016.05.035
76. Phan AT, Doedens AL, Palazon A, Tyrakis PA, Cheung KP, Johnson RS, et al. Constitutive glycolytic metabolism supports CD8+ T cell effector memory differentiation during viral infection. Immunity (2016) 45(5):1024–37. doi:10.1016/j.immuni.2016.10.017
77. Ardawi MS, Newsholme EA. Glutamine metabolism in lymphocytes of the rat. Biochem J (1983) 212(3):835–42. doi:10.1042/bj2120835
78. Carr EL, Kelman A, Wu GS, Gopaul R, Senkevitch E, Aghvanyan A, et al. Glutamine uptake and metabolism are coordinately regulated by ERK/MAPK during T lymphocyte activation. J Immunol (2010) 185(2):1037–44. doi:10.4049/jimmunol.0903586
79. Spittler A, Winkler S, Gotzinger P, Oehler R, Willheim M, Tempfer C, et al. Influence of glutamine on the phenotype and function of human monocytes. Blood (1995) 86(4):1564–9.
80. He L, Weber KJ, Schilling JD. Glutamine modulates macrophage lipotoxicity. Nutrients (2016) 8(4):215. doi:10.3390/nu8040215
81. Swamy M, Pathak S, Grzes KM, Damerow S, Sinclair LV, van Aalten DM, et al. Glucose and glutamine fuel protein O-GlcNAcylation to control T cell self-renewal and malignancy. Nat Immunol (2016) 17(6):712–20. doi:10.1038/ni.3439
82. Munn DH, Shafizadeh E, Attwood JT, Bondarev I, Pashine A, Mellor AL. Inhibition of T cell proliferation by macrophage tryptophan catabolism. J Exp Med (1999) 189(9):1363–72. doi:10.1084/jem.189.9.1363
83. Srivastava MK, Sinha P, Clements VK, Rodriguez P, Ostrand-Rosenberg S. Myeloid-derived suppressor cells inhibit T-cell activation by depleting cystine and cysteine. Cancer Res (2010) 70(1):68–77. doi:10.1158/0008-5472.CAN-09-2587
84. Van de Velde LA, Subramanian C, Smith AM, Barron L, Qualls JE, Neale G, et al. T cells encountering myeloid cells programmed for amino acid-dependent immunosuppression use Rictor/mTORC2 protein for proliferative checkpoint decisions. J Biol Chem (2017) 292(1):15–30. doi:10.1074/jbc.M116.766238
85. Geiger R, Rieckmann JC, Wolf T, Basso C, Feng Y, Fuhrer T, et al. l-Arginine modulates T cell metabolism and enhances survival and anti-tumor activity. Cell (2016) 167(3):829.e–42.e. doi:10.1016/j.cell.2016.09.031
86. Pages F, Galon J, Dieu-Nosjean MC, Tartour E, Sautes-Fridman C, Fridman WH. Immune infiltration in human tumors: a prognostic factor that should not be ignored. Oncogene (2010) 29(8):1093–102. doi:10.1038/onc.2009.416
87. Whiteside TL. Signaling defects in T lymphocytes of patients with malignancy. Cancer Immunol Immunother (1999) 48(7):346–52. doi:10.1007/s002620050585
88. Radoja S, Frey AB. Cancer-induced defective cytotoxic T lymphocyte effector function: another mechanism how antigenic tumors escape immune-mediated killing. Mol Med (2000) 6(6):465–79.
