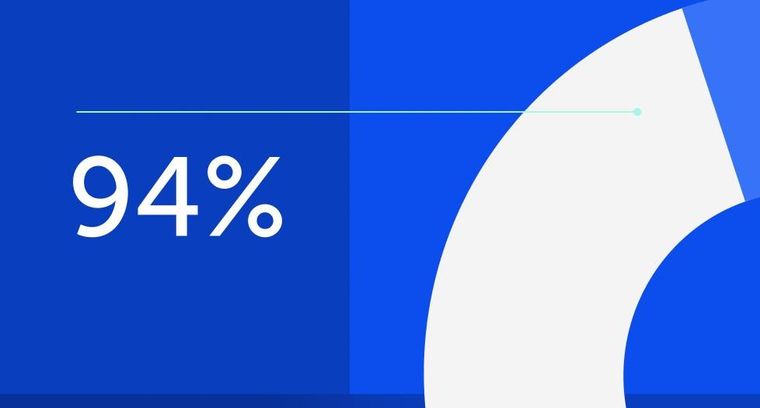
94% of researchers rate our articles as excellent or good
Learn more about the work of our research integrity team to safeguard the quality of each article we publish.
Find out more
REVIEW article
Front. Immunol., 17 November 2016
Sec. Alloimmunity and Transplantation
Volume 7 - 2016 | https://doi.org/10.3389/fimmu.2016.00507
This article is part of the Research TopicCellular therapies: past, present and futureView all 9 articles
The timely reconstitution and regain of function of a donor-derived immune system is of utmost importance for the recovery and long-term survival of patients after allogeneic hematopoietic stem cell transplantation (HSCT). Of note, new developments such as umbilical cord blood or haploidentical grafts were associated with prolonged immunodeficiency due to delayed immune reconstitution, raising the need for better understanding and enhancing the process of immune reconstitution and finding strategies to further optimize these transplant procedures. Immune reconstitution post-HSCT occurs in several phases, innate immunity being the first to regain function. The slow T cell reconstitution is regarded as primarily responsible for deleterious infections with latent viruses or fungi, occurrence of graft-versus-host disease, and relapse. Here we aim to summarize the major steps of the adaptive immune reconstitution and will discuss the importance of immune balance in patients after HSCT.
The reconstitution of different immune cell subsets after allogeneic hematopoietic stem cell transplantation (HSCT) (Figure 1) occurs at different time points summarized in Table 1. After conditioning therapy, patients undergo an “aplastic phase” (severe neutropenia or pre-engraftment phase) until neutrophils recover. The total nucleated cell (TNC) dose and CD34+ cell dose within the graft source are important factors contributing to the rate of engraftment and outcome after HSCT. Umbilical cord blood (UCB) grafts contain lower TNC levels compared to bone marrow transplant (BMT) and peripheral blood stem cell transplant (PBSCT), what increase the time of neutrophil engraftment from ~14 days after PBSCT and 21 days after BMT to 30 days after UCB transplantation (1, 2). Moreover, recent study showed that high TNC cell dose was associated with improved overall survival (OS), decreased relapse, and increased incidence of chronic graft-versus-host disease (GvHD) in patients receiving PBSCT (3). On the other hand, it has been presented that patients with higher CD34+ dose within PBSCT had faster platelet engraftment, but lower OS and increased relapse (4).
Figure 1. Overview of immune cell differentiation. The figure shows the different types of immune cells and their development from different precursors. The reconstitution of innate immunity occurs rapidly within 20–30 days after allogeneic HSCT while reconstitution of adaptive immunity is delayed following HSCT and can require up to 1 year. Natural killer (NK) cells, monocytes, granulocytes, and dendritic cells are derived from myelomonocytic progenitor cells. B and T cells differentiate from lymphoid progenitor cells and require specialized microenvironments in order to efficiently differentiate from primitive progenitors, and typically show delayed and incomplete recovery. Reprinted by permission from Macmillan Publishers Ltd.: Bone Marrow Transplantation (5), copyright (2005).
The infections encountered during the pre-engraftment phase consist primarily of bacterial and fungal infections that are reasonably well controlled by medications given for prophylaxis and treatment (6) (Figure 2). The first 100 days after HSCT (engraftment phase) are characterized by cellular immunodeficiencies due to a reduced number of natural killer (NK) cells of the innate immune system and T cells of the adaptive immune system. This renders patients especially susceptible to viral reactivations including cytomegalovirus (CMV) and Epstein–Barr virus (EBV) as well as viral diseases (7, 8).
Figure 2. Time line of complications after allogeneic HSCT. The figure shows the most prevalent complications after HSCT according to the three phases of engraftment. Concomitant infectious complications consisting of bacterial, fungal, and viral infections are shown according to their occurrence as well as association with acute and chronic GvHD during different phases of follow-up: (1) pre-engraftment, (2) engraftment, and (3) post-engraftment phase. Abbreviations: CMV, cytomegalovirus; aGvHD, acute graft-versus-host disease; cGvHD, chronic graft-versus-host disease.
The recovery of the T cell compartment relies on peripheral expansion of memory T cells, driven by cytokines as well as allogeneic antigens encountered in the host, and is followed by the production of naive T cells in the thymus (5). CD4+ T cells reconstitute later than CD8+ T cells and depend more on thymic generation of CD4+CD45RA+ naive T cells after HSCT explaining the reported inversion of the CD4/CD8 ratio (9). About 3 months after HSCT, CD4+ T cell numbers of about 200/μL have been observed (10). T cell receptor (TCR) rearrangement excision DNA circles (TRECs) have been investigated as surrogate parameters for reconstitution of thymus-derived CD4+CD45RA+ naive T cells (11). TREC levels remain low until 3–6 months after allogeneic HSCT (5). A special subgroup of CD4+ cells are regulatory T cells (Tregs), which may be important for a better outcome after allogenic HSCT (12). Tregs suppress the activity of effector T cells, thus reducing inflammation and promoting immune homeostasis after allogenic HSCT (13). Clinical, preclinical, and experimental models have shown that Treg reconstitution plays a critical role in amelioration of GvHD while preserving the graft-versus-leukemia (GvL) effect (14, 15). Increasing age is associated with thymic atrophy and loss of function (16). Cycling of mature lymphocytes maintains numbers of mature T cells by homeostatic peripheral expansion (5). Naive CD4+ and CD8+ T cells rely on interleukin-7 (IL-7) and TCR engagement for survival and expansion (17). CD8+CD27+ memory T cells can be maintained and expanded by cytokine signals alone involving IL-7 and interleukin-15 (IL-15) (18). In older patients, the lack of CD4+CD45RA+ naive T cells with a broad TCR repertoire leads to an increased risk for opportunistic infections and probably also to increased risk of leukemic relapse (19, 20). The lack of CD4+CD45RA+ naive T cells is additionally aggravated by GvHD (21, 22).
The B cell compartment representing the humoral immunity is the slowest to reconstitute and may take up to 5 years after allogeneic HSCT. Transitional CD19+CD21lowCD38high B cells are the first B cells emigrating from the bone marrow (BM) and are elevated in the peripheral blood (PB) in the first months after HSCT before their percentage progressively decreases, while the proportion of more mature B cell subpopulations increases (23). The lack of CD19+CD27+ memory B cells, decreased levels of circulating immunoglobulins, impaired immunoglobulin class switching, and a loss of complexity in immunoglobulin gene rearrangement patterns leave allogeneic HSCT patients vulnerable to encapsulated bacteria such as Streptococcus pneumoniae and Haemophilus influenzae (1, 24). In this review, we summarize the reconstitution of the adaptive immunity and discuss the importance of achieving immune balance after HSCT.
