- Immunology Programme, Department of Microbiology and Immunology, Yoon Loo Lin School of Medicine, Life Science Institute, National University of Singapore, Singapore, Singapore
Lymphatic vessels have been traditionally considered as passive transporters of fluid and lipids. However, it is apparent from recent literature that the function of lymphatic vessels is not only restricted to fluid balance homeostasis but also extends to regulation of immune cell trafficking, antigen presentation, tolerance, and immunity, all which may impact the progression of inflammatory responses and diseases such as cancer. The lymphatic system and the immune system are intimately connected, and there is emergent evidence for a crosstalk between T cell and lymphatic endothelial cell (LEC). This review describes how LECs in lymph nodes can affect multiple functional properties of T cells and the impact of these LEC-driven effects on adaptive immunity and, conversely, how T cells can modulate LEC growth. The significance of such crosstalk between T cells and LECs in cancer will also be discussed.
Lymph Node (LN) Architecture
Lymph nodes are strategically positioned and highly organized organs that serve as “rendez-vous” points for dendritic cells (DCs), T cells, and B cells. The maintenance of LN structure and compartmentalization are essential for the elicitation and development of effective immune response. LN can be subdivided into three main regions, namely, the cortex, the paracortex, and the medulla. Encapsulated LNs receive lymph from peripheral tissue and organs through the afferent lymphatic. Molecules, antigens, microorganisms, and cells such as lymphocytes and antigen-presenting cells (APCs) within the lymph are emptied into to the subcapsular sinus (SCS) of the LN. Subcapsular and medullary sinuses are directly interconnected, and hence, lymph-borne cells, fluid, and soluble molecules can pass through LN without percolating through the cortex (1). Within the SCS resides CD169-expressing macrophage and DC; these cells capture large molecules, particles, and microorganisms; and then display antigens to the lymphocytes (2–4). Densely packed B cells and follicular dendritic cells (FDCs) are organized into discrete B cell follicles in the cortex. FDCs cluster in the center of the follicles and form a dense network in which B cells contact with the antigens. Lymphocytes mainly enter LNs from the blood via high endothelial venules (HEVs) (5). T cell zones of the paracortex contain CD4+ and CD8+ T cells and subsets of DCs in close contact with a network of conduits formed by fibroblastic reticular cells (FRCs). The medulla is composed of a three-dimensional labyrinthine structure of sinus channels starting as cortical sinusoids and expands to become wider medullary sinuses that finally drain collectively into the efferent lymphatic vessel (6).
Lymph nodes consist of not only hematopoietic cells (CD45+) but also heterogeneous populations of non-hematopoietic cells (CD45−). Currently, there are five major stromal cell subsets that have been characterized, namely, the marginal reticular cells (MRCs), FRCs, lymphatic endothelial cells (LECs), blood endothelial cells (BECs), and FDCs. They can be identified by their anatomical location within the LN and by the expression of CD31, podoplanin (also known as Gp38), CD35 (complement receptor 1), and mucosal addressin cell adhesion molecule-1 (MadCAM-1). MRCs and FRCs express Gp38 but not CD35 and CD31. MRCs can be delineated from FRCs not only by their expression of MadCAM-1 but also by their localization in the outer follicular region immediately underneath the SCS (7). LECs express both CD31 and Gp38, whereas BECs express only CD31. FDCs are centrally located within B cell follicles and are often classified based on the expression of CD21/CD35 (8), FDC-M1 (9), and FDC-M2 (complement C4) (10). Conventionally, stromal cells have long been perceived to provide structural support to the LNs during homeostasis and inflammation. Emerging evidence also indicates that stromal compartments of LNs play active roles in the immune response through their interactions with hematopoietic cells. We will briefly discuss here the role of FRCs as it has been covered recently in excellent reviews (11–13), and this review focuses on LECs.
Fibroblastic Reticular Cells
Fibroblastic reticular cells are resident mesenchymal cells, primarily residing in the T cells zone and capable of secreting and forming an elaborate reticular network within the LN. Single layer of FRCs enwrap extracellular matrix (ECM) that consists of a central core formed by 20–200 parallel bundles of fibrillar collagens (I and III) and intervening matrix of fibrils (14–16). These collagen bundles are surrounded by a layer of fibrillin-constituted microfibrils that are further ensheathed by a unique basement membrane-type structure (15, 16). In addition, stabilizing and cross-linking molecules such as fibromodulin, decorin, and lumican are also associated with the collagen fibers (17). FRCs also express other ECM component including ER-TR7 and common basement membrane component such as laminin and fibronectin (13). Integrin subunits and adhesion ligands such as intercellular adhesion molecule 1 (ICAM-I) and vascular cell adhesion molecule 1 are also found in FRCs (13). The three-dimensional tubular conduit system formed by FRCs extend the SCS throughout the T cell zone and form a contiguous lumen with fluid channels around the HEVs (18). Small lymph-borne molecules including chemokines and antigens from upstream periphery are transported within the core of FRC conduits from the SCS toward the HEVs. Molecules of high molecular mass (>70 kDa) cannot gain access to the conduit lumen and hence circumvent the lymphoid compartment and drained along the sinuses into the efferent lymphatic vessels (1, 4). Large particles including whole virus particles can also be captured by SCS macrophages and presented to migrating B cells in the underlying follicles (2, 4, 19).
In addition to acting as a key structural component in the LNs, FRCs are actively engaged in functional interactions with hematopoietic cells by forming conduits for antigens and inflammatory stimuli (1, 18), maintaining T cell survival (20), providing “tracks” and chemokines cue to guide cellular movement (21, 22), and supporting DC–T–B cell interactions during immune response (23) and peripheral tolerance (24–26). Disruption of FRC integrity and organization in the LNs during viral infection leads to profound loss of immunocompetence (27) strongly underscoring the roles of FRCs in maintaining proper immune response.
Lymphatic Endothelial Cells
Lymphatic vessels are present in most tissues and are important for maintenance of fluid homeostasis, immune cells trafficking, and movement of soluble antigens (28). Lymph from upstream peripheral tissues first passes through the SCS, a space underneath the collagen-rich fibrous capsule that covers the LN. The floor of SCS is lined by LECs expressing lymphatic vessel endothelial hyaluronan receptor 1 (LYVE-1) and is interspersed with CD169+ macrophages and DCs. From there, lymph percolates through the highly branched medullary sinuses and blind-ended cortical sinuses before leaving the LNs via the efferent lymphatic vessel (6). Cortical LECs form the vessels and branch into the T cell zone and have been indicated to facilitate B and T cell egress (29–31). Medullary sinuses lined by LYVE-1+ endothelium are found at LN exit within the medulla. Recently, the markers to delineate the LECs located in the SCS, cortex, and medulla have been reported and include programmed death ligand 1 (PD-L1), ICAM-1, MadCAM-1, and lymphotoxin β receptor (32).
Research on LN LECs in the past decades has demonstrated that lymphatic vessels are not “inert conduits” but rather plastic structures that actively sense and respond to changes in the peripheral tissue environment. For example, inflammation induced by bacterial pathogen, immunization in the presence of complete Freund’s adjuvant, and contact sensitization have been shown to promote the growth of lymphatic vessels from preexisting ones, a process named lymphangiogenesis, in LNs (33–37). Furthermore, it becomes apparent that such lymphatic remodeling in LN can have important biological consequences including modulation of inflammation and adaptive immune responses (38–41). Indeed, a growing body of evidence is now demonstrating that LECs themselves can help shape adaptive immune responses through their interactions with key immune cells including DCs, macrophages, and lymphocytes. Owing to their migration through and within lymphatic vessels and their anatomical distribution in LNs, T cells frequently encounter LECs. This review focuses on the crosstalk between T cells and LECs in LNs and its immunological consequences.
LN LECs Control T Cell Pool
LECs Regulate T Cell Migration to, within, and out of LN
We will briefly discuss in this section how LECs attract and facilitate the trafficking of T cells from the periphery to LN and within the LN since this topic has been covered in depth in excellent reviews (42, 43). Although LECs have been shown to express a large number of chemokines that attract T cells (38), the role of CCL21 is the most established in the homing of naïve, memory, and T regulatory (Treg) T cells to LNs. The signaling induced by CCL21 binding to its receptor, CCR7, on the surface of migratory T cells is critical for T cell trafficking from the periphery to the LN as shown in mice deficient for CCR7 ligands (44). Then, LECs in the cortical sinuses regulate intranodal lymphocyte trafficking by collecting lymphocytes for further transit to medullary sinuses (45). Moreover, lymphocytes can frequently move from the lymphatic sinuses back to the LN parenchyma (45). In line with these findings, it was reported that lymph-borne lymphocytes are passively transported into the peripheral medullary sinuses. Subsequently, they enter the LN parenchyma independently of CCR7 signals by migrating into adjacent peripheral medullary cords (46).
