- 1Laboratory of Respiratory Mucosal Immunity, School of Biomedical Sciences, The University of Queensland, St. Lucia, QLD, Australia
- 2Translational Research Institute, The University of Queensland Diamantina Institute, The University of Queensland, St. Lucia, QLD, Australia
- 3The School of Agriculture and Food Sciences, The University of Queensland, St. Lucia, QLD, Australia
- 4School of Paediatrics and Child Health, University of Western Australia, Perth, WA, Australia
- 5Australian Infectious Diseases Research Centre, The University of Queensland, St. Lucia, QLD, Australia
Severe viral lower respiratory infections are a major cause of infant morbidity. In developing countries, respiratory syncytial virus (RSV)-bronchiolitis induces significant mortality, whereas in developed nations the disease represents a major risk factor for subsequent asthma. Susceptibility to severe RSV-bronchiolitis is governed by gene–environmental interactions that affect the host response to RSV infection. Emerging evidence suggests that the excessive inflammatory response and ensuing immunopathology, typically as a consequence of insufficient immunoregulation, leads to long-term changes in immune cells and structural cells that render the host susceptible to subsequent environmental incursions. Thus, the initial host response to RSV may represent a tipping point in the balance between long-term respiratory health or chronic disease (e.g., asthma). The composition and diversity of the microbiota, which in humans stabilizes in the first year of life, critically affects the development and function of the immune system. Hence, perturbations to the maternal and/or infant microbiota are likely to have a profound impact on the host response to RSV and susceptibility to childhood asthma. Here, we review recent insights describing the effects of the microbiota on immune system homeostasis and respiratory disease and discuss the environmental factors that promote microbial dysbiosis in infancy. Ultimately, this knowledge will be harnessed for the prevention and treatment of severe viral bronchiolitis as a strategy to prevent the onset and development of asthma.
Introduction
Viral lower respiratory infections (vLRI) caused by respiratory syncytial virus (RSV) are a major cause of morbidity and mortality in young infants. In developed countries, epidemiological studies have reproducibly implicated RSV-bronchiolitis as a major risk factor for the onset and progression of asthma [reviewed extensively in Ref. (1)]. The immunopathology associated with RSV-bronchiolitis in early-life may lead to long-lived alterations to airway structural and/or resident immune cells that render the host susceptible toward allergic sensitization and asthma (2–4). Susceptibility to both RSV-bronchiolitis and asthma has been attributed to defects in immunoregulatory and antiviral pathways, the cause of which may be genetic and/or environmental (1, 2, 5, 6). Interestingly, rates of RSV-bronchiolitis peak at 2–6 months of age (7, 8), whereas asthma typically begins to develop later in childhood (2), highlighting a window of susceptibility to RSV in early-life, which, if engaged, may leave the host vulnerable to asthma development. Hence, factors that influence the development and/or function of the immune system may critically impact the host response to RSV. It is now recognized that the composition and diversity of the microbiota, which stabilizes around 12 months of age in humans (9–13), fundamentally affects host physiology and immunity (12, 14–19). Several recent studies have demonstrated changes in the respiratory microbiome in subjects with RSV-bronchiolitis (20–23). Similar observations have been reported in asthma (24–28). Whether microbial dysbiosis—changes in the composition of the gut and/or lung microbiota—predisposes to RSV-bronchiolitis and asthma or is simply a consequence of disease remains unclear. In this review, we discuss recent clinical and experimental findings implicating a role for the microbiota in infant respiratory health and disease. We also consider how this knowledge might be harnessed for the prevention and treatment of severe bronchiolitis as a strategy to curtail the short- and long-term burden of disease, with a specific emphasis on the onset and development of asthma.
The Link Between Severe or Frequent vLRI and Subsequent Asthma Development
Numerous birth cohort studies have assessed the association between vLRIs in early-life and asthma risk (29–46). Although this association is moderate for certain viruses [e.g., influenza (47) and human metapneumovirus (44, 48–50)], the association is much stronger for RSV and rhinovirus (RV) (29–42). This relationship also appears to extend to allergic sensitization, but whereas severe RSV vLRIs appear to precede allergic sensitization this is less common for RV; rather allergic sensitization is a precursor to severe RV vLRIs (51, 52). A recent study evaluating two large cohorts of infants (>80,000 and >180,000) found that the population-attributable risk for asthma contributed by infant bronchiolitis during the RSV season was 10 and 13%. However, in the subset of children with infant RSV-bronchiolitis, the attributable risk was 49 and 47% (41). The relationship between RSV disease severity and subsequent asthma inception is dose-dependent lending support to a causal relationship (42, 52, 53). In further support of this notion, RSV-immunoprophylaxis is effective in reducing rates of recurrent wheeze and asthma (54–56). However, because all humans have been infected with RSV by the age of 3 and the population at large is not asthmatic, other co-factors (e.g., genetic and/or environmental) likely collude with RSV, to increase the risk of bronchiolitis in infancy. Thus the asthma-prone phenotype is likely established early in life and progresses to persistent disease following subsequent rounds of allergen and/or viral exposure (2). Using a high-fidelity mouse model, we have demonstrated that defects in plasmacytoid dendritic cells (pDCs) or toll-like receptor (TLR) 7/interferon regulatory factor (IRF) 7 signaling predisposes to severe viral bronchiolitis and subsequent asthma (57–61). In this scenario, the impaired immune response may lead to enhanced epithelial injury and secondary tissue repair leading to long-lived changes to the epithelium (genetic or epigenetic). In turn, this may promote the development of Th2 immunity by modulating the underlying network of DCs and innate lymphoid cells (ILCs). We and others have shown that house dust mite (HDM)-induced epithelial-derived IL-1α and high-mobility group box (HMGB) 1 act in a cytokine cascade and in feed-forward loops to induce the production of Th2-instructive cytokines, such as IL-33, thymic stromal lymphopoietin, IL-25, and granulocyte-macrophage colony-stimulating factor (GM-CSF), which license local DCs and type 2 ILC to promote Th2 immunity (62–64). Hence, epithelial stress as a consequence of allergen exposure or respiratory viral infection-associated cytopathology in predisposed individuals leads to the release of tissue alarmins—IL-1α, HMGB1, and IL-33—to promote a cytokine microenvironment that is conducive to the generation of Th2 immunity. In addition to providing pro-type 2 inflammatory signals, these cytokines also act by inhibiting pro-type I inflammatory signals. For example, allergen-induced IL-33 amplifies the allergic response in part by inhibiting CD11b+ DCs (DC2) IL-12p35 production (65). IL-33 can also suppress the production of interferon alpha, suppressing its negative regulatory effects on type 2 immunity (59, 66).
The teleological role of Th2 immunity is to initiate tissue repair; however, following repeated environmental insult(s) throughout life, type 2 inflammation may lead to pathologic tissue remodeling (67–69). Once established, this inflammatory response is maintained by both structural cells [e.g., airway epithelial cells (AECs), endothelial cells, and airway smooth muscle cells] and immune cells (e.g., type 2 ILCs, DCs, macrophages, and T helper cell subsets), which act in concert to produce an array of pro-inflammatory lipids, cytokines, and chemokines [recently reviewed in Ref. (4)]. The relative importance of type 2 ILCs and CD4+ Th2 in this process has been the subject of much interest and debate in the field with recent studies implicating type 2 ILCs in antigen presentation (70) and potentiating memory CD4+ Th2 cells (via IL-13-dependent licensing of DCs) (64). Intriguingly, “innate” CD4+ Th2 cells have been proposed. This novel cell subset can be primed locally in the lung by Th2-instructive cytokines, together with an activator of the signal transducer and activator of transcription 1 family of transcription factors, in the absence of antigen presentation (71).
Now, that a number of type 2 instructive cytokines have been identified, this has opened up opportunities to halt the progression and/or decrease the severity of asthma through the use of humanized monoclonal antibodies. Additionally, a better understanding of the factors that confer susceptibility to bronchiolitis and its nexus with asthma onset, may yield new opportunities for targeted intervention. Critically, this raises the possibility of primary prevention. Emerging evidence discussed in this review highlights the supportive influence of the microbiome on the maturing immune system. Thus perturbations to the microbiome, occurring prenatally or postnatally, could adversely affect host defense against RSV, and this might lead to long-term alterations as a consequence of aberrant programming (genetic or epigenetic) of structural and/or immune cells. In this paradigm, further exposure of these susceptible individuals to environmental triggers of asthma (e.g., allergen and/or viral infection) in later-life may progress the asthma-prone phenotype to established and persistent disease.
The Airway Bacterial Microbiota is Dysbiotic in Asthma, but Why?
For centuries, the lower airways have been considered a sterile environment, a dogma based primarily on culture-based studies in which any culturable microbes from bronchoalveolar lavage (BAL) samples were considered to be contamination or of little clinical significance (72). In fact, the lung harbors an abundant and diverse microbial community (a microbiota) that is highly dynamic and underpinned by the immigration and emigration of microbes with every breath of air (approximately 7,000 L/day) and the occasional subclinical aspiration of the oropharyngeal contents (73–75). Culture-independent techniques involving high-throughput sequencing of the 16S rRNA gene, a highly conserved locus of the bacterial genome, has led to a revolution in our understanding of the airway microbiome. Using this approach, investigators have begun to probe the human airways in health and disease, and pioneering studies have revealed that the microbial community inhabiting the lower airways of asthmatics is indeed quite different from that of healthy subjects (25, 26, 28). Whether the asthmatic airways are more highly populated (i.e., grater bacterial load) remains unclear with some studies finding increases in abundance (25) and others reporting no difference compared to control (26). Several studies report that the airway microbiota, sampled by BAL (26, 76) or nasal swab (28), of mild-to-moderate asthmatics is enriched in members of the Gram-negative bacterial phylum Proteobacteria including the potential pathogens Haemophilis, Moraxella, and Neisseria (26, 28, 76) and reduced in the commensal phylum Bacteroidetes (28, 76). However, this profile appears to differ somewhat according to the inflammatory phenotype and/or severity status, as well as corticosteroid treatment (27). For example, in a study directly comparing the sputum microbiota in severe and non-severe asthma, Zhang and colleagues found that mild-to-moderate and, to a lesser extent, severe disease was associated with increased Proteobacteria, while both severe and non-severe asthmatic individuals had a lower abundance of Bacteroidetes (particularly Prevotella spp.) and Fusobacteria (76). Moreover, a greater abundance of Streptococcus spp., which are affiliated with the phylum Firmicutes, was uniquely associated with severe asthma. In this study, severe asthma was associated with blood and sputum eosinophilia, but not neutrophilia. Green et al. found that sputum neutrophilia and IL-8 levels directly correlated with the abundance of Streptococcus spp, as well as Haemophilus and Moraxella in severe asthmatics (77), while Goleva and colleagues reported that Haemophilus parainfluenzae was uniquely present in the airways of corticosteroid-resistant asthmatics (26). Additionally, a study comparing the sputum microbiome in chronic and persistent/severe asthmatics found reduced bacterial diversity combined with a high prevalence of Haemophilus influenzae in asthmatics with neutrophilic inflammation, whereas asthmatics with eosinophilic inflammation had abundant Tropheryma whipplei (78). One interpretation of these data is that disease severity and possibly the inflammatory profile or asthma phenotype relate to the composition or dysbiosis of the airway microbiota. Further work is needed to determine whether the presence or absence of certain microbial communities underpins different asthma phenotypes or whether these changes are secondary to the pathological environment characteristic of the asthma subtype. Whereas several studies have examined the lung microbiota in stable asthma, very few have examined the microbiota during acute exacerbation(s) of asthma. This is surprising, since 80% of asthma exacerbations are attributed to a viral infection (34, 79), predominantly a species of RV, which likely affects or is affected by the bacterial community of the airways. An interesting study in which healthy adult subjects were experimentally infected with RV revealed increases in the relative abundances of upper airway H. parainfluenzae and Neisseria subflava following infection (80), two potentially pathogenic bacterial species reported to be present in the lower airways in asthma. Whether the upper airway microbiota reflects the lower airways remains an unresolved question, but these findings do highlight a shift toward an asthma-like microbiota caused by RV infection, which may impact the mechanism by which RV causes asthma exacerbations. In an attempt to address this issue, Kloepfer et al. studied a population of 308 school-age children, half with asthma and half healthy controls using PCR-based analysis of nasal secretions for a period of 5 weeks during autumn over 2 consecutive years (81). The authors found that the presence and abundance of either Moraxella catarrhalis or Streptococcus pneumoniae at the time of RV infection was positively associated with the severity of RV-associated exacerbations. The authors speculated that the viral infection/exacerbation could lead to a lung environment that is conducive to colonization with potential pathogens.