89. Qian BZ, Pollard JW. Macrophage diversity enhances tumor progression and metastasis. Cell (2010) 141(1):39–51. doi:10.1016/j.cell.2010.03.014
90. Singer K, Kastenberger M, Gottfried E, Hammerschmied CG, Buttner M, Aigner M, et al. Warburg phenotype in renal cell carcinoma: high expression of glucose-transporter 1 (GLUT-1) correlates with low CD8(+) T-cell infiltration in the tumor. Int J Cancer (2011) 128(9):2085–95. doi:10.1002/ijc.25543
91. Chang CH, Qiu J, O’Sullivan D, Buck MD, Noguchi T, Curtis JD, et al. Metabolic competition in the tumor microenvironment is a driver of cancer progression. Cell (2015) 162(6):1229–41. doi:10.1016/j.cell.2015.08.016
92. Ho PC, Bihuniak JD, Macintyre AN, Staron M, Liu X, Amezquita R, et al. Phosphoenolpyruvate is a metabolic checkpoint of anti-tumor T cell responses. Cell (2015) 162(6):1217–28. doi:10.1016/j.cell.2015.08.012
93. Gottfried E, Kunz-Schughart LA, Ebner S, Mueller-Klieser W, Hoves S, Andreesen R, et al. Tumor-derived lactic acid modulates dendritic cell activation and antigen expression. Blood (2006) 107(5):2013–21. doi:10.1182/blood-2005-05-1795
94. Dietl K, Renner K, Dettmer K, Timischl B, Eberhart K, Dorn C, et al. Lactic acid and acidification inhibit TNF secretion and glycolysis of human monocytes. J Immunol (2010) 184(3):1200–9. doi:10.4049/jimmunol.0902584
95. Shime H, Yabu M, Akazawa T, Kodama K, Matsumoto M, Seya T, et al. Tumor-secreted lactic acid promotes IL-23/IL-17 proinflammatory pathway. J Immunol (2008) 180(11):7175–83. doi:10.4049/jimmunol.180.11.7175
96. Colegio OR, Chu NQ, Szabo AL, Chu T, Rhebergen AM, Jairam V, et al. Functional polarization of tumour-associated macrophages by tumour-derived lactic acid. Nature (2014) 513(7519):559–63. doi:10.1038/nature13490
97. Husain Z, Huang Y, Seth P, Sukhatme VP. Tumor-derived lactate modifies antitumor immune response: effect on myeloid-derived suppressor cells and NK cells. J Immunol (2013) 191(3):1486–95. doi:10.4049/jimmunol.1202702
98. Fischer K, Hoffmann P, Voelkl S, Meidenbauer N, Ammer J, Edinger M, et al. Inhibitory effect of tumor cell-derived lactic acid on human T cells. Blood (2007) 109(9):3812–9. doi:10.1182/blood-2006-07-035972
99. Haas R, Smith J, Rocher-Ros V, Nadkarni S, Montero-Melendez T, D’Acquisto F, et al. Lactate regulates metabolic and pro-inflammatory circuits in control of T cell migration and effector functions. PLoS Biol (2015) 13(7):e1002202. doi:10.1371/journal.pbio.1002202
100. Mendler AN, Hu B, Prinz PU, Kreutz M, Gottfried E, Noessner E. Tumor lactic acidosis suppresses CTL function by inhibition of p38 and JNK/c-Jun activation. Int J Cancer (2012) 131(3):633–40. doi:10.1002/ijc.26410
101. Brand A, Singer K, Koehl GE, Kolitzus M, Schoenhammer G, Thiel A, et al. LDHA-associated lactic acid production blunts tumor immunosurveillance by T and NK cells. Cell Metab (2016) 24(5):657–71. doi:10.1016/j.cmet.2016.08.011
102. Brandacher G, Perathoner A, Ladurner R, Schneeberger S, Obrist P, Winkler C, et al. Prognostic value of indoleamine 2,3-dioxygenase expression in colorectal cancer: effect on tumor-infiltrating T cells. Clin Cancer Res (2006) 12(4):1144–51. doi:10.1158/1078-0432.CCR-05-1966
103. Fallarino F, Grohmann U, You S, McGrath BC, Cavener DR, Vacca C, et al. The combined effects of tryptophan starvation and tryptophan catabolites down-regulate T cell receptor zeta-chain and induce a regulatory phenotype in naive T cells. J Immunol (2006) 176(11):6752–61. doi:10.4049/jimmunol.176.11.6752
104. Moretti S, Menicali E, Voce P, Morelli S, Cantarelli S, Sponziello M, et al. Indoleamine 2,3-dioxygenase 1 (IDO1) is up-regulated in thyroid carcinoma and drives the development of an immunosuppressant tumor microenvironment. J Clin Endocrinol Metab (2014) 99(5):E832–40. doi:10.1210/jc.2013-3351
105. Bronte V, Kasic T, Gri G, Gallana K, Borsellino G, Marigo I, et al. Boosting antitumor responses of T lymphocytes infiltrating human prostate cancers. J Exp Med (2005) 201(8):1257–68. doi:10.1084/jem.20042028
106. Choi BS, Martinez-Falero IC, Corset C, Munder M, Modolell M, Muller I, et al. Differential impact of l-arginine deprivation on the activation and effector functions of T cells and macrophages. J Leukoc Biol (2009) 85(2):268–77. doi:10.1189/jlb.0508310
107. Fletcher M, Ramirez ME, Sierra RA, Raber P, Thevenot P, Al-Khami AA, et al. l-Arginine depletion blunts antitumor T-cell responses by inducing myeloid-derived suppressor cells. Cancer Res (2015) 75(2):275–83. doi:10.1158/0008-5472.CAN-14-1491
108. Klysz D, Tai XG, Robert PA, Craveiro M, Cretenet G, Oburoglu L, et al. Glutamine-dependent alpha-ketoglutarate production regulates the balance between T helper 1 cell and regulatory T cell generation. Sci Signal (2015) 8(396):ra97. doi:10.1126/scisignal.aab2610
109. Droge W, Eck HP, Betzler M, Schlag P, Drings P, Ebert W. Plasma glutamate concentration and lymphocyte activity. J Cancer Res Clin Oncol (1988) 114(2):124–8. doi:10.1007/BF00417824
110. Pacheco R, Gallart T, Lluis C, Franco R. Role of glutamate on T-cell mediated immunity. J Neuroimmunol (2007) 185(1–2):9–19. doi:10.1016/j.jneuroim.2007.01.003
111. Huang S, Apasov S, Koshiba M, Sitkovsky M. Role of A2a extracellular adenosine receptor-mediated signaling in adenosine-mediated inhibition of T-cell activation and expansion. Blood (1997) 90(4):1600–10.