Patients undergoing HSCT often experience late recovery of B cell numbers leading to a defect of B cell mediated immunity. Generally, B cell numbers recover to normal counts within 12 months after HSCT (25), although complete recovery may take up to 2 years. In the first few months, very few circulating B cells have been observed (25, 26) and within 1–2 years following HSCT, B cell numbers reach levels exceeding normal adult individual ones followed by gradual decline, similarly to the normal ontogeny in young children (26). First B cells emerging into the periphery are CD19+CD21lowCD38high transitional B cells, which subsequently decrease in percentages while mature CD19+CD21highCD27− naive B cells are being replenished (1, 23). Transitional B cells were first described as CD24highCD38high (23). Later on, another marker of transitional B cells was identified, distinguishing between T1 and T2 transitional cells. T1 cells were reported as CD21low and described as the first B cell population emigrating from the BM, which subsequently differentiate toward CD21+, T2 phenotype and serve as precursors of the CD19+CD21highCD27− naive B cell pool in PB and tissues (27). Complete reconstitution of the B cell compartment includes the recovery of both CD19+CD21highCD27− naive and CD19+CD27+ memory B cells. Reconstitution of memory B cells occurs upon environmental or vaccine-based antigen exposure and requires CD4+ T cell help (28). Complete CD19+CD27+ memory B cell development may take up to 5 years after HSCT (26). In the study by Corre and colleagues, numbers of CD19+CD21highCD27− naive B cells normalized by 6 months and reached above normal values around 24 months after myeloablative conditioning for allogeneic HSCT (29). CD19+CD27+ memory B cells remained persistently low during the 2 years of follow-up (29). Other authors similarly reported relatively fast naive B cell reconstitution followed by delayed memory B cell recovery (30, 31). In addition, early expansion of CD19+CD5+ B cells has been reported (29, 32), a subset described as pre-naive circulating B cells representing a distinct intermediate phenotype between transitional and naive B cells (33). These cells showed only partial responses to B cell receptor (BCR) stimulation and CD40 ligation, but similarly to CD19+CD21highCD27− naive B cells, these were capable to differentiate into plasma cells and had the ability to function as antigen-presenting cells (APCs) (33).
In the first 2 years following allogeneic HSCT, B cell function remains compromised. Different B cell subpopulations often reconstitute over a different period of time contributing to a defective humoral response. Delayed T cell recovery and the reversed CD4/CD8 ratio may also contribute to low circulating B cell numbers following HSCT (26). Furthermore, CD19+CD27+ memory B cells can be influenced by low T helper cells as they require their help for isotype switching (26). In addition, somatic hypermutation seems to be diminished even in the presence of normal donor CD4+ T cell numbers, implying an environmental defect (26, 34). Normal levels of serum IgM are usually measurable 3–6 months after HSCT (35, 36), followed by normalization of serum IgG1/IgG3, IgG2/IgG4, and IgA similar to that observed during normal development in the first years of life (37). However, in some patients, long-term antibody class deficiencies have been reported (38). The immunoglobuline heavy chain (IgH) repertoire is often characterized by delayed class switching and oligoclonal dominance with specific rearrangements dominating at different time points in these patients (36, 39). Measurement of B lymphocyte repertoire diversity by analysis of IgH complementarity determining region 3 (CDR3) revealed limited variation of IgH CDR3 repertoire in CD19+CD27+ memory B lymphocytes compared to CD19+CD21highCD27− naive B cells at 3 and 6 months after allogeneic HSCT. Decrease in CD19+CD27+ memory B cell IgH CDR3 repertoire, but not CD19+CD21highCD27− naive B cell one, was also observed when compared to healthy controls suggesting a role of CD19+CD27+ memory B cells in oligoclonal restriction (35). Both CD19+CD27+ memory B cells and CD19+CD21highCD27− naive B cells reach normal diversity, comparable to healthy individuals, 12 months after HSCT (35).
Different settings of HSCT may also influence B cell recovery. Patients receiving antithymocyte globulin-fresenius (ATG-F) presented delayed CD19+ B cell recovery up to 5 months after HSCT compared to non-ATG-F patients (40). ATG is a potent immunosuppressant administrated before HSCT to prevent graft rejection and to reduce incidence of acute and chronic GvHD in patients receiving grafts from unrelated donors (40, 41). Absolute CD19+ B cells normalized 1 year after HSCT in both groups. ATG-treated patients had significantly worse CD19+CD21highCD27− naive B cell and CD19+CD27+ memory B cell regeneration within the first month after HSCT indicating a negative impact of ATG on B cell immune reconstitution (40). Depending on the brand, ATG may also have immunomodulatory effects on B cells (42). Slow B cell recovery has been observed in patients receiving non-myeloablative conditioning compared to those given myeloablative therapy, with reduced B cell numbers observed in most patients up to 12 months after non-myeloablative therapy for HSCT (43). However, these findings may in part be explained by older patient age and higher incidence of acute GvHD in this patient cohort (43). Both acute and chronic GvHD have been associated with delayed B cell reconstitution, and reduction or lack of B cell precursors in the BM has been observed in these patients compared to patients without GvHD (44). In a study on 93 allograft recipients, the number of BM B cell precursors on day 30 after HSCT was significantly lower in patients later developing grades 2–4 acute GvHD compared to patients with grades 0–1 disease (44). Moreover, patients developing extensive chronic GvHD within 1 year after transplantation had lower percentages of B cell precursors on day 365 compared with patients without chronic GvHD or with limited chronic GvHD (44). However, the effect of acute and chronic GvHD could not be separated from the possible influence of glucocorticoid treatment in this study due to low patient numbers suggesting B cell deficiency after transplantation may in part be a result of inhibition of B lymphopoiesis by GvHD and/or its treatment (44). In addition, a decrease of absolute CD19+ B cells in patients at first diagnosis of chronic GvHD and a disturbance of B cell homeostasis in patients with active chronic GvHD have been observed (45, 46). Stem cell source may also influence numbers of circulating B cells with higher counts detected in recipients of peripheral blood stem cells (PBSC); however, this observation may be attributed to the higher amount of mature B cells in PBSC grafts compared with BM (44, 47, 48).
Even patients who show recovery of overall CD19+ B cell numbers are not considered fully immunocompetent and as a result of decreased B cell function, impaired vaccine responses to infectious antigens have been observed (26). Lack of CD19+CD27+ memory B cells, decrease of circulating immunoglobulins, and impaired immunoglobulin gene rearrangement render these patients susceptible to encapsulated bacteria and viruses (1, 24).
CD4+ and CD8+ T cells reconstitute within the first year after HSCT and enable defense against viral or fungal infections, as well as maintaining the GvL effect. A subset of CD4+ T cells are so called regulatory T cells (Tregs). In the next paragraph, we aim to summarize their development and function in patients after allogeneic HSCT.
Tregs are a subset of CD4+ T cells whose function is to suppress immune responses and maintain self-tolerance (49). A transcription factor called FoxP3, a member of the fork head family of transcription factors, is critical for the development and function of Tregs and is used as a definite marker to identify Tregs (49, 50). Tregs are a functionally mature subpopulation of T cells and can also be induced from CD4+CD45RA+ naive T cells in the periphery (51). Natural Tregs (nTregs) are derived from the thymus and are characterized by the co-expression of CD4, high expression of CD25 and FoxP3 (52). Induced or adaptive Tregs (iTregs) are generated in peripheral lymphoid organs in the presence of transforming growth factor beta (TGF-β) (53) (Figure 3).
Figure 3. Development of natural and induced regulatory T cells. Natural regulatory T cells (nTregs) are derived from the thymus and are characterized by the co-expression of CD4, high expression of CD25 and FoxP3, and are collectively represented as CD4+CD25+FoxP3+ Tregs. Induced or adaptive regulatory T cells (iTregs) are generated in the peripheral lymphoid organs in the presence of transforming growth factor beta (TGF-β) and interleukin-2 (IL-2).
Some recent studies have shown that nTregs are more stable than iTregs in relation to their differential DNA methylation profiles and other epigenetic regulations of FoxP3 (54, 55).
Tregs can downregulate immune responses by (a) production of inhibitory cytokines and (b) a contact-mediated effect on APCs. Tregs produce the anti-inflammatory cytokine interleukin-10 (IL-10) that inhibits production of interleukin-12 (IL-12) by activated dendritic cells (DCs) and macrophages (56, 57). IL-10 also inhibits the expression of co-stimulators and major histocompatibility complex (MHC) class II molecules on DCs and macrophages and thus inducing tolerance within the immune system (56–58). Another anti-inflammatory cytokine produced by Tregs, TGF-β, inhibits the proliferation and effector functions of T cells and the activation of macrophages (59, 60). TGF-β also regulates the differentiation of functionally distinct subsets of T cells, stimulates production of immunoglobulin A (IgA) antibodies, promotes tissue repair after local immune and inflammatory reactions subside, and confers Treg-mediated immune reconstitution (56–58, 61). Tregs play a major role in regulation of epithelial inflammation and are strongly influenced by the interaction with the epithelial microbial environment (62, 63).
Tregs play an indispensable role in both solid organ transplant tolerance and in allograft tolerance after HSCT. In rodents and humans, a subpopulation of thymus-derived naive CD4+ T cells that co-express the IL-2R alpha chain, CD25, have potent suppressive activity (64). Tregs mediate transplantation tolerance in experimental models of skin and/or solid organ transplantation (65) as well as tolerance to BM allografts (66). By allogeneic HSCT, malignant and non-malignant hematological disorders can be cured, but at the same time, treatment efficacy is limited due to occurrence of GvHD (67). Regulatory T cells have received considerable attention in recent years due to their ability to suppress the proliferation of conventional T cells when added to donor grafts and prevention of GvHD in animal models (68). Using a mouse model, Edinger and colleagues have shown that CD4+CD25+ Tregs suppress GvHD after BMT without abrogating the GvL or graft-versus-tumor (GvT) effect (14) supporting the importance of Tregs in allogenic HSCT. Furthermore, Nguyen and colleagues demonstrated that the adoptive transfer of Tregs preserved thymic and lymphoid architecture of the host and hence accelerated posttransplant T cell immune reconstitution in a murine GvHD model (69).