Medullary sinuses are directly connected to the efferent lymphatic vessel and have been proposed in addition to cortical sinuses as exit routes for the egress of lymphocytes from LNs (29, 30, 45). The molecular mechanisms of lymphocyte egress mediated by LECs remain elusive, and further investigations will be needed to explain how medullary sinuses can serve as both entry and egress structures for T cells. Most work on T cell egress has focused on mechanisms that lymphocytes uses to reach efferent lymphatic vessels and has identified sphingosine-1-phosphate (S1P)/S1P1 as a critical signal axis in promoting T cell egress (47). S1P levels are low in LN parenchyma but high in lymph fluid, thus creating a gradient. This S1P gradient guides T cells exhibiting decreased CCR7-retention signals from LN parenchyma into medullary and cortical sinuses and ultimately facilitates T cell egress (48). Notably, S1P in cortical sinuses and efferent lymph has been shown to be produced by LYVE-1+ LECs. Mice lacking specifically S1P kinase, the enzyme responsible for S1P synthesis, in LECs show compromised T cell egress (49). It is well established that local immune responses and inflammation are accompanied by alterations in the trafficking of lymphocytes through LNs. Specifically, the entry of lymphocytes into LNs increased, whereas their egress into efferent lymph is temporarily inhibited for few hours to days, depending on the nature of the stimulus (50–52). Few years ago, we reported that inflammation in LN, as it evolves from early to late phases, can induce a biphasic remodeling of lymphatic network, with the SCSs being expanded first, followed by the cortical and medullary sinuses. We showed that the early expansion of SCSs enhances the migration of DCs from the periphery, whereas the preferential expansion of cortical and medullary sinuses at later stages of inflammation supports the restoration of lymphocyte egress to steady-state levels (53).
LN LECs Support the Survival of T Cells
Several emerging evidence indicates that LECs may not only regulate the homeostasis of T cells in LNs through the modulation of their migration but also their survival. Interleukin (IL)-7 binds to IL-7Rα chain in combination with the common-γ chain and is essential for T lymphocyte homeostasis within the secondary lymphoid organs. IL-7 expression in vivo, which appears to limit the size of the lymphocyte pool, was thought to be regulated by IL-7 receptor α (IL-7Rα)-mediated consumption rather than the rate of IL-7 expression (54, 55). However, this concept has been recently challenged by a study showing that IL-7 expression can be induced in the liver in response to Toll-like receptor signaling and can directly control T cell responses (56). In line with this latter study, an earlier report by the same group demonstrated that excessive IL-6 expression increases IL-7 expression, which in turn was associated with the development of autoimmune reaction (57). These studies underscore that production of IL-7 by non-hematopoietic cells is tightly and dynamically regulated. In LNs, IL-7 provides antiapoptotic and proliferative signals to naïve and memory T cells (58–61). Although FRCs have been shown to be a major producer of IL-7 in LNs (20), it appears now evident that LECs are also an important source of IL-7 in murine and human LNs (62, 63). Interestingly, during inflammation-induced LN remodeling that influences intranodal lymphocyte dynamics, IL-7-expressing cortical sinus LECs have been shown to be essential for LN remodeling (63). In line with the role of IL-7 in maintaining memory T cells, a recent study revealed that LECs in lungs from mouse and humans can support the survival of memory T-helper cells through the production of IL-7 and IL-33 during allergic airway inflammation (64). IL-33 is a pro-inflammatory cytokine that initiates chronic inflammation in the lung, and its receptor is highly expressed on memory Th2 cells. IL-33 has been shown to directly induce memory Th2 cells to produce IL-5 and induces eosinophilic inflammation. Although this study focuses on lung LECs, it raises the possibility that LECs through the production of diverse cytokines may control the survival of pathogenic T cells during chronic inflammation, which in turn may have serious pathological consequences. Furthermore, the fact that IL-7 has been shown to mediate the transition from effector into memory T cells (65, 66) may also suggest the potential implication of LECs in shaping T cell differentiation in LNs during immune response.
LN LECs Regulate T Cell Activation
LN LECs Function as APCs for Peripheral T Cell Tolerance
Peripheral immune tolerance is generally ascribed to quiescent tissue-resident DCs cross-presentation of tissue-associated antigens to self-reactive T cells that have escaped thymic negative selection (67). More recently, accumulating evidence demonstrates that direct presentation of self-antigens by LN stromal cell subsets including FRCs and LECs can also mediate peripheral tolerance (25, 26, 68). Among LN stromal cell populations, LECs are likely the first cells that are in direct contact with the antigens, danger signals, and immune cells that carry peripheral blueprint to the draining LN. LECs express MHC class I (68–70) and MHC class II (41, 71, 72) and are capable of inducing T cell tolerance directly and suppressing DC-mediated T cell activation. In addition, T cell activation is also affected by the cytokine environment and relative balance between costimulatory and inhibitory signals from the APCs (41, 71, 73–75).
There are several potential pathways by which LECs can induce T cell tolerance. For instance, LN LECs express multiple peripheral tissue antigens (PTAs) (25, 69). In steady state, LECs lack costimulatory molecules such as CD80, CD86, or 4-1BBL that normally drive immunogenic T cell response. Instead, high expression of PD-L1 on LECs and engagement with its receptor on T cells predispose them to promote peripheral T cell tolerance (41). In a model of LEC-induced tolerance of melanocytes differentiation protein tyrosinase-specific CD8+ T cells, lack of stimulation through 4-1BB led to rapid and increased expression level of PD-1. Signaling through PD-1 inhibits upregulation of IL-2R on CD8+ T cells, culminating in apoptotic death associated with the loss of IL-2 prosurvival signaling (41). On the other hand, rescue of tyrosinase-specific CD8+ T cells by interfering PD-1 signaling or providing costimulatory signals gain effector function and induce autoimmune vitiligo, demonstrating that LECs are important and specialized APCs for peripheral T cell tolerance (41). This latter finding is in line with the observation in severe enteric autoimmunity that loss of PD-1/PD L1 inhibitory pathway blocks CD8+ T cell tolerance to intestinal self-antigens (76). It is worth to note that tyrosinase and PD-L1 are expressed at higher levels in LN LECs as opposed to LECs in periphery (diaphragm or colon), indicating that the LN microenvironment endows LN LECs with tolerogenic properties not found in tissue LECs (32). Given that LECs express various PTAs, dysregulation of LEC-associated tolerance is likely expected to contribute to the development of several autoimmune disorders.
In addition to transcriptionally expressed PTAs, LN LECs have also been shown to scavenge and cross-present exogenous antigen to naïve CD8+ T cells in the model of B16 F10 melanoma expressing the foreign antigen ovalbumin (OVA) and overexpressing vascular endothelial growth factor (VEGF)-C (70). VEGF-C-induced LN lymphangiogenesis suppresses anti-tumor immunity by local deletion of OVA-specific CD8+ T cells, which in turn drives disease progression and metastatic outgrowth. Similar observation was also reported under homeostatic conditions whereby intradermal injection of fluorescently labeled OVA protein was engulfed by LN LECs, processed, and presented on MHC class I to cognate CD8+ T cells in a TAP1-dependent manner (77). Such T cell/LEC interaction was shown to lead to decreased cytokine production and increased expression of Annexin V and exhaustion markers (PD-1, CD80, and CTLA-4) in vitro (77). These experimental findings suggest that regardless of the source of antigen (exogenous or endogenous), constitutive expression of inhibitory molecules and lack of costimulatory molecules on LECs will predominantly induce peripheral tolerance.
Furthermore, LECs express intermediate levels of MHC class II molecules suggesting that they might also tolerize CD4+ T cells (41, 71). MHC class II on LECs has shown to be either acquired from the DCs or endogenously expressed (24, 72). Rouhani et al. employed transgenic systems where antigens β-galactosidase (β-gal) and hemagglutinin (HA) were conditionally expressed in LECs under the control of Prox-1 and LYVE-1 promoters (72). Both CD8+ and CD4+ T cell receptors are available in these models and hence allowing comparative evaluation of the ability of LECs to drive tolerance to epitopes from the same protein presented by either MHC class I or MHC class II molecules. The authors demonstrated that PTA β-gal and HA epitopes on MHC class I were directly presented to CD8+ T cells, whereas these epitopes on MHC class II molecules were not presented to CD4+ T cells both in vivo and in vitro. Instead, these antigens were transferred to DC and then presented to CD4+ T cell to induce anergy. Therefore, LECs serve as a reservoir and repertoire of PTAs in the LN that may be acquired by DCs to induce tolerogenic CD4+ T cells. Similarly, Dubrot et al. showed that LECs acquire peptide: MHC class II complexes from DCs (24). However, in contrast to Rouhani et al., these complexes were not observed to be transferred back to LECs in sufficient quantities to induce CD4+ T cells recognition and subsequent antigen-specific T cells apoptosis.