In summary, it is evident that lung microbial dysbiosis represents a component of the asthmatic syndrome; however, the nature of this dysbiosis and its relationship to asthma phenotype(s) remain ill-defined and needs further investigation. More importantly, it remains an open question as to whether microbial dysbiosis precedes and is causal for asthma onset or simply develops as a consequence of the dysregulated lung environment. In this regard, we must consider the early-life origins of asthma.
Airway Microbial Dysbiosis and the RSV-Bronchiolitis to Asthma Nexus
The notion that asthma risk may be influenced by inappropriate colonization by microbes stems from the seminal findings of the Copenhagen Birth Cohort Study (82). Using culture-dependent profiling, this study was the first to uncover the presence of potentially pathogenic species in the oropharynx of 1-month-old infants significantly at risk of developing asthma in later childhood. Pathogenic species detected in these subjects included M. catarrhalis, H. influenzae, or S. pneumoniae. Additional studies by this group that showed these same bacterial species were present during periods of episodic wheeze caused by infection with a respiratory virus (83). These paradigm-shifting studies implicated colonization of the airways by opportunistic pathogens in the inception of asthma. Whether these bacterial species are causative for later asthma is not yet clear and remains a hotly debated subject (84–86). Some investigators argue that if pathogenic bacteria were causative then antibiotic therapies should reduce wheezy episodes and decrease asthma risk, but meta-analyses of population cohort studies suggest they do not (85–87). However, this interpretation is oversimplified since antibiotic treatment also ablates bacteria that are beneficial, for example those species which generate metabolites that promote the differentiation of regulatory T (Treg) cells (discussed later in more detail). Modern 16S RNA gene sequencing has revolutionized this area of research and continues to provide new insights. Recent studies armed with this technology have begun analyzing airway and gastrointestinal samples from birth cohort studies (22, 23, 88). Such analyses have also attempted to address important questions surrounding which bacterial species first colonize the airways and whether this differs in settings of acute respiratory illness and asthma risk. For example, an elegant study by Teo et al. sought to address this gap in knowledge in a recent study of children enrolled in the Childhood Asthma Study (22). In this study, nasopharangeal aspirates were collected from subjects during planned visits at 2, 6, and 12 months of age and within 48 h from the onset of an acute respiratory infection. This high intensity sampling regimen allowed the authors to temporally assess changes in the nasopharangeal microbiota and the relationship between upper airway colonization and chronic wheeze outcome at age 5. They found that Staphylococcus and Corynebacterium, common components of the human skin microbiome, were the dominant bacterial species present in the first 2 months of life before switching to either Alloiococcus or Moraxella at 6–12 months, concomitant with a stabilization of the microbiome. Because of the presence of species typically found on the skin, the authors speculated that infants are likely to be colonized initially with skin-dwelling bacteria from their parents and others, and that these founder populations are replaced over time by Moraxella or Alloiococcus. This upper respiratory microbiota then remains stable over time in healthy individuals. However, in infants suffering from virus-associated acute respiratory infection(s), a greater abundance of potential pathogens Streptococcus, Moraxella, and Haemophilus was observed, verifying earlier findings (82). Interestingly, when examining upper and lower respiratory infections separately, the authors found that early Moraxella colonization was associated with younger age of first upper respiratory infection, whereas early Streptococcus colonization was associated with earlier initial lower respiratory infection. Additionally, the level of subsequent asthma risk in these infants appeared to relate inversely with the age at initial Streptococcus colonization, leading the authors to speculate that tissue damage mediated by Streptococcus and/or infection with RSV/RV during this early-life period might have long-term effects that predispose to later asthma. A recent study in mice found that infection of 1-week-old neonates with a non-lethal dose of S. pneumoniae (D39) followed by aluminum hydroxide (alum)/ovalbumin (OVA) administration and OVA challenge in adulthood led to Th17 type inflammation, neutrophilia, and airway hyperreactivity (AHR) (89). Type 2 inflammation was also observed but did not correlate with increased AHR or gross lung pathology in co-exposed mice. Rather, the exaggerated Th17 phenotype was associated with impaired accrual of lung FoxP3+ Treg cells which typically suppress pathogenic effector T cell driven inflammation, including that mediated by Th17 cells. The function of these Treg cells was not assessed in this study and the nature of this altered immune response and tissue damage remains unclear. Nevertheless, these data suggest that S. pneumoniae exposure in early-life may act to dampen Treg cell responses, rendering the host susceptible to allergic airway inflammation. Because reduced numbers of Tregs have been observed in infants with RSV-bronchiolitis (90), and animal models have revealed a crucial role for these cells in regulating the inflammatory response to RSV (91), one possibility is that early colonization of the respiratory tract with S. pneumoniae dampens Treg cell responses thereby enhancing the severity of RSV disease, and in turn predisposing to allergic sensitization and asthma.
Notably, the study by Teo et al. also found that the presence of Moraxella was associated with increased severity of RSV infection. The relationship between RSV and Moraxella co-infection is an interesting one. Both virus and bacterium are more abundant during cooler months, and co-infection has been associated with a greater incidence of otitis media (92), suggesting that RSV infection may alter the upper respiratory microbiome of the infant host in a way that is conducive to bacterial infection (e.g., Moraxella). A recent study by Mansbach and colleagues explored this idea in a prospective cohort of >1,000 infants at 3 months of age (23). Of these infants, the major viral pathogen detected was RSV with a smaller proportion of subjects exhibiting a co-infection with RV, and a smaller proportion still infected with RV alone. However, healthy controls were not included in this study. Comparing the nasopharyngeal microbiota of these three groups, the authors found a high level of Firmicutes and the genus Streptococcus and a low level of Proteobacteria and the genera Haemophilus and Moraxella in the RSV-only group, while the opposite was true for the RV-only group and the RSV/RV co-infection group exhibited intermediate abundances of these phyla and genera. This pattern of specific virus and bacteria detected in the upper airways is akin to that observed by Teo and colleagues. However, it is not yet sufficiently clear whether these pathogenic bacteria contribute to disease or are simply reflective of opportunistic colonization/expansion in individuals with more severe illness. This question requires further work using both clinical cohorts and translational animal models of disease. The latter will be fundamental to unraveling the complex interplay between host, virus and bacteria (airway microbiota and pathogens) and the long-term consequences for asthma risk.
Microbial Colonization and the Development of Early-Life Respiratory Disease
Admission rates for bronchiolitis (93) and the prevalence of asthma and allergies have increased substantially over the past 30 years (94–96). This has implicated a key role for environmental factors, most notably those associated with a “Western lifestyle.” Initial evidence for this was garnered from epidemiological studies in Europe linking decreases in family size and increased personal hygiene with allergic disease development and led to the generation of the “hygiene hypothesis” (97, 98). The central tenet of the hygiene hypothesis is that components of the mammalian immune system fail to develop appropriately or become dysregulated as a consequence of insufficient exposure to microbes. Subsequent studies of European children growing up in rural environments have strongly supported and extended this concept. For example, Reidler et al. demonstrated in a cross-sectional study that children who grew up in rural farming environments were less likely to develop allergy and asthma and that disease risk was even lower if the mother had also experienced this microbe-rich environment during pregnancy (99). Other studies in farming communities have shown that high levels of lipopolysaccharide (LPS) in barn-dust and gastrointestinal exposure to unpasteurized milk are associated with tolerance to ubiquitous allergens and the prevention of atopy (100, 101). The protective “farm effect” on childhood asthma has been reproduced in several studies around the world, and a recent meta-analysis concluded that an overall 25% reduction in asthma risk can occur despite heterogeneity in the study populations (102). However, the strongest protection from childhood asthma was recently reported in Amish populations in the US, which retain traditional farming methods (e.g., use of horses for transportation, single-family dairy farms) (103). By contrast Hutterites living in the US, with otherwise similar genetic background and lifestyle factors, embrace modern farming technologies (e.g., highly industrialized, communal farms). Among these children, the prevalence of asthma in Amish compared to Hutterite school children was 5.2 versus 21.3% and the prevalence of allergic sensitization was 7.2 versus 33.3%. Interestingly, LPS levels were 6.8 times higher in Amish homes than in Hutterite homes, a finding that was associated with greater numbers of peripheral blood neutrophil which expressed lower levels of the activation markers (CD11b, CXCR4, and CD11c), lower numbers of peripheral blood eosinophils and lower production of the Th2-associated cytokines IL-33, IL-25, IL-5, and IL-4 by peripheral blood lymphocytes in the Amish children compared to the Hutterite children. By exposing mice to Amish or Hutterite house dust extracts prior to performing an i.p. OVA model of allergic airway inflammation, the authors showed that the high LPS levels of the Amish house dust conferred protection from AHR and airway eosinophilia, whereas the Hutterite house dust did not. As had been shown in a previous report of European farm children (104), the beneficial effect of house dust from the Amish children was associated with higher expression of the A20 gene (TNFAIP3) in peripheral blood lymphocytes; however, an increase in IRF7, a hub gene and master regulator of type-I IFN production, was also found in Amish children. Hence, it will be important to determine whether the beneficial effects of farm house dust exposure extend to protecting against vLRI and viral exacerbations of asthma.
Further evidence for the role of local microbial exposures protecting against allergic disease development in early-life comes from studies of microbial exposures associated with pet ownership. For example, one study showed that exposure to dogs and to a lesser extent cats in infancy is associated with protection against the development of allergies in childhood, although asthma rates were not examined (105). Notably, environments with no household pet exhibit low levels of bacteria in the local environment (105–107), and thus in contrast to farm environments, these “microbe low” residences confer a higher risk of allergic disease development. Intriguingly, these homes are reported to possess a wider range of fungal species (108), which have long been associated with the development of asthma (109), although whether they shape the microbiome in early-life in a way that renders the host susceptible toward the development of allergies and asthma in later childhood remains to be determined.
These findings have fueled experimental animal studies to establish the mechanisms underlying the link between microbial exposure and protection from asthma. For example, a recent study by Schuijs et al. examined the beneficial effects of barn-dust in an acute HDM model of allergic airway inflammation in mice (104). In this murine model, a key initial step in the process of sensitization to the HDM is allergen-induced activation of AECs through TLR4. TLR4-signaling leads to the secretion of the chemokine CCL20 and GM-CSF, both of which are required for the recruitment and functional maturation of airway DCs. Consistent with the results of the European epidemiologic barn-dust studies, Schuijs and colleagues found that airway exposure to LPS before or during HDM exposure markedly attenuated the asthma-like pattern of pro-inflammatory responses to HDM and reduced the activation of local DC functions mediated by CCL20 and GM-CSF. They further demonstrated that exposure to LPS suppresses AEC responsiveness to TLR4-induced activation by HDM and that this suppression is dependent on the attenuation of signaling by nuclear factor κB (NF-κB). This effect was mediated by an increase in the synthesis of the enzyme A20, encoded by the Tnfaip3 gene, in AECs. Ex vivo cultures of human bronchial epithelial cells revealed a similar inverse association between LPS-mediated stimulation of GM-CSF production and activation of the TNFAIP3 gene. This association was further examined in a case–control study in which the investigators observed a relative deficiency in the LPS-mediated TNFAIP3 gene expression in AECs from asthmatic subjects as compared with healthy controls. Moreover, the authors observed a positive association between a polymorphism in the TNFAIP3 gene and susceptibility to asthma in the cohort of GABRIELA [Multidisciplinary Study to Identify the Genetic and Environmental Causes of Asthma in the European Community (GABRIEL) Advanced Study]. Thus, exposure to aerosolised bacterial components appears to regulate the threshold for AEC activation, highlighting A20 expression by AECs as a potential therapeutic target for asthma. Although, the effects of A20 knockdown in AECs abrogated LPS-mediated protection against the airway pathology induced by HDM, CCL20, and GM-CSF were not fully suppressed. This suggests the existence of other pathways by which LPS suppresses asthma. This apparent heterogeneity is not unexpected and perhaps reflects the complex nature of the causal pathways underlying asthma development, particularly in relation to other environmental stimuli.