Taylor and colleagues demonstrated that in vivo depletion of CD25+ T cells and depletion of CD25+ T cells in the transplant inoculum, worsened GvHD whereas adoptive transfer of CD4+ CD25+ nTregs together with the BM graft ameliorated GvHD (70).
While an increasing number of publications have focused on the biology of CD4+CD25+Foxp3+CD45ROlo naive Tregs in GvHD, less attention has been given to iTregs, probably due to the lack of proven cell surface marker that differentiate nTregs from iTregs. Fantini and colleagues demonstrated that iTregs can be generated from CD4+ T cells in the presence of TGF-β and can be expanded in culture (71). On the other hand, Koenecke and colleagues showed that administration of in vitro generated iTregs along with BM grafts containing alloreactive donor T cells did not provide any significant protection from lethal GvHD, due to limited in vivo survival of these cells (72). They also demonstrated that iTregs lost their Foxp3 expression, along with a loss of suppressive function early after transplantation, thus making iTregs unsuitable for use in a therapeutic approach (72) if administered as an external cellular product. Not only iTregs but also nTregs have been shown to loose Foxp3 expression in a STAT3-dependent manner and can revert to a proinflammatory phenotype under inflammatory conditions (73). Therefore, inflammation seems to affect Foxp3 expression in both natural and induced Tregs.
Although Tregs have long been assumed to be solely a subset of the CD4+ T cell compartment, a CD8+ Treg population has been recently described and shown to be capable of suppressing T cell responses (74). In terms of GvHD, Robb and colleagues reported that CD8+Foxp3+ Tregs suppressed GvHD and attenuated GvHD mortality after BMT in a mouse model (75). Interestingly, CD8+Foxp3+ cells were more suppressive than CD4+Foxp3+ cells (75). Using a rat model, Xystrakis and colleagues provided a first report on CD8+ Tregs conferring their regulatory properties via a cell to cell contact dependent mechanism to prevent GvHD and thus confirming CD8+Foxp3+ Tregs in a second species (76). Clinical studies on CD8+ Tregs at a functional level are scarce to date. However, Zheng and colleagues reported that human CD8+ Tregs potentially inhibit GvHD without compromising general immunity and GvL activity in humanized mouse models (77). Taken together, these findings provide an insight into the efficacy of both CD4+ and CD8+ Tregs as potential novel therapeutic approaches in clinic.
Many researchers have focused on evaluating Treg cell numbers after HSCT, since they play an important role in the amelioration of GvHD. Using PB of patients after transplantation, Li and colleagues demonstrated that the frequency of CD4+CD25+ Tregs was significantly downregulated in patients with severe acute or chronic GvHD (78). They also showed that a decreased level of CD4+CD25+ Tregs correlated with increased severity of GvHD (78). While the majority of studies focused on blood derived Tregs, there is little information on Tregs isolated from intestinal tissues due to the lack of availability of repeated gut biopsies. Using immunoenzymatic labeling, Rieger and colleagues were the first to demonstrate that infiltrating Tregs decreased the signs of acute and chronic GvHD in intestinal mucosa (79). They showed that patients with acute and chronic GvHD had a complete lack of counter regulation indicated by a Foxp3+/CD8+ T cell ratio identical to that of healthy individuals, while this ratio was increased in patients without GvHD (79). These results have been discussed controversially in the literature since Lord and colleagues demonstrated that Foxp3+ T cells were not decreased in PB or gastrointestinal tissues and that the frequency of Tregs did not correspond to disease incidence or severity (80). On the contrary, these investigators reported that Foxp3+ T cells were significantly upregulated in GvHD-afflicted intestinal mucosa when compared to non-GvHD mucosal tissues (80). This finding was further supported by Ratajczak and colleagues who observed an increased proportion of CD4+Foxp3+ T cells in patients with grades 2–4 compared to grades 0–1 acute GvHD (81). One possible explanation for these conflicting results may be the difficulty to discriminate natural and induced Tregs. It is possible that nTregs are decreased in GvHD while iTregs may be increased in order to compensate for the exaggerated inflammation during GvHD. Imanguli and colleagues observed an upregulation of functional markers such as CD3+, CD4+, CD27+, ICOS+, and CD39+ in Tregs that traffic into tissue including skin and oral mucosa exerting a suppressive function in patients with chronic GvHD (82). Interestingly, normal numbers of activated CD45−Foxp3hi Tregs were observed in tissue and PB of patients with chronic GvHD whereas naive or resting CD45RA+Foxp3+ Tregs that presumably control chronic GvHD effector cells were reduced compared to patients without chronic GvHD.
Studies in mouse models of GvHD have provided information on the suppressive nature of Tregs and their potential to suppress and ameliorate GvHD without impairing the GvL effect. The first clinical trial using Tregs to suppress acute/chronic GvHD in patients were completed recently. This “first-in-man-study” reported the adoptive transfer of ex vivo expanded CD4+CD25+CD127− Tregs in one patient with chronic GvHD and another with acute GvHD after HSCT with an HLA-identical sibling donor (83). Transfer of Tregs resulted in a reduction of the steroid dose administered, increased levels of circulating Tregs, and a decrease in inflammatory cytokine levels in the PB (83). Another “first-in-man-study” was reported after double UCBT in 23 patients, who received in vitro expanded 0.1–30 × 105 UCB CD4+CD25+CD127− Tregs per kilogram derived from partially HLA-matched third-party UCB units (15). There was a significant reduction in the incidence of acute GvHD grades II–IV (43 versus 61%, P = 0.05) when compared to 108 historical controls without transfusion of Tregs. No toxicities, infections, relapse, or early mortality were observed suggesting that UCB Tregs could be beneficial for preventing acute GvHD (15). Furthermore, Di Ianni and colleagues reported a clinical trial in 28 patients receiving adoptively transferred CD4+CD25+CD127− Tregs after T-cell-depleted haploidentical HSCT without further immunosuppression (13). Only 2 out of 28 patients developed grades II–IV acute GvHD and no chronic GvHD was observed. They showed that adoptive transfer of freshly isolated donor-derived Tregs 4 days before inoculating the CD34+ stem cells prevented acute and chronic GvHD in the absence of further immunosuppression. Tregs promoted lymphoid reconstitution, improvement of immunity to opportunistic pathogens (no CMV-related death of patients) without abrogating the GvL effect (13). In addition, Hoffmann reported in vitro expansion of highly purified polyclonal human CD4+CD25high Tregs through the use of artificial APCs for repeated stimulation via CD3 and CD28 in the presence of high-dose IL-2 (84). These cells not only maintained their phenotype and expressed suppressive activity but also maintained the expression of the lymph node homing receptors l-selectin and CCR7 (84). Furthermore, the same group reported results of a small phase I safety and feasibility trial where freshly isolated donor-derived CD4+CD25high Tregs were infused into nine patients with high risk for leukemic relapse after cessation of systemic GvHD prophylaxis (12). After 8 weeks, additional CD4+ T cells were administered to promote GvL activity. Patients showed no signs of GvHD nor opportunistic infections or early disease relapse supporting the safety and efficacy of Treg transfusion (12). This has led to a phase II clinical trial for the treatment of patients with steroid-refractory acute GvHD using freshly isolated CD4+CD25high Tregs that is currently ongoing. Taken together, these early trials suggest that Tregs could be a novel approach for prophylaxis and treatment of patients with acute GvHD in larger clinical trials. The impact of Treg transfusion on the immune reconstitution has to be further investigated.