112. Hausler SF, Montalban del Barrio I, Strohschein J, Chandran PA, Engel JB, Honig A, et al. Ectonucleotidases CD39 and CD73 on OvCA cells are potent adenosine-generating enzymes responsible for adenosine receptor 2A-dependent suppression of T cell function and NK cell cytotoxicity. Cancer Immunol Immunother (2011) 60(10):1405–18. doi:10.1007/s00262-011-1040-4
113. Ryzhov S, Novitskiy SV, Goldstein AE, Biktasova A, Blackburn MR, Biaggioni I, et al. Adenosinergic regulation of the expansion and immunosuppressive activity of CD11b+Gr1+ cells. J Immunol (2011) 187(11):6120–9. doi:10.4049/jimmunol.1101225
114. Henrich FC, Singer K, Poller K, Bernhardt L, Strobl CD, Limm K, et al. Suppressive effects of tumor cell-derived 5’-deoxy-5’-methylthioadenosine on human T cells. Oncoimmunology (2016) 5(8):e1184802. doi:10.1080/2162402X.2016.1184802
115. Thakur A, Schalk D, Tomaszewski E, Kondadasula SV, Yano H, Sarkar FH, et al. Microenvironment generated during EGFR targeted killing of pancreatic tumor cells by ATC inhibits myeloid-derived suppressor cells through COX2 and PGE2 dependent pathway. J Transl Med (2013) 11:35. doi:10.1186/1479-5876-11-35
116. Gottfried E, Peter K, Kreutz M. The metabolic achilles heel: tumor cell metabolism as therapeutic target. In: Reichle A, editor. The Tumor Microenvironment: From Molecular to Modular Tumor Therapy: Tumors are Reconstructible Communicatively Evolving Systems. Dordrecht, Heidelberg, London, New York: Springer (2010). p. 111–32.
117. Landau BR, Laszlo J, Stengle J, Burk D. Certain metabolic and pharmacologic effects in cancer patients given infusions of 2-deoxy-d-glucose. J Natl Cancer Inst (1958) 21(3):485–94.
118. Maschek G, Savaraj N, Priebe W, Braunschweiger P, Hamilton K, Tidmarsh GF, et al. 2-Deoxy-d-glucose increases the efficacy of adriamycin and paclitaxel in human osteosarcoma and non-small cell lung cancers in vivo. Cancer Res (2004) 64(1):31–4. doi:10.1158/0008-5472.CAN-03-3294
119. Cheong JH, Park ES, Liang J, Dennison JB, Tsavachidou D, Nguyen-Charles C, et al. Dual inhibition of tumor energy pathway by 2-deoxyglucose and metformin is effective against a broad spectrum of preclinical cancer models. Mol Cancer Ther (2011) 10(12):2350–62. doi:10.1158/1535-7163.MCT-11-0497
120. Pelicano H, Martin DS, Xu RH, Huang P. Glycolysis inhibition for anticancer treatment. Oncogene (2006) 25(34):4633–46. doi:10.1038/sj.onc.1209597
121. Eberhart K, Renner K, Ritter I, Kastenberger M, Singer K, Hellerbrand C, et al. Low doses of 2-deoxy-glucose sensitize acute lymphoblastic leukemia cells to glucocorticoid-induced apoptosis. Leukemia (2009) 23(11):2167–70. doi:10.1038/leu.2009.154
122. Hulleman E, Kazemier KM, Holleman A, VanderWeele DJ, Rudin CM, Broekhuis MJ, et al. Inhibition of glycolysis modulates prednisolone resistance in acute lymphoblastic leukemia cells. Blood (2009) 113(9):2014–21. doi:10.1182/blood-2008-05-157842