Microbiota Hypothesis
Following birth, the infant is exposed to a diverse host and environmentally associated microbiota. During natural birth, the infant is principally colonized by a founder community of microbes derived from the mother’s vagina and feces (13, 110). Postnatally, breast milk further diversifies the neonatal microbiota by providing secretory immunoglobulin (Ig)A, prebiotic glycans (e.g., milk oligosaccharides), and other microbial metabolites [e.g., short-chain fatty acids (SCFAs)] (12, 111, 112). Cesarean birth and formula feeding disturb the natural process of colonization. In offspring born by Cesarean section, the predominant bacterial populations in gut closely align with that of the mother’s skin (111, 113), an effect that it is further compounded in formula-fed infants (114). The observation that formula-fed infants are at greater risk of chronic inflammatory and metabolic diseases indicates that prebiotic- or probiotic-supplemented infant formulas do not fully replicate the effect of breast milk on the development of the neonatal microbiota (115). This highlights that our knowledge of the factors that influence the emergence of the neonatal microbiota remains incomplete. Nevertheless, it is now appreciated that the composition of the intestinal microbiota is highly plastic during the early-postnatal period and into early childhood, changing rapidly in response to environmental interventions, including invasion by pathogenic microorganisms, antibiotic treatment, and diet (116), all of which likely affect immune homeostasis. Thus, the hygiene hypothesis has now been extended to incorporate a wider array of environmental conditions that can perturb the microbiome, especially in the gastrointestinal tract. This conceptual evolution has led to a new theory coined the “microbiota hypothesis.” Studies of gnotobiotic mice [also called germ-free (GF)] mice have established a key role for the host microbiota in influencing the development and function of the immune system GF mice exhibit several gross physiological and functional abnormalities in the gastrointestinal tract including enlarged cecum, reduced gastrointestinal motility, and reduced production of antimicrobial peptides (117, 118). The absence of commensals also has profound effects on the development of the immune system, including defects in lymphoid tissue development within the spleen, thymus, and peripheral lymph nodes (119). Of relevance, an elegant study by Olszak and colleagues found that invariant natural killer T cells accumulate in the colonic lamina propria and lung in GF mice, resulting in increased morbidity in models of inflammatory bowel disease and allergic asthma as compared with that of specific pathogen-free mice (120). Notably, the elevated expression of the chemokine ligand CXCL16 in the lungs of GF mice could be normalized by conventionalization (introduction of GF mice to SPF conditions) but only during the first 2 weeks of life, an effect that was dependent on microbiota-induced epigenetic modifications at the Cxcl16 locus. Conventionalization after this 2-week period failed to rescue the asthma phenotype, highlighting a critical window of susceptibility during the postnatal period. Beyond the Cxcl16 gene, it will be important to elucidate the genetic or epigenetic mechanisms by which the microbiota programs airway immune and structural cells as this appears to fine-tune the host response and affect not just the initial encounter with an environmental stimuli, such as an allergen or pathogen, but also the development of long-term immunity.
It is emerging that microbial metabolites play a key role in regulating host physiology and immunity. Herein, we focus on the SCFAs acetic acid, butyric acid, and propanoic acid; however, it is noteworthy that other lipid derivatives are known to have immunomodulatory effects [reviewed in Ref. (121)]. SCFAs range in concentration from 50 to 100 mM in the gut and are the main metabolic end products from bacterial fermentation, particularly fermentation of dietary fiber. Hence, changes in dietary habits and factors that perturb the microbiota such as antibiotic use have a profound effect on SCFA production (122, 123). In the gastrointestinal tract, SCFAs represent an important energy source for both the local microbiota and intestinal epithelial cells. But, as well as being local substrates for energy production, it is now appreciated that SCFAs have diverse regulatory functions in host physiology and immunity affecting both hematopoietic and non-hematopoietic cells. SCFAs function as histone deacetylase (HDAC) inhibitors and ligands, predominantly agonists, of G protein-coupled receptors (GPRs). The ability of SCFAs to inhibit HDACs generally promotes a tolerogenic, anti-inflammatory cell phenotype necessary for the maintenance of immune homeostasis, and this functional property supports the concept that the gut microbiota can act as an epigenetic regulator of host physiology. Exposure of peripheral blood mononuclear cells or neutrophils to acetate, propionate, or butyrate suppresses NF-κB and downregulates the production of the pro-inflammatory cytokine TNF-α (124, 125). Subsequent studies revealed that these anti-inflammatory effects, mediated by HDAC inhibition, extend to both macrophages (126, 127) and DCs (128, 129). SCFAs can also modulate T cells, particularly Treg cells, through HDAC inhibition. For example, inhibition of HDAC9 increases FoxP3 expression, enhancing the suppressive function of Treg cells during homeostasis and in mouse models of colitis (130). Studies characterizing the ability of specific SCFAs to regulate the quality of the colonic Treg cell pool have shown that propionate and butyrate induce FoxP3 in an HDAC-dependent manner, while acetate is less effective (15, 16). Of direct relevance to the function of SCFAs in asthma, Thorburn and colleagues explored the effect of modifying dietary fiber intake on the susceptibility of mice toward HDM-induced allergic airway inflammation (131). They began by comparing the gut microbiota of mice fed a high-fiber diet to mice fed an equicaloric control diet or a no-fiber diet for 3 weeks. As expected, the high-fiber diet significantly increased circulating levels of acetate and propionate, a phenotype that was associated with greater representation of Bacteroides, Clostridium, and Pandoraea spp. compared to mice fed the control diet. Mice fed on a no-fiber diet exhibited reduced (although not statistically significant) circulating SCFA levels and a greater abundance of Proteobacteria compared to the control diet group. When the effect of diet on HDM-induced allergic airways inflammation was assessed, mice fed a high-fiber diet or treated with acetate in the drinking were protected exhibiting reduced levels of airway eosinophils, type 2 cytokine production (IL-4, IL-5, and IL-13), serum IgE, and AHR. The no-fiber diet did not alter susceptibility to asthma in this model, consistent with the lack of effect on SCFA levels, which was unexpected given previous findings from another group (129). Because asthma typically develops in childhood, the authors next explored the effect of their dietary perturbations or acetate exposure during late-stage pregnancy (from E13) until weaning (3 weeks of age). The offspring of mice fed a high-fiber diet were protected from HDM-induced allergic airway inflammation whereas the offspring from mice fed a no-fiber diet developed disease to the same degree as mice fed the control diet. This latter finding is quite surprising given the importance of the gut microbiota and circulating SCFAs for immune development in early-life. A possible explanation stems from the use of a high-dose of HDM which likely induced a maximal response in the control fiber diet fed mice, and hence this response could not be further elevated in the group fed on a no-fiber diet. Nevertheless, the effectiveness of the high-fiber maternal diet suggests that protective effects can occur in utero. In support of this, the investigators found that either acetate administration or high-fiber diet feeding until birth was sufficient to protect the offspring. This finding was further confirmed by litter swapping experiments, although the effect was less profound, for example, IL-5/13 production from lymph node T cell cultures was unchanged in the high-fiber group, suggesting that some of the protective effects of the high-fiber diet occur postnatally. To translate their findings, the authors next explored whether human SCFA levels correlated with fiber intake in pregnant mothers and wheeze in their offspring. A significant but weak inverse correlation was observed between circulating maternal acetate levels and the percentage of infants requiring two or more general practitioner visits for wheeze and cough. Although viruses are a major cause of wheeze in infancy, the authors did not report which respiratory viruses were detected in these subjects. Importantly, it remains unknown whether lower SCFA levels, because of changes in maternal diet or other postnatal perturbations, increase the risk of respiratory viral illness, a major risk factor for asthma. Since the high-fiber diet conferred protection in utero and this persisted into adulthood the investigators hypothesized that this effect might be due to epigenetic regulation of Treg cells. They found that, through HDAC9 inhibition, acetate treatment endowed greater numbers of Treg cells and increased the suppressive capacity of these cells. However, it remains to be tested whether a high-fiber diet also mediates this effect specifically via acetate. The authors speculated that propionate played a less important role in their model, primarily because it was found at lower concentrations in the circulation; however, propionate is also a potent HDAC inhibitor in Treg cells, and other investigators have demonstrated a key role for propionate in protecting against HDM-induced asthma (129). Thus, the in utero effects of propionate remain ill-defined.
Short-Chain Fatty Acids and Asthma
As well as their ability to inhibit HDAC activity, several studies have determined that SCFAs affect host immunity via engagement of GPRs, including OLFR78, GPR43 (FFAR2), GPR41 (FFAR3), and GPR109A (HCAR2). These receptors are expressed by numerous cell types, including immune cells and intestinal epithelial cells and are activated by various endogenous agonists including those summarized in Table 1. GPR43 signaling plays an important role in SCFA-induced neutrophil chemotaxis (124) and the expansion and suppressive function of Treg cells induced by SCFAs. GPR41 has aslo been implicated in supporting host immune function (132). In wild-type mice, but not in GPR41-deficient mice, SCFAs arrest the maturation of pro-inflammatory DCs in a GPR41-dependent mechanism, thereby preventing the development of allergic airway inflammation in mice (129). It is noteworthy that a recent study reported mRNA and protein expression of GPR41 and GPR43 in bronchial epithelial cells (133), raising the possibility that SCFAs may regulate the airway epithelium, although functional studies remain to be performed. Studies in man have begun to test the hypothesis that decreases in gut microbial SCFAs antedate asthma development. In a recent high-profile study of Canadian infants, acetate levels in feces and serum were found to be lower in atopic/wheezy infants (at high risk of childhood asthma) compared to healthy controls at 3 months (but not 12 months) of age (134). This was associated with reductions in specific bacterial genera: Faecalibacterium, Lachnospira, Veillonella, and Rothia in the feces. Similarly, in a cohort of Swedish infants, the composition and diversity of the gut microbiota during the first month of life was significantly lower and associated with asthma diagnosis at 7 years of age (135). Collectively, these data suggest that a lack of gut microbial diversity in early-life has long-term consequences on host immunity. However, the direct cause of low infant SCFA levels in these studies remains unclear and may stem from a combination of factors affecting the maternal and neonatal microbiota. It will be important to address whether low SCFA levels are causally linked to vLRIs and asthma development and therein the underlying mechanisms. Additionally, whether poor diet and perturbations to the gut microbiota increase bronchiolitis risk as a consequence of a reduction in circulating SCFA levels remains to be determined; however, one might speculate this would have important long-term consequences for predisposition toward asthma and possibly other chronic respiratory diseases such as chronic obstructive pulmonary disease. In this regard, establishing whether SCFAs enhance lung epithelial barrier integrity is likely to be of great importance. Given the accumulating data discussed in this review linking vLRIs and microbiota-associated perturbations in early-life it will be important to elucidate the biological mechanisms underlying these clinical observations. We propose that maternal and/or infant microbial dysbiosis increases and prolongs the window of bronchiolitis risk in infancy (see Figure 1); this may occur through functional aberrancy of the host response to initial RSV infection, leading to severe illness, and this alone may be sufficient to cause long-lasting changes to host immunity that predisposes to asthma in later life. In this proposed scenario, environmental factors that affect microbial colonization in the infant, such as maternal diet, cesarean delivery, transmission of microbial dysbiosis from the mother, maternal/infant antibiotic use, breast-feeding, as well as others will impact on the production of microbial metabolites and as a consequence affect the development and function of immune cells (e.g., DCs and Tregs) and structural cells (e.g., AECs) that in turn, control RSV immunopathogenicity.
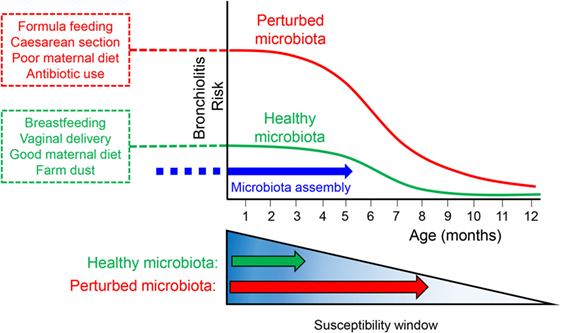
Figure 1. Schematic showing the timing of microbiota assembly with respect to bronchiolitis risk within the first year of life and how perturbations to the maternal or neonatal microbiota might prolong the window of susceptibility to bronchiolitis and increase overall risk.
Breast-Feeding and Asthma
As well as being the primary source of nutrition for the infant, breast milk contains immunomodulatory factors that affect the host immune response. The intestinal contents of breast-fed infants are acidic (pH 5.0), whereas those of non-breast fed infants is neutral (pH 7.1) (143). Milk oligosaccharides are the principal factor that support the growth of bifidobacteria (112) and hence may influence gastrointestinal and circulating SCFA levels in the infant. Thus, milk oligosaccharides likely serve as prebiotics to support the selective growth of “beneficial” bacteria, providing these commensals with a competitive advantage over potential pathogens. Milk oligosaccharides have also been postulated to exert direct anti-pathogenic and pro-tolerogenic roles in the intestine by acting as glycan receptor decoys for microbial adhesion factors and preventing pathogen attachment. Additionally, they can modulate the balance between Th1/Th2 immunity and provide essential nutrients for brain development and cognition [for a detailed review, see Ref. (112)]. Breast milk also contains a variety of food borne and aeroallergens that are transferred to the infant and influence sensitization to these antigens. In an elegant study by Verhasselt and colleagues, transforming growth factor-β transferred in breast milk was shown to facilitate T cell tolerance, protecting the offspring from OVA/alum-induced allergic airways disease (144). Birth cohort studies demonstrate that non-breast-fed infants are at greater risk of developing asthma (145–148), strongly implicating maternal factors in protecting against disease. Moreover, several epidemiological studies have linked a lack of breast-feeding to the severity of RSV infection (149–152), although this finding is not universal (153). Taken together, these studies demonstrate that breast milk represents a critical environmental determinant of colonization and profoundly influences immune homeostasis and postnatal development. However, the specific breast-milk derivatives and the causal pathways relating these to protection against respiratory pathogens and allergic sensitization in early-life require further investigation.
