- 1Center for Transplantation Sciences, Department of Surgery, Massachusetts General Hospital, Harvard Medical School, Boston, MA, USA
- 2Department of Immunology, Lerner Research Institute, Cleveland Clinic, Cleveland, OH, USA
Memory T cells are characterized by their low activation threshold, robust effector functions, and resistance to conventional immunosuppression and costimulation blockade. Unlike their naïve counterparts, memory T cells reside in and recirculate through peripheral non-lymphoid tissues. Alloreactive memory T cells are subdivided into different categories based on their origins, phenotypes, and functions. Recipients whose immune systems have been directly exposed to allogeneic major histocompatibility complex (MHC) molecules display high affinity alloreactive memory T cells. In the absence of any prior exposure to allogeneic MHC molecules, endogenous alloreactive memory T cells are regularly generated through microbial infections (heterologous immunity). Regardless of their origin, alloreactive memory T cells represent an essential element of the allograft rejection process and a major barrier to tolerance induction in clinical transplantation. This article describes the different subsets of alloreactive memory T cells involved in transplant rejection and examine their generation, functional properties, and mechanisms of action. In addition, we discuss strategies developed to target deleterious allospecific memory T cells in experimental animal models and clinical settings.
Introduction
Rapid and robust protective responses against previously encountered antigens are beneficial during infections, vaccinations, and tumor surveillance. Conversely, memory immune responses against donor antigens are detrimental in the context of transplantation and are commonly associated with poor graft outcome. The danger of preexisting donor-specific alloantibody (DSA) was recognized early in transplant history, and all transplant candidates are tested for the presence of serum DSA prior to transplantation. Despite well documented harmful effects of memory T cells in transplantation (1–4), the potential impact of such cells is mostly neglected while choosing treatment regimens. In this review, we initially outline characteristics of alloreactive memory T cells and their functions. We also describe existing and emerging strategies designed to delete or suppress memory T cells in transplant recipients. To conclude, we discuss future areas of investigation that may translate experimental knowledge of alloreactive memory T cells into clinical practice and thus improve transplant outcome in sensitized recipients.
Basic Biology of Alloreactive Memory T Cells
Origins of Alloreactive Memory T Cells
Laboratory rodents display low frequencies of memory T cells (5–10% of all T cells). In the absence of prior exposure to alloantigens, 1–10% of these memory T cells can react to allogeneic major histocompatibility complex (MHC) molecules in vitro (5). In mice, these cells called endogenous or natural alloreactive memory T cells recognize intact allogeneic MHC molecules through the direct allorecognition pathway (6, 7). It is likely that these memory cells are generated through the recognition of peptides from commensal bacteria or environmental antigens presented by self-MHC, which can mimic complexes formed by allogeneic MHC molecules bound to other peptides (8). Such antigen mimicry, named “heterologous immunity,” is well documented in both humans and experimental animal models. Humans and non-human primates raised in a non-sterile environment are exposed to more infectious and pro-inflammatory agents during their development and thereby likely to develop potent heterologous immunity (9). For instance, following an EBV infection, HLA-B8+ individuals can become sensitized to the allo-MHC molecule HLA-B4402 through antigen mimicry resulting from the presentation of some viral or parasitic peptides (10, 11).
In laboratory mice, direct sensitization with skin allografts or spleen cell immunization is a common approach for generating donor-reactive memory T cells. In humans, transplant patients can be sensitized from exposures to alloantigens such as previous transplants, pregnancies, and blood transfusions. Until now, only memory T cells recognizing intact alloantigens directly have been reported (2, 12). Yet, it is probable that sensitized patients exhibiting high titers of allospecific antibodies display memory T cells recognizing alloantigens indirectly as donor peptides–self-MHC complexes.
Memory T cells can also be generated through homeostatic proliferation in a lymphopenic environment, including potentially alloreactive and pathogenic T cells (13–15). Such homeostatically expanded memory T cells can impair tolerance induction to allografts (15–17).
The accumulation of alloreactive memory T cells may be influenced by the end stage organ disease or treatment common in transplant candidates. For example, prolonged exposure to dialysis increases the risk of developing alloreactive memory T cells (18). In addition, Sawinski et al. reported that low serum levels of 25-OH-vitamin D in dialysis patients correlates with the frequency of alloreactive memory T cells independent of age, gender, previous transplants, or time on dialysis (19).
Location of Memory T Cells
Memory T cells have been traditionally divided into two major subsets with largely overlapping functions but distinct trafficking patterns (Figure 1). Central memory T cells (Tcm) express lymphoid homing markers CCR7 and CD62L, whereas effector memory T cells (Tem) are CCR7−CD62L− but instead express molecules that promote migration into peripheral tissues (20–23). In humans, but not in mice, some memory T cells [terminally differentiated effector memory T cells (Temra)] re-express naive T cell surface marker CD45RA, while downregulating expression of CCR7, CD62L, and CD28, and represent a terminal stage of effector differentiation (21, 24, 25). Recent studies demonstrated that some T cells in peripheral tissues do not circulate and represent a distinct subset of tissue-resident memory T cells (Trm) (24, 26–28). Trm cells express early activation marker CD69 and αEβ7 integrin CD103 along with a number of tissue-specific chemokine receptors (26, 29–32). There is accumulating evidence that Trm cells play an important role in host protection against infections. It is conceivable that Trm cells of both donor and recipient origins may influence transplant outcome by facilitating GVHD or allograft rejection, respectively. However, the proportion of alloreactive T cells among Trm subset and the potential contribution of such cells following transplantation remain to be addressed. Another important type of memory T cells relevant to transplantation is CD4+CXCR5hi follicular helper (Tfh) cells that reside in B cell follicles within secondary lymphoid organs and are essential for optimal B cell responses and antibody generation (33). As memory T cells in secondary lymphoid and non-lymphoid peripheral tissues are spared by antibody-mediated lymphoablation (34) Trm cells may be harder to control compared to circulating memory T cells.
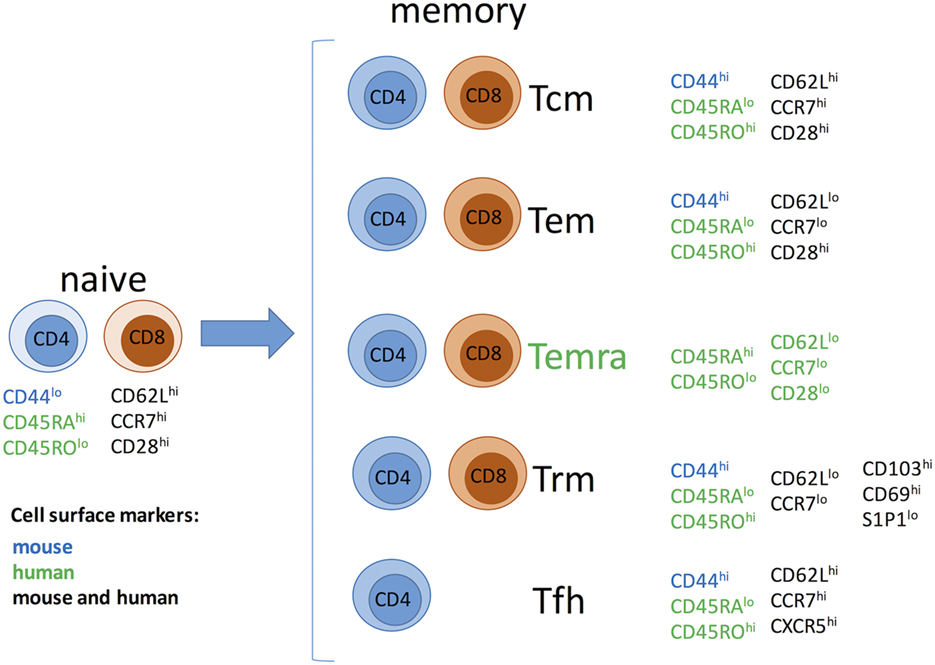
Figure 1. Memory T cell subsets. Abbreviations: Tcm, central memory T cells; Tem, effector memory T cells; Temra, terminally differentiated effector memory T cells; Trm, resident memory T cells; Tfh, follicular helper memory T cells.