LN LECs Modulate DC Functions
LECs may also regulate T cell activation indirectly by modulating antigen-presenting functions of DCs. Under steady state, immature DCs typically capture autoantigens from apoptotic cells, migrate to LNs, and promote T cell tolerance (78–80). Exposure of DCs to danger signals during inflammation or infection increases the expression of MHC class II molecules, costimulatory molecules, and cytokine that ultimately can trigger immunity and prevent tolerance. LECs have been shown to attenuate T cell response by suppressing DC maturation (73, 74, 81). Direct contact of immature DCs with an inflamed, TNF-α-stimulated LECs decreases expression of CD86 on DCs, dampening their ability to stimulate T cell proliferation (81). This interaction was mediated by the binding of ICAM-1 on LECs to Mac-1 on DCs and was observed in the absence of PAMPs (81). LECs also restrain T cell proliferation through upregulation of nitric oxide synthase-2 and production of NO in response to interferon (IFN)-γ and TNF-α released from activated T cells (73). Furthermore, IFN- γ-stimulated cultured human LN LEC produces inhibitory indoleamine 2,3 dioxygenase that in turn impairs CD4+ T cell proliferation (74). Interestingly, in different contexts such as viral challenge and subunit vaccination, viral antigens are captured and archived in LECs and subsequently transferred to DCs for the maintenance of memory T cells and enhancement of protective immunity (82). Therefore, crosstalk between LECs and DCs within the LN can either drive tolerogenic or immunogenic responses depending on the antigenic stimuli, immune cells encountered, and the type of inflammatory challenges.
LN LECs Archive Antigens
Several studies have reported that persistence of virally associated antigens after acute infection and subsequent viral clearance or so-called the reservoir of antigens was localized within the LNs draining the site of initial infection (82–86). A recent report demonstrated that LN LECs retain persisting antigens for weeks after vaccination (82). This antigen archiving was dependent on the induction of LN lymphatic proliferation. However, LECs did not present directly the archived antigen to T cells but instead required hematopoietic APCs. The number and percentage of CD8+ T cell-producing IFN-γ and IL-2 were significantly increased when antigen was retained in LECs. Notably, we previously reported that LN lymphangiogenesis persists during prolonged inflammation (53). Thus, it is plausible that the persistence of an expanded LN lymphatic network after viral infection or vaccination may allow the long-term storage of viral antigens. As a consequence, ongoing antigen presentation and recognition by memory T cells may lead to selective enrichment of virus-specific memory T cells in the draining LN even after the clearance of the infectious agent. This enriched population of antigen-specific T cells may provide more rapid effector responses in the periphery and better control of secondary infections.
T Cells Control LEC Growth upon Inflammation
Because lymphangiogenesis in LN has been shown to have diverse functional consequences on inflammation and immune responses depending on the context and timeframe of its occurrence (39, 40), this process is expected to be highly regulated. Indeed, a large number of studies have identified cellular and molecular mechanisms promoting the growth of lymphatic vessels. In contrast, little knowledge is currently available on pathways counter-regulating lymphangiogenesis. Both non-immune and immune cells have been described to orchestrate the expansion of lymphatic vessel network within LN. Interestingly, among immune cells, B and T cells have been shown to have opposite effects, namely, B cells support inflammatory lymphangiogenesis in LNs, whereas T cells have antilymphangiogenic effects. The first evidence supporting a role for T cells as negative regulators of LEC growth arises from a mouse study in which T cells were ablated using athymic mice (37). This antilymphangiogenic effect of T cells in the athymic mice was restored by the adoptive transfer of CD4+ or CD8+ T cells. This study suggests that both CD4+ and CD8+ T cells may harbor an antilymphangiogenic property. Other studies in different mouse models of inflammatory lymphangiogenesis have further confirmed the regulatory function of CD4+ T cells on LEC growth (34, 87). In the model of LN lymphangiogenesis induced by bacterial lipopolysaccharide, the authors demonstrated that the secretion of IFN-γ by T cells accounts for the inhibitory effect of T cells on LN lymphangiogenesis (37). Moreover, in line with an earlier study (88), they showed using in vitro cultured LECs that IFN-γ can act directly on LECs and affect their proliferation and survival (see Table 1) (37, 88).
These two latter studies provided the first evidence for a role of cytokines in controlling the expansion of lymphatic vessels. Since then, this notion has been further validated by several recent studies reporting the effect of other cytokines including IL-10, IL-17, TGF-β, and IL-4/IL-13 on LEC growth in vitro and/or in diverse models of inflammatory or de novo lymphangiogenesis induced in LN or other tissues (Table 1). From these studies, it becomes apparent that (i) cytokines are not always antilymphangiogenic; (ii) one given cytokine may have prolymphangiogenic or antilymphangiogenic properties depending on the context in which lymphatic growth occurs; and (iii) modulation of lymphatic proliferation, survival, and migration by cytokines can be mediated by a direct effect on LECs or indirectly by controlling the expression of lymphangiogenic factors such as VEGF-A, -C, and -D. Interestingly, all these cytokines can be secreted by different CD4+ T subsets including Th1, Th2, Th17, and Treg cells raising the possibility that different T cell subsets recruited to LN may affect LEC growth. Although this notion is indirectly supported by the studies cited in Table 1 and a recent study reporting the effect of Treg on lymphatic transport in a mouse model of lymphedema (100), direct evidence for a role of these T cell subsets and their cytokines in controlling LN lymphangiogenesis is lacking.
Implications of LEC Immunomodulatory Properties in Cancer Progression
The ever-growing research on tumor biology, immunology, and lymphatic biology has recently highlighted the multifaceted roles of lymphatic vessels in shaping tumor immunity and in cancer progression. One of the cardinal functions of lymphatic vessel is to transport components of the local tissue containing interstitial solutes, cytokines, growth factors, and immune cells to the downstream LN for the maintenance of tissue fluid homeostasis and peripheral immune tolerance. Tumor cells can “hijack” the lymphatic and induce the expansion of lymphatic vessels for their dissemination, colonization, and the formation of metastasis in the tumor-draining LNs (101, 102) (Figure 1). Via the lymphatic route, tumor cells can also modify the microenvironment of the metastatic organs from the distal sites before their arrival—referred to premetastatic niche. LN lymphangiogenesis preceding metastasis is an important mechanism and is associated with cancer progression (103–106).