123. Le A, Cooper CR, Gouw AM, Dinavahi R, Maitra A, Deck LM, et al. Inhibition of lactate dehydrogenase A induces oxidative stress and inhibits tumor progression. Proc Natl Acad Sci U S A (2010) 107(5):2037–42. doi:10.1073/pnas.0914433107
124. Maftouh M, Avan A, Sciarrillo R, Granchi C, Leon LG, Rani R, et al. Synergistic interaction of novel lactate dehydrogenase inhibitors with gemcitabine against pancreatic cancer cells in hypoxia. Br J Cancer (2014) 110(1):172–82. doi:10.1038/bjc.2013.681
125. Stockwin LH, Yu SX, Borgel S, Hancock C, Wolfe TL, Phillips LR, et al. Sodium dichloroacetate selectively targets cells with defects in the mitochondrial ETC. Int J Cancer (2010) 127(11):2510–9. doi:10.1002/ijc.25499
126. Eleftheriadis T, Pissas G, Karioti A, Antoniadi G, Antoniadis N, Liakopoulos V, et al. Dichloroacetate at therapeutic concentration alters glucose metabolism and induces regulatory T-cell differentiation in alloreactive human lymphocytes. J Basic Clin Physiol Pharmacol (2013) 24(4):271–6. doi:10.1515/jbcpp-2013-0001
127. Murray CM, Hutchinson R, Bantick JR, Belfield GP, Benjamin AD, Brazma D, et al. Monocarboxylate transporter MCT1 is a target for immunosuppression. Nat Chem Biol (2005) 1(7):371–6. doi:10.1038/nchembio744
128. Eichner R, Heider M, Fernandez-Saiz V, van Bebber F, Garz AK, Lemeer S, et al. Immunomodulatory drugs disrupt the cereblon-CD147-MCT1 axis to exert antitumor activity and teratogenicity. Nat Med (2016) 22(7):735–43. doi:10.1038/nm.4128
129. Gandhi AK, Shi T, Li M, Jungnelius U, Romano A, Tabernero J, et al. Immunomodulatory effects in a phase II study of lenalidomide combined with cetuximab in refractory KRAS-mutant metastatic colorectal cancer patients. PLoS One (2013) 8(11):e80437. doi:10.1371/journal.pone.0080437
130. Gottfried E, Lang SA, Renner K, Bosserhoff A, Gronwald W, Rehli M, et al. New aspects of an old drug – diclofenac targets MYC and glucose metabolism in tumor cells. PLoS One (2013) 8(7):e66987. doi:10.1371/journal.pone.0066987
131. Sasaki S, Futagi Y, Ideno M, Kobayashi M, Narumi K, Furugen A, et al. Effect of diclofenac on SLC16A3/MCT4 by the Caco-2 cell line. Drug Metab Pharmacokinet (2016) 31(3):218–23. doi:10.1016/j.dmpk.2016.03.004
132. Chirasani SR, Leukel P, Gottfried E, Hochrein J, Stadler K, Neumann B, et al. Diclofenac inhibits lactate formation and efficiently counteracts local immune suppression in a murine glioma model. Int J Cancer (2013) 132(4):843–53. doi:10.1002/ijc.27712
133. Guba M, von Breitenbuch P, Steinbauer M, Koehl G, Flegel S, Hornung M, et al. Rapamycin inhibits primary and metastatic tumor growth by antiangiogenesis: involvement of vascular endothelial growth factor. Nat Med (2002) 8(2):128–35. doi:10.1038/nm0202-128
134. Zhao X, Jiang P, Deng X, Li Z, Tian F, Guo F, et al. Inhibition of mTORC1 signaling sensitizes hepatocellular carcinoma cells to glycolytic stress. Am J Cancer Res (2016) 6(10):2289–98.