Low Activation Threshold and Resistance to Conventional Costimulatory Blockade
In the process of memory T cell differentiation, the T cell receptor and costimulatory signaling cascades are adjusted to ensure rapid activation of high magnitude upon antigen reencounter (35, 36). This results in the ability of memory T cells to respond to lower antigen doses with limited costimulation, i.e., to antigen presented by non-professional antigen-presenting cells (36–38). While this process is essential for host defense, it renders alloreactive memory T cells more dangerous in transplant settings. Numerous studies in animal models have demonstrated that donor-reactive memory T cells can induce allograft rejection despite interruption of essential costimulatory pathways, CD28/CD80/CD86 and CD40/CD154 (11, 15, 39–43).
Contribution of Memory T Cells to Allograft Rejection and Tolerance
Role in Allograft Rejection
During the past decade, studies investigating CD4+ versus CD8+ memory T cells revealed that these subsets contribute to allograft rejection through distinct mechanisms. Indeed, memory CD4+ T cells not only become effector cells upon reactivation, but also provide help for the robust activation of donor-reactive effector CD8+ T cells (40). These effector CD8+ T cells then are the main driving force behind allograft rejection facilitated by memory CD4+ T cells in heart-transplanted mice, and CD8+ T cell depletion or limiting their trafficking into the graft significantly extends allograft survival (40, 44).
While de novo responses by naïve T cells can be efficiently controlled by current immunosuppression, memory CD4+ T cells are resistant to these therapies and can provide help for the generation of DSA leading to alloantibody-mediated graft injury (40, 44). Recent studies in a mouse model of heart transplantation identified potential therapeutic targets to control CD40-independent DSA generation by memory CD4+ T cells. First, gamma interferon (IFNγ) secretion by memory helper T cells is required for de novo DSA generation (45). Second, CD40-independent helper functions of donor-reactive memory CD4+ T cells and heart allograft rejection were markedly inhibited by neutralizing B cell activating factor and a proliferation-inducing ligand, cytokines critical for B cell survival, activation, and differentiation (46).
The fate and functions of donor-reactive memory CD8+ T cells following transplantation are equally fascinating. Early direct contact of circulating memory CD8+ T cells with donor endothelium upregulates the expression of adhesion molecules and chemokines thus facilitating infiltration of recipient leukocytes into the graft (47, 48). A proportion of endogenous memory CD8+ T cells react to donor MHC class I molecules and can infiltrate cardiac allografts within hours after reperfusion. Once in the graft parenchyma, these memory CD8+ T cells proliferate extensively, upregulate the expression of ICOS, and secrete IFNγ in ICOS-dependent manner (49, 50). Although this early expression of effector functions was found to be insufficient to mediate allograft rejection (51), the potential danger of endogenous memory CD8+ T cells should not be underestimated. The approximation of clinical situation by increasing graft cold ischemia storage time enhanced effector functions of endogenous memory CD8+ T cells enabling them to promptly reject a cardiac allograft despite costimulatory blockade with CTLA4-Ig (52).
Influence of Memory T Cells on Allograft Tolerance
In laboratory rodents, endogenous memory T cells generated through heterologous immunity have little ability to prevent tolerance induction given that hematopoietic chimerism and/or costimulation blockade regularly achieve tolerance of fully allogeneic transplants (53–55). In contrast, mice that have been sensitized to allogeneic MHC through transplantation or multiple viral infections become resistant to tolerance induction (11, 39, 56, 57). Moreover, naïve mice adoptively transferred with alloreactive memory T cells display similar resistance to tolerogenesis via hematopoietic chimerism or costimulation blockade (11, 39, 56, 57). Therefore, in laboratory rodents, antigen-induced rather than endogenous memory T cells prevent transplant tolerance. It is still unclear whether this difference relies on the low frequency of endogenous memory T cells or on the fact that these two subsets of memory T cells are different in nature.
The presence of memory T cells has been often correlated with poor outcomes in clinical transplantation. In humans, the presence of memory T cells pretransplantation has been associated with an increased risk for acute rejection of kidney transplants (2). However, while EBV- and CMV-specific memory T cells displaying alloreactivity have been detected in human transplant recipients, so far there is no indication that the presence of “heterologous immunity” in transplant recipients correlates with worse graft outcomes (10, 58–60).
Our laboratory showed that a sizable proportion of endogenous memory T cells found in peripheral blood, and secondary lymphoid organs of naïve cynomolgus monkeys are allospecific. Most Tem were CD8+CD95+CD28− IFNγ-producing cells located in the spleen, peripheral blood, and bone marrow while IL-2-producing Tcm were primarily CD4+CD95+CD28+ and limited to the lymph nodes and spleen (12). Based upon this observation, we studied the influence of pretransplant memory T cell alloreactivity on rejection versus tolerance of kidney allografts in monkeys (61). A series of cynomolgus monkeys were conditioned [whole body and thymic irradiations + horse antithymocyte globulin (ATG) treatment] and received a combined kidney and bone marrow transplantation from the same allogeneic donor (62). The animals then received a short-term immunosuppression treatment comprised of anti-CD40L antibodies and cyclosporine A (62). This procedure resulted in a transient multilineage hematopoietic chimerism and achieved long-term survival of kidney allografts (>1 year) after withdrawal of immunosuppression in 70% of the monkeys (62). On the other hand, approximately 30% of the treated monkeys rejected their allograft in an acute fashion within 100–200 days posttransplantation (61). In this model, we observed that the vast majority of tolerant animals displayed low frequencies of donor-reactive memory T cells (61). It is noteworthy that no differences between homeostatic expansion of memory T cells were observed between monkeys which rejected or accepted kidney allografts (61).
Even though memory T cells are generally viewed as pathogenic in the context of transplantation, under certain circumstances, they demonstrate regulatory capacity and suppress deleterious pro-inflammatory immune responses. Krupnick et al. have reported that early infiltration of central memory CD8+ T cells is essential for lung allograft acceptance after treatment with CTLA4-Ig and anti-CD154 mAbs (63). Similarly, CD8+CD45RClo cells with regulatory properties have been described in rat models of solid organ transplantation and GVHD (64, 65). These findings raise a concern that lymphoablative approaches targeting memory T cells may interfere with allograft acceptance of certain types of transplants.
Recent Developments in Targeting Alloreactive T Cell Memory
Lymphoablation
Induction therapy is widely used in clinical transplantation to overcome the deleterious effects of preexisting donor-reactive immunity. Antibody-mediated lymphocyte depletion is most commonly used induction strategy, particularly in highly sensitized patients and in patients receiving marginal grafts (66–69). Although memory T cells are the primary targets of induction therapies, they are less susceptible to depletion than naïve T cells (70–73). T cells with an effector/memory phenotype are detectable after anti-CD52 mAb or ATG induction and are associated with acute rejection episodes in non-human primates and human transplant recipients (74, 75). In rodents, preexisting memory T cells rapidly recover following lymphocyte depletion with ATG and dominate anti-donor immune responses. The efficiency of memory CD4+ T cell depletion is generally lower than that of CD8+ T cells (34, 76–79). Additional depletion of residual CD4+ T cells severely impairs the recovery of memory CD8+ T cells after ATG treatment (80). Limiting CD4+ T helper signals during lymphoablation increases the efficacy of mATG in controlling memory T cell expansion and significantly extends heart allograft survival in sensitized recipients (80). These findings are consistent with previous observations describing a synergistic effect between ATG lymphoablation and costimulatory blockade (81, 82).