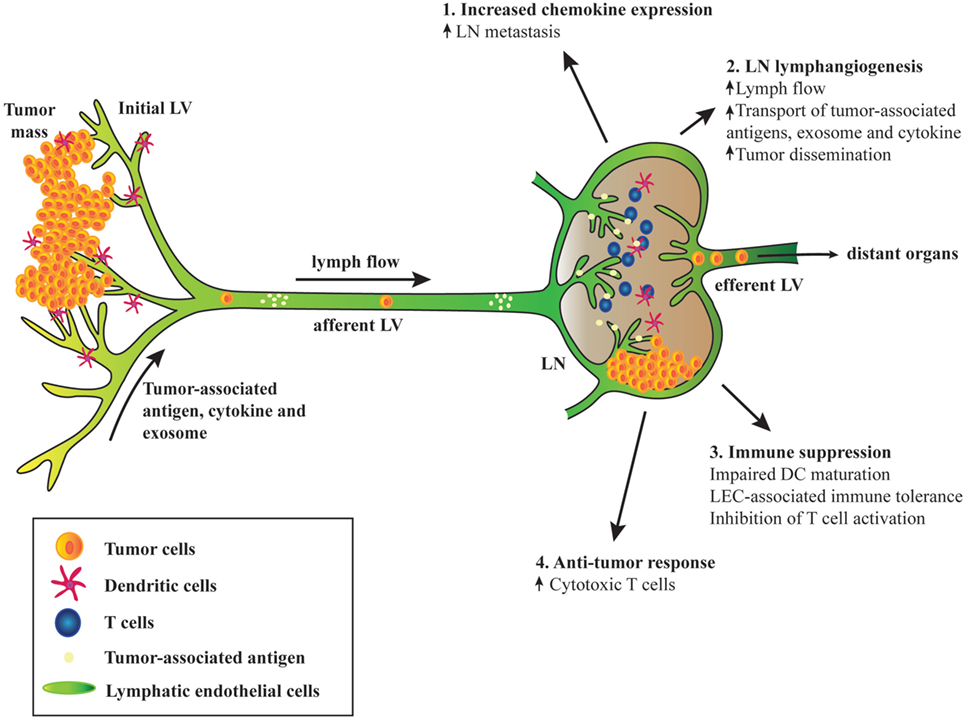
Figure 1. Schematic diagram depicting the involvement of tumor-associated lymphatic endothelial cell (LEC) in cancer. (1) Tumor-associated upregulation of chemokine expression in lymph node (LN) LECs mediates metastasis of tumor cells expressing the cognate chemokine receptors. (2) Tumor-associated factors, cytokines, and exosome draining from the upstream tumors and afferent lymphatic induce LN lymphangiogenesis, leading to increased lymph flow, transport of tumor-derived factors, and enhanced tumor cell dissemination. (3) Tumor-associated LECs can suppress immunity and promote tolerance. Interaction between LN LECs and dendritic cells (DCs) via intercellular adhesion molecule 1 and Mac-1 inhibits DC maturation and hence limiting effective T cell activation. Tumor antigen presentation to naïve CD8+ T cells by LN LECs induces dysfunctional T cell activation and tolerance due to expression of inhibitory receptor programmed death ligand 1 and lack of costimulatory molecules on LEC surface. LECs activated by T cell-derived pro-inflammatory cytokines produce factors such as NO and indoleamine 2,3 dioxygenase that inhibit T cell proliferation. (4) Robust CD8+ T cells priming occurs in tumor-draining LN. Although tolerogenic LN microenvironment may dominate and sustain immune suppression, immune checkpoint blockades can reverse T cell exhaustion and increase effector T cell activities that may lead to tumor regression.
Moreover, LN LECs express several chemokines that can attract cancer cells expressing the cognate chemokine receptors. For instance, constitutive CCL21 expression by LEC can serve as a guide for CCR7-expressing breast cancer and melanoma cells invading the LNs (107). Overexpression of CCR7 in melanoma has been shown to promote LN metastasis in mice (108), and CCR7 expression in human cancer samples correlates positively with LN metastasis (109–111). Upregulation of CXCL12 expression has been reported to enhance LN metastasis of CXCR4+ tumor cells (112). CCL1 is another chemokine produced by the SCS LEC, which has been shown to control CCR8+ tumor cell entry and subsequent migration and colonization in the LN cortex (113). Blocking of CCL1-CCR8 signaling results in the arrest of tumor cells at the junction of the afferent lymphatic vessels and the LN.
As discussed earlier, LN LECs can profoundly affect T cell survival, fate, and activation that can be of significant importance in tumor immune responses (Figure 1). The primary tumor is connected to the downstream afferent lymphatic vessel and draining LNs, and this connection may allow the entry of tumor-derived factors to the draining LNs and consequently may alter regional immune responses. Such alterations were reported to occur even before LN metastasis (114). Moreover, owing to the lack of costimulatory molecules expression and high levels of inhibitory ligand PD-L1 on LN LECs, lymphatic antigen presentation via MHC-I can induce deletional tolerance, a mechanism by which tumor cells may evade host immunity (41, 69, 70). VEGF-C-induced LN lymphangiogenesis can further promote immune tolerance in B16 melanoma-implanted mouse model (70). However, these studies suggest that manipulating LEC-associated tolerance or cancer dissemination may create opportunities for a new generation of antitumor immunotherapy. Importantly, cancer immunotherapies targeting the immune checkpoints, PD-1 and PD-L1, are revolutionizing current cancer treatments (115, 116). In humans, anti-PD-1 antibodies that target tumor-specific T cells (117–119) and anti-PD-L1 antibodies that bind to ligand expressed by the tumor and intratumor immune cells (120, 121) show promising clinical benefits. One can speculate that targeting this PD-1/PD-L1 immune checkpoint via systemic administration may also interrupt the tolerogenic signaling pathway between LN LECs and CD8+ T cells. Perhaps, a more LN-specific delivery of these blocking antibodies or other anticancer vaccine may lead to a greater impact on antitumor immune responses (122).
Although LN LECs may contribute to immune suppressive environment within the tumor-draining LNs (whether by direct interaction with CD8+ T cells or by draining the immunosuppressive cytokines from the upstream tumors), their roles in tumor immune surveillance cannot be neglected (Figure 1). Indeed, circulating tumor-specific T cells in metastatic melanoma patients are functional although those isolated from tumor-draining LNs exhibit exhausted characteristics (decreased IFN-γ and increased CTLA-4 and LAG-3 expression) (123). Interestingly, co-administration of anti-CTLA-4 and PD-1 antibodies reverses T cell exhaustion by increasing effector T cell activity and cytokine production and hence augmenting tumor inhibition (124). Tumor immunity was examined in the context of impaired lymphatic function using a kCYC transgenic mouse model expressing Kaposi’s sarcoma-associated herpes virus latent-cycle gene, k-cyclin, and under the control of VEGFR-3 promoter (101). In this model, antigen-presenting ability of DCs and cytotoxicity of CD8+ T cells isolated from the draining LNs of kCYC mice were attenuated. Furthermore, adoptive transfer of CD8+ T cells derived from kCYC mice to naïve WT mice show impaired antitumor function (101). In another model of dermal lymphatic insufficiency (K14-VEGFR3-Ig mice), implanted melanoma grew robustly and exhibited marked reduction in leukocyte infiltration compared with those implanted in control mice, suggesting that lymphatic vessels are essential for the generation of tumor immune responses (125). In addition, we showed in a spontaneous mouse model of uveal melanoma that early resection of TDLNs promotes primary tumor growth, cancer cell dissemination, and metastasis (102). Even though we did not examine the role of immune responses in the absence of tumor-draining LNs, it is plausible that uncontrollable growth of primary tumor may be due to the lack of antitumor immunity since the depletion of CD8+ T cells accelerates tumor growth and dissemination in the same model (126). These reports strongly indicate that functional lymphatic and presence of tumor-draining LNs are required for cancer immune surveillance. To further support this, current cancer immunotherapies targeting the immune checkpoints have demonstrated and supported the evidence that antitumor immunity exists even in the most advanced stages of cancer (116, 127–129).
Concluding Remarks
The ever-growing research on lymphatic biology has clearly identified LECs as key players in regulating adaptive immunity particularly by affecting T cell functions. However, the dynamics of T cells/LECs interactions and their immunological consequences in the context of cancer need to be further delineated. LN LECs are intricately affected by peripheral tumor, tumor-associated factors, and immune cells that in turn enhance tumor cell dissemination and drive the balance between host immunity and tolerance. Hence, LN LECs may represent a potential therapeutic target in addition to immunotherapy strategies for cancer progression and metastasis. Although tumor-associated LN lymphangiogenesis can contribute to tumor dissemination and increased immune tolerance, LN LECs are also important for the communication between tumors and immune cells to mount antitumor immune responses. For these reasons, combined research on immunology, lymphatic, and tumor biology is essential to further elucidate the immunological roles of LN LECs in cancer and their impact on disease progression.
Author Contributions
All the authors listed have written the manuscript and VA approved it for publication.
Conflict of Interest Statement
The authors declare that the research was conducted in the absence of any commercial or financial relationships that could be construed as a potential conflict of interest.
Funding
This work was supported by grants from National Medical Research Council, Ministry of Education, and National Research Foundation to VA.