Tregs induce tolerance and maintain immune homeostasis (51). A major challenge of Treg cell therapy is their relative scarcity in PB (0.5–1% of CD4+CD25high T cells) (85). In 2011, Hippen and colleagues presented two individual reports regarding the generation of induced Tregs on a large scale (86) and ex vivo expansion of natural Tregs (86). Both methods focus on the development of expansion protocols for either type of Tregs to obtain higher yields for clinical trials on treatment or prevention of GvHD (86). In patients with chronic GvHD, Matsuoka and co-workers reported that daily administration of low-dose IL-2 induced selective expansion of functional CD4+CD25+CD127− Tregs, improved chronic GvHD, restored CD4+ T cell homeostasis, and promoted the reestablishment of immune tolerance (87). Koreth et al. reported the case of 29 chronic GvHD patients that the administration of subcutaneous low dose IL-2 rapidly induced preferential and sustained Tregs expansion without any immune impairment (88). This suggests that low-dose IL-2 could be a potential therapy to restore immune balance after HSCT. Another approach to manipulate Tregs in vivo was reported by Furusawa and colleagues (89). Clostridial products, like short chain fatty acids (SCFA) or mainly butyrate, can induce the differentiation of colonic Tregs in vitro and in vivo in mouse models (89). This points toward the necessity of host–microbiome interaction to establish immunological tolerance and homeostasis in the gut. Moreover, Mathewson and colleagues reported that restoring clostridial metabolites or the strain itself modulated intestinal epithelial cell integrity and mitigated GvHD in mice (63). Taken together, these findings strongly suggest that the right balance of gut microbiome may be crucial to induce Tregs for intestinal tolerance.
Memory T cells [central memory (TCM) and effector memory (TEM)], tissue resident memory cells (TRM), and effector cells (TEFF) cells are essential to control viral reactivations after allogeneic HSCT. Upon encountering antigens, memory cells differentiate to TEFF and lyse the infected cells and secret proinflammatory cytokines [e.g., IFN-γ and tumor necrosis factor-α (TNF-α)] (90). Immune surveillance of TCM occurs trafficking through secondary lymphoid organs, TEM and TEFF, through non-lymphoid organs (91). In contrary, TRM cells reside at various sites (e.g., liver, lungs, gut, and skin) and provide immediate antiviral response (cytotoxicity and secretion of IFN-γ) without trafficking (92). The reconstitution of CD4+CD45RA+ naive T cells, providing the broad range of TCR repertoire needed to control infections and to avoid the reappearance of leukemic cells, is essential after allogeneic HSCT (11, 93). The conditioning regimens applied, increasing patient age and occurrence of acute and chronic GvHD, have devastating effects on thymic function after HSCT (28, 93–95). Reconstitution of CD4+CD45RA+ naive T cells can be demonstrated by measuring TRECs. Immune reconstitution of CD4+ and CD8+ T cells is also essential for maintaining a GvL effect (1). Reconstitution of CD8+ T cells is faster than that of CD4+ T cells, which usually occurs around day +100 or later and is indicated by the inversion of the CD4+/CD8+ T cell ratio (1) early after HSCT (Table 1). The time period until complete reconstitution of CD4+ T cells can take up to 2 years after allogenic HSCT (96).
Acute GvHD is one of the severe complications occurring early after HSCT contributing substantially to non-relapse mortality (NRM). Development of acute GvHD is influenced by human leukocyte antigen (HLA) disparities or gender mismatches between donor and recipient, the intensity of the conditioning regimen applied, CMV reactivation, and the stem cell source (97, 98). Acute GvHD can also occur in the HLA-identical transplant setting (siblings or matched unrelated donors) due to minor histocompatibility antigen differences between donor and recipient (98). Acute GvHD is an immune response directed against the host immune system, tissues, and organs (99, 100). GvHD by itself can inhibit T cell functions by limiting TCR diversity, T cell development, and dysfunction in cytokine production, most likely through damage of the BM and/or thymus, apoptosis, and release of cytokines in a so-called “cytokine storm” (101).
Bone marrow gives rise to all hematopoietic lineages and is the homing site for memory cells of the adaptive immunity (102). Recently, BM has been established as an additional target of alloreactivity observed during GvHD leading to the depletion of both hematopoietic progenitors and niche-forming cells (103), resulting in disrupted hematopoiesis and delayed immune reconstitution (104). Along with the BM, the thymus plays an important role in the maturation of hematopoietic precursors and T cell development (93). Acute GvHD substantially decreases thymic output and thus recovery of CD4+ T cells and diversified T cell repertoires (93). Acute GvHD leads to a further skewing of the TCR repertoires of both CD4+ and CD8+ T cells as well as antigen-specific T cells (99). Both T lymphopenia and inadequate repertoire of CD4+ and CD8+ T cells for at least 1 year after transplant foster recurrent infections with latent viruses.
In addition, treatment of patients with acute GvHD with corticosteroids or other immunosuppressive drugs increases the risk of viral reactivations (98, 105). It has been reported that the risk of CMV infections is directly related to the dose and duration of steroid administration (106). Administration of high doses of steroids was shown to be an independent risk factor for impaired functional recovery of CMV-specific CD4+ and CD8+ T cells (106). Moreover, Özdemir and colleagues reported that steroids induced a significant impairment of CD8+ T cells for producing TNF-α (107).
Although T cell depletion (TCD) of the stem cell graft reduces GvHD, it is associated with delayed immune reconstitution, infectious complications, and an increased risk of relapse (108). Thus, ex vivo T-cell depletion by either CD34+ cell selection or CD3+/CD19+ cell depletion has not been routinely performed and repletion protocols have been widely studied [e.g., HSV-Tk-transduced T cell transfer, other donor lymphocyte infusion-based protocols (109, 110)]. In vivo T cell reducing or impairing agents include ATG [e.g., ATG-Fresenius; Germany, or thymoglobulin (thymo); Genzyme; USA] or anti-CD52 antibody (alemtuzumab or campath), a particularly powerful reagent for immunosuppression (108, 111). ATG administration leads to prolonged immunosuppression of both CD4+ T cells and CD4+CD25+CD127− Treg cells (111) and appears to have less severe effect on immune reconstitution when compared to campath (112).
The source of stem cells can impact on both complications as well as time to immune reconstitution after allogeneic HSCT (Table 2). Investigators reported that the source of stem cells is a predictive factor for recovery of CMV-specific cytotoxic T lymphocytes (CTL) (10). Recipients of PBSCs had improved functional CMV–CTL recovery and earlier CMV-specific CD4+ T cell reconstitution than patients given BM grafts (106, 113). These findings can be explained by the fact that PBSC grafts compared to BM contain more lymphocytes and higher numbers of CD4+CD45RO+ memory T cells (114).
Graft-versus-leukemia is defined as an immune response directed against leukemia/tumor cells of the recipient after allogeneic HSCT. Over the years, several studies have shown that CD4+ and CD8+ T cells play an important role in establishing a GvL effect through various mechanisms such as cytotoxic T cells releasing granzyme B and apoptosis mediated by FAS ligands (115). GvL is often associated with GvHD, but GvL responses against, e.g., minor histocompatibility antigens solely expressed on hematopoietic cells (mHA1) may be specifically directed against leukemic cells or the recipients’ hematopoietic cells. The precise role of CD4+ and CD8+ T cells for achievement of a GvL effect is not clearly understood today (115, 116). Complete depletion of T cells by CD34+ cell selection leads to a high incidence of relapse, resulting in death in about 20–50% of patients (117). T cell repletion or donor lymphocyte infusions (DLI) can prevent relapse, but can lead to a higher probability of acute and chronic GvHD (118, 119). Several protocols tried to circumvent the problem of increased acute and chronic GvHD by delayed add-back of genetically modified T cells (109) or other manipulations of the donor’s lymphocytes such as selection of either CD4+ or CD8+ T cells prior to transfusion (120, 121).
T cells are the most important effector cells in the control of viral infections. Thus, T cell reconstitution after allogeneic HSCT has a significant impact on the control of infectious complications. The first phase of virus-specific T cell reconstitution and expansion early after HSCT depends on the transfer of mature (effector, memory, or naive) virus-specific T cells within the donor graft and the resident antigen-specific cells (10, 122). Viral infections occur mostly between engraftment and day +90 posttransplant (123). However, also late (after day +90) and recurrent CMV reactivations have been observed, which have been associated with impaired reconstitution or function of antiviral immunity (106). CMV is a latent virus, which belongs to the family of herpesviruses and is one among the common viral pathogens that can reactivate after HSCT. It reactivates in about 60–70% of CMV-seropositive patients, and the primary infection affects 20–30% of CMV seronegative recipients transplanted from CMV-seropositive donors (124). Uncontrolled CMV reactivations can lead to a life-threatening, multi-organ CMV disease such as retinitis, gastroenteritis, or pneumonia (125–127). Advances in CMV monitoring, preemptive antiviral therapy, and quantification of CMV–CTLs are crucial in the prevention of CMV disease (128). The most important risk factors for CMV infection include recipient CMV-seropositivity, TCD of the graft, and acute GvHD (123). Early reconstitution of antiviral immunity remains an essential issue for the control of CMV reactivations after HSCT. The recovery of both CD8+ and CD4+ CMV-specific T cells may be a marker for protection against CMV reactivations (129).