Alefacept, a fusion protein combining extracellular domain of LFA-3 with constant regions of human IgG1 (83–85). LFA-3 is a ligand for CD2, a molecule that is predominantly detected on human T and NK cells. As CD2 expression is upregulated on CD45RO+ effector/memory T cells, alefacept selectively depletes this subset and spares other T cell populations (86–88). Alefacept is currently being used in clinic for the treatment of severe psoriasis (89, 90) and is showing promise for targeting alloreactive effector/memory T cells in solid organ and bone marrow transplantation (91–95). Most importantly, pretransplant alefacept therapy synergizes with CTLA4-Ig presumably by targeting costimulatory blockade-resistant CD8+CD2hiCD28− effector/memory T cells (91).
In addition to direct lymphoablation, manipulating T cell survival and homeostasis by regulating cell metabolic pathways may be a promising therapeutic strategy in transplantation. Recent studies suggest that immune cells subsets use different mechanisms of energy generation, and this information can be exploited to selectively target undesirable memory T cells [reviewed in Ref. (96)].
Costimulatory Blockade
Belatacept, a second generation of CTLA4-Ig, is currently used in clinical transplantation to prevent allograft rejection and minimize the toxic side effects of calcineurin inhibitors (97). Despite reduced side effects and improved graft survival, belatacept-treated patients have higher rates of acute cellular rejection compared to CNI treatment (98, 99). As memory T cells are more resistant to the effects of CTLA4-Ig in animal transplantation models, it is possible that presensitized T cells could account for some belatacept-resistant rejection episodes. Indeed, terminally differentiated memory CD4+ and CD8+ T cells in humans (Temra) lose CD28 expression and become insensitive to the lack of CD28/B7 costimulation (100–104). Not surprisingly, increased numbers of both CD4+ and CD8+ CD28− memory T cells are associated with a poor outcome in renal and lung transplant patients (105–108). A recent report by Espinosa et al. identified yet another population of CD57+CD4+ T cells as potential mediators of belatacept-resistant renal allograft rejection. These cells are more common in patients with kidney failure, express high levels of adhesion molecules CD2, LFA-1, and VLA-4, downregulate CD28, and produce IFNγ, tumor necrosis factor alpha (TNFα), and granzyme B consistent with effector/memory phenotype (109).
Recent reports suggest that the pedigree of alloreactive memory T cells in a given recipient may have important practical implications. Using three different pathogens to generate donor-reactive memory T cells in a mouse model of skin transplantation, Badell et al. demonstrated that the sensitivity of memory T cells to immunosuppression is dependent on their origin (110). In this study, Tcm with a less differentiated phenotype were most sensitive to the effects of costimulatory blockade. Consistent with these findings, in vitro comparison of CMV- and alloreactive T cells suggested that virus-specific fully differentiated T cells secreting IFNγ, TNFα, and IL-2 simultaneously are more resistant to the effects of CTLA4-Ig, whereas tacrolimus inhibits responses by both allo- and virus-specific T cells (111).
In addition to blocking CD28/B7 costimulation, CTLA4-Ig also prevents signaling through CTLA-4, which can have negative effects on generation and functions of regulatory T cells (Tregs) (112–117). To circumvent this problem, several antagonistic anti-CD28 mAbs and Ab F(ab′)2 fragments have been generated and showed promise in animal transplantation models (118–121). The selective effects of these reagents on memory T cell subsets and the potential pathogenicity of CD28lo Temra cells during such therapies remain to be determined. Attempts to target another major costimulatory pathway, CD40/CD154, encountered early difficulties because of thromboembolic effects of anti-CD154 (CD40L) blocking antibodies (122). To avoid cross-linking CD154 that is highly expressed on platelets, an alternative approach has been the generation of non-activating anti-CD40 antibodies. Several such reagents have been successfully tested in non-human primate recipients of renal and islet allografts (123–128).
In addition to CD28/B7 and CD40/CD154 costimulation, several other costimulatory pathways may play a role in effector/memory T cell functions. Inhibition or genetic lack of ICOS/B7RP-1, CD134/CD134L, CD70/CD27, or CD137/CD137L improved allograft survival even in donor-sensitized recipients, or after delayed administration which allowed initial priming of donor-reactive T cells [reviewed in Ref. (129)]. It was revealed that these costimulatory pathways might control distinct aspects of the alloimmune response. For example, blocking anti-CD134L mAb inhibits proliferation of effector T cells while supporting the survival of Tregs (71, 130). Conversely, signaling through CD134 inhibits immunosuppressive properties of FoxP3+ Tregs and promotes allograft rejection (131, 132). ICOS/B7RP-1 blockade of resting memory CD4+ T cells inhibits their helper functions and decreases alloantibody production. In contrast, circulating memory CD8+ T cells are ICOSlo, but rapidly upregulate ICOS surface expression upon graft infiltration. These examples demonstrate that the complexity of costimulatory pathways governing alloimmune responses must be considered when costimulatory blockade is used as part of immunosuppression regimen.
Limiting Trafficking of Alloreactive Memory T Cells
While preventing memory T cell entrance into graft tissue should improve transplant outcome, the attempts to neutralize chemokines or chemokine receptors such as CCR5 or CXCR3 did not live up to the initial expectations, most likely due to the redundancy of chemokine/receptor network. On other hand, reagents blocking LFA-1 (leukocyte function-associated antigen-1, an αLβ2 integrin) and VLA-4 (very late antigen-4, an α4β1 integrin) have been demonstrated to prolong allograft survival in experimental transplantation [reviewed in Ref. (133)]. Treatment with either anti-LFA-1 or anti-VLA-4 blocking mAbs prolonged skin allograft survival in a mouse model of costimulatory blockade-resistant rejection by memory CD8+ T cells (134). In another study, pretransplant treatment with anti-LFA-1 mAbs inhibited early infiltration of endogenous donor-reactive memory CD8+ T cells into cardiac allografts, and significantly prolonged allograft survival (135). These findings suggest that a short course of integrin blockade may be instrumental in controlling T cell memory while avoiding side effects of long-term treatments.
Concluding Remarks
While other types of immunologic memory lymphocytes such as memory B cells, preexisting alloantibodies, and “innate memory” described for NK cells and macrophages can impact transplant outcomes, in this review, we focused exclusively on T cell memory. It is now firmly established that alloreactive memory T cells accelerate allograft rejection and prevent transplant tolerance. However, the implementation of accumulated experimental knowledge in clinical transplantation is impeded by several factors. First, the diagnostics of T cell allosensitization in transplant candidates is problematic. Due to heterogeneity in phenotype and functions of memory T cells, complementary tests will be required including analyses of cytokine producing, cytotoxic, and follicular helper T cells. The resulting information is likely to be complex and hard to use in clinical decision-making. Second, memory T cells in humans are sampled only in peripheral blood. So far, there is no information on pathogenicity of tissue-resident alloreactive memory T cells. Third, memory T cell susceptibility to immunosuppression may depend on their origins. As immunological histories of individuals are difficult to trace, the situation may arise when patients with similar T cell memory profile require distinct treatment strategies. Finally, despite rapidly accumulating data on alloreactive T cell memory, the discrepancies between animal models and transplantation in human patients are profound. Ideally, animal transplantation models approximating clinical situation should take into account frequencies of total and donor-reactive memory T cells in different species, time of graft cold ischemia storage, and the presence of DSA in recipient serum. Including these considerations into experimental design will facilitate the development of novel approaches to control memory T cells and improve transplant survival in sensitized recipients.
Author Contributions
GB, AV, BG, JM, and KA wrote portions of the manuscript; GB and AV edited the manuscript and prepared it for submission.
Conflict of Interest Statement
The authors declare that the research was conducted in the absence of any commercial or financial relationships that could be construed as a potential conflict of interest.
Acknowledgments
This study was supported by NIH grants R21AI111191 and R21AI117466 to GB and R01AI113142 and 2PO1 AI087586-06 to AV.