References
1. Gretz JE, Norbury CC, Anderson AO, Proudfoot AE, Shaw S. Lymph-borne chemokines and other low molecular weight molecules reach high endothelial venules via specialized conduits while a functional barrier limits access to the lymphocyte microenvironments in lymph node cortex. J Exp Med (2000) 192:1425–40. doi:10.1084/jem.192.10.1425
2. Carrasco YR, Batista FD. B cells acquire particulate antigen in a macrophage-rich area at the boundary between the follicle and the subcapsular sinus of the lymph node. Immunity (2007) 27:160–71. doi:10.1016/j.immuni.2007.06.007
3. Gerner MY, Torabi-Parizi P, Germain RN. Strategically localized dendritic cells promote rapid T cell responses to lymph-borne particulate antigens. Immunity (2015) 42:172–85. doi:10.1016/j.immuni.2014.12.024
4. Junt T, Moseman EA, Iannacone M, Massberg S, Lang PA, Boes M, et al. Subcapsular sinus macrophages in lymph nodes clear lymph-borne viruses and present them to antiviral B cells. Nature (2007) 450:110–4. doi:10.1038/nature06287
5. Girard JP, Springer TA. High endothelial venules (HEVs): specialized endothelium for lymphocyte migration. Immunol Today (1995) 16:449–57. doi:10.1016/0167-5699(95)80023-9
6. Forster R, Braun A, Worbs T. Lymph node homing of T cells and dendritic cells via afferent lymphatics. Trends Immunol (2012) 33:271–80. doi:10.1016/j.it.2012.02.007
7. Katakai T, Suto H, Sugai M, Gonda H, Togawa A, Suematsu S, et al. Organizer-like reticular stromal cell layer common to adult secondary lymphoid organs. J Immunol (2008) 181:6189–200. doi:10.4049/jimmunol.181.9.6189
8. Roozendaal R, Carroll MC. Complement receptors CD21 and CD35 in humoral immunity. Immunol Rev (2007) 219:157–66. doi:10.1111/j.1600-065X.2007.00556.x
9. Kranich J, Krautler NJ, Heinen E, Polymenidou M, Bridel C, Schildknecht A, et al. Follicular dendritic cells control engulfment of apoptotic bodies by secreting Mfge8. J Exp Med (2008) 205:1293–302. doi:10.1084/jem.20071019
10. Taylor PR, Pickering MC, Kosco-Vilbois MH, Walport MJ, Botto M, Gordon S, et al. The follicular dendritic cell restricted epitope, FDC-M2, is complement C4; localization of immune complexes in mouse tissues. Eur J Immunol (2002) 32:1888–96. doi:10.1002/1521-4141(200207)32:7<1883::AID-IMMU1888>3.0.CO;2-8
11. Brown FD, Turley SJ. Fibroblastic reticular cells: organization and regulation of the T lymphocyte life cycle. J Immunol (2015) 194:1389–94. doi:10.4049/jimmunol.1402520
12. Fletcher AL, Acton SE, Knoblich K. Lymph node fibroblastic reticular cells in health and disease. Nat Rev Immunol (2015) 15:350–61. doi:10.1038/nri3846
13. Mueller SN, Germain RN. Stromal cell contributions to the homeostasis and functionality of the immune system. Nat Rev Immunol (2009) 9:618–29. doi:10.1038/nri2588
14. Hayakawa M, Kobayashi M, Hoshino T. Microfibrils: a constitutive component of reticular fibers in the mouse lymph node. Cell Tissue Res (1990) 262:199–201. doi:10.1007/BF00327763
15. Kaldjian EP, Gretz JE, Anderson AO, Shi Y, Shaw S. Spatial and molecular organization of lymph node T cell cortex: a labyrinthine cavity bounded by an epithelium-like monolayer of fibroblastic reticular cells anchored to basement membrane-like extracellular matrix. Int Immunol (2001) 13:1243–53. doi:10.1093/intimm/13.10.1243
16. Sixt M, Kanazawa N, Selg M, Samson T, Roos G, Reinhardt DP, et al. The conduit system transports soluble antigens from the afferent lymph to resident dendritic cells in the T cell area of the lymph node. Immunity (2005) 22:19–29. doi:10.1016/j.immuni.2004.11.013
17. Lammermann T, Sixt M. The microanatomy of T-cell responses. Immunol Rev (2008) 221:26–43. doi:10.1111/j.1600-065X.2008.00592.x
18. Gretz JE, Anderson AO, Shaw S. Cords, channels, corridors and conduits: critical architectural elements facilitating cell interactions in the lymph node cortex. Immunol Rev (1997) 156:11–24. doi:10.1111/j.1600-065X.1997.tb00955.x
19. Phan TG, Grigorova I, Okada T, Cyster JG. Subcapsular encounter and complement-dependent transport of immune complexes by lymph node B cells. Nat Immunol (2007) 8:992–1000. doi:10.1038/ni1494
20. Link A, Vogt TK, Favre S, Britschgi MR, Acha-Orbea H, Hinz B, et al. Fibroblastic reticular cells in lymph nodes regulate the homeostasis of naive T cells. Nat Immunol (2007) 8:1255–65. doi:10.1038/ni1513
21. Bajenoff M, Egen JG, Koo LY, Laugier JP, Brau F, Glaichenhaus N, et al. Stromal cell networks regulate lymphocyte entry, migration, and territoriality in lymph nodes. Immunity (2006) 25:989–1001. doi:10.1016/j.immuni.2006.10.011
22. Worbs T, Mempel TR, Bolter J, von Andrian UH, Forster R. CCR7 ligands stimulate the intranodal motility of T lymphocytes in vivo. J Exp Med (2007) 204:489–95. doi:10.1084/jem.20061706
23. Katakai T, Hara T, Lee JH, Gonda H, Sugai M, Shimizu A. A novel reticular stromal structure in lymph node cortex: an immuno-platform for interactions among dendritic cells, T cells and B cells. Int Immunol (2004) 16:1133–42. doi:10.1093/intimm/dxh113
24. Dubrot J, Duraes FV, Potin L, Capotosti F, Brighouse D, Suter T, et al. Lymph node stromal cells acquire peptide-MHCII complexes from dendritic cells and induce antigen-specific CD4(+) T cell tolerance. J Exp Med (2014) 211:1153–66. doi:10.1084/jem.20132000
25. Fletcher AL, Lukacs-Kornek V, Reynoso ED, Pinner SE, Bellemare-Pelletier A, Curry MS, et al. Lymph node fibroblastic reticular cells directly present peripheral tissue antigen under steady-state and inflammatory conditions. J Exp Med (2010) 207:689–97. doi:10.1084/jem.20092642
26. Lee JW, Epardaud M, Sun J, Becker JE, Cheng AC, Yonekura AR, et al. Peripheral antigen display by lymph node stroma promotes T cell tolerance to intestinal self. Nat Immunol (2007) 8:181–90. doi:10.1038/ni1427
27. Schacker TW, Brenchley JM, Beilman GJ, Reilly C, Pambuccian SE, Taylor J, et al. Lymphatic tissue fibrosis is associated with reduced numbers of naive CD4+ T cells in human immunodeficiency virus type 1 infection. Clin Vaccine Immunol (2006) 13:556–60. doi:10.1128/CVI.13.5.556-560.2006
29. Grigorova IL, Schwab SR, Phan TG, Pham TH, Okada T, Cyster JG. Cortical sinus probing, S1P1-dependent entry and flow-based capture of egressing T cells. Nat Immunol (2009) 10:58–65. doi:10.1038/ni.1682
30. Pham TH, Okada T, Matloubian M, Lo CG, Cyster JG. S1P1 receptor signaling overrides retention mediated by G alpha i-coupled receptors to promote T cell egress. Immunity (2008) 28:122–33. doi:10.1016/j.immuni.2007.11.017
31. Sinha RK, Park C, Hwang IY, Davis MD, Kehrl JH. B lymphocytes exit lymph nodes through cortical lymphatic sinusoids by a mechanism independent of sphingosine-1-phosphate-mediated chemotaxis. Immunity (2009) 30(3):434–46. doi:10.1016/j.immuni.2008.12.018
32. Cohen JN, Tewalt EF, Rouhani SJ, Buonomo EL, Bruce AN, Xu X, et al. Tolerogenic properties of lymphatic endothelial cells are controlled by the lymph node microenvironment. PLoS One (2014) 9:e87740. doi:10.1371/journal.pone.0087740
33. Angeli V, Ginhoux F, Llodra J, Quemeneur L, Frenette PS, Skobe M, et al. B cell-driven lymphangiogenesis in inflamed lymph nodes enhances dendritic cell mobilization. Immunity (2006) 24:203–15. doi:10.1016/j.immuni.2006.01.003