Epstein–Barr virus infection is also a frequent viral complication after allogeneic HSCT, which may progress to EBV-associated posttransplant lymphoproliferative disease (PTLD) that causes unspecific symptoms such as fever and lymphadenopathy with a high viral load in the PB (130). These complications are mediated by several risk factors including TCD combined with reduced intensity conditioning (RIC) leading to impaired anti-EBV T cell-mediated immunity and persistence of residual recipient B cells (131). In addition, HLA disparity and acute GvHD have also been known to increase the risk of PTLD due to the delayed or impaired specific immune reconstitution (132).
However, other viral pathogens such as adenovirus (ADV), human herpes virus 6 (HHV6), BK-polyoma virus (BKV), and respiratory viruses occur less frequently in adult patients in comparison to CMV and EBV after allogeneic HSCT (133). The control of these viruses again depends upon the reconstitution of antiviral immunity.
Among the viruses mentioned above, T cell immune reconstitution against CMV has been studied most intensively and will be described in more detail below, as an example for virus (or any antigen)-specific T cell reconstitution and expansion. Apart from the above mentioned factors influencing T cell reconstitution (TCD of the graft; stem cell source, occurrence of acute or chronic GvHD), CMV serostatus of patient and donor is one of the most important variables influencing CMV-specific T cell immune reconstitution. CMV-seropositive recipients and donors (R+D+) have much faster reconstitution of CMV–CTLs (prior to day +50) and a subclinical CMV reactivation can even boost this development (106). On the other hand, CMV-seropositive recipients transplanted from CMV-seronegative donors (R+D−) lack the protective donor-derived immunity and hence have delayed recovery of antiviral immunity (between days +120 and +150) and a higher risk for recurrent CMV reactivations (134). In Figure 4, examples of patients with typical CMV–CTL immune reconstitution for R+D+ and R+D− groups are shown to demonstrate the impact of CMV serostatus on CMV–CTL immune reconstitution. Additionally, it has been shown, that CMV–CTLs of recipient origin can survive the conditioning regimen and can add to the protection against CMV, especially in R+D− patients (135).
Figure 4. Recovery of CMV-specific cytotoxic T lymphocytes after HSCT. Examples of reconstitution of CMV-specific cytotoxic T lymphocytes (CTLs) after HSCT for CMV-seropositive recipients transplanted from CMV-seropositive donors (R+/D+) (A) and CMV-seropositive recipients transplanted from CMV-seronegative donors (R+D−) (B) are shown. CMV–CTL numbers per microliter of whole blood (left y-axis) were plotted against the time after HSCT (days). The right y-axis shows the number of pp65-positive cells/400,000 leukocytes (detection of CMV-reactivation). The CMV R+D+ patient had a CMV-reactivation by day +39 and responded by an expansion of CMV–CTLs. No significant reconstitution of CMV–CTLs within the CMV R+D− patient was detected until day +100 despite the early CMV reactivation. Adapted from Ref. (136).
Recent technological developments in cellular immunology have aided in the understanding of antigen-specific T cell responses and the antiviral immunity after HSCT. With the instigation of multimer (e.g., tetramer and streptamer) technology, antigen-specific T cells are readily detected and isolated without stimulation (137–139). In order to study those cell functions, we can choose from a broad variety of assays including cytokine secretion assays, ELISPOT, intracellular staining, which require stimulation of cells with viral lysates, viral proteins, or peptides (137). As for CMV, immunity toward CMV immunodominant epitopes, which include pp65 and IE-1 antigens have been most intensively studied (140, 141).
Initiation of multimer technology allowed the investigation of CMV–CTLs in patients after allogeneic HSCT in order to search for a protective threshold (113, 137, 142). A chronological overview of selected publications on monitoring of CMV-specific T cell responses after HSCT, with the focus on the protective numbers of CMV–CTLs is provided in Table 3. It has been shown that the inability to control CMV reactivation following HSCT is due to the impaired function of antigen-specific CD8+ T cells rather than an inability to recover sufficient numbers of CMV-specific T cells (143). Although CMV–CD8+ CTLs have been considered as the main antiviral effector cells, CMV-specific CD4+ T cells have been shown to play a crucial role in expansion and activation of CMV–CTLs, maintaining a long-term and efficient immunity against CMV (129). It has been reported that CD4+ and CD8+ CMV-specific T cells follow similar patterns of reconstitution (144), and their functional reconstitution is correlated with the absolute CD4+ or CD8+ T cell numbers (106, 145). So far, there is no threshold for protective levels of CMV-specific T cells applicable for all patients. Sequential monitoring of individual patients for the kinetics of CMV–CTL recovers, their ability to produce cytokines and expansion upon reactivation, are currently being used to detect recurrent CMV reactivations (136) (Figure 4). In summary, both the quantity and quality of immune reconstitution are important for preventing viral infection after allogeneic HSCT.
Reconstitution of the donor-derived immune system is essential for control of infectious complications, modulation of GvHD, and relapse control, thus contributing to long-term survival. In this review, we have described the major events in immune cell reconstitution, considering the most important cell types, their approximate time of reconstitution, and their interaction after HSCT. The recovery of the innate immunity is vital, especially in the absence of CD4+CD45RO+ memory and CD4+CD45RA+ naive T cells.
Today, the understanding of CD4+CD25+CD127− regulatory T cells has advanced significantly in both preclinical and clinical models for GvHD. The remaining challenge is to generate large amounts of CD4+CD25+CD127− Tregs with high purity and stable Foxp3-expression in a cost effective way. A further clinical problem is the optimal time point of Treg application. If Tregs are administered to treat patients with steroid-refractory GvHD, there may be a substantial delay between production and application and, thus, lack of feasibility and treatment success. Furthermore, the impact of ongoing systemic immunosuppression on Treg cell function has to be considered in clinical trials. A further aspect to be solved in the future is to optimize tissue conditions for survival and expansion of T regs, as these cells are under the strong control of local microbiota especially in the main target tissues of GvHD.
EW, EH, and HG designed the review and revised it critically for important intellectual content. JO, MKJ, SG, and PV provided the draft, summarized available data, and selected the references. All the authors approved the final version of the manuscript.
The authors declare that the research was conducted in the absence of any commercial or financial relationships that could be construed as a potential conflict of interest.
The project was supported by a grant of the Marie Curie Initial Training Networks Project Number 315963 “Improving HSCT by Validation of Biomarkers & Development of Novel Cellular Therapies.”
1. Seggewiss R, Einsele H. Immune reconstitution after allogeneic transplantation and expanding options for immunomodulation: an update. Blood (2010) 115(19):3861–8. doi:10.1182/blood-2009-12-234096
2. Danby R, Rocha V. Improving engraftment and immune reconstitution in umbilical cord blood transplantation. Front Immunol (2014) 5:68. doi:10.3389/fimmu.2014.00068
3. Martin PS, Li S, Nikiforow S, Alyea EP, Antin JH, Armand P, et al. Infused total nucleated cell dose is a better predictor of transplant outcomes than CD34(+) cell number in reduced-intensity mobilized peripheral blood allogeneic hematopoietic cell transplantation. Haematologica (2016) 101(4):499–505. doi:10.3324/haematol.2015.134841
4. Remberger M, Törlén J, Ringdén O, Engström M, Watz E, Uhlin M, et al. Effect of total nucleated and CD34+ cell dose on outcome after allogeneic hematopoietic stem cell transplantation. Biol Blood Marrow Transplant (2015) 21(5):889–93. doi:10.1016/j.bbmt.2015.01.025
5. Fry TJ, Mackall CL. Immune reconstitution following hematopoietic progenitor cell transplantation: challenges for the future. Bone Marrow Transplant (2005) 35(Suppl 1):S53–7. doi:10.1038/sj.bmt.1704848
6. Moss P, Rickinson A. Cellular immunotherapy for viral infection after HSC transplantation. Nat Rev Immunol (2005) 5(1):9–20. doi:10.1038/nri1526
7. Chatzidimitriou D, Gavriilaki E, Sakellari I, Diza E. Hematopoietic cell transplantation and emerging viral infections. J Med Virol (2010) 82(3):528–38. doi:10.1002/jmv.21696
8. Gennery AR, Maggina P. Infection following haematopoietic stem cell transplantation. Paediatr Child Health (2014) 24(6):236–41. doi:10.1016/j.paed.2013.11.005
9. Huttunen P, Taskinen M, Siitonen S, Saarinen-Pihkala UM. Impact of very early CD4+/CD8+ T cell counts on the occurrence of acute graft-versus-host disease and NK cell counts on outcome after pediatric allogeneic hematopoietic stem cell transplantation. Pediatr Blood Cancer (2015) 62(3):522–8. doi:10.1002/pbc.25347
10. Luo X-H, Chang Y-J, Huang X-J. Improving cytomegalovirus-specific T cell reconstitution after haploidentical stem cell transplantation. J Immunol Res (2014) 2014:12. doi:10.1155/2014/631951
11. Toubert A, Glauzy S, Douay C, Clave E. Thymus and immune reconstitution after allogeneic hematopoietic stem cell transplantation in humans: never say never again. Tissue Antigens (2012) 79(2):83–9. doi:10.1111/j.1399-0039.2011.01820.x
12. Edinger M, Hoffmann P. Regulatory T cells in stem cell transplantation: strategies and first clinical experiences. Curr Opin Immunol (2011) 23(5):679–84. doi:10.1016/j.coi.2011.06.006
13. Di Ianni M, Falzetti F, Carotti A, Terenzi A, Castellino F, Bonifacio E, et al. Tregs prevent GVHD and promote immune reconstitution in HLA-haploidentical transplantation. Blood (2011) 117(14):3921–8. doi:10.1182/blood-2010-10-311894
14. Edinger M, Hoffmann P, Ermann J, Drago K, Fathman CG, Strober S, et al. CD4+ CD25+ regulatory T cells preserve graft-versus-tumor activity while inhibiting graft-versus-host disease after bone marrow transplantation. Nat Med (2003) 9(9):1144–50. doi:10.1038/nm915
15. Brunstein CG, Miller JS, Cao Q, McKenna DH, Hippen KL, Curtsinger J, et al. Infusion of ex vivo expanded T regulatory cells in adults transplanted with umbilical cord blood: safety profile and detection kinetics. Blood (2011) 117(3):1061–70. doi:10.1182/blood-2010-07-293795
16. Hakim FT, Memon SA, Cepeda R, Jones EC, Chow CK, Kasten-Sportes C, et al. Age-dependent incidence, time course, and consequences of thymic renewal in adults. J Clin Invest (2005) 115(4):930–9. doi:10.1172/JCI200522492
17. Bolotin E, Smogorzewska M, Smith S, Widmer M, Weinberg K. Enhancement of thymopoiesis after bone marrow transplant by in vivo interleukin-7. Blood (1996) 88(5):1887–94.