The Maternal Microbiota and Infant Respiratory Health
The composition of the maternal intestinal microbiota and the infant intestinal microbiota has been shown to affect the risk of infant wheeze (88), although the underling mechanisms have remained elusive. In an elegant study designed to address whether transient colonization during gestation alone can affect immune cell development and immune responses in the offspring, de Agüero and colleagues inoculated pregnant GF mice during late gestation with a bioengineered strain of Escherichia coli (HA107) that is cleared from the intestine within 72 h (154). Interestingly, colonization with E. coli during late-gestation led to a more competent intestinal immune system in the offspring, including increases in type-3 ILCs and F4/80+ CD11c+ mononuclear cells. This more physiologically normal cellular phenotype attenuated the overt inflammatory response observed in GF mice in response to microbial molecules and penetration of intestinal pathogens. Importantly, the protective effect of gestational colonization was not restricted to the gastrointestinal tract as the investigators demonstrated improved orchestration of the immune response within the spleen following intraperitoneal LPS administration. These intriguing data, albeit from an experimental system, suggest for the first time that the systemic immune responses of the offspring can be shaped by maternal gestational colonization alone. Whether similar processes operate to control the seeding of ILC subsets at other mucosal surfaces remain to be determined. The bidirectional signaling between type 2 ILCs and epithelial cells in the respiratory tract is important for tissue homeostasis and repair (155), and so it will be important to learn whether the maternal microbiota can influence lung barrier immunity to respiratory pathogens and other stimuli. Mechanistically, de Agüero et al. found that gestation-only colonization conferred protection in part through the generation of maternal antibodies. However, maternal antibody transfer alone was not entirely sufficient to mediate the positive effects of gestational E. coli on immunity, rather it assisted in the transfer of bacterial metabolites including aryl hydrocarbon receptor ligands, known to drive ILC3 expansion (156) and limit adult bacterial translocation across the intestinal epithelium. Consistent with the literature, the authors found that aryl hydrocarbon receptor ligand administration restored the levels of ILC3 in the gut, although other peripheral tissues were not assessed. The authors found no role for SCFAs, as exogenous administration of a butyrate, propionate and acetate mixture during pregnancy had no effect on the numbers of type-3 ILCs and mononuclear cells in the offspring’s intestine, although the in utero effects of SCFAs on other aspects of immune responsiveness in the offspring was not assessed.
The influence of the maternal microbiota and provision of various constituents in the breast milk must now implicate an important role for the maternal diet in the early development and training of the offspring’s immune system. Of relevance, a recent prospective birth cohort study of >56,000 Argentinian children under 2 years of age examined maternal dietary preferences and the impact on the children’s respiratory health (7). Of the 1,293 children who had a respiratory infection, >60% were infected with RSV, 22% with human RV, and 4% with influenza, highlighting RSV as the most significant pathogen affecting infant respiratory health. Intriguingly, when the maternal diet was high in carbohydrates and low in fruit and vegetables, this had profound effects on RSV-associated disease in the offspring, increasing the odds ratio of developing severe hypoxemia and life-threatening disease. The mechanisms underpinning this association remain unclear but might relate to alterations in the maternal microbiome and possibly fetal development. Most notably, it will be important to ascertain the relative contribution of elevated carbohydrate intake versus low fiber intake on disease outcome, i.e., was severe RSV-bronchiolitis caused by a lack of fiber and the resulting decrease immune cell-supportive microbial metabolites (e.g., SCFAs) or refined sugars promoting the outgrowth of bacterial pathogens or a combination of both.
Understanding the processes by which maternal diet influences asthma susceptibility is of crucial importance. As discussed above, feeding pregnant mice a high-fiber diet or SCFA-supplementation via the drinking water suppresses the development of allergic airway inflammation by increasing the suppressive activity of Treg cells via a HDAC9-dependent mechanism (131). Importantly, the suppressive effects of the maternal high-fiber diet were passed on to the offspring, indicating the epigenetic potential of SCFAs in the development of the immune system and in protection from asthma. In addition, feeding adult mice with a high-fiber diet or treating with exogenous propionate is protective against HDM-induced airway inflammation (129). Collectively, these data highlight the potential for modulating SCFA levels as a preventative strategy to protect against RSV-bronchiolitis and asthma.
Harnessing the Microbiota to Prevent and/or Treat Bronchiolitis
Immunological tolerance is an important goal for the prevention of asthma and allergic diseases and thus mechanisms to promote a healthy intestinal microbiota are under investigation (157–159). A new wave of Th2-targeted biologics has recently been approved for asthma (160); however, we speculate that this approach is unlikely to reverse the aberrantly programmed epithelium and/or immune response (61, 161). Microbial metabolites and beneficial commensals shape the immune response in early-life, suggesting that supplementing or mimicking these factors may represent a new approach for disease prevention. Of greater interest will be to determine whether such strategies can alter the course of disease or even reverse established asthma, a far greater challenge should the epigenetic programming of immune and structural cells already be set to an aberrant phenotype.
Prebiotics, Probiotics, and Synbiotics
In the 2013 update to the Cochrane Review, a meta-analysis was performed on two studies examining the effect of prebiotic treatment on asthma outcomes (162, 163). In the first study, Moro et al. reported a significant reduction in asthma outcome following treatment of 102 infants “at risk” of allergies with galacto-oligosaccharides and fructo-oligosaccharides (0.8 g/dL) compared to 104 treated with placebo. In the second report, Westerbeek et al. treated similarly high-risk infants with acidic and neutral oligosaccharides (20/80%; 1.2 g/L) and found no significant difference in asthma outcomes. The meta-analysis reported no significant effect of the prebiotic treatment overall, although significant heterogeneity was found between these reports. One possible explanation for the discrepant findings is the prebiotic employed and their respective effects on the infant microbiota. Although both of these oligosaccharides have been shown to increase bifidobacterial growth, their impact on other potential beneficial gut microbes remains to be fully determined. Moreover, in both studies prebiotic treatment began at approximately 6 months of age though microbial dysbiosis has been reported at 3 months of age in infants that go on to develop asthma in later childhood (134) and may commence even earlier, as described above. Thus, an earlier intervention that positively affects the assembly of the host microbiota is likely to be more successful. The use of prebiotics as therapeutics and preventatives is in its infancy; however, the nature and timing of these prebiotic strategies will improve as our knowledge of the composition of the human microbiota and its functional effects on host physiology and immune development increases.
A meta-analysis of four studies investigating effect of probiotics on established asthma found little or no benefit (164). However, the authors highlighted that marked heterogeneity among the studies made direct statistical comparisons difficult. This meta-analysis did not include a subsequently published study by Chen et al. (165). In this double-blinded, placebo-controlled trial, oral administration of Lactobacillus gasseri over a 5-week period was found to be effective in reducing bronchial hyperreactivity, improving lung function, and reducing day-time asthma symptoms in school-age (6–12 years) children with allergic asthma (165). L. gasseri treatment decreased the production of pro-inflammatory cytokines (e.g., TNF-α, IL-13) from phytohemagglutinin- or Derp1-stimulated peripheral blood mononuclear cells, although unfortunately Treg cells were not measured as part of the study. Similarly, oral inoculation with Lactobacillus rhamnosus or Lactobacillus reuteri protected against allergic airway inflammation in mice by increasing the number and function of Treg cells in the systemic circulation (158, 166, 167). In another study, oral administration of the bacterial preparation OM-85BV ameliorated the hallmark features of allergic asthma in mice (IgE, airway eosinophilia, and AHR) via the induction of gastrointestinal Treg cells. These suppressive Treg cells trafficked to the lung and inhibited airway mucosal CD86+ DCs, blunting the type 2 inflammatory response (168). In the context of allergic disease, few studies have evaluated the clinical efficacy of a probiotic in combination with prebiotic oligosaccharides (known as a synbiotic). One randomized trial examined 29 adults with asthma and HDM allergy treated with synbiotics (Bifidobacterium breve M-16V and a mixture of 90% short-chain galacto-oligosaccharides and 10% long-chain fructo-oligosaccharides) or placebo for 4 weeks followed by a bronchial challenge with HDM allergen (169). Although peak expiratory flow and systemic Th2 cytokines were reduced in the synbiotic-treated group, there was no effect on sputum eosinophilia or neutrophilia. These preliminary findings require replication by other investigators.
Interestingly, pre-treatment of mice with L. rhamnosus has also been shown to protect against pathogenic type 2 inflammation during RSV infection. This was associated with an increase in the production of IFN-β, IFN-γ, and IL-10 as well as both CD103+ and CD11b high DCs, although Treg cells were not assessed (170). A number of clinical studies have demonstrated the efficacy of prebiotic (171, 172) or probiotic interventions (172–175) for the treatment of respiratory viral infections. For example, in a randomized, placebo-controlled, double-blind trial, Luoto et al. investigated the efficacy of galacto-oligosaccharide and polydextrose mixture (1:1), known to promote bifidobacteria and lactobacilli, and the probiotic L. rhamnosus in a cohort of preterm infants in Finland. Strikingly, both the prebiotic mixture of galacto-oligosaccharide and polydextrose and L. rhamnosus significantly reduced the incidence of RV-induced episodes, which was the cause of the infection in 80% of the infants in the cohort. Although RSV-associated vLRIs were not affected by either treatment, the RSV group represented only a small proportion of the cohort, and therefore, larger studies will be required to determine the effect of synbiotics on RSV-bronchiolitis risk. Moreover, it will be important for longer term studies to assess whether decreasing the severity of vLRI in these children can protect from the development of asthma in later-life.
SCFAs and SCFA Mimetics
Human epidemiology and experimental studies in preclinical models of asthma suggest that dietary fiber protects against asthma risk by increasing fecal and systemic levels of SCFA. Accordingly, mimicking the anti-inflammatory effects of SCFAs pharmacologically is an attractive approach therapeutically. The anti-inflammatory effects of acetate and propionate have been ascribed to HDAC inhibition and the activation of GPR41 and/or GPR43 signaling, and as described above, these pathways ameliorate allergic airway inflammation in mice by increasing the function of Treg cells or by dampening pro-inflammatory DCs (15, 129, 131, 176). Although GPR109a, the primary receptor for butyrate, is reported to be expressed in lung tissue, its cellular distribution and function in the lung remains unclear (177). An additional target of SCFAs may be the bronchial epithelium, which expresses both GPR41 and GPR43, although increases in SCFA levels and GRP41 expression are proposed to be deleterious in the context of cystic fibrosis (133). Notably, recent pharmacological studies have reported development of small molecules with greater selectivity than endogenous SCFAs (140, 178), although increasing the potency of these compounds has yet to be achieved. Moreover, the efficacy of this current generation of molecules in animal models of asthma remains unexplored. Therefore, in addition to further elucidating the biology of these receptors, it will be important for drug discovery programs to continue to improve the selectivity and potency of synthetic agonists and to test their efficacy in clinically relevant models of viral bronchiolitis and asthma. pDCs present an attractive target for the prevention of severe RSV-bronchiolitis and asthma due to their capacity to produce vast amounts of type I IFN via engagement of TLRs (e.g., TLR7) and promote Treg cell expansion (179). We have shown that infection with pneumonia virus of mice (58), a mouse-specific Pneumovirus of the same genus as RSV, predisposes to severe bronchiolitis in Tlr7-deficient mice, while the adoptive transfer of Tlr7-sufficient pDC to Tlr7-deficient mice confers protection, implicating a critical role for TLR7 on pDCs. Moreover, secondary infection of TLR7-deficient mice induced the cardinal features of asthma, including AHR, airway remodeling (e.g., smooth muscle hyperplasia), and airway inflammation (180). Collectively, these findings suggest that the nexus between severe bronchiolitis and subsequent asthma is underpinned by perturbations to the pDC compartment (61). Hence, it will be important to determine whether SCFAs or SCFA mimetics are able to modulate pDC function.
Conclusion
Illuminating epidemiological, clinical, and preclinical studies suggest that the microbiome plays a critical role in protecting against asthma and allergies, particularly in early-life. Despite the fact that RSV-bronchiolitis predominantly affects infants under 6 months of age, the influence of gut microbial colonization and microbial metabolites on susceptibility to severe RSV-bronchiolitis has yet to be investigated. Programming of airway immune and structural cells likely occurs during this window of susceptibility and will have long-term consequences for susceptibility toward asthma-inducing stimuli. Identifying the molecular pathways that link the gut microbiota and the development of lung mucosal immunity has the potential to identify new therapeutic targets for the treatment of RSV-bronchiolitis. In view of the strong link between severe RSV-bronchiolitis and subsequent asthma, such a strategy has the potential to ameliorate the current high rates of allergic asthma in developed nations.