Abbreviations
MHC, major histocompatibility complex; APC, antigen-presenting cell; Treg, regulatory T cell; IFNγ, gamma interferon; TNFα, tumor necrosis factor alpha; CTL, cytotoxic T lymphocyte; DC, dendritic cell; DSAs, donor-specific antibodies.
References
1. Augustine JJ, Siu DS, Clemente MJ, Schulak JA, Heeger PS, Hricik DE. Pre-transplant IFN-gamma ELISPOTs are associated with post-transplant renal function in African American renal transplant recipients. Am J Transplant (2005) 5(8):1971–5. doi: 10.1111/j.1600-6143.2005.00958.x
2. Heeger PS, Greenspan NS, Kuhlenschmidt S, Dejelo C, Hricik DE, Schulak JA, et al. Pretransplant frequency of donor-specific, IFN-gamma-producing lymphocytes is a manifestation of immunologic memory and correlates with the risk of posttransplant rejection episodes. J Immunol (1999) 163(4):2267–75.
3. Hricik DE, Rodriguez V, Riley J, Bryan K, Tary-Lehmann M, Greenspan N, et al. Enzyme linked immunosorbent spot (ELISPOT) assay for interferon-gamma independently predicts renal function in kidney transplant recipients. Am J Transplant (2003) 3(7):878–84. doi:10.1034/j.1600-6143.2003.00132.x
4. Poggio ED, Augustine JJ, Clemente M, Danzig JM, Volokh N, Zand MS, et al. Pretransplant cellular alloimmunity as assessed by a panel of reactive T cells assay correlates with acute renal graft rejection. Transplantation (2007) 83(7):847–52. doi:10.1097/01.tp.0000258730.75137.39
5. Lombardi G, Sidhu S, Daly M, Batchelor JR, Makgoba W, Lechler RI. Are primary alloresponses truly primary? Int Immunol (1990) 2(1):9–13.
6. Benichou G, Valujskikh A, Heeger PS. Contributions of direct and indirect T cell alloreactivity during allograft rejection in mice. J Immunol (1999) 162(1):352–8.
7. Benichou G. Direct and indirect antigen recognition: the pathways to allograft immune rejection. Front Biosci (1999) 4:D476–80.
8. Lechler R, Lombardi G. Structural aspects of allorecognition. Curr Opin Immunol (1991) 3(5):715–21.
9. Mbitikon-Kobo FM, Vocanson M, Michallet MC, Tomkowiak M, Cottalorda A, Angelov GS, et al. Characterization of a CD44/CD122int memory CD8 T cell subset generated under sterile inflammatory conditions. J Immunol (2009) 182(6):3846–54. doi:10.4049/jimmunol.0802438
10. Amir AL, D’Orsogna LJ, Roelen DL, van Loenen MM, Hagedoorn RS, de Boer R, et al. Allo-HLA reactivity of virus-specific memory T cells is common. Blood (2010) 115(15):3146–57. doi:10.1182/blood-2009-07-234906
11. Pantenburg B, Heinzel F, Das L, Heeger PS, Valujskikh A. T cells primed by Leishmania major infection cross-react with alloantigens and alter the course of allograft rejection. J Immunol (2002) 169(7):3686–93. doi:10.4049/jimmunol.169.7.3686
12. Nadazdin O, Boskovic S, Murakami T, O’Connor DH, Wiseman RW, Karl JA, et al. Phenotype, distribution and alloreactive properties of memory T cells from cynomolgus monkeys. Am J Transplant (2010) 10(6):1375–84. doi:10.1111/j.1600-6143.2010.03119.x
13. Cho BK, Rao VP, Ge Q, Eisen HN, Chen J. Homeostasis-stimulated proliferation drives naive T cells to differentiate directly into memory T cells. J Exp Med (2000) 192(4):549–56. doi:10.1084/jem.192.4.549
14. Tchao NK, Turka LA. Lymphodepletion and homeostatic proliferation: implications for transplantation. Am J Transplant (2012) 12(5):1079–90. doi:10.1111/j.1600-6143.2012.04008.x
15. Wu Z, Bensinger SJ, Zhang J, Chen C, Yuan X, Huang X, et al. Homeostatic proliferation is a barrier to transplantation tolerance. Nat Med (2004) 10(1):87–92. doi:10.1038/nm965
16. Taylor DK, Neujahr D, Turka LA. Heterologous immunity and homeostatic proliferation as barriers to tolerance. Curr Opin Immunol (2004) 16(5):558–64. doi:10.1016/j.coi.2004.07.007
17. Moxham VF, Karegli J, Phillips RE, Brown KL, Tapmeier TT, Hangartner R, et al. Homeostatic proliferation of lymphocytes results in augmented memory-like function and accelerated allograft rejection. J Immunol (2008) 180(6):3910–8. doi:10.4049/jimmunol.180.6.3910
18. Augustine JJ, Poggio ED, Clemente M, Aeder MI, Bodziak KA, Schulak JA, et al. Hemodialysis vintage, black ethnicity, and pretransplantation antidonor cellular immunity in kidney transplant recipients. J Am Soc Nephrol (2007) 18(5):1602–6. doi:10.1681/ASN.2006101105
19. Sawinski D, Uribarri J, Peace D, Yao T, Wauhop P, Trzcinka P, et al. 25-OH-vitamin D deficiency and cellular alloimmunity as measured by panel of reactive T cell testing in dialysis patients. Am J Transplant (2010) 10(10):2287–95. doi:10.1111/j.1600-6143.2010.03264.x
20. Ahmadzadeh M, Hussain SF, Farber DL. Heterogeneity of the memory CD4 T cell response: persisting effectors and resting memory T cells. J Immunol (2001) 166(2):926–35. doi:10.4049/jimmunol.166.2.926
21. Sallusto F, Geginat J, Lanzavecchia A. Central memory and effector memory T cell subsets: function, generation, and maintenance. Annu Rev Immunol (2004) 22:745–63. doi:10.1146/annurev.immunol.22.012703.104702
22. Sallusto F, Lenig D, Forster R, Lipp M, Lanzavecchia A. Two subsets of memory T lymphocytes with distinct homing potentials and effector functions. Nature (1999) 401(6754):708–12.
23. Wherry EJ, Teichgraber V, Becker TC, Masopust D, Kaech SM, Antia R, et al. Lineage relationship and protective immunity of memory CD8 T cell subsets. Nat Immunol (2003) 4(3):225–34. doi:10.1038/ni889
24. Sathaliyawala T, Kubota M, Yudanin N, Turner D, Camp P, Thome JJ, et al. Distribution and compartmentalization of human circulating and tissue-resident memory T cell subsets. Immunity (2013) 38(1):187–97. doi:10.1016/j.immuni.2012.09.020
25. Surh CD, Sprent J. Homeostasis of naive and memory T cells. Immunity (2008) 29(6):848–62. doi:10.1016/j.immuni.2008.11.002
26. Casey KA, Fraser KA, Schenkel JM, Moran A, Abt MC, Beura LK, et al. Antigen-independent differentiation and maintenance of effector-like resident memory T cells in tissues. J Immunol (2012) 188(10):4866–75. doi:10.4049/jimmunol.1200402
27. Gebhardt T, Wakim LM, Eidsmo L, Reading PC, Heath WR, Carbone FR. Memory T cells in nonlymphoid tissue that provide enhanced local immunity during infection with herpes simplex virus. Nat Immunol (2009) 10(5):524–30. doi:10.1038/ni.1718
28. Masopust D, Choo D, Vezys V, Wherry EJ, Duraiswamy J, Akondy R, et al. Dynamic T cell migration program provides resident memory within intestinal epithelium. J Exp Med (2010) 207(3):553–64. doi:10.1084/jem.20090858
29. Mackay LK, Rahimpour A, Ma JZ, Collins N, Stock AT, Hafon ML, et al. The developmental pathway for CD103(+)CD8+ tissue-resident memory T cells of skin. Nat Immunol (2013) 14(12):1294–301. doi:10.1038/ni.2744
30. Steinert EM, Schenkel JM, Fraser KA, Beura LK, Manlove LS, Igyarto BZ, et al. Quantifying memory CD8 T cells reveals regionalization of immunosurveillance. Cell (2015) 161(4):737–49. doi:10.1016/j.cell.2015.03.031
31. Turner DL, Gordon CL, Farber DL. Tissue-resident T cells, in situ immunity and transplantation. Immunol Rev (2014) 258(1):150–66. doi:10.1111/imr.12149
32. Watanabe R, Gehad A, Yang C, Scott LL, Teague JE, Schlapbach C, et al. Human skin is protected by four functionally and phenotypically discrete populations of resident and recirculating memory T cells. Sci Transl Med (2015) 7(279):279ra39. doi:10.1126/scitranslmed.3010302
33. Hale JS, Ahmed R. Memory T follicular helper CD4 T cells. Front Immunol (2015) 6:16. doi:10.3389/fimmu.2015.00016
34. Ayasoufi K, Yu H, Fan R, Wang X, Williams J, Valujskikh A. Pretransplant antithymocyte globulin has increased efficacy in controlling donor-reactive memory T cells in mice. Am J Transplant (2013) 13(3):589–99. doi:10.1111/ajt.12068
35. Chandok MR, Farber DL. Signaling control of memory T cell generation and function. Semin Immunol (2004) 16(5):285–93. doi:10.1016/j.smim.2004.08.009
36. Jameson SC, Masopust D. Diversity in T cell memory: an embarrassment of riches. Immunity (2009) 31(6):859–71. doi:10.1016/j.immuni.2009.11.007
37. Croft M, Bradley LM, Swain SL. Naive versus memory CD4 T cell response to antigen. Memory cells are less dependent on accessory cell costimulation and can respond to many antigen-presenting cell types including resting B cells. J Immunol (1994) 152(6):2675–85.