18. Guimond M, Fry TJ, Mackall CL. Cytokine signals in T-cell homeostasis. J Immunother (2005) 28(4):289–94. doi:10.1097/01.cji.0000165356.03924.e7
19. van Heijst JWJ, Ceberio I, Lipuma LB, Samilo DW, Wasilewski GD, Gonzales AMR, et al. Quantitative assessment of T-cell repertoire recovery after hematopoietic stem cell transplantation. Nat Med (2013) 19(3):372–7. doi:10.1038/nm.3100
20. Yew PY, Alachkar H, Yamaguchi R, Kiyotani K, Fang H, Yap KL, et al. Quantitative characterization of T-cell repertoire in allogeneic hematopoietic stem cell transplant recipients. Bone Marrow Transplant (2015) 50(9):1227–34. doi:10.1038/bmt.2015.133
21. Storek J, Joseph A, Dawson MA, Douek DC, Storer B, Maloney DG. Factors influencing T-lymphopoiesis after allogeneic hematopoietic cell transplantation. Transplantation (2002) 73(7):1154–8. doi:10.1097/00007890-200204150-00026
22. Storek J, Geddes M, Khan F, Huard B, Helg C, Chalandon Y, et al. Reconstitution of the immune system after hematopoietic stem cell transplantation in humans. Semin Immunopathol (2008) 30(4):425–37. doi:10.1007/s00281-008-0132-5
23. Marie-Cardine A, Divay F, Dutot I, Green A, Perdrix A, Boyer O, et al. Transitional B cells in humans: characterization and insight from B lymphocyte reconstitution after hematopoietic stem cell transplantation. Clin Immunol (2008) 127(1):14–25. doi:10.1016/j.clim.2007.11.013
24. D’Orsogna LJ, Wright MP, Krueger RG, McKinnon EJ, Buffery SI, Witt CS, et al. Allogeneic hematopoietic stem cell transplantation recipients have defects of both switched and igm memory B cells. Biol Blood Marrow Transplant (2009) 15(7):795–803. doi:10.1016/j.bbmt.2008.11.024
25. Park BG, Park CJ, Jang S, Chi HS, Kim DY, Lee JH, et al. Reconstitution of lymphocyte subpopulations after hematopoietic stem cell transplantation: comparison of hematologic malignancies and donor types in event-free patients. Leuk Res (2015) 39(12):1334–41. doi:10.1016/j.leukres.2015.09.010
26. Williams KM, Gress RE. Immune reconstitution and implications for immunotherapy following haematopoietic stem cell transplantation. Best Pract Res Clin Haematol (2008) 21(3):579–96. doi:10.1016/j.beha.2008.06.003
27. Suryani S, Fulcher DA, Santner-Nanan B, Nanan R, Wong M, Shaw PJ, et al. Differential expression of CD21 identifies developmentally and functionally distinct subsets of human transitional B cells. Blood (2010) 115(3):519–29. doi:10.1182/blood-2009-07-234799
28. Mackall C, Fry T, Gress R, Peggs K, Storek J, Toubert A. Background to hematopoietic cell transplantation, including post transplant immune recovery. Bone Marrow Transplant (2009) 44(8):457–62. doi:10.1038/bmt.2009.255
29. Corre E, Carmagnat M, Busson M, de Latour RP, Robin M, Ribaud P, et al. Long-term immune deficiency after allogeneic stem cell transplantation: B-cell deficiency is associated with late infections. Haematologica (2010) 95(6):1025–9. doi:10.3324/haematol.2009.018853
30. Storek J, Saxon A. Reconstitution of B cell immunity following bone marrow transplantation. Bone Marrow Transplant (1992) 9(6):395–408.
31. Storek J, Ferrara S, Ku N, Giorgi JV, Champlin RE, Saxon A. B cell reconstitution after human bone marrow transplantation: recapitulation of ontogeny? Bone Marrow Transplant (1993) 12(4):387–98.
32. Moins-Teisserenc H, Busson M, Herda A, Apete S, Peffault de Latour R, Robin M, et al. CD19+CD5+ B cells and B1-like cells following allogeneic hematopoietic stem cell transplantation. Biol Blood Marrow Transplant (2013) 19(6):988–91. doi:10.1016/j.bbmt.2013.03.006
33. Lee J, Kuchen S, Fischer R, Chang S, Lipsky PE. Identification and characterization of a human CD5+ pre-naive B cell population. J Immunol (2009) 182(7):4116–26. doi:10.4049/jimmunol.0803391
34. Glas AM, van Montfort EH, Storek J, Green EG, Drissen RP, Bechtold VJ, et al. B-cell-autonomous somatic mutation deficit following bone marrow transplant. Blood (2000) 96(3):1064–9.
35. Omazic B, Lundkvist I, Mattsson J, Permert J, Nasman-Bjork I. Memory B lymphocytes determine repertoire oligoclonality early after haematopoietic stem cell transplantation. Clin Exp Immunol (2003) 134(1):159–66. doi:10.1046/j.1365-2249.2003.02260.x
36. Small TN, Robinson WH, Miklos DB. B cells and transplantation: an educational resource. Biol Blood Marrow Transplant (2009) 15(1 Suppl):104–13. doi:10.1016/j.bbmt.2008.10.016
37. Bemark M, Holmqvist J, Abrahamsson J, Mellgren K. Translational mini-review series on B cell subsets in disease. Reconstitution after haematopoietic stem cell transplantation – revelation of B cell developmental pathways and lineage phenotypes. Clin Exp Immunol (2012) 167(1):15–25. doi:10.1111/j.1365-2249.2011.04469.x
38. Aucouturier P, Barra A, Intrator L, Cordonnier C, Schulz D, Duarte F, et al. Long lasting IgG subclass and antibacterial polysaccharide antibody deficiency after allogeneic bone marrow transplantation. Blood (1987) 70(3):779–85.