Author Contributions
JL performed background research and wrote the review. MS, BC, RW, and JS helped research the ancillary literature. PC, PD, and ME provided insightful discussion and edited the review. SP conceived the idea for the review and edited drafts.
Conflict of Interest Statement
The authors declare that the research was conducted in the absence of any commercial or financial relationships that could be construed as a potential conflict of interest.
Funding
SP has been funded by the National Health and Medical Research Council of Australia, the Australian Research Council Future Fellowship scheme, Pfizer, and GSK. All other authors declare that they have no competing financial interests.
References
1. Feldman AS, He Y, Moore ML, Hershenson MB, Hartert TV. Toward primary prevention of asthma. Reviewing the evidence for early-life respiratory viral infections as modifiable risk factors to prevent childhood asthma. Am J Respir Crit Care Med (2015) 191:34–44. doi: 10.1164/rccm.201405-0901PP
2. Holt PG, Sly PD. Viral infections and atopy in asthma pathogenesis: new rationales for asthma prevention and treatment. Nat Med (2012) 18:726–35. doi:10.1038/nm.2768
3. Holt PG, Sly PD. Interactions between RSV infection, asthma, and atopy: unraveling the complexities. J Exp Med (2002) 196:1271–5. doi:10.1084/jem.20021572
4. Lambrecht BN, Hammad H. The immunology of asthma. Nat Immunol (2015) 16:45–56. doi:10.1038/ni.3049
5. Holt PG, Strickland DH, Hales BJ, Sly PD. Defective respiratory tract immune surveillance in asthma: a primary causal factor in disease onset and progression. Chest (2014) 145:370–8. doi:10.1378/chest.13-1341
6. Caballero MT, Serra ME, Acosta PL, Marzec J, Gibbons L, Salim M, et al. TLR4 genotype and environmental LPS mediate RSV bronchiolitis through Th2 polarization. J Clin Invest (2015) 125:571–82. doi:10.1172/JCI75183
7. Ferolla FM, Hijano DR, Acosta PL, Rodriguez A, Duenas K, Sancilio A, et al. Macronutrients during pregnancy and life-threatening respiratory syncytial virus infections in children. Am J Respir Crit Care Med (2013) 187:983–90. doi:10.1164/rccm.201301-0016OC
8. Meissner HC. Viral bronchiolitis in children. N Engl J Med (2016) 374:62–72. doi:10.1056/NEJMra1413456
9. Yatsunenko T, Rey FE, Manary MJ, Trehan I, Dominguez-Bello MG, Contreras M, et al. Human gut microbiome viewed across age and geography. Nature (2012) 486:222–7. doi:10.1038/nature11053
10. Bokulich NA, Chung J, Battaglia T, Henderson N, Jay M, Li H, et al. Antibiotics, birth mode, and diet shape microbiome maturation during early life. Sci Transl Med (2016) 8:343ra82. doi:10.1126/scitranslmed.aad7121
11. Yassour M, Vatanen T, Siljander H, Hamalainen AM, Harkonen T, Ryhanen SJ, et al. Natural history of the infant gut microbiome and impact of antibiotic treatment on bacterial strain diversity and stability. Sci Transl Med (2016) 8:343ra81. doi:10.1126/scitranslmed.aad0917
12. Planer JD, Peng Y, Kau AL, Blanton LV, Ndao IM, Tarr PI, et al. Development of the gut microbiota and mucosal IgA responses in twins and gnotobiotic mice. Nature (2016) 534:263–6. doi:10.1038/nature17940
13. Koenig JE, Spor A, Scalfone N, Fricker AD, Stombaugh J, Knight R, et al. Succession of microbial consortia in the developing infant gut microbiome. Proc Natl Acad Sci U S A (2011) 108(Suppl 1):4578–85. doi:10.1073/pnas.1000081107
14. Hooper LV, Littman DR, Macpherson AJ. Interactions between the microbiota and the immune system. Science (2012) 336:1268–73. doi:10.1126/science.1223490
15. Arpaia N, Campbell C, Fan X, Dikiy S, Van Der Veeken J, Deroos P, et al. Metabolites produced by commensal bacteria promote peripheral regulatory T-cell generation. Nature (2013) 504:451–5. doi:10.1038/nature12726
16. Furusawa Y, Obata Y, Fukuda S, Endo TA, Nakato G, Takahashi D, et al. Commensal microbe-derived butyrate induces the differentiation of colonic regulatory T cells. Nature (2013) 504:446–50. doi:10.1038/nature12721
17. Mathewson ND, Jenq R, Mathew AV, Koenigsknecht M, Hanash A, Toubai T, et al. Gut microbiome-derived metabolites modulate intestinal epithelial cell damage and mitigate graft-versus-host disease. Nat Immunol (2016) 17:505–13. doi:10.1038/ni.3400
18. Desai MS, Seekatz AM, Koropatkin NM, Kamada N, Hickey CA, Wolter M, et al. A dietary fiber-deprived gut microbiota degrades the colonic mucus barrier and enhances pathogen susceptibility. Cell (2016) 167:1339.e–53.e. doi:10.1016/j.cell.2016.10.043
19. Beura LK, Hamilton SE, Bi K, Schenkel JM, Odumade OA, Casey KA, et al. Normalizing the environment recapitulates adult human immune traits in laboratory mice. Nature (2016) 532:512–6. doi:10.1038/nature17655
20. Hasegawa K, Mansbach JM, Ajami NJ, Petrosino JF, Freishtat RJ, Teach SJ, et al. Serum cathelicidin, nasopharyngeal microbiota, and disease severity among infants hospitalized with bronchiolitis. J Allergy Clin Immunol (2016). doi:10.1016/j.jaci.2016.09.037
21. Depner M, Ege MJ, Cox MJ, Dwyer S, Walker AW, Birzele LT, et al. Bacterial microbiota of the upper respiratory tract and childhood asthma. J Allergy Clin Immunol (2016). doi:10.1016/j.jaci.2016.05.050
22. Teo SM, Mok D, Pham K, Kusel M, Serralha M, Troy N, et al. The infant nasopharyngeal microbiome impacts severity of lower respiratory infection and risk of asthma development. Cell Host Microbe (2015) 17:704–15. doi:10.1016/j.chom.2015.03.008
23. Mansbach JM, Hasegawa K, Henke DM, Ajami NJ, Petrosino JF, Shaw CA, et al. Respiratory syncytial virus and rhinovirus severe bronchiolitis are associated with distinct nasopharyngeal microbiota. J Allergy Clin Immunol (2016) 137:1909–1913.e4. doi:10.1016/j.jaci.2016.01.036
24. Rosas-Salazar C, Shilts MH, Tovchigrechko A, Chappell JD, Larkin EK, Nelson KE, et al. Nasopharyngeal microbiome in respiratory syncytial virus resembles profile associated with increased childhood asthma risk. Am J Respir Crit Care Med (2016) 193:1180–3. doi:10.1164/rccm.201512-2350LE
25. Huang YJ, Nelson CE, Brodie EL, Desantis TZ, Baek MS, Liu J, et al. Airway microbiota and bronchial hyperresponsiveness in patients with suboptimally controlled asthma. J Allergy Clin Immunol (2011) 127:.e1–3. doi:10.1016/j.jaci.2010.10.048
26. Goleva E, Jackson LP, Harris JK, Robertson CE, Sutherland ER, Hall CF, et al. The effects of airway microbiome on corticosteroid responsiveness in asthma. Am J Respir Crit Care Med (2013) 188:1193–201. doi:10.1164/rccm.201304-0775OC
27. Durack J, Lynch SV, Nariya S, Bhakta NR, Beigelman A, Castro M, et al. Features of the bronchial bacterial microbiome associated with atopy, asthma and responsiveness to inhaled corticosteroid treatment. J Allergy Clin Immunol (2016). doi:10.1016/j.jaci.2016.08.055
28. Hilty M, Burke C, Pedro H, Cardenas P, Bush A, Bossley C, et al. Disordered microbial communities in asthmatic airways. PLoS One (2010) 5:e8578. doi:10.1371/journal.pone.0008578
29. Khetsuriani N, Kazerouni NN, Erdman DD, Lu X, Redd SC, Anderson LJ, et al. Prevalence of viral respiratory tract infections in children with asthma. J Allergy Clin Immunol (2007) 119:314–21. doi:10.1016/j.jaci.2006.08.041
30. Jackson DJ, Gangnon RE, Evans MD, Roberg KA, Anderson EL, Pappas TE, et al. Wheezing rhinovirus illnesses in early life predict asthma development in high-risk children. Am J Respir Crit Care Med (2008) 178:667–72. doi:10.1164/rccm.200802-309OC
31. Kusel MM, De Klerk NH, Kebadze T, Vohma V, Holt PG, Johnston SL, et al. Early-life respiratory viral infections, atopic sensitization, and risk of subsequent development of persistent asthma. J Allergy Clin Immunol (2007) 119:1105–10. doi:10.1016/j.jaci.2006.12.669
32. Kusel MM, Kebadze T, Johnston SL, Holt PG, Sly PD. Febrile respiratory illnesses in infancy and atopy are risk factors for persistent asthma and wheeze. Eur Respir J (2012) 39:876–82. doi:10.1183/09031936.00193310
33. Kusel MM, De Klerk NH, Holt PG, Kebadze T, Johnston SL, Sly PD. Role of respiratory viruses in acute upper and lower respiratory tract illness in the first year of life: a birth cohort study. Pediatr Infect Dis J (2006) 25:680–6. doi:10.1097/01.inf.0000226912.88900.a3
34. Johnston SL, Pattemore PK, Sanderson G, Smith S, Lampe F, Josephs L, et al. Community study of role of viral infections in exacerbations of asthma in 9-11 year old children. BMJ (1995) 310:1225–9. doi:10.1136/bmj.310.6989.1225
35. Sigurs N, Gustafsson PM, Bjarnason R, Lundberg F, Schmidt S, Sigurbergsson F, et al. Severe respiratory syncytial virus bronchiolitis in infancy and asthma and allergy at age 13. Am J Respir Crit Care Med (2005) 171:137–41. doi:10.1164/rccm.200406-730OC
36. Sigurs N, Bjarnason R, Sigurbergsson F, Kjellman B, Bjorksten B. Asthma and immunoglobulin E antibodies after respiratory syncytial virus bronchiolitis: a prospective cohort study with matched controls. J Pediatr (1995) 95:500–5.