34. Avraham T, Zampell JC, Yan A, Elhadad S, Weitman ES, Rockson SG, et al. Th2 differentiation is necessary for soft tissue fibrosis and lymphatic dysfunction resulting from lymphedema. FASEB J (2013) 27:1114–26. doi:10.1096/fj.12-222695
35. Halin C, Tobler NE, Vigl B, Brown LF, Detmar M. VEGF-A produced by chronically inflamed tissue induces lymphangiogenesis in draining lymph nodes. Blood (2007) 110:3158–67. doi:10.1182/blood-2007-01-066811
36. Kataru RP, Jung K, Jang C, Yang H, Schwendener RA, Baik JE, et al. Critical role of CD11b+ macrophages and VEGF in inflammatory lymphangiogenesis, antigen clearance, and inflammation resolution. Blood (2009) 113:5650–9. doi:10.1182/blood-2008-09-176776
37. Kataru RP, Kim H, Jang C, Choi DK, Koh BI, Kim M, et al. T lymphocytes negatively regulate lymph node lymphatic vessel formation. Immunity (2011) 34:96–107. doi:10.1016/j.immuni.2010.12.016
38. Card CM, Yu SS, Swartz MA. Emerging roles of lymphatic endothelium in regulating adaptive immunity. J Clin Invest (2014) 124:943–52. doi:10.1172/JCI73316
39. Kim H, Kataru RP, Koh GY. Inflammation-associated lymphangiogenesis: a double-edged sword? J Clin Invest (2014) 124:936–42. doi:10.1172/JCI71607
40. Tan KW, Chong SZ, Angeli V. Inflammatory lymphangiogenesis: cellular mediators and functional implications. Angiogenesis (2014) 17:373–81. doi:10.1007/s10456-014-9419-4
41. Tewalt EF, Cohen JN, Rouhani SJ, Guidi CJ, Qiao H, Fahl SP, et al. Lymphatic endothelial cells induce tolerance via PD-L1 and lack of costimulation leading to high-level PD-1 expression on CD8 T cells. Blood (2012) 120:4772–82. doi:10.1182/blood-2012-04-427013
42. Girard JP, Moussion C, Forster R. HEVs, lymphatics and homeostatic immune cell trafficking in lymph nodes. Nat Rev Immunol (2012) 12:762–73. doi:10.1038/nri3298
43. Johnson LA, Jackson DG. Cell traffic and the lymphatic endothelium. Ann N Y Acad Sci (2008) 1131:119–33. doi:10.1196/annals.1413.011
44. Forster R, Davalos-Misslitz AC, Rot A. CCR7 and its ligands: balancing immunity and tolerance. Nat Rev Immunol (2008) 8:362–71. doi:10.1038/nri2297
45. Grigorova IL, Panteleev M, Cyster JG. Lymph node cortical sinus organization and relationship to lymphocyte egress dynamics and antigen exposure. Proc Natl Acad Sci U S A (2010) 107:20447–52. doi:10.1073/pnas.1009968107
46. Braun A, Worbs T, Moschovakis GL, Halle S, Hoffmann K, Bolter J, et al. Afferent lymph-derived T cells and DCs use different chemokine receptor CCR7-dependent routes for entry into the lymph node and intranodal migration. Nat Immunol (2011) 12:879–87. doi:10.1038/ni.2085
47. Schwab SR, Cyster JG. Finding a way out: lymphocyte egress from lymphoid organs. Nat Immunol (2007) 8:1295–301. doi:10.1038/ni1545
48. Lo CG, Xu Y, Proia RL, Cyster JG. Cyclical modulation of sphingosine-1-phosphate receptor 1 surface expression during lymphocyte recirculation and relationship to lymphoid organ transit. J Exp Med (2005) 201:291–301. doi:10.1084/jem.20041509
49. Pham TH, Baluk P, Xu Y, Grigorova I, Bankovich AJ, Pappu R, et al. Lymphatic endothelial cell sphingosine kinase activity is required for lymphocyte egress and lymphatic patterning. J Exp Med (2010) 207:17–27. doi:10.1084/jem.20091619
50. Cahill RN, Frost H, Trnka Z. The effects of antigen on the migration of recirculating lymphocytes through single lymph nodes. J Exp Med (1976) 143:870–88. doi:10.1084/jem.143.4.870
51. Hall JG, Morris B. The immediate effect of antigens on the cell output of a lymph node. Br J Exp Pathol (1965) 46:450–4.
52. Mackay CR, Marston W, Dudler L. Altered patterns of T cell migration through lymph nodes and skin following antigen challenge. Eur J Immunol (1992) 22:2205–10. doi:10.1002/eji.1830220904
53. Tan KW, Yeo KP, Wong FH, Lim HY, Khoo KL, Abastado JP, et al. Expansion of cortical and medullary sinuses restrains lymph node hypertrophy during prolonged inflammation. J Immunol (2012) 188:4065–80. doi:10.4049/jimmunol.1101854
54. Ma A, Koka R, Burkett P. Diverse functions of IL-2, IL-15, and IL-7 in lymphoid homeostasis. Annu Rev Immunol (2006) 24:657–79. doi:10.1146/annurev.immunol.24.021605.090727
55. Mazzucchelli R, Durum SK. Interleukin-7 receptor expression: intelligent design. Nat Rev Immunol (2007) 7:144–54. doi:10.1038/nri2023
56. Sawa Y, Arima Y, Ogura H, Kitabayashi C, Jiang JJ, Fukushima T, et al. Hepatic interleukin-7 expression regulates T cell responses. Immunity (2009) 30:447–57. doi:10.1016/j.immuni.2009.01.007
57. Sawa S, Kamimura D, Jin GH, Morikawa H, Kamon H, Nishihara M, et al. Autoimmune arthritis associated with mutated interleukin (IL)-6 receptor gp130 is driven by STAT3/IL-7-dependent homeostatic proliferation of CD4+ T cells. J Exp Med (2006) 203:1459–70. doi:10.1084/jem.20052187
58. Mackall CL, Fry TJ, Gress RE. Harnessing the biology of IL-7 for therapeutic application. Nat Rev Immunol (2011) 11:330–42. doi:10.1038/nri2970
59. Schluns KS, Kieper WC, Jameson SC, Lefrancois L. Interleukin-7 mediates the homeostasis of naive and memory CD8 T cells in vivo. Nat Immunol (2000) 1:426–32. doi:10.1038/80868
60. Schluns KS, Lefrancois L. Cytokine control of memory T-cell development and survival. Nat Rev Immunol (2003) 3:269–79. doi:10.1038/nri1052
61. Surh CD, Sprent J. Homeostasis of naive and memory T cells. Immunity (2008) 29:848–62. doi:10.1016/j.immuni.2008.11.002
62. Hara T, Shitara S, Imai K, Miyachi H, Kitano S, Yao H, et al. Identification of IL-7-producing cells in primary and secondary lymphoid organs using IL-7-GFP knock-in mice. J Immunol (2012) 189:1577–84. doi:10.4049/jimmunol.1200586
63. Onder L, Narang P, Scandella E, Chai Q, Iolyeva M, Hoorweg K, et al. IL-7-producing stromal cells are critical for lymph node remodeling. Blood (2012) 120:4675–83. doi:10.1182/blood-2012-03-416859
64. Shinoda K, Hirahara K, Iinuma T, Ichikawa T, Suzuki AS, Sugaya K, et al. Thy1+IL-7+ lymphatic endothelial cells in iBALT provide a survival niche for memory T-helper cells in allergic airway inflammation. Proc Natl Acad Sci U S A (2016) 113:E2842–51. doi:10.1073/pnas.1512600113
65. Kondrack RM, Harbertson J, Tan JT, McBreen ME, Surh CD, Bradley LM. Interleukin 7 regulates the survival and generation of memory CD4 cells. J Exp Med (2003) 198:1797–806. doi:10.1084/jem.20030735
66. Woodland DL, Kohlmeier JE. Migration, maintenance and recall of memory T cells in peripheral tissues. Nat Rev Immunol (2009) 9:153–61. doi:10.1038/nri2496
67. Belz GT, Behrens GM, Smith CM, Miller JF, Jones C, Lejon K, et al. The CD8alpha(+) dendritic cell is responsible for inducing peripheral self-tolerance to tissue-associated antigens. J Exp Med (2002) 196:1099–104. doi:10.1084/jem.20020861
68. Nichols LA, Chen Y, Colella TA, Bennett CL, Clausen BE, Engelhard VH. Deletional self-tolerance to a melanocyte/melanoma antigen derived from tyrosinase is mediated by a radio-resistant cell in peripheral and mesenteric lymph nodes. J Immunol (2007) 179:993–1003. doi:10.4049/jimmunol.179.2.993