39. Nasman-Bjork I, Lundkvist I. Oligoclonal dominance of immunoglobulin VH3 rearrangements following allogeneic bone marrow transplantation. Bone Marrow Transplant (1998) 21(12):1223–30. doi:10.1038/sj.bmt.1701261
40. Roll P, Muhammad K, Stuhler G, Grigoleit U, Einsele H, Tony HP. Effect of ATG-F on B-cell reconstitution after hematopoietic stem cell transplantation. Eur J Haematol (2015) 95(6):514–23. doi:10.1111/ejh.12524
41. Fuji S, Kim SW, Yano S, Hagiwara S, Nakamae H, Hidaka M, et al. A prospective multicenter study of unrelated bone marrow transplants using a reduced-intensity conditioning regimen with low-dose ATG-F. Bone Marrow Transplant (2015) 51(3):451–3. doi:10.1038/bmt.2015.268
42. Zand MS. B-cell activity of polyclonal antithymocyte globulins. Transplantation (2006) 82(11):1387–95. doi:10.1097/01.tp.0000244063.05338.27
43. Petersen SL, Ryder LP, Bjork P, Madsen HO, Heilmann C, Jacobsen N, et al. A comparison of T-, B- and NK-cell reconstitution following conventional or nonmyeloablative conditioning and transplantation with bone marrow or peripheral blood stem cells from human leucocyte antigen identical sibling donors. Bone Marrow Transplant (2003) 32(1):65–72. doi:10.1038/sj.bmt.1704084
44. Storek J, Wells D, Dawson MA, Storer B, Maloney DG. Factors influencing B lymphopoiesis after allogeneic hematopoietic cell transplantation. Blood (2001) 98(2):489–91. doi:10.1182/blood.V98.2.489
45. Greinix HT, Pohlreich D, Kouba M, Kormoczi U, Lohmann I, Feldmann K, et al. Elevated numbers of immature/transitional CD21- B lymphocytes and deficiency of memory CD27+ B cells identify patients with active chronic graft-versus-host disease. Biol Blood Marrow Transplant (2008) 14(2):208–19. doi:10.1016/j.bbmt.2007.10.009
46. Greinix HT, Kuzmina Z, Weigl R, Kormoczi U, Rottal A, Wolff D, et al. CD19+CD21low B cells and CD4+CD45RA+CD31+ T cells correlate with first diagnosis of chronic graft-versus-host disease. Biol Blood Marrow Transplant (2015) 21(2):250–8. doi:10.1016/j.bbmt.2014.11.010
47. Storek J, Witherspoon RP, Maloney DG, Chauncey TR, Storb R. Improved reconstitution of CD4 T cells and B cells but worsened reconstitution of serum IgG levels after allogeneic transplantation of blood stem cells instead of marrow. Blood (1997) 89(10):3891–3.
48. Storek J, Dawson MA, Storer B, Stevens-Ayers T, Maloney DG, Marr KA, et al. Immune reconstitution after allogeneic marrow transplantation compared with blood stem cell transplantation. Blood (2001) 97(11):3380–9. doi:10.1182/blood.V97.11.3380
49. Fontenot JD, Rudensky AY. A well adapted regulatory contrivance: regulatory T cell development and the forkhead family transcription factor Foxp3. Nat Immunol (2005) 6(4):331–7. doi:10.1038/ni1179
50. Feuerer M, Hill JA, Mathis D, Benoist C. Foxp3+ regulatory T cells: differentiation, specification, subphenotypes. Nat Immunol (2009) 10(7):689–95. doi:10.1038/ni.1760
51. Sakaguchi S, Yamaguchi T, Nomura T, Ono M. Regulatory T cells and immune tolerance. Cell (2008) 133(5):775–87. doi:10.1016/j.cell.2008.05.009
52. Hori S, Nomura T, Sakaguchi S. Control of regulatory T cell development by the transcription factor Foxp3. Science (2003) 299(5609):1057–61. doi:10.1126/science.1079490
53. Cobbold SP, Castejon R, Adams E, Zelenika D, Graca L, Humm S, et al. Induction of foxP3+ regulatory T cells in the periphery of T cell receptor transgenic mice tolerized to transplants. J Immunol (2004) 172(10):6003–10. doi:10.4049/jimmunol.172.10.6003
54. Baron U, Floess S, Wieczorek G, Baumann K, Grützkau A, Dong J, et al. DNA demethylation in the human FOXP3 locus discriminates regulatory T cells from activated FOXP3+ conventional T cells. Eur J Immunol (2007) 37(9):2378–89. doi:10.1002/eji.200737594
55. Zheng Y, Josefowicz S, Chaudhry A, Peng XP, Forbush K, Rudensky AY. Role of conserved non-coding DNA elements in the Foxp3 gene in regulatory T-cell fate. Nature (2010) 463(7282):808–12. doi:10.1038/nature08750
56. Maloy KJ, Powrie F. Regulatory T cells in the control of immune pathology. Nat Immunol (2001) 2(9):816–22. doi:10.1038/ni0901-816
57. Vignali DA, Collison LW, Workman CJ. How regulatory T cells work. Nat Rev Immunol (2008) 8(7):523–32. doi:10.1038/nri2343
58. Campbell DJ, Koch MA. Phenotypical and functional specialization of FOXP3+ regulatory T cells. Nat Rev Immunol (2011) 11(2):119–30. doi:10.1038/nri2916
59. Kehrl JH, Wakefield LM, Roberts AB, Jakowlew S, Alvarez-Mon M, Derynck R, et al. Production of transforming growth factor beta by human T lymphocytes and its potential role in the regulation of T cell growth. J Exp Med (1986) 163(5):1037–50. doi:10.1084/jem.163.5.1037
60. Haak-Frendscho M, Wynn T, Czuprynski C, Paulnock D. Transforming growth factor-beta 1 inhibits activation of macrophage cell line RAW 264.7 for cell killing. Clin Exp Immunol (1990) 82(2):404. doi:10.1111/j.1365-2249.1990.tb05461.x
61. Belkaid Y, Rouse BT. Natural regulatory T cells in infectious disease. Nat Immunol (2005) 6(4):353–60. doi:10.1038/ni1181
62. Arpaia N, Campbell C, Fan X, Dikiy S, van der Veeken J, Liu H, et al. Metabolites produced by commensal bacteria promote peripheral regulatory T-cell generation. Nature (2013) 504(7480):451–5. doi:10.1038/nature12726
63. Mathewson ND, Jenq R, Mathew AV, Koenigsknecht M, Hanash A, Toubai T, et al. Gut microbiome-derived metabolites modulate intestinal epithelial cell damage and mitigate graft-versus-host disease. Nat Immunol (2016) 17(5):505–13. doi:10.1038/ni.3400
64. Shevach EM. CD4+ CD25+ suppressor T cells: more questions than answers. Nat Rev Immunol (2002) 2(6):389–400. doi:10.1038/nri821
65. Kingsley CI, Karim M, Bushell AR, Wood KJ. CD25+ CD4+ regulatory T cells prevent graft rejection: CTLA-4-and IL-10-dependent immunoregulation of alloresponses. J Immunol (2002) 168(3):1080–6. doi:10.4049/jimmunol.168.3.1080
66. Joffre O, Gorsse N, Romagnoli P, Hudrisier D, Van Meerwijk JP. Induction of antigen-specific tolerance to bone marrow allografts with CD4+ CD25+ T lymphocytes. Blood (2004) 103(11):4216–21. doi:10.1182/blood-2004-01-0005
67. Ferrara JL, Levine JE, Reddy P, Holler E. Graft-versus-host disease. Lancet (2009) 373(9674):1550–61. doi:10.1016/S0140-6736(09)60237-3
68. Cohen JL, Boyer O. The role of CD4+ CD25 hi regulatory T cells in the physiopathogeny of graft-versus-host disease. Curr Opin Immunol (2006) 18(5):580–5. doi:10.1016/j.coi.2006.07.007
69. Nguyen VH, Shashidhar S, Chang DS, Ho L, Kambham N, Bachmann M, et al. The impact of regulatory T cells on T-cell immunity following hematopoietic cell transplantation. Blood (2008) 111(2):945–53. doi:10.1182/blood-2007-07-103895
70. Taylor PA, Lees CJ, Blazar BR. The infusion of ex vivo activated and expanded CD4+ CD25+ immune regulatory cells inhibits graft-versus-host disease lethality. Blood (2002) 99(10):3493–9. doi:10.1182/blood.V99.10.3493
71. Fantini MC, Becker C, Monteleone G, Pallone F, Galle PR, Neurath MF. Cutting edge: TGF-β induces a regulatory phenotype in CD4+ CD25− T cells through Foxp3 induction and down-regulation of Smad7. J Immunol (2004) 172(9):5149–53. doi:10.4049/jimmunol.172.9.5149
72. Koenecke C, Czeloth N, Bubke A, Schmitz S, Kissenpfennig A, Malissen B, et al. Alloantigen-specific de novo-induced Foxp3+ Treg revert in vivo and do not protect from experimental GVHD. Eur J Immunol (2009) 39(11):3091–6. doi:10.1002/eji.200939432