37. Sigurs N, Bjarnason R, Sigurbergsson F, Kjellman B. Respiratory syncytial virus bronchiolitis in infancy is an important risk factor for asthma and allergy at age 7. Am J Respir Crit Care Med (2000) 161:1501–7. doi:10.1164/ajrccm.161.5.9906076
38. Sigurs N, Aljassim F, Kjellman B, Robinson PD, Sigurbergsson F, Bjarnason R, et al. Asthma and allergy patterns over 18 years after severe RSV bronchiolitis in the first year of life. Thorax (2010) 65:1045–52. doi:10.1136/thx.2009.121582
39. Stein RT, Sherrill D, Morgan WJ, Holberg CJ, Halonen M, Taussig LM, et al. Respiratory syncytial virus in early life and risk of wheeze and allergy by age 13 years. Lancet (1999) 354:541–5. doi:10.1016/S0140-6736(98)10321-5
40. Mok JY, Simpson H. Symptoms, atopy, and bronchial reactivity after lower respiratory infection in infancy. Arch Dis Child (1984) 59:299–305. doi:10.1136/adc.59.4.299
41. James KM, Gebretsadik T, Escobar GJ, Wu P, Carroll KN, Li SX, et al. Risk of childhood asthma following infant bronchiolitis during the respiratory syncytial virus season. J Allergy Clin Immunol (2013) 132:227–9. doi:10.1016/j.jaci.2013.01.009
42. Wu P, Dupont WD, Griffin MR, Carroll KN, Mitchel EF, Gebretsadik T, et al. Evidence of a causal role of winter virus infection during infancy in early childhood asthma. Am J Respir Crit Care Med (2008) 178:1123–9. doi:10.1164/rccm.200804-579OC
43. Zhu Z, Homer RJ, Wang Z, Chen Q, Geba GP, Wang J, et al. Pulmonary expression of interleukin-13 causes inflammation, mucus hypersecretion, subepithelial fibrosis, physiologic abnormalities, and eotaxin production. J Clin Invest (1999) 103:779–88. doi:10.1172/JCI5909
44. Johnston SL, Pattemore PK, Sanderson G, Smith S, Campbell MJ, Josephs LK, et al. The relationship between upper respiratory infections and hospital admissions for asthma: a time-trend analysis. Am J Respir Crit Care Med (1996) 154:654–60. doi:10.1164/ajrccm.154.3.8810601
45. Rawlinson WD, Waliuzzaman Z, Carter IW, Belessis YC, Gilbert KM, Morton JR. Asthma exacerbations in children associated with rhinovirus but not human metapneumovirus infection. J Infect Dis (2003) 187:1314–8. doi:10.1086/368411
46. Lodge CJ, Lowe AJ, Gurrin LC, Hill DJ, Hosking CS, Khalafzai RU, et al. House dust mite sensitization in toddlers predicts current wheeze at age 12 years. J Allergy Clin Immunol (2011) 128:782.e–8.e. doi:10.1016/j.jaci.2011.06.038
47. Hasegawa S, Hirano R, Hashimoto K, Haneda Y, Shirabe K, Ichiyama T. Characteristics of atopic children with pandemic H1N1 influenza viral infection: pandemic H1N1 influenza reveals ‘occult’ asthma of childhood. Pediatr Allergy Immunol (2011) 22:e119–23. doi:10.1111/j.1399-3038.2010.01090.x
48. Williams JV, Harris PA, Tollefson SJ, Halburnt-Rush LL, Pingsterhaus JM, Edwards KM, et al. Human metapneumovirus and lower respiratory tract disease in otherwise healthy infants and children. N Engl J Med (2004) 350:443–50. doi:10.1056/NEJMoa025472
49. Williams JV, Crowe JE, Enriquez R, Minton P, Peebles RS, Hamilton RG, et al. Human metapneumovirus infection plays an etiologic role in acute asthma exacerbations requiring hospitalization in adults. J Infect Dis (2005) 192:1149–53. doi:10.1086/444392
50. Jartti T, Van Den Hoogen B, Garofalo RP, Osterhaus AD, Ruuskanen O. Metapneumovirus and acute wheezing in children. Lancet (2002) 360:1393–4. doi:10.1016/S0140-6736(02)11391-2
51. Kotaniemi-Syrjanen A, Vainionpaa R, Reijonen TM, Waris M, Korhonen K, Korppi M. Rhinovirus-induced wheezing in infancy – the first sign of childhood asthma? J Allergy Clin Immunol (2003) 111:66–71. doi:10.1067/mai.2003.33
52. Escobar GJ, Ragins A, Li SX, Prager L, Masaquel AS, Kipnis P. Recurrent wheezing in the third year of life among children born at 32 weeks’ gestation or later: relationship to laboratory-confirmed, medically attended infection with respiratory syncytial virus during the first year of life. Arch Pediatr Adolesc Med (2010) 164:915–22. doi:10.1001/archpediatrics.2010.177
53. Lemanske RF Jr, Jackson DJ, Gangnon RE, Evans MD, Li Z, Shult PA, et al. Rhinovirus illnesses during infancy predict subsequent childhood wheezing. J Allergy Clin Immunol (2005) 116:571–7. doi:10.1016/j.jaci.2005.06.024
54. Blanken MO, Rovers MM, Molenaar JM, Winkler-Seinstra PL, Meijer A, Kimpen JLL, et al. Respiratory syncytial virus and recurrent wheeze in healthy preterm infants. N Engl J Med (2013) 368:1791–9. doi:10.1056/NEJMoa1211917
55. Simoes EA, Carbonell-Estrany X, Rieger CH, Mitchell I, Fredrick L, Groothuis JR, et al. The effect of respiratory syncytial virus on subsequent recurrent wheezing in atopic and nonatopic children. J Allergy Clin Immunol (2010) 126:256–62. doi:10.1016/j.jaci.2010.05.026
56. Chen CH, Lin YT, Yang YH, Wang LC, Lee JH, Kao CL, et al. Ribavirin for respiratory syncytial virus bronchiolitis reduced the risk of asthma and allergen sensitization. Pediatr Allergy Immunol (2008) 19:166–72. doi:10.1111/j.1399-3038.2007.00610.x
57. Kaiko GE, Loh Z, Spann K, Lynch JP, Lalwani A, Zheng Z, et al. Toll-like receptor 7 gene deficiency and early-life Pneumovirus infection interact to predispose toward the development of asthma-like pathology in mice. J Allergy Clin Immunol (2013) 131:1331.e–9.e. doi:10.1016/j.jaci.2013.02.041
58. Davidson S, Kaiko G, Loh Z, Lalwani A, Zhang V, Spann K, et al. Plasmacytoid dendritic cells promote host defense against acute pneumovirus infection via the TLR7-MyD88-dependent signaling pathway. J Immunol (2011) 186:5938–48. doi:10.4049/jimmunol.1002635
59. Lynch JP, Werder RB, Simpson J, Loh Z, Zhang V, Haque A, et al. Aeroallergen-induced IL-33 predisposes to respiratory virus-induced asthma by dampening antiviral immunity. J Allergy Clin Immunol (2016) 138:1326–37. doi:10.1016/j.jaci.2016.02.039
60. Spann KM, Loh Z, Lynch JP, Ullah A, Zhang V, Baturcam E, et al. IRF-3, IRF-7, and IPS-1 promote host defense against acute human metapneumovirus infection in neonatal mice. Am J Pathol (2014) 184:1795–806. doi:10.1016/j.ajpath.2014.02.026
61. Lynch JP, Mazzone SB, Rogers MJ, Arikkatt JJ, Loh Z, Pritchard AL, et al. The plasmacytoid dendritic cell: at the cross-roads in asthma. Eur Respir J (2014) 43:264–75. doi:10.1183/09031936.00203412
62. Willart MA, Deswarte K, Pouliot P, Braun H, Beyaert R, Lambrecht BN, et al. Interleukin-1alpha controls allergic sensitization to inhaled house dust mite via the epithelial release of GM-CSF and IL-33. J Exp Med (2012) 209:1505–17. doi:10.1084/jem.20112691
63. Ullah MA, Loh Z, Gan WJ, Zhang V, Yang H, Li JH, et al. Receptor for advanced glycation end products and its ligand high-mobility group box-1 mediate allergic airway sensitization and airway inflammation. J Allergy Clin Immunol (2014) 134(2):440–50. doi:10.1016/j.jaci.2013.12.1035
64. Halim TYF, Hwang YY, Scanlon ST, Zaghouani H, Garbi N, Fallon PG, et al. Group 2 innate lymphoid cells license dendritic cells to potentiate memory TH2 cell responses. Nat Immunol (2016) 17:57–64. doi:10.1038/ni.3294
65. De Kleer IM, Kool M, De Bruijn MJ, Willart M, van Moorleghem J, Schuijs MJ, et al. Perinatal activation of the interleukin-33 pathway promotes type 2 immunity in the developing lung. Immunity (2016) 45:1285–98. doi:10.1016/j.immuni.2016.10.031
66. Pritchard AL, Carroll ML, Burel JG, White OJ, Phipps S, Upham JW. Innate IFNs and plasmacytoid dendritic cells constrain Th2 cytokine responses to rhinovirus: a regulatory mechanism with relevance to asthma. J Immunol (2012) 188:5898–905. doi:10.4049/jimmunol.1103507
67. Kay AB, Phipps S, Robinson DS. A role for eosinophils in airway remodelling in asthma. Trends Immunol (2004) 25:477–82. doi:10.1016/j.it.2004.07.006
68. Allen JE, Wynn TA. Evolution of Th2 immunity: a rapid repair response to tissue destructive pathogens. PLoS Pathog (2011) 7:e1002003. doi:10.1371/journal.ppat.1002003
69. Palm NW, Rosenstein RK, Medzhitov R. Allergic host defences. Nature (2012) 484:465–72. doi:10.1038/nature11047
70. Oliphant CJ, Hwang YY, Walker JA, Salimi M, Wong SH, Brewer JM, et al. MHCII-mediated dialog between group 2 innate lymphoid cells and CD4 T cells potentiates type 2 immunity and promotes parasitic helminth expulsion. Immunity (2014) 41(2):283–95. doi:10.1016/j.immuni.2014.06.016
71. Guo L, Huang Y, Chen X, Hu-Li J, Urban JF Jr, Paul WE. Innate immunological function of TH2 cells in vivo. Nat Immunol (2015) 16:1051–9. doi:10.1038/ni.3244
72. Dickson RP, Erb-Downward JR, Martinez FJ, Huffnagle GB. The microbiome and the respiratory tract. Annu Rev Physiol (2016) 78:481–504. doi:10.1146/annurev-physiol-021115-105238
73. Gleeson K, Eggli DF, Maxwell SL. Quantitative aspiration during sleep in normal subjects. Chest (1997) 111:1266–72. doi:10.1378/chest.111.5.1266
74. Wilson MT, Hamilos DL. The nasal and sinus microbiome in health and disease. Curr Allergy Asthma Rep (2014) 14:485. doi:10.1007/s11882-014-0485-x
75. Huxley EJ, Viroslav J, Gray WR, Pierce AK. Pharyngeal aspiration in normal adults and patients with depressed consciousness. Am J Med (1978) 64:564–8. doi:10.1016/0002-9343(78)90574-0
76. Zhang Q, Cox M, Liang Z, Brinkmann F, Cardenas PA, Duff R, et al. Airway microbiota in severe asthma and relationship to asthma severity and phenotypes. PLoS One (2016) 11:e0152724. doi:10.1371/journal.pone.0152724
77. Green BJ, Wiriyachaiporn S, Grainge C, Rogers GB, Kehagia V, Lau L, et al. Potentially pathogenic airway bacteria and neutrophilic inflammation in treatment resistant severe asthma. PLoS One (2014) 9:e100645. doi:10.1371/journal.pone.0100645
78. Simpson JL, Daly J, Baines KJ, Yang IA, Upham JW, Reynolds PN, et al. Airway dysbiosis: Haemophilus influenzae and Tropheryma in poorly controlled asthma. Eur Respir J (2016) 47:792–800. doi:10.1183/13993003.00405-2015
79. Nicholson KG, Kent J, Ireland DC. Respiratory viruses and exacerbations of asthma in adults. BMJ (1993) 307:982–6. doi:10.1136/bmj.307.6910.982
80. Hofstra JJ, Matamoros S, Van De Pol MA, De Wever B, Tanck MW, Wendt-Knol H, et al. Changes in microbiota during experimental human Rhinovirus infection. BMC Infect Dis (2015) 15:336. doi:10.1186/s12879-015-1081-y
81. Kloepfer KM, Lee WM, Pappas TE, Kang TJ, Vrtis RF, Evans MD, et al. Detection of pathogenic bacteria during rhinovirus infection is associated with increased respiratory symptoms and asthma exacerbations. J Allergy Clin Immunol (2014) 133:.e1–3. doi:10.1016/j.jaci.2014.02.030
82. Bisgaard H, Hermansen MN, Buchvald F, Loland L, Halkjaer LB, Bønnelykke K, et al. Childhood asthma after bacterial colonization of the airway in neonates. N Engl J Med (2007) 357:1487–95. doi:10.1056/NEJMoa052632
83. Bisgaard H, Hermansen MN, Bønnelykke K, Stokholm J, Baty F, Skytt NL, et al. Association of bacteria and viruses with wheezy episodes in young children: prospective birth cohort study. BMJ (2010) 341:c4978. doi:10.1136/bmj.c4978
84. Von Mutius E. Of attraction and rejection – asthma and the microbial world. N Engl J Med (2007) 357:1545–7. doi:10.1056/NEJMe078119
85. Penders J, Kummeling I, Thijs C. Infant antibiotic use and wheeze and asthma risk: a systematic review and meta-analysis. Eur Respir J (2011) 38:295–302. doi:10.1183/09031936.00105010
86. Rollins DR, Beuther DA, Martin RJ. Update on infection and antibiotics in asthma. Curr Allergy Asthma Rep (2010) 10:67–73. doi:10.1007/s11882-009-0086-2
87. Marra F, Lynd L, Coombes M, Richardson K, Legal M, Fitzgerald JM, et al. Does antibiotic exposure during infancy lead to development of asthma? A systematic review and metaanalysis. Chest (2006) 129:610–8. doi:10.1378/chest.129.3.610
88. Lange NE, Celedón JC, Forno E, Ly NP, Onderdonk A, Bry L, et al. Maternal intestinal flora and wheeze in early childhood. Clin Exp Allergy (2012) 42:901–8. doi:10.1111/j.1365-2222.2011.03950.x
89. Yang B, Liu R, Yang T, Jiang X, Zhang L, Wang L, et al. Neonatal Streptococcus pneumoniae infection may aggravate adulthood allergic airways disease in association with IL-17A. PLoS One (2015) 10:e0123010. doi:10.1371/journal.pone.0123010