38. Khayyamian S, Hutloff A, Buchner K, Grafe M, Henn V, Kroczek RA, et al. ICOS-ligand, expressed on human endothelial cells, costimulates Th1 and Th2 cytokine secretion by memory CD4+ T cells. Proc Natl Acad Sci U S A (2002) 99(9):6198–203. doi:10.1073/pnas.092576699
39. Adams AB, Williams MA, Jones TR, Shirasugi N, Durham MM, Kaech SM, et al. Heterologous immunity provides a potent barrier to transplantation tolerance. J Clin Invest (2003) 111(12):1887–95. doi:10.1172/JCI17477
40. Chen Y, Heeger PS, Valujskikh A. In vivo helper functions of alloreactive memory CD4+ T cells remain intact despite donor-specific transfusion and anti-CD40 ligand therapy. J Immunol (2004) 172(9):5456–66. doi:10.4049/jimmunol.172.9.5456
41. Valujskikh A, Pantenburg B, Heeger PS. Primed allospecific T cells prevent the effects of costimulatory blockade on prolonged cardiac allograft survival in mice. Am J Transplant (2002) 2(6):501–9. doi:10.1034/j.1600-6143.2002.20603.x
42. Welsh RM, Markees TG, Woda BA, Daniels KA, Brehm MA, Mordes JP, et al. Virus-induced abrogation of transplantation tolerance induced by donor-specific transfusion and anti-CD154 antibody. J Virol (2000) 74(5):2210–8. doi:10.1128/JVI.74.5.2210-2218.2000
43. Zhai Y, Meng L, Gao F, Busuttil RW, Kupiec-Weglinski JW. Allograft rejection by primed/memory CD8+ T cells is CD154 blockade resistant: therapeutic implications for sensitized transplant recipients. J Immunol (2002) 169(8):4667–73. doi:10.4049/jimmunol.169.8.4667
44. Zhang Q, Chen Y, Fairchild RL, Heeger PS, Valujskikh A. Lymphoid sequestration of alloreactive memory CD4 T cells promotes cardiac allograft survival. J Immunol (2006) 176(2):770–7. doi:10.4049/jimmunol.176.2.770
45. Gorbacheva V, Fan R, Wang X, Baldwin WM III, Fairchild RL, Valujskikh A. IFN-gamma production by memory helper T cells is required for CD40-independent alloantibody responses. J Immunol (2015) 194(3):1347–56. doi:10.4049/jimmunol.1401573
46. Gorbacheva V, Ayasoufi K, Fan R, Baldwin WM III, Valujskikh A. B cell activating factor (BAFF) and a proliferation inducing ligand (APRIL) mediate CD40-independent help by memory CD4 T cells. Am J Transplant (2015) 15(2):346–57. doi:10.1111/ajt.12984
47. El-Sawy T, Belperio JA, Strieter RM, Remick DG, Fairchild RL. Inhibition of polymorphonuclear leukocyte-mediated graft damage synergizes with short-term costimulatory blockade to prevent cardiac allograft rejection. Circulation (2005) 112(3):320–31. doi:10.1161/CIRCULATIONAHA.104.516708
48. El-Sawy T, Miura M, Fairchild R. Early T cell response to allografts occurring prior to alloantigen priming up-regulates innate-mediated inflammation and graft necrosis. Am J Pathol (2004) 165(1):147–57. doi:10.1016/S0002-9440(10)63283-X
49. Schenk AD, Gorbacheva V, Rabant M, Fairchild RL, Valujskikh A. Effector functions of donor-reactive CD8 memory T cells are dependent on ICOS induced during division in cardiac grafts. Am J Transplant (2009) 9(1):64–73. doi:10.1111/j.1600-6143.2008.02460.x
50. Schenk AD, Nozaki T, Rabant M, Valujskikh A, Fairchild RL. Donor-reactive CD8 memory T cells infiltrate cardiac allografts within 24-h posttransplant in naive recipients. Am J Transplant (2008) 8(8):1652–61. doi:10.1111/j.1600-6143.2008.02302.x
51. Setoguchi K, Hattori Y, Iida S, Baldwin WM III, Fairchild RL. Endogenous memory CD8 T cells are activated within cardiac allografts without mediating rejection. Am J Transplant (2013) 13(9):2293–307. doi:10.1111/ajt.12372
52. Su CA, Iida S, Abe T, Fairchild RL. Endogenous memory CD8 T cells directly mediate cardiac allograft rejection. Am J Transplant (2014) 14(3):568–79. doi:10.1111/ajt.12605
53. Wood KJ, Sakaguchi S. Regulatory T cells in transplantation tolerance. Nat Rev Immunol (2003) 3(3):199–210. doi:10.1038/nri1027
54. Kurtz J, Shaffer J, Lie A, Anosova N, Benichou G, Sykes M. Mechanisms of early peripheral CD4 T-cell tolerance induction by anti-CD154 monoclonal antibody and allogeneic bone marrow transplantation: evidence for anergy and deletion but not regulatory cells. Blood (2004) 103(11):4336–43. doi:10.1182/blood-2003-08-2642
55. Larsen CP, Elwood ET, Alexander DZ, Ritchie SC, Hendrix R, Tucker-Burden C, et al. Long-term acceptance of skin and cardiac allografts after blocking CD40 and CD28 pathways. Nature (1996) 381(6581):434–8.
56. Brehm MA, Markees TG, Daniels KA, Greiner DL, Rossini AA, Welsh RM. Direct visualization of cross-reactive effector and memory allo-specific CD8 T cells generated in response to viral infections. J Immunol (2003) 170(8):4077–86. doi:10.4049/jimmunol.170.8.4077
57. Macdonald WA, Chen Z, Gras S, Archbold JK, Tynan FE, Clements CS, et al. T cell allorecognition via molecular mimicry. Immunity (2009) 31(6):897–908. doi:10.1016/j.immuni.2009.09.025
58. Burrows SR, Silins SL, Khanna R, Burrows JM, Rischmueller M, McCluskey J, et al. Cross-reactive memory T cells for Epstein-Barr virus augment the alloresponse to common human leukocyte antigens: degenerate recognition of major histocompatibility complex-bound peptide by T cells and its role in alloreactivity. Eur J Immunol (1997) 27(7):1726–36.