69. Cohen JN, Guidi CJ, Tewalt EF, Qiao H, Rouhani SJ, Ruddell A, et al. Lymph node-resident lymphatic endothelial cells mediate peripheral tolerance via Aire-independent direct antigen presentation. J Exp Med (2010) 207:681–8. doi:10.1084/jem.20092465
70. Lund AW, Duraes FV, Hirosue S, Raghavan VR, Nembrini C, Thomas SN, et al. VEGF-C promotes immune tolerance in B16 melanomas and cross-presentation of tumor antigen by lymph node lymphatics. Cell Rep (2012) 1:191–9. doi:10.1016/j.celrep.2012.01.005
71. Malhotra D, Fletcher AL, Astarita J, Lukacs-Kornek V, Tayalia P, Gonzalez SF, et al. Transcriptional profiling of stroma from inflamed and resting lymph nodes defines immunological hallmarks. Nat Immunol (2012) 13:499–510. doi:10.1038/ni.2262
72. Rouhani SJ, Eccles JD, Riccardi P, Peske JD, Tewalt EF, Cohen JN, et al. Roles of lymphatic endothelial cells expressing peripheral tissue antigens in CD4 T-cell tolerance induction. Nat Commun (2015) 6:6771. doi:10.1038/ncomms7771
73. Lukacs-Kornek V, Malhotra D, Fletcher AL, Acton SE, Elpek KG, Tayalia P, et al. Regulated release of nitric oxide by nonhematopoietic stroma controls expansion of the activated T cell pool in lymph nodes. Nat Immunol (2011) 12:1096–104. doi:10.1038/ni.2112
74. Norder M, Gutierrez MG, Zicari S, Cervi E, Caruso A, Guzman CA. Lymph node-derived lymphatic endothelial cells express functional costimulatory molecules and impair dendritic cell-induced allogenic T-cell proliferation. FASEB J (2012) 26:2835–46. doi:10.1096/fj.12-205278
75. Podgrabinska S, Braun P, Velasco P, Kloos B, Pepper MS, Skobe M. Molecular characterization of lymphatic endothelial cells. Proc Natl Acad Sci U S A (2002) 99:16069–74. doi:10.1073/pnas.242401399
76. Reynoso ED, Elpek KG, Francisco L, Bronson R, Bellemare-Pelletier A, Sharpe AH, et al. Intestinal tolerance is converted to autoimmune enteritis upon PD-1 ligand blockade. J Immunol (2009) 182:2102–12. doi:10.4049/jimmunol.0802769
77. Hirosue S, Vokali E, Raghavan VR, Rincon-Restrepo M, Lund AW, Corthesy-Henrioud P, et al. Steady-state antigen scavenging, cross-presentation, and CD8+ T cell priming: a new role for lymphatic endothelial cells. J Immunol (2014) 192:5002–11. doi:10.4049/jimmunol.1302492
78. Hawiger D, Inaba K, Dorsett Y, Guo M, Mahnke K, Rivera M, et al. Dendritic cells induce peripheral T cell unresponsiveness under steady state conditions in vivo. J Exp Med (2001) 194:769–79. doi:10.1084/jem.194.6.769
79. Steinman RM, Hawiger D, Nussenzweig MC. Tolerogenic dendritic cells. Annu Rev Immunol (2003) 21:685–711. doi:10.1146/annurev.immunol.21.120601.141040
80. Steinman RM, Turley S, Mellman I, Inaba K. The induction of tolerance by dendritic cells that have captured apoptotic cells. J Exp Med (2000) 191:411–6. doi:10.1084/jem.191.3.411
81. Podgrabinska S, Kamalu O, Mayer L, Shimaoka M, Snoeck H, Randolph GJ, et al. Inflamed lymphatic endothelium suppresses dendritic cell maturation and function via Mac-1/ICAM-1-dependent mechanism. J Immunol (2009) 183:1767–79. doi:10.4049/jimmunol.0802167
82. Tamburini BA, Burchill MA, Kedl RM. Antigen capture and archiving by lymphatic endothelial cells following vaccination or viral infection. Nat Commun (2014) 5:3989. doi:10.1038/ncomms4989
83. Jelley-Gibbs DM, Brown DM, Dibble JP, Haynes L, Eaton SM, Swain SL. Unexpected prolonged presentation of influenza antigens promotes CD4 T cell memory generation. J Exp Med (2005) 202:697–706. doi:10.1084/jem.20050227
84. Kim TS, Hufford MM, Sun J, Fu YX, Braciale TJ. Antigen persistence and the control of local T cell memory by migrant respiratory dendritic cells after acute virus infection. J Exp Med (2010) 207:1161–72. doi:10.1084/jem.20092017
85. Takamura S, Roberts AD, Jelley-Gibbs DM, Wittmer ST, Kohlmeier JE, Woodland DL. The route of priming influences the ability of respiratory virus-specific memory CD8+ T cells to be activated by residual antigen. J Exp Med (2010) 207:1153–60. doi:10.1084/jem.20090283
86. Zammit DJ, Turner DL, Klonowski KD, Lefrancois L, Cauley LS. Residual antigen presentation after influenza virus infection affects CD8 T cell activation and migration. Immunity (2006) 24:439–49. doi:10.1016/j.immuni.2006.01.015
87. Zampell JC, Yan A, Elhadad S, Avraham T, Weitman E, Mehrara BJ. CD4(+) cells regulate fibrosis and lymphangiogenesis in response to lymphatic fluid stasis. PLoS One (2012) 7:e49940. doi:10.1371/journal.pone.0049940
88. Shao X, Liu C. Influence of IFN-alpha and IFN-gamma on lymphangiogenesis. J Interferon Cytokine Res (2006) 26:568–74. doi:10.1089/jir.2006.26.568
89. Savetsky IL, Ghanta S, Gardenier JC, Torrisi JS, Garcia Nores GD, Hespe GE, et al. Th2 cytokines inhibit lymphangiogenesis. PLoS One (2015) 10:e0126908. doi:10.1371/journal.pone.0126908
90. Shin K, Kataru RP, Park HJ, Kwon BI, Kim TW, Hong YK, et al. TH2 cells and their cytokines regulate formation and function of lymphatic vessels. Nat Commun (2015) 6:6196. doi:10.1038/ncomms7196
91. Shi VY, Bao L, Chan LS. Inflammation-driven dermal lymphangiogenesis in atopic dermatitis is associated with CD11b+ macrophage recruitment and VEGF-C up-regulation in the IL-4-transgenic mouse model. Microcirculation (2012) 19:567–79. doi:10.1111/j.1549-8719.2012.00189.x
92. Chauhan SK, Jin Y, Goyal S, Lee HS, Fuchsluger TA, Lee HK, et al. A novel pro-lymphangiogenic function for Th17/IL-17. Blood (2011) 118:4630–4. doi:10.1182/blood-2011-01-332049
93. Hos D, Bucher F, Regenfuss B, Dreisow ML, Bock F, Heindl LM, et al. IL-10 indirectly regulates corneal lymphangiogenesis and resolution of inflammation via macrophages. Am J Pathol (2016) 186:159–71. doi:10.1016/j.ajpath.2015.09.012
94. Oka M, Iwata C, Suzuki HI, Kiyono K, Morishita Y, Watabe T, et al. Inhibition of endogenous TGF-beta signaling enhances lymphangiogenesis. Blood (2008) 111:4571–9. doi:10.1182/blood-2007-10-120337
95. Clavin NW, Avraham T, Fernandez J, Daluvoy SV, Soares MA, Chaudhry A, et al. TGF-beta1 is a negative regulator of lymphatic regeneration during wound repair. Am J Physiol Heart Circ Physiol (2008) 295:H2113–27. doi:10.1152/ajpheart.00879.2008
96. Avraham T, Daluvoy S, Zampell J, Yan A, Haviv YS, Rockson SG, et al. Blockade of transforming growth factor-beta1 accelerates lymphatic regeneration during wound repair. Am J Pathol (2010) 177:3202–14. doi:10.2353/ajpath.2010.100594
97. Suzuki Y, Ito Y, Mizuno M, Kinashi H, Sawai A, Noda Y, et al. Transforming growth factor-beta induces vascular endothelial growth factor-C expression leading to lymphangiogenesis in rat unilateral ureteral obstruction. Kidney Int (2012) 81:865–79. doi:10.1038/ki.2011.464
98. Kinashi H, Ito Y, Mizuno M, Suzuki Y, Terabayashi T, Nagura F, et al. TGF-beta1 promotes lymphangiogenesis during peritoneal fibrosis. J Am Soc Nephrol (2013) 24:1627–42. doi:10.1681/ASN.2012030226
99. James JM, Nalbandian A, Mukouyama YS. TGFbeta signaling is required for sprouting lymphangiogenesis during lymphatic network development in the skin. Development (2013) 140:3903–14. doi:10.1242/dev.095026
100. Gousopoulos E, Proulx ST, Bachmann SB, Scholl J, Dionyssiou D, Demiri E, et al. Regulatory T cell transfer ameliorates lymphedema and promotes lymphatic vessel function. JCI Insight (2016) 1:e89081. doi:10.1172/jci.insight.89081