90. Raiden S, Pandolfi J, Payasliàn F, Anderson M, Rivarola N, Ferrero F, et al. Depletion of circulating regulatory T cells during severe respiratory syncytial virus infection in young children. Am J Respir Crit Care Med (2014) 189:865–8. doi:10.1164/rccm.201311-1977LE
91. Durant LR, Makris S, Voorburg CM, Loebbermann J, Johansson C, Openshaw PJ. Regulatory T cells prevent Th2 immune responses and pulmonary eosinophilia during respiratory syncytial virus infection in mice. J Virol (2013) 87:10946–54. doi:10.1128/JVI.01295-13
92. Brockson ME, Novotny LA, Jurcisek JA, Mcgillivary G, Bowers MR, Bakaletz LO. Respiratory syncytial virus promotes Moraxella catarrhalis-induced ascending experimental otitis media. PLoS One (2012) 7:e40088. doi:10.1371/journal.pone.0040088
93. Green CA, Yeates D, Goldacre A, Sande C, Parslow RC, Mcshane P, et al. Admission to hospital for bronchiolitis in England: trends over five decades, geographical variation and association with perinatal characteristics and subsequent asthma. Arch Dis Child (2015) 101(2):140–6. doi:10.1136/archdischild-2015-308723
94. Adcock IM, Caramori G, Chung KF. New targets for drug development in asthma. Lancet (2008) 372:1073–87. doi:10.1016/S0140-6736(08)61449-X
95. Eder W, Ege MJ, Erika Von M. Current concepts: the asthma epidemic. N Engl J Med (2006) 355:2226–35. doi:10.1056/NEJMra054308
96. Pearce N, Aït-Khaled N, Beasley R, Mallol J, Keil U, Mitchell E, et al. Worldwide trends in the prevalence of asthma symptoms: phase III of the International Study of Asthma and Allergies in Childhood (ISAAC). Thorax (2007) 62:758–66. doi:10.1136/thx.2006.070169
97. Strachan DP. Hay fever, hygiene, and household size. BMJ (1989) 299:1259–60. doi:10.1136/bmj.299.6710.1259
98. Strachan DP. Family size, infection and atopy: the first decade of the “hygiene hypothesis”. Thorax (2000) 55(Suppl 1):S2–10. doi:10.1136/thorax.55.suppl_1.S2
99. Riedler J, Braun-Fahrlander C, Eder W, Schreuer M, Waser M, Maisch S, et al. Exposure to farming in early life and development of asthma and allergy: a cross-sectional survey. Lancet (2001) 358:1129–33. doi:10.1016/S0140-6736(01)06252-3
100. Braun-Fahrlander C, Riedler J, Herz U, Eder W, Waser M, Grize L, et al. Environmental exposure to endotoxin and its relation to asthma in school-age children. N Engl J Med (2002) 347:869–77. doi:10.1056/NEJMoa020057
101. Gereda JE, Leung DY, Liu AH. Levels of environmental endotoxin and prevalence of atopic disease. JAMA (2000) 284:1652–3. doi:10.1001/jama.284.13.1647
102. Genuneit J, Strachan DP, Buchele G, Weber J, Loss G, Sozanska B, et al. The combined effects of family size and farm exposure on childhood hay fever and atopy. Pediatr Allergy Immunol (2013) 24:293–8. doi:10.1111/pai.12053
103. Stein MM, Hrusch CL, Gozdz J, Igartua C, Pivniouk V, Murray SE, et al. Innate immunity and asthma risk in Amish and Hutterite farm children. N Engl J Med (2016) 375:411–21. doi:10.1056/NEJMoa1508749
104. Schuijs MJ, Willart MA, Vergote K, Gras D, Deswarte K, Ege MJ, et al. Farm dust and endotoxin protect against allergy through A20 induction in lung epithelial cells. Science (2015) 349:1106–10. doi:10.1126/science.aac6623
105. Ownby DR, Johnson CC, Peterson EL. Exposure to dogs and cats in the first year of life and risk of allergic sensitization at 6 to 7 years of age. JAMA (2002) 288:963–72. doi:10.1001/jama.288.8.963
106. Hesselmar B, Aberg N, Aberg B, Eriksson B, Bjorksten B. Does early exposure to cat or dog protect against later allergy development? Clin Exp Allergy (1999) 29:611–7. doi:10.1046/j.1365-2222.1999.00534.x
107. Gereda JE, Leung DY, Thatayatikom A, Streib JE, Price MR, Klinnert MD, et al. Relation between house-dust endotoxin exposure, type 1 T-cell development, and allergen sensitisation in infants at high risk of asthma. Lancet (2000) 355:1680–3. doi:10.1016/S0140-6736(00)02239-X
108. Fujimura KE, Johnson CC, Ownby DR, Cox MJ, Brodie EL, Havstad SL, et al. Man’s best friend? The effect of pet ownership on house dust microbial communities. J Allergy Clin Immunol (2010) 126:410–2. doi:10.1016/j.jaci.2010.05.042
109. Gravesen S. Fungi as a cause of allergic disease. Allergy (1979) 34:135–54. doi:10.1111/j.1398-9995.1979.tb01562.x
110. Pantoja-Feliciano IG, Clemente JC, Costello EK, Perez ME, Blaser MJ, Knight R, et al. Biphasic assembly of the murine intestinal microbiota during early development. ISME J (2013) 7:1112–5. doi:10.1038/ismej.2013.15
111. Dominguez-Bello MG, Costello EK, Contreras M, Magris M, Hidalgo G, Fierer N, et al. Delivery mode shapes the acquisition and structure of the initial microbiota across multiple body habitats in newborns. Proc Natl Acad Sci U S A (2010) 107:11971–5. doi:10.1073/pnas.1002601107
112. Bode L. Human milk oligosaccharides: every baby needs a sugar mama. Glycobiology (2012) 22:1147–62. doi:10.1093/glycob/cws074
113. Mueller N, Pizoni A, Goldani H, Werlang I, Matte U, Goldani M, et al. Delivery mode and neonate gut microbiota. FASEB J (2015) 29. doi:10.1096/fj.1530-6860
114. Guaraldi F, Salvatori G. Effect of breast and formula feeding on gut microbiota shaping in newborns. Front Cell Infect Microbiol (2012) 2:94. doi:10.3389/fcimb.2012.00094
115. Jackson KM, Nazar AM. Breastfeeding, the immune response, and long-term health. J Am Osteopath Assoc (2006) 106:203–7.
116. Blaser MJ, Dominguez-Bello MG. The human microbiome before birth. Cell Host Microbe (2016) 20:558–60. doi:10.1016/j.chom.2016.10.014
117. Salzman NH, Ghosh D, Huttner KM, Paterson Y, Bevins CL. Protection against enteric salmonellosis in transgenic mice expressing a human intestinal defensin. Nature (2003) 422:522–6. doi:10.1038/nature01520
118. Vaishnava S, Behrendt CL, Ismail AS, Eckmann L, Hooper LV. Paneth cells directly sense gut commensals and maintain homeostasis at the intestinal host-microbial interface. Proc Natl Acad Sci U S A (2008) 105:20858–63. doi:10.1073/pnas.0808723105
119. Bauer H, Horowitz RE, Levenson SM, Popper H. The response of the lymphatic tissue to the microbial flora. Studies on germfree mice. Am J Pathol (1963) 42:471–83.
120. Olszak T, An D, Zeissig S, Vera MP, Richter J, Franke A, et al. Microbial exposure during early life has persistent effects on natural killer T cell function. Science (2012) 336:489–93. doi:10.1126/science.1219328
121. Julia V, Macia L, Dombrowicz D. The impact of diet on asthma and allergic diseases. Nat Rev Immunol (2015) 15:308–22. doi:10.1038/nri3830
122. Tannock GW. Analysis of the intestinal microflora using molecular methods. Eur J Clin Nutr (2002) 56(Suppl 4):S44–9. doi:10.1038/sj.ejcn.1601661
123. Hoverstad T, Carlstedt-Duke B, Lingaas E, Midtvedt T, Norin KE, Saxerholt H, et al. Influence of ampicillin, clindamycin, and metronidazole on faecal excretion of short-chain fatty acids in healthy subjects. Scand J Gastroenterol (1986) 21:621–6. doi:10.3109/00365528608996411
124. Vinolo MA, Rodrigues HG, Hatanaka E, Sato FT, Sampaio SC, Curi R. Suppressive effect of short-chain fatty acids on production of proinflammatory mediators by neutrophils. J Nutr Biochem (2011) 22:849–55. doi:10.1016/j.jnutbio.2010.07.009
125. Usami M, Kishimoto K, Ohata A, Miyoshi M, Aoyama M, Fueda Y, et al. Butyrate and trichostatin A attenuate nuclear factor kappaB activation and tumor necrosis factor alpha secretion and increase prostaglandin E2 secretion in human peripheral blood mononuclear cells. Nutr Res (2008) 28:321–8. doi:10.1016/j.nutres.2008.02.012
126. Kendrick SF, O’BOYLE G, Mann J, Zeybel M, Palmer J, Jones DE, et al. Acetate, the key modulator of inflammatory responses in acute alcoholic hepatitis. Hepatology (2010) 51:1988–97. doi:10.1002/hep.23572
127. Chang PV, Hao L, Offermanns S, Medzhitov R. The microbial metabolite butyrate regulates intestinal macrophage function via histone deacetylase inhibition. Proc Natl Acad Sci U S A (2014) 111:2247–52. doi:10.1073/pnas.1322269111
128. Singh N, Thangaraju M, Prasad PD, Martin PM, Lambert NA, Boettger T, et al. Blockade of dendritic cell development by bacterial fermentation products butyrate and propionate through a transporter (Slc5a8)-dependent inhibition of histone deacetylases. J Biol Chem (2010) 285:27601–8. doi:10.1074/jbc.M110.102947
129. Trompette A, Gollwitzer ES, Yadava K, Sichelstiel AK, Sprenger N, Ngom-Bru C, et al. Gut microbiota metabolism of dietary fiber influences allergic airway disease and hematopoiesis. Nat Med (2014) 20(2):159–66. doi:10.1038/nm.3444
130. Tao R, De Zoeten EF, Ozkaynak E, Chen C, Wang L, Porrett PM, et al. Deacetylase inhibition promotes the generation and function of regulatory T cells. Nat Med (2007) 13:1299–307. doi:10.1038/nm1652
131. Thorburn AN, Mckenzie CI, Shen S, Stanley D, Macia L, Mason LJ, et al. Evidence that asthma is a developmental origin disease influenced by maternal diet and bacterial metabolites. Nat Commun (2015) 6:7320. doi:10.1038/ncomms8320
132. Smith PM, Howitt MR, Panikov N, Michaud M, Gallini CA, Bohlooly YM, et al. The microbial metabolites, short-chain fatty acids, regulate colonic Treg cell homeostasis. Science (2013) 341:569–73. doi:10.1126/science.1241165
133. Mirkovic B, Murray MA, Lavelle GM, Molloy K, Azim AA, Gunaratnam C, et al. The role of short-chain fatty acids, produced by anaerobic bacteria, in the cystic fibrosis airway. Am J Respir Crit Care Med (2015) 192:1314–24. doi:10.1164/rccm.201505-0943OC
134. Arrieta MC, Stiemsma LT, Dimitriu PA, Thorson L, Russell S, Yurist-Doutsch S, et al. Early infancy microbial and metabolic alterations affect risk of childhood asthma. Sci Transl Med (2015) 7:307ra152. doi:10.1126/scitranslmed.aab2271
135. Abrahamsson TR, Jakobsson HE, Andersson AF, Bjorksten B, Engstrand L, Jenmalm MC. Low gut microbiota diversity in early infancy precedes asthma at school age. Clin Exp Allergy (2014) 44:842–50. doi:10.1111/cea.12253
136. Pluznick JL, Protzko RJ, Gevorgyan H, Peterlin Z, Sipos A, Han J, et al. Olfactory receptor responding to gut microbiota-derived signals plays a role in renin secretion and blood pressure regulation. Proc Natl Acad Sci U S A (2013) 110:4410–5. doi:10.1073/pnas.1215927110
137. Brown AJ, Goldsworthy SM, Barnes AA, Eilert MM, Tcheang L, Daniels D, et al. The orphan G protein-coupled receptors GPR41 and GPR43 are activated by propionate and other short chain carboxylic acids. J Biol Chem (2003) 278:11312–9. doi:10.1074/jbc.M211609200
138. Le Poul E, Loison C, Struyf S, Springael JY, Lannoy V, Decobecq ME, et al. Functional characterization of human receptors for short chain fatty acids and their role in polymorphonuclear cell activation. J Biol Chem (2003) 278:25481–9. doi:10.1074/jbc.M301403200
139. Nilsson NE, Kotarsky K, Owman C, Olde B. Identification of a free fatty acid receptor, FFA2R, expressed on leukocytes and activated by short-chain fatty acids. Biochem Biophys Res Commun (2003) 303:1047–52. doi:10.1016/S0006-291X(03)00488-1
140. Schmidt J, Smith NJ, Christiansen E, Tikhonova IG, Grundmann M, Hudson BD, et al. Selective orthosteric free fatty acid receptor 2 (FFA2) agonists: identification of the structural and chemical requirements for selective activation of FFA2 versus FFA3. J Biol Chem (2011) 286:10628–40. doi:10.1074/jbc.M110.210872
141. Xiong Y, Miyamoto N, Shibata K, Valasek MA, Motoike T, Kedzierski RM, et al. Short-chain fatty acids stimulate leptin production in adipocytes through the G protein-coupled receptor GPR41. Proc Natl Acad Sci U S A (2004) 101:1045–50. doi:10.1073/pnas.2637002100
142. Taggart AK, Kero J, Gan X, Cai TQ, Cheng K, Ippolito M, et al. (d)-beta-Hydroxybutyrate inhibits adipocyte lipolysis via the nicotinic acid receptor PUMA-G. J Biol Chem (2005) 280:26649–52. doi:10.1074/jbc.C500213200
143. Yoshioka H, Iseki K, Fujita K. Development and differences of intestinal flora in the neonatal period in breast-fed and bottle-fed infants. Pediatrics (1983) 72:317–21.