59. Heutinck KM, Yong S, Tonneijck L, van den Heuvel H, van der Weerd NC, van der Pant KA, et al. Virus-specific CD8 T-cells cross-reactive to donor-alloantigen are transiently present in the circulation of kidney transplant recipients infected with CMV and/or EBV. Am J Transplant (2016) 16(5):1480–91. doi:10.1111/ajt.13618
60. Mifsud NA, Nguyen TH, Tait BD, Kotsimbos TC. Quantitative and functional diversity of cross-reactive EBV-specific CD8+ T cells in a longitudinal study cohort of lung transplant recipients. Transplantation (2010) 90(12):1439–49. doi:10.1097/TP.0b013e3181ff4ff3
61. Nadazdin O, Boskovic S, Murakami T, Tocco G, Smith RN, Colvin RB, et al. Host alloreactive memory T cells influence tolerance to kidney allografts in nonhuman primates. Sci Transl Med (2011) 3(86):86ra51. doi:10.1126/scitranslmed.3002093
62. Kawai T, Sogawa H, Boskovic S, Abrahamian G, Smith RN, Wee SL, et al. CD154 blockade for induction of mixed chimerism and prolonged renal allograft survival in nonhuman primates. Am J Transplant (2004) 4(9):1391–8. doi:10.1111/j.1600-6143.2004.00523.x
63. Krupnick AS, Lin X, Li W, Higashikubo R, Zinselmeyer BH, Hartzler H, et al. Central memory CD8+ T lymphocytes mediate lung allograft acceptance. J Clin Invest (2014) 124(3):1130–43. doi:10.1172/JCI71359
64. Guillonneau C, Picarda E, Anegon I. CD8+ regulatory T cells in solid organ transplantation. Curr Opin Organ Transplant (2010) 15(6):751–6. doi:10.1097/MOT.0b013e32834016d1
65. Li XL, Menoret S, Bezie S, Caron L, Chabannes D, Hill M, et al. Mechanism and localization of CD8 regulatory T cells in a heart transplant model of tolerance. J Immunol (2010) 185(2):823–33. doi:10.4049/jimmunol.1000120
66. Fehr T, Sykes M. Tolerance induction in clinical transplantation. Transpl Immunol (2004) 13(2):117–30. doi:10.1016/j.trim.2004.05.009
67. Golshayan D, Pascual M. Tolerance-inducing immunosuppressive strategies in clinical transplantation: an overview. Drugs (2008) 68(15):2113–30. doi:10.2165/00003495-200868150-00004
68. Haudebourg T, Poirier N, Vanhove B. Depleting T-cell subpopulations in organ transplantation. Transpl Int (2009) 22(5):509–18. doi:10.1111/j.1432-2277.2008.00788.x
69. Mohty M. Mechanisms of action of antithymocyte globulin: T-cell depletion and beyond. Leukemia (2007) 21(7):1387–94. doi:10.1038/sj.leu.2404683
70. Koyama I, Nadazdin O, Boskovic S, Ochiai T, Smith RN, Sykes M, et al. Depletion of CD8 memory T cells for induction of tolerance of a previously transplanted kidney allograft. Am J Transplant (2007) 7(5):1055–61. doi:10.1111/j.1600-6143.2006.01703.x
71. Kroemer A, Xiao X, Vu MD, Gao W, Minamimura K, Chen M, et al. OX40 controls functionally different T cell subsets and their resistance to depletion therapy. J Immunol (2007) 179(8):5584–91. doi:10.4049/jimmunol.179.8.5584
72. Neujahr DC, Chen C, Huang X, Markmann JF, Cobbold S, Waldmann H, et al. Accelerated memory cell homeostasis during T cell depletion and approaches to overcome it. J Immunol (2006) 176(8):4632–9. doi:10.4049/jimmunol.176.8.4632
73. Zeevi A, Husain S, Spichty KJ, Raza K, Woodcock JB, Zaldonis D, et al. Recovery of functional memory T cells in lung transplant recipients following induction therapy with alemtuzumab. Am J Transplant (2007) 7(2):471–5. doi:10.1111/j.1600-6143.2006.01641.x
74. Haanstra KG, Sick EA, Ringers J, Wubben JA, Kuhn EM, t Hart BA, et al. No synergy between ATG induction and costimulation blockade induced kidney allograft survival in rhesus monkeys. Transplantation (2006) 82(9):1194–201.
75. Pearl JP, Parris J, Hale DA, Hoffmann SC, Bernstein WB, McCoy KL, et al. Immunocompetent T-cells with a memory-like phenotype are the dominant cell type following antibody-mediated T-cell depletion. Am J Transplant (2005) 5(3):465–74. doi:10.1111/j.1600-6143.2005.00759.x
76. Hu Y, Turner MJ, Shields J, Gale MS, Hutto E, Roberts BL, et al. Investigation of the mechanism of action of alemtuzumab in a human CD52 transgenic mouse model. Immunology (2009) 128(2):260–70. doi:10.1111/j.1365-2567.2009.03115.x
77. Marco MR, Dons EM, van der Windt DJ, Bhama JK, Lu LT, Zahorchak AF, et al. Post-transplant repopulation of naive and memory T cells in blood and lymphoid tissue after alemtuzumab-mediated depletion in heart-transplanted cynomolgus monkeys. Transpl Immunol (2013) 29(1–4):88–98. doi:10.1016/j.trim.2013.10.002
78. Ruzek MC, Waire JS, Hopkins D, Lacorcia G, Sullivan J, Roberts BL, et al. Characterization of in vitro antimurine thymocyte globulin-induced regulatory T cells that inhibit graft-versus-host disease in vivo. Blood (2008) 111(3):1726–34. doi:10.1182/blood-2007-08-106526
79. Yokota N, Daniels F, Crosson J, Rabb H. Protective effect of T cell depletion in murine renal ischemia-reperfusion injury. Transplantation (2002) 74(6):759–63.
80. Ayasoufi K, Fan R, Fairchild RL, Valujskikh A. CD4 T cell help via B cells is required for lymphopenia-induced CD8 T cell proliferation. J Immunol (2016) 196(7):3180–90. doi:10.4049/jimmunol.1501435
81. D’Addio F, Yuan X, Habicht A, Williams J, Ruzek M, Iacomini J, et al. A novel clinically relevant approach to tip the balance toward regulation in stringent transplant model. Transplantation (2010) 90(3):260–9. doi:10.1097/TP.0b013e3181e64217
82. Kim EJ, Kwun J, Gibby AC, Hong JJ, Farris AB III, Iwakoshi NN, et al. Costimulation blockade alters germinal center responses and prevents antibody-mediated rejection. Am J Transplant (2014) 14(1):59–69. doi:10.1111/ajt.12526
83. da Silva AJ, Brickelmaier M, Majeau GR, Li Z, Su L, Hsu YM, et al. Alefacept, an immunomodulatory recombinant LFA-3/IgG1 fusion protein, induces CD16 signaling and CD2/CD16-dependent apoptosis of CD2(+) cells. J Immunol (2002) 168(9):4462–71. doi:10.4049/jimmunol.168.9.4462
84. Majeau GR, Meier W, Jimmo B, Kioussis D, Hochman PS. Mechanism of lymphocyte function-associated molecule 3-Ig fusion proteins inhibition of T cell responses. Structure/function analysis in vitro and in human CD2 transgenic mice. J Immunol (1994) 152(6):2753–67.
85. Miller GT, Hochman PS, Meier W, Tizard R, Bixler SA, Rosa MD, et al. Specific interaction of lymphocyte function-associated antigen 3 with CD2 can inhibit T cell responses. J Exp Med (1993) 178(1):211–22.