135. Battaglia M, Stabilini A, Roncarolo MG. Rapamycin selectively expands CD4+CD25+FoxP3+ regulatory T cells. Blood (2005) 105(12):4743–8. doi:10.1182/blood-2004-10-3932
136. Battaglia M, Stabilini A, Migliavacca B, Horejs-Hoeck J, Kaupper T, Roncarolo MG. Rapamycin promotes expansion of functional CD4+CD25+FOXP3+ regulatory T cells of both healthy subjects and type 1 diabetic patients. J Immunol (2006) 177(12):8338–47. doi:10.4049/jimmunol.177.12.8338
137. Rao RR, Li Q, Odunsi K, Shrikant PA. The mTOR kinase determines effector versus memory CD8+ T cell fate by regulating the expression of transcription factors T-bet and eomesodermin. Immunity (2010) 32(1):67–78. doi:10.1016/j.immuni.2009.10.010
138. Rovira J, Sabet-Baktach M, Eggenhofer E, Lantow M, Koehl GE, Schlitt HJ, et al. A color-coded reporter model to study the effect of immunosuppressants on CD8+ T-cell memory in antitumor and alloimmune responses. Transplantation (2013) 95(1):54–62. doi:10.1097/TP.0b013e318276d358
139. Geissler EK. Post-transplantation malignancies: here today, gone tomorrow? Nat Rev Clin Oncol (2015) 12(12):705–17. doi:10.1038/nrclinonc.2015.186
140. Eikawa S, Nishida M, Mizukami S, Yamazaki C, Nakayama E, Udono H. Immune-mediated antitumor effect by type 2 diabetes drug, metformin. Proc Natl Acad Sci U S A (2015) 112(6):1809–14. doi:10.1073/pnas.1417636112
141. Busse A, Asemissen AM, Nonnenmacher A, Braun F, Ochsenreither S, Stather D, et al. Immunomodulatory effects of sorafenib on peripheral immune effector cells in metastatic renal cell carcinoma. Eur J Cancer (2011) 47(5):690–6. doi:10.1016/j.ejca.2010.11.021
142. Miraki-Moud F, Ghazaly E, Ariza-McNaughton L, Hodby KA, Clear A, Anjos-Afonso F, et al. Arginine deprivation using pegylated arginine deiminase has activity against primary acute myeloid leukemia cells in vivo. Blood (2015) 125(26):4060–8. doi:10.1182/blood-2014-10-608133
143. Veltman JD, Lambers ME, van Nimwegen M, Hendriks RW, Hoogsteden HC, Aerts JG, et al. COX-2 inhibition improves immunotherapy and is associated with decreased numbers of myeloid-derived suppressor cells in mesothelioma. Celecoxib influences MDSC function. BMC Cancer (2010) 10:464. doi:10.1186/1471-2407-10-464
144. Yen MC, Lin CC, Chen YL, Huang SS, Yang HJ, Chang CP, et al. A novel cancer therapy by skin delivery of indoleamine 2,3-dioxygenase siRNA. Clin Cancer Res (2009) 15(2):641–9. doi:10.1158/1078-0432.CCR-08-1988
145. Zheng X, Koropatnick J, Chen D, Velenosi T, Ling H, Zhang X, et al. Silencing IDO in dendritic cells: a novel approach to enhance cancer immunotherapy in a murine breast cancer model. Int J Cancer (2013) 132(4):967–77. doi:10.1002/ijc.27710
146. Platten M, von Knebel Doeberitz N, Oezen I, Wick W, Ochs K. Cancer immunotherapy by targeting IDO1/TDO and their downstream effectors. Front Immunol (2014) 5:673. doi:10.3389/fimmu.2014.00673
147. Allard B, Pommey S, Smyth MJ, Stagg J. Targeting CD73 enhances the antitumor activity of anti-PD-1 and anti-CTLA-4 mAbs. Clin Cancer Res (2013) 19(20):5626–35. doi:10.1158/1078-0432.CCR-13-0545
Keywords: tumor metabolism, immune cell metabolism, immune escape, glycolysis and oxidative phosphorylation, cytokines, immune cell functions
Citation: Renner K, Singer K, Koehl GE, Geissler EK, Peter K, Siska PJ and Kreutz M (2017) Metabolic Hallmarks of Tumor and Immune Cells in the Tumor Microenvironment. Front. Immunol. 8:248. doi: 10.3389/fimmu.2017.00248
Received: 22 December 2016; Accepted: 20 February 2017;
Published: 08 March 2017
Edited by:
Christoph Hess, University of Basel, SwitzerlandReviewed by:
Markus Munder, University of Mainz, GermanyAlfred Zippelius, University of Basel, Switzerland
Copyright: © 2017 Renner, Singer, Koehl, Geissler, Peter, Siska and Kreutz. This is an open-access article distributed under the terms of the Creative Commons Attribution License (CC BY). The use, distribution or reproduction in other forums is permitted, provided the original author(s) or licensor are credited and that the original publication in this journal is cited, in accordance with accepted academic practice. No use, distribution or reproduction is permitted which does not comply with these terms.
*Correspondence: Marina Kreutz, bWFyaW5hLmtyZXV0ekB1a3IuZGU=