144. Verhasselt V, Milcent V, Cazareth J, Kanda A, Fleury S, Dombrowicz D, et al. Breast milk-mediated transfer of an antigen induces tolerance and protection from allergic asthma. Nat Med (2008) 14:170–5. doi:10.1038/nm1718
145. Scholtens S, Wijga AH, Brunekreef B, Kerkhof M, Hoekstra MO, Gerritsen J, et al. Breast feeding, parental allergy and asthma in children followed for 8 years. The PIAMA Birth Cohort Study. Thorax (2009) 64:604–9. doi:10.1136/thx.2007.094938
146. Oddy WH, De Klerk NH, Sly PD, Holt PG. The effects of respiratory infections, atopy, and breastfeeding on childhood asthma. Eur Respir J (2002) 19:899–905. doi:10.1183/09031936.02.00103602
147. Oddy WH, Holt PG, Sly PD, Read AW, Landau LI, Stanley FJ, et al. Association between breast feeding and asthma in 6 year old children: findings of a prospective birth cohort study. BMJ (1999) 319:815–9. doi:10.1136/bmj.319.7213.815
148. Bachrach VRG, Schwarz E, Bachrach LR. Breastfeeding and the risk of hospitalization for respiratory disease in infancy – a meta-analysis. Arch Pediatr Adolesc Med (2003) 157:237–43. doi:10.1001/archpedi.157.3.237
149. Cushing AH, Samet JM, Lambert WE, Skipper BJ, Hunt WC, Young SA, et al. Breastfeeding reduces risk of respiratory illness in infants. Am J Epidemiol (1998) 147:863–70. doi:10.1093/oxfordjournals.aje.a009540
150. Wright AL, Holberg CJ, Martinez FD, Morgan WJ, Taussig LM. Breast feeding and lower respiratory tract illness in the first year of life. Group Health Medical Associates. BMJ (1989) 299:946–9. doi:10.1136/bmj.299.6705.946
151. Nishimura T, Suzue J, Kaji H. Breastfeeding reduces the severity of respiratory syncytial virus infection among young infants: a multi-center prospective study. Pediatr Int (2009) 51:812–6. doi:10.1111/j.1442-200X.2009.02877.x
152. Downham MA, Scott R, Sims DG, Webb JK, Gardner PS. Breast-feeding protects against respiratory syncytial virus infections. Br Med J (1976) 2:274–6. doi:10.1136/bmj.2.6030.274
153. Simoes EAF. Environmental and demographic risk factors for respiratory syncytial virus lower respiratory tract disease. J Pediatr (2003) 143:118–26. doi:10.1067/S0022-3476(03)00511-0
154. Hapfelmeier S, Lawson MA, Slack E, Kirundi JK, Stoel M, Heikenwalder M, et al. Reversible microbial colonization of germ-free mice reveals the dynamics of IgA immune responses. Science (2010) 328:1705–9. doi:10.1126/science.1188454
155. Sonnenberg GF, Artis D. Innate lymphoid cells in the initiation, regulation and resolution of inflammation. Nat Med (2015) 21:698–708. doi:10.1038/nm.3892
156. Kiss EA, Vonarbourg C, Kopfmann S, Hobeika E, Finke D, Esser C, et al. Natural aryl hydrocarbon receptor ligands control organogenesis of intestinal lymphoid follicles. Science (2011) 334:1561–5. doi:10.1126/science.1214914
157. Hunt JR, Martinelli R, Adams VC, Rook GA, Brunet LR. Intragastric administration of Mycobacterium vaccae inhibits severe pulmonary allergic inflammation in a mouse model. Clin Exp Allergy (2005) 35:685–90. doi:10.1111/j.1365-2222.2005.02239.x
158. Forsythe P, Inman MD, Bienenstock J. Oral treatment with live Lactobacillus reuteri inhibits the allergic airway response in mice. Am J Respir Crit Care Med (2007) 175:561–9. doi:10.1164/rccm.200606-821OC
159. Atarashi K, Tanoue T, Shima T, Imaoka A, Kuwahara T, Momose Y, et al. Induction of colonic regulatory T cells by indigenous Clostridium species. Science (2011) 331:337–41. doi:10.1126/science.1198469
160. Fajt ML, Wenzel SE. Asthma phenotypes and the use of biologic medications in asthma and allergic disease: the next steps toward personalized care. J Allergy Clin Immunol (2015) 135:299–310. doi:10.1016/j.jaci.2014.12.1871
161. Lynch JP, Ferreira MA, Phipps S. Th2/Th17 reciprocal regulation: twists and turns in the complexity of asthma phenotypes. Ann Transl Med (2016) 4(1):S59. doi:10.21037/atm.2016.10.69
162. Moro G, Arslanoglu S, Stahl B, Jelinek J, Wahn U, Boehm G. A mixture of prebiotic oligosaccharides reduces the incidence of atopic dermatitis during the first six months of age. Arch Dis Child (2006) 91:814–9. doi:10.1136/adc.2006.098251
163. Westerbeek EA, Van Den Berg A, Lafeber HN, Fetter WP, Van Elburg RM. The effect of enteral supplementation of a prebiotic mixture of non-human milk galacto-, fructo- and acidic oligosaccharides on intestinal permeability in preterm infants. Br J Nutr (2011) 105:268–74. doi:10.1017/S0007114510003405
164. Vliagoftis H, Kouranos VD, Betsi GI, Falagas ME. Probiotics for the treatment of allergic rhinitis and asthma: systematic review of randomized controlled trials. Ann Allergy Asthma Immunol (2008) 101:570–9. doi:10.1016/S1081-1206(10)60219-0
165. Chen YS, Jan RL, Lin YL, Chen HH, Wang JY. Randomized placebo-controlled trial of Lactobacillus on asthmatic children with allergic rhinitis. Pediatr Pulmonol (2010) 45:1111–20. doi:10.1002/ppul.21296
166. Karimi K, Inman MD, Bienenstock J, Forsythe P. Lactobacillus reuteri-induced regulatory T cells protect against an allergic airway response in mice. Am J Respir Crit Care Med (2009) 179:186–93. doi:10.1164/rccm.200806-951OC
167. Jang S-O, Kim H-J, Kim Y-J, Kang M-J, Kwon J-W, Seo J-H, et al. Asthma prevention by Lactobacillus rhamnosus in a mouse model is associated with CD4(+)CD25(+)Foxp3(+) T cells. Allergy Asthma Immunol Res (2012) 4:150–6. doi:10.4168/aair.2012.4.3.150
168. Strickland DH, Judd S, Thomas JA, Larcombe AN, Sly PD, Holt PG. Boosting airway T-regulatory cells by gastrointestinal stimulation as a strategy for asthma control. Mucosal Immunol (2011) 4:43–52. doi:10.1038/mi.2010.43
169. Van De Pol MA, Lutter R, Smids BS, Weersink EJ, Van Der Zee JS. Synbiotics reduce allergen-induced T-helper 2 response and improve peak expiratory flow in allergic asthmatics. Allergy (2011) 66:39–47. doi:10.1111/j.1398-9995.2010.02454.x
170. Chiba E, Tomosada Y, Vizoso-Pinto MG, Salva S, Takahashi T, Tsukida K, et al. Immunobiotic Lactobacillus rhamnosus improves resistance of infant mice against respiratory syncytial virus infection. Int Immunopharmacol (2013) 17:373–82. doi:10.1016/j.intimp.2013.06.024
171. Arslanoglu S, Moro GE, Boehm G. Early supplementation of prebiotic oligosaccharides protects formula-fed infants against infections during the first 6 months of life. J Nutr (2007) 137:2420–4.
172. Luoto R, Ruuskanen O, Waris M, Kalliomäki M, Salminen S, Isolauri E. Prebiotic and probiotic supplementation prevents rhinovirus infections in preterm infants: a randomized, placebo-controlled trial. J Allergy ClinImmunol (2014) 133:405–13. doi:10.1016/j.jaci.2013.08.020
173. Rautava S, Salminen S, Isolauri E. Specific probiotics in reducing the risk of acute infections in infancy – a randomised, double-blind, placebo-controlled study. Br J Nutr (2009) 101:1722–6. doi:10.1017/S0007114508116282
174. Taipale T, Pienihakkinen K, Isolauri E, Larsen C, Brockmann E, Alanen P, et al. Bifidobacterium animalis subsp. lactis BB-12 in reducing the risk of infections in infancy. Br J Nutr (2011) 105:409–16. doi:10.1017/S0007114510003685
175. Maldonado J, Canabate F, Sempere L, Vela F, Sanchez AR, Narbona E, et al. Human milk probiotic Lactobacillus fermentum CECT5716 reduces the incidence of gastrointestinal and upper respiratory tract infections in infants. J Pediatr Gastroenterol Nutr (2012) 54:55–61. doi:10.1097/MPG.0b013e3182333f18
176. Maslowski KM, Vieira AT, Ng A, Kranich J, Sierro F, Yu D, et al. Regulation of inflammatory responses by gut microbiota and chemoattractant receptor GPR43. Nature (2009) 461:1282–6. doi:10.1038/nature08530
177. Wise A, Foord SM, Fraser NJ, Barnes AA, Elshourbagy N, Eilert M, et al. Molecular identification of high and low affinity receptors for nicotinic acid. J Biol Chem (2003) 278:9869–74. doi:10.1074/jbc.M210695200
178. Ulven T. Short-chain free fatty acid receptors FFA2/GPR43 and FFA3/GPR41 as new potential therapeutic targets. Front Endocrinol (2012) 3:111. doi:10.3389/fendo.2012.00111
179. Palomares O, Ruckert B, Jartti T, Kucuksezer UC, Puhakka T, Gomez E, et al. Induction and maintenance of allergen-specific FOXP3+ Treg cells in human tonsils as potential first-line organs of oral tolerance. J Allergy Clin Immunol (2012) 129:510–20. doi:10.1016/j.jaci.2011.09.031
Keywords: viral lower respiratory tract infection, RSV, asthma, microbiome, microbiota, microbiota and immunity, PVM
Citation: Lynch JP, Sikder MAA, Curren BF, Werder RB, Simpson J, Cuív PÓ, Dennis PG, Everard ML and Phipps S (2017) The Influence of the Microbiome on Early-Life Severe Viral Lower Respiratory Infections and Asthma—Food for Thought? Front. Immunol. 8:156. doi: 10.3389/fimmu.2017.00156
Received: 13 December 2016; Accepted: 30 January 2017;
Published: 16 February 2017
Edited by:
Catherine Thornton, Swansea University, UKReviewed by:
Shi Yue, University of Southern California, USASwapna Mahurkar-Joshi, University of California Los Angeles, USA
Copyright: © 2017 Lynch, Sikder, Curren, Werder, Simpson, Cuív, Dennis, Everard and Phipps. This is an open-access article distributed under the terms of the Creative Commons Attribution License (CC BY). The use, distribution or reproduction in other forums is permitted, provided the original author(s) or licensor are credited and that the original publication in this journal is cited, in accordance with accepted academic practice. No use, distribution or reproduction is permitted which does not comply with these terms.
*Correspondence: Simon Phipps, cy5waGlwcHMmI3gwMDA0MDt1cS5lZHUuYXU=