86. Chamian F, Lowes MA, Lin SL, Lee E, Kikuchi T, Gilleaudeau P, et al. Alefacept reduces infiltrating T cells, activated dendritic cells, and inflammatory genes in psoriasis vulgaris. Proc Natl Acad Sci U S A (2005) 102(6):2075–80. doi:10.1073/pnas.0409569102
87. Ortonne JP, Lebwohl M, Em Griffiths C; Alefacept Clinical Study Group. Alefacept-induced decreases in circulating blood lymphocyte counts correlate with clinical response in patients with chronic plaque psoriasis. Eur J Dermatol (2003) 13(2):117–23.
88. Larsen R, Ryder LP, Svejgaard A, Gniadecki R. Changes in circulating lymphocyte subpopulations following administration of the leucocyte function-associated antigen-3 (LFA-3)/IgG1 fusion protein alefacept. Clin Exp Immunol (2007) 149(1):23–30. doi:10.1111/j.1365-2249.2007.03380.x
89. Chamian F, Lin SL, Lee E, Kikuchi T, Gilleaudeau P, Sullivan-Whalen M, et al. Alefacept (anti-CD2) causes a selective reduction in circulating effector memory T cells (Tem) and relative preservation of central memory T cells (Tcm) in psoriasis. J Transl Med (2007) 5:27. doi:10.1186/1479-5876-5-27
90. Ellis CN, Krueger GG; Alefacept Clinical Study Group. Treatment of chronic plaque psoriasis by selective targeting of memory effector T lymphocytes. N Engl J Med (2001) 345(4):248–55. doi:10.1056/NEJM200107263450403
91. Lo DJ, Weaver TA, Stempora L, Mehta AK, Ford ML, Larsen CP, et al. Selective targeting of human alloresponsive CD8+ effector memory T cells based on CD2 expression. Am J Transplant (2011) 11(1):22–33. doi:10.1111/j.1600-6143.2010.03317.x
92. Shapira MY, Abdul-Hai A, Resnick IB, Bitan M, Tsirigotis P, Aker M, et al. Alefacept treatment for refractory chronic extensive GVHD. Bone Marrow Transplant (2009) 43(4):339–43. doi:10.1038/bmt.2008.324
93. Shapira MY, Resnick IB, Bitan M, Ackerstein A, Tsirigotis P, Gesundheit B, et al. Rapid response to alefacept given to patients with steroid resistant or steroid dependent acute graft-versus-host disease: a preliminary report. Bone Marrow Transplant (2005) 36(12):1097–101. doi:10.1038/sj.bmt.1705185
94. Shapira MY, Resnick IB, Dray L, Aker M, Stepensky P, Elad S, et al. A new induction protocol for the control of steroid refractory/dependent acute graft versus host disease with alefacept and tacrolimus. Cytotherapy (2009) 11(1):61–7. doi:10.1080/14653240802644669
95. Weaver TA, Charafeddine AH, Agarwal A, Turner AP, Russell M, Leopardi FV, et al. Alefacept promotes co-stimulation blockade based allograft survival in nonhuman primates. Nat Med (2009) 15(7):746–9. doi:10.1038/nm.1993
96. Priyadharshini B, Turka LA. T-cell energy metabolism as a controller of cell fate in transplantation. Curr Opin Organ Transplant (2015) 20(1):21–8. doi:10.1097/MOT.0000000000000149
97. Larsen CP, Pearson TC, Adams AB, Tso P, Shirasugi N, Strobert E, et al. Rational development of LEA29Y (belatacept), a high-affinity variant of CTLA4-Ig with potent immunosuppressive properties. Am J Transplant (2005) 5(3):443–53. doi:10.1111/j.1600-6143.2005.00749.x
98. Rostaing L, Vincenti F, Grinyo J, Rice KM, Bresnahan B, Steinberg S, et al. Long-term belatacept exposure maintains efficacy and safety at 5 years: results from the long-term extension of the BENEFIT study. Am J Transplant (2013) 13(11):2875–83. doi:10.1111/ajt.12460
99. Vincenti F, Larsen CP, Alberu J, Bresnahan B, Garcia VD, Kothari J, et al. Three-year outcomes from BENEFIT, a randomized, active-controlled, parallel-group study in adult kidney transplant recipients. Am J Transplant (2012) 12(1):210–7. doi:10.1111/j.1600-6143.2011.03785.x
100. de Graav GN, Hesselink DA, Dieterich M, Kraaijeveld R, Weimar W, Baan CC. Down-regulation of surface CD28 under belatacept treatment: an escape mechanism for antigen-reactive T-cells. PLoS One (2016) 11(2):e0148604. doi:10.1371/journal.pone.0148604
101. Hamann D, Kostense S, Wolthers KC, Otto SA, Baars PA, Miedema F, et al. Evidence that human CD8+CD45RA+CD27- cells are induced by antigen and evolve through extensive rounds of division. Int Immunol (1999) 11(7):1027–33.
102. Rufer N, Zippelius A, Batard P, Pittet MJ, Kurth I, Corthesy P, et al. Ex vivo characterization of human CD8+ T subsets with distinct replicative history and partial effector functions. Blood (2003) 102(5):1779–87. doi:10.1182/blood-2003-02-0420
103. Sze DM, Giesajtis G, Brown RD, Raitakari M, Gibson J, Ho J, et al. Clonal cytotoxic T cells are expanded in myeloma and reside in the CD8(+)CD57(+)CD28(-) compartment. Blood (2001) 98(9):2817–27. doi:10.1182/blood.V98.9.2817
104. Weekes MP, Carmichael AJ, Wills MR, Mynard K, Sissons JG. Human CD28-CD8+ T cells contain greatly expanded functional virus-specific memory CTL clones. J Immunol (1999) 162(12):7569–77.
105. Baeten D, Louis S, Braud C, Braudeau C, Ballet C, Moizant F, et al. Phenotypically and functionally distinct CD8+ lymphocyte populations in long-term drug-free tolerance and chronic rejection in human kidney graft recipients. J Am Soc Nephrol (2006) 17(1):294–304. doi:10.1681/ASN.2005020178
106. Bai Y, Liu J, Wang Y, Honig S, Qin L, Boros P, et al. L-selectin-dependent lymphoid occupancy is required to induce alloantigen-specific tolerance. J Immunol (2002) 168(4):1579–89. doi:10.4049/jimmunol.168.4.1579
107. Pawlik A, Florczak M, Masiuk M, Dutkiewicz G, Machalinski B, Rozanski J, et al. The expansion of CD4+CD28- T cells in patients with chronic kidney graft rejection. Transplant Proc (2003) 35(8):2902–4. doi:10.1016/j.transproceed.2003.10.061
108. Studer SM, George MP, Zhu X, Song Y, Valentine VG, Stoner MW, et al. CD28 down-regulation on CD4 T cells is a marker for graft dysfunction in lung transplant recipients. Am J Respir Crit Care Med (2008) 178(7):765–73. doi:10.1164/rccm.200701-013OC
109. Espinosa JR, Samy KP, Kirk AD. Memory T cells in organ transplantation: progress and challenges. Nat Rev Nephrol (2016) 12(6):339–47. doi:10.1038/nrneph.2016.9
110. Badell IR, Kitchens WH, Wagener ME, Lukacher AE, Larsen CP, Ford ML. Pathogen stimulation history impacts donor-specific CD8(+) T cell susceptibility to costimulation/integrin blockade-based therapy. Am J Transplant (2015) 15(12):3081–94. doi:10.1111/ajt.13399
111. Xu H, Perez SD, Cheeseman J, Mehta AK, Kirk AD. The allo- and viral-specific immunosuppressive effect of belatacept, but not tacrolimus, attenuates with progressive T cell maturation. Am J Transplant (2014) 14(2):319–32. doi:10.1111/ajt.12574
112. Ariyan C, Salvalaggio P, Fecteau S, Deng S, Rogozinski L, Mandelbrot D, et al. Cutting edge: transplantation tolerance through enhanced CTLA-4 expression. J Immunol (2003) 171(11):5673–7. doi:10.4049/jimmunol.171.11.5673
113. Fecteau S, Basadonna GP, Freitas A, Ariyan C, Sayegh MH, Rothstein DM. CTLA-4 up-regulation plays a role in tolerance mediated by CD45. Nat Immunol (2001) 2(1):58–63. doi:10.1038/83175
114. Iwakoshi NN, Mordes JP, Markees TG, Phillips NE, Rossini AA, Greiner DL. Treatment of allograft recipients with donor-specific transfusion and anti-CD154 antibody leads to deletion of alloreactive CD8+ T cells and prolonged graft survival in a CTLA4-dependent manner. J Immunol (2000) 164(1):512–21. doi:10.4049/jimmunol.164.1.512
115. Judge TA, Wu Z, Zheng XG, Sharpe AH, Sayegh MH, Turka LA. The role of CD80, CD86, and CTLA4 in alloimmune responses and the induction of long-term allograft survival. J Immunol (1999) 162(4):1947–51.