101. Kimura T, Sugaya M, Oka T, Blauvelt A, Okochi H, Sato S. Lymphatic dysfunction attenuates tumor immunity through impaired antigen presentation. Oncotarget (2015) 6:18081–93. doi:10.18632/oncotarget.4018
102. Pin YK, Khoo K, Tham M, Karwai T, Hwee TC, Puaux AL, et al. Lymphadenectomy promotes tumor growth and cancer cell dissemination in the spontaneous RET mouse model of human uveal melanoma. Oncotarget (2015) 6:44806–18. doi:10.18632/oncotarget.6326
103. Harrell MI, Iritani BM, Ruddell A. Tumor-induced sentinel lymph node lymphangiogenesis and increased lymph flow precede melanoma metastasis. Am J Pathol (2007) 170:774–86. doi:10.2353/ajpath.2007.060761
104. Hirakawa S, Brown LF, Kodama S, Paavonen K, Alitalo K, Detmar M. VEGF-C-induced lymphangiogenesis in sentinel lymph nodes promotes tumor metastasis to distant sites. Blood (2007) 109:1010–7. doi:10.1182/blood-2006-05-021758
105. Hirakawa S, Kodama S, Kunstfeld R, Kajiya K, Brown LF, Detmar M. VEGF-A induces tumor and sentinel lymph node lymphangiogenesis and promotes lymphatic metastasis. J Exp Med (2005) 201:1089–99. doi:10.1084/jem.20041896
106. Watanabe M, Tanaka H, Ohira M, Yoshii M, Sakurai K, Toyokawa T, et al. Intranodal lymphangiogenesis precedes development of lymph node metastasis and accelerates progression of gastric cancer. J Gastrointest Surg (2014) 18:481–90. doi:10.1007/s11605-013-2407-y
107. Muller A, Homey B, Soto H, Ge N, Catron D, Buchanan ME, et al. Involvement of chemokine receptors in breast cancer metastasis. Nature (2001) 410:50–6. doi:10.1038/35065016
108. Wiley HE, Gonzalez EB, Maki W, Wu MT, Hwang ST. Expression of CC chemokine receptor-7 and regional lymph node metastasis of B16 murine melanoma. J Natl Cancer Inst (2001) 93:1638–43. doi:10.1093/jnci/93.21.1638
109. Cabioglu N, Yazici MS, Arun B, Broglio KR, Hortobagyi GN, Price JE, et al. CCR7 and CXCR4 as novel biomarkers predicting axillary lymph node metastasis in T1 breast cancer. Clin Cancer Res (2005) 11:5686–93. doi:10.1158/1078-0432.CCR-05-0014
110. Ishigami S, Natsugoe S, Nakajo A, Tokuda K, Uenosono Y, Arigami T, et al. Prognostic value of CCR7 expression in gastric cancer. Hepatogastroenterology (2007) 54:1025–8.
111. Mashino K, Sadanaga N, Yamaguchi H, Tanaka F, Ohta M, Shibuta K, et al. Expression of chemokine receptor CCR7 is associated with lymph node metastasis of gastric carcinoma. Cancer Res (2002) 62:2937–41.
112. Kim M, Koh YJ, Kim KE, Koh BI, Nam DH, Alitalo K, et al. CXCR4 signaling regulates metastasis of chemoresistant melanoma cells by a lymphatic metastatic niche. Cancer Res (2010) 70:10411–21. doi:10.1158/0008-5472.CAN-10-2591
113. Das S, Sarrou E, Podgrabinska S, Cassella M, Mungamuri SK, Feirt N, et al. Tumor cell entry into the lymph node is controlled by CCL1 chemokine expressed by lymph node lymphatic sinuses. J Exp Med (2013) 210:1509–28. doi:10.1084/jem.20111627
114. Mansfield AS, Holtan SG, Grotz TE, Allred JB, Jakub JW, Erickson LA, et al. Regional immunity in melanoma: immunosuppressive changes precede nodal metastasis. Mod Pathol (2011) 24:487–94. doi:10.1038/modpathol.2010.227
115. Sharma P, Allison JP. The future of immune checkpoint therapy. Science (2015) 348:56–61. doi:10.1126/science.aaa8172
116. Smyth MJ, Ngiow SF, Ribas A, Teng MW. Combination cancer immunotherapies tailored to the tumour microenvironment. Nat Rev Clin Oncol (2016) 13:143–58. doi:10.1038/nrclinonc.2015.209
117. Taube JM, Anders RA, Young GD, Xu H, Sharma R, McMiller TL, et al. Colocalization of inflammatory response with B7-h1 expression in human melanocytic lesions supports an adaptive resistance mechanism of immune escape. Sci Transl Med (2012) 4:127ra137. doi:10.1126/scitranslmed.3003689
118. Taube JM, Klein A, Brahmer JR, Xu H, Pan X, Kim JH, et al. Association of PD-1, PD-1 ligands, and other features of the tumor immune microenvironment with response to anti-PD-1 therapy. Clin Cancer Res (2014) 20:5064–74. doi:10.1158/1078-0432.CCR-13-3271
119. Tumeh PC, Harview CL, Yearley JH, Shintaku IP, Taylor EJ, Robert L, et al. PD-1 blockade induces responses by inhibiting adaptive immune resistance. Nature (2014) 515:568–71. doi:10.1038/nature13954
120. Brahmer JR, Tykodi SS, Chow LQ, Hwu WJ, Topalian SL, Hwu P, et al. Safety and activity of anti-PD-L1 antibody in patients with advanced cancer. N Engl J Med (2012) 366:2455–65. doi:10.1056/NEJMoa1200694
121. Herbst RS, Soria JC, Kowanetz M, Fine GD, Hamid O, Gordon MS, et al. Predictive correlates of response to the anti-PD-L1 antibody MPDL3280A in cancer patients. Nature (2014) 515:563–7. doi:10.1038/nature14011
122. Jeanbart L, Ballester M, de Titta A, Corthesy P, Romero P, Hubbell JA, et al. Enhancing efficacy of anticancer vaccines by targeted delivery to tumor-draining lymph nodes. Cancer Immunol Res (2014) 2:436–47. doi:10.1158/2326-6066.CIR-14-0019-T
123. Baitsch L, Baumgaertner P, Devevre E, Raghav SK, Legat A, Barba L, et al. Exhaustion of tumor-specific CD8(+) T cells in metastases from melanoma patients. J Clin Invest (2011) 121:2350–60. doi:10.1172/JCI46102
124. Duraiswamy J, Kaluza KM, Freeman GJ, Coukos G. Dual blockade of PD-1 and CTLA-4 combined with tumor vaccine effectively restores T-cell rejection function in tumors. Cancer Res (2013) 73:3591–603. doi:10.1158/0008-5472.CAN-12-4100
125. Lund AW, Wagner M, Fankhauser M, Steinskog ES, Broggi MA, Spranger S, et al. Lymphatic vessels regulate immune microenvironments in human and murine melanoma. J Clin Invest (2016) 126:3389–402. doi:10.1172/JCI79434
126. Eyles J, Puaux AL, Wang X, Toh B, Prakash C, Hong M, et al. Tumor cells disseminate early but immunosurveillance limits metastatic outgrowth in a mouse model of melanoma. J Clin Invest (2010) 120(6):2030–9. doi:10.1172/JCI42002
127. Hodi FS, O’Day SJ, McDermott DF, Weber RW, Sosman JA, Haanen JB, et al. Improved survival with ipilimumab in patients with metastatic melanoma. N Engl J Med (2010) 363:711–23. doi:10.1056/NEJMoa1003466
128. Topalian SL, Hodi FS, Brahmer JR, Gettinger SN, Smith DC, McDermott DF, et al. Safety, activity, and immune correlates of anti-PD-1 antibody in cancer. N Engl J Med (2012) 366:2443–54. doi:10.1056/NEJMoa1200690
Keywords: lymphatic endothelial cells, T cell, lymph node, cancer, inflammation, tolerance, cytokine
Citation: Yeo KP and Angeli V (2017) Bidirectional Crosstalk between Lymphatic Endothelial Cell and T Cell and Its Implications in Tumor Immunity. Front. Immunol. 8:83. doi: 10.3389/fimmu.2017.00083
Received: 02 December 2016; Accepted: 18 January 2017;
Published: 06 February 2017
Edited by:
Sonia Elhadad, Weill Cornell Medical College, USACopyright: © 2017 Yeo and Angeli. This is an open-access article distributed under the terms of the Creative Commons Attribution License (CC BY). The use, distribution or reproduction in other forums is permitted, provided the original author(s) or licensor are credited and that the original publication in this journal is cited, in accordance with accepted academic practice. No use, distribution or reproduction is permitted which does not comply with these terms.
*Correspondence: Veronique Angeli, micva@nus.edu.sg