116. Lin H, Rathmell JC, Gray GS, Thompson CB, Leiden JM, Alegre ML. Cytotoxic T lymphocyte antigen 4 (CTLA4) blockade accelerates the acute rejection of cardiac allografts in CD28-deficient mice: CTLA4 can function independently of CD28. J Exp Med (1998) 188(1):199–204.
117. Markees TG, Phillips NE, Gordon EJ, Noelle RJ, Shultz LD, Mordes JP, et al. Long-term survival of skin allografts induced by donor splenocytes and anti-CD154 antibody in thymectomized mice requires CD4(+) T cells, interferon-gamma, and CTLA4. J Clin Invest (1998) 101(11):2446–55.
118. Poirier N, Azimzadeh AM, Zhang T, Dilek N, Mary C, Nguyen B, et al. Inducing CTLA-4-dependent immune regulation by selective CD28 blockade promotes regulatory T cells in organ transplantation. Sci Transl Med (2010) 2(17):17ra10. doi:10.1126/scitranslmed.3000116
119. Poirier N, Mary C, Dilek N, Hervouet J, Minault D, Blancho G, et al. Preclinical efficacy and immunological safety of FR104, an antagonist anti-CD28 monovalent Fab’ antibody. Am J Transplant (2012) 12(10):2630–40. doi:10.1111/j.1600-6143.2012.04164.x
120. Vanhove B, Laflamme G, Coulon F, Mougin M, Vusio P, Haspot F, et al. Selective blockade of CD28 and not CTLA-4 with a single-chain Fv-alpha1-antitrypsin fusion antibody. Blood (2003) 102(2):564–70. doi:10.1182/blood-2002-08-2480
121. Zhang T, Fresnay S, Welty E, Sangrampurkar N, Rybak E, Zhou H, et al. Selective CD28 blockade attenuates acute and chronic rejection of murine cardiac allografts in a CTLA-4-dependent manner. Am J Transplant (2011) 11(8):1599–609. doi:10.1111/j.1600-6143.2011.03624.x
122. Kawai T, Andrews D, Colvin RB, Sachs DH, Cosimi AB. Thromboembolic complications after treatment with monoclonal antibody against CD40 ligand. Nat Med (2000) 6(2):114. doi:10.1038/72162
123. Adams AB, Shirasugi N, Jones TR, Durham MM, Strobert EA, Cowan S, et al. Development of a chimeric anti-CD40 monoclonal antibody that synergizes with LEA29Y to prolong islet allograft survival. J Immunol (2005) 174(1):542–50. doi:10.4049/jimmunol.174.1.542
124. Aoyagi T, Yamashita K, Suzuki T, Uno M, Goto R, Taniguchi M, et al. A human anti-CD40 monoclonal antibody, 4D11, for kidney transplantation in cynomolgus monkeys: induction and maintenance therapy. Am J Transplant (2009) 9(8):1732–41. doi:10.1111/j.1600-6143.2009.02693.x
125. Badell IR, Russell MC, Cardona K, Shaffer VO, Turner AP, Avila JG, et al. CTLA4Ig prevents alloantibody formation following nonhuman primate islet transplantation using the CD40-specific antibody 3A8. Am J Transplant (2012) 12(7):1918–23. doi:10.1111/j.1600-6143.2012.04029.x
126. Haanstra KG, Ringers J, Sick EA, Ramdien-Murli S, Kuhn EM, Boon L, et al. Prevention of kidney allograft rejection using anti-CD40 and anti-CD86 in primates. Transplantation (2003) 75(5):637–43. doi:10.1097/01.TP.0000054835.58014.C2
127. Imai A, Suzuki T, Sugitani A, Itoh T, Ueki S, Aoyagi T, et al. A novel fully human anti-CD40 monoclonal antibody, 4D11, for kidney transplantation in cynomolgus monkeys. Transplantation (2007) 84(8):1020–8. doi:10.1097/01.tp.0000286058.79448.c7
128. Pearson TC, Trambley J, Odom K, Anderson DC, Cowan S, Bray R, et al. Anti-CD40 therapy extends renal allograft survival in rhesus macaques. Transplantation (2002) 74(7):933–40.
129. Valujskikh A. The challenge of inhibiting alloreactive T-cell memory. Am J Transplant (2006) 6(4):647–51. doi:10.1111/j.1600-6143.2005.01215.x
130. Ge W, Jiang J, Liu W, Lian D, Saito A, Garcia B, et al. Regulatory T cells are critical to tolerance induction in presensitized mouse transplant recipients through targeting memory T cells. Am J Transplant (2010) 10(8):1760–73. doi:10.1111/j.1600-6143.2010.03186.x
131. Burrell BE, Lu G, Li XC, Bishop DK. OX40 costimulation prevents allograft acceptance induced by CD40-CD40L blockade. J Immunol (2009) 182(1):379–90. doi:10.4049/jimmunol.182.1.379
132. Chen M, Xiao X, Demirci G, Li XC. OX40 controls islet allograft tolerance in CD154 deficient mice by regulating FOXP3+ Tregs. Transplantation (2008) 85(11):1659–62. doi:10.1097/TP.0b013e3181726987
133. Kitchens WH, Larsen CP, Ford ML. Integrin antagonists for transplant immunosuppression: panacea or peril? Immunotherapy (2011) 3(3):305–7. doi:10.2217/imt.10.113
134. Kitchens WH, Haridas D, Wagener ME, Song M, Kirk AD, Larsen CP, et al. Integrin antagonists prevent costimulatory blockade-resistant transplant rejection by CD8(+) memory T cells. Am J Transplant (2012) 12(1):69–80. doi:10.1111/j.1600-6143.2011.03762.x
Keywords: memory T cells, allotransplantation, tolerance, heterologous immunity, transplant rejection, immune suppression, costimulation blockade
Citation: Benichou G, Gonzalez B, Marino J, Ayasoufi K and Valujskikh A (2017) Role of Memory T Cells in Allograft Rejection and Tolerance. Front. Immunol. 8:170. doi: 10.3389/fimmu.2017.00170
Received: 28 September 2016; Accepted: 02 February 2017;
Published: 28 February 2017
Edited by:
Alok Srivastava, Christian Medical College & Hospital, IndiaReviewed by:
Régis Josien, University of Nantes, FranceIgnacio Anegon, University of Nantes, France
Copyright: © 2017 Benichou, Gonzalez, Marino, Ayasoufi and Valujskikh. This is an open-access article distributed under the terms of the Creative Commons Attribution License (CC BY). The use, distribution or reproduction in other forums is permitted, provided the original author(s) or licensor are credited and that the original publication in this journal is cited, in accordance with accepted academic practice. No use, distribution or reproduction is permitted which does not comply with these terms.
*Correspondence: Gilles Benichou, Z2JlbmljaG91JiN4MDAwNDA7cGFydG5lcnMub3Jn;
Anna Valujskikh, dmFsdWpzYSYjeDAwMDQwO2NjZi5vcmc=