73. Laurence A, Amarnath S, Mariotti J, Kim YC, Foley J, Eckhaus M, et al. STAT3 transcription factor promotes instability of nTreg cells and limits generation of iTreg cells during acute murine graft-versus-host disease. Immunity (2012) 37(2):209–22. doi:10.1016/j.immuni.2012.05.027
74. Ligocki AJ, Niederkorn JY. Advances on non-CD4 + Foxp3+ T regulatory cells: CD8+, type 1, and double negative t regulatory cells in organ transplantation. Transplantation (2015) 99(8):1553–9. doi:10.1097/TP.0000000000000813
75. Robb RJ, Lineburg KE, Kuns RD, Wilson YA, Raffelt NC, Olver SD, et al. Identification and expansion of highly suppressive CD8+ FoxP3+ regulatory T cells after experimental allogeneic bone marrow transplantation. Blood (2012) 119(24):5898–908. doi:10.1182/blood-2011-12-396119
76. Xystrakis E, Dejean AS, Bernard I, Druet P, Liblau R, Gonzalez-Dunia D, et al. Identification of a novel natural regulatory CD8 T-cell subset and analysis of its mechanism of regulation. Blood (2004) 104(10):3294–301. doi:10.1182/blood-2004-03-1214
77. Zheng J, Liu Y, Liu Y, Liu M, Xiang Z, Lam K-T, et al. Human CD8+ regulatory T cells inhibit GVHD and preserve general immunity in humanized mice. Sci Transl Med (2013) 5(168):ra169. doi:10.1126/scitranslmed.3004943
78. Li Q, Zhai Z, Xu X, Shen Y, Zhang A, Sun Z, et al. Decrease of CD4+ CD25+ regulatory T cells and TGF-β at early immune reconstitution is associated to the onset and severity of graft-versus-host disease following allogeneic haematogenesis stem cell transplantation. Leuk Res (2010) 34(9):1158–68. doi:10.1016/j.leukres.2010.03.017
79. Rieger K, Loddenkemper C, Maul J, Fietz T, Wolff D, Terpe H, et al. Mucosal FOXP3+ regulatory T cells are numerically deficient in acute and chronic GvHD. Blood (2006) 107(4):1717–23. doi:10.1182/blood-2005-06-2529
80. Lord JD, Hackman RC, Gooley TA, Wood BL, Moklebust AC, Hockenbery DM, et al. Blood and gastric FOXP3+ T cells are not decreased in human gastric graft-versus-host disease. Biol Blood Marrow Transplant (2011) 17(4):486–96. doi:10.1016/j.bbmt.2010.09.015
81. Ratajczak P, Janin A, De Latour RP, Leboeuf C, Desveaux A, Keyvanfar K, et al. Th17/Treg ratio in human graft-versus-host disease. Blood (2010) 116(7):1165–71. doi:10.1182/blood-2009-12-255810
82. Imanguli M, Cowen E, Rose J, Dhamala S, Swaim W, Lafond S, et al. Comparative analysis of FoxP3(+); regulatory T cells in the target tissues and blood in chronic graft versus host disease. Leukemia (2014) 28(10):2016–27. doi:10.1038/leu.2014.92
83. Trzonkowski P, Bieniaszewska M, Juścińska J, Dobyszuk A, Krzystyniak A, Marek N, et al. First-in-man clinical results of the treatment of patients with graft versus host disease with human ex vivo expanded CD4+ CD25+ CD127− T regulatory cells. Clin Immunol (2009) 133(1):22–6. doi:10.1016/j.clim.2009.06.001
84. Hoffmann P, Eder R, Kunz-Schughart LA, Andreesen R, Edinger M. Large-scale in vitro expansion of polyclonal human CD4+ CD25high regulatory T cells. Blood (2004) 104(3):895–903. doi:10.1182/blood-2004-01-0086
85. Strauss L, Whiteside TL, Knights A, Bergmann C, Knuth A, Zippelius A. Selective survival of naturally occurring human CD4+ CD25+ Foxp3+ regulatory T cells cultured with rapamycin. J Immunol (2007) 178(1):320–9. doi:10.4049/jimmunol.178.1.320
86. Hippen K, Merkel S, Schirm D, Nelson C, Tennis N, Riley J, et al. Generation and large-scale expansion of human inducible regulatory T cells that suppress graft-versus-host disease. Am J Transplant (2011) 11(6):1148–57. doi:10.1111/j.1600-6143.2011.03558.x
87. Matsuoka K-I, Koreth J, Kim HT, Bascug G, McDonough S, Kawano Y, et al. Low-dose interleukin-2 therapy restores regulatory T cell homeostasis in patients with chronic graft-versus-host disease. Sci Transl Med (2013) 5(179):ra143–79. doi:10.1126/scitranslmed.3005265
88. Koreth J, Matsuoka K, Kim HT, McDonough SM, Bindra B, Alyea EP III, et al. Interleukin-2 and regulatory T cells in graft-versus-host disease. N Engl J Med (2011) 365(22):2055–66. doi:10.1056/NEJMoa1108188
89. Furusawa Y, Obata Y, Fukuda S, Endo TA, Nakato G, Takahashi D, et al. Commensal microbe-derived butyrate induces the differentiation of colonic regulatory T cells. Nature (2013) 504(7480):446–50. doi:10.1038/nature12721
90. Mahnke YD, Brodie TM, Sallusto F, Roederer M, Lugli E. The who’s who of T-cell differentiation: human memory T-cell subsets. Eur J Immunol (2013) 43(11):2797–809. doi:10.1002/eji.201343751
91. von Andrian UH, Mempel TR. Homing and cellular traffic in lymph nodes. Nat Rev Immunol (2003) 3(11):867–78. doi:10.1038/nri1222
92. Smith CJ, Quinn M, Snyder CM. CMV-specific CD8 T cell differentiation and localization: implications for adoptive therapies. Front Immunol (2016) 7:352. doi:10.3389/fimmu.2016.00352
93. Clave E, Busson M, Douay C, Peffault de Latour R, Berrou J, Rabian C, et al. Acute graft-versus-host disease transiently impairs thymic output in young patients after allogeneic hematopoietic stem cell transplantation. Blood (2009) 113(25):6477–84. doi:10.1182/blood-2008-09-176594
94. Fujimaki K, Maruta A, Yoshida M, Kodama F, Matsuzaki M, Fujisawa S, et al. Immune reconstitution assessed during five years after allogeneic bone marrow transplantation. Bone Marrow Transplant (2001) 27(12):1275–81. doi:10.1038/sj.bmt.1703056
95. Le RQ, Melenhorst JJ, Battiwalla M, Hill B, Memon S, Savani BN, et al. Evolution of the donor T-cell repertoire in recipients in the second decade after allogeneic stem cell transplantation. Blood (2011) 117(19):5250–6. doi:10.1182/blood-2011-01-329706
96. Heining C, Spyridonidis A, Bernhardt E, Schulte-Monting J, Behringer D, Grullich C, et al. Lymphocyte reconstitution following allogeneic hematopoietic stem cell transplantation: a retrospective study including 148 patients. Bone Marrow Transplant (2007) 39(10):613–22. doi:10.1038/sj.bmt.1705648
97. Jacobsohn DA, Vogelsang GB. Acute graft versus host disease. Orphanet J Rare Dis (2007) 2:35. doi:10.1186/1750-1172-2-35
98. Cantoni N, Hirsch HH, Khanna N, Gerull S, Buser A, Bucher C, et al. Evidence for a bidirectional relationship between cytomegalovirus replication and acute graft-versus-host disease. Biol Blood Marrow Transplant (2010) 16(9):1309–14. doi:10.1016/j.bbmt.2010.03.020
99. Auletta JJ, Lazarus HM. Immune restoration following hematopoietic stem cell transplantation: an evolving target. Bone Marrow Transplant (2005) 35(9):835–57. doi:10.1038/sj.bmt.1704966
100. Blazar BR, Murphy WJ, Abedi M. Advances in graft-versus-host disease biology and therapy. Nat Rev Immunol (2012) 12(6):443–58. doi:10.1038/nri3212
101. Ferrara JL, Cooke KR, Teshima T. The pathophysiology of acute graft-versus-host disease. Int J Hematol (2003) 78(3):181–7. doi:10.1007/BF02983793
102. Szyska M, Na I-K. Bone marrow GvHD after allogeneic hematopoietic stem cell transplantation. Front Immunol (2016) 7:118. doi:10.3389/fimmu.2016.00118
103. Shono Y, Ueha S, Wang Y, Abe J, Kurachi M, Matsuno Y, et al. Bone marrow graft-versus-host disease: early destruction of hematopoietic niche after MHC-mismatched hematopoietic stem cell transplantation. Blood (2010) 115(26):5401–11. doi:10.1182/blood-2009-11-253559
104. Müller AMS, Linderman JA, Florek M, Miklos D, Shizuru JA. Allogeneic T cells impair engraftment and hematopoiesis after stem cell transplantation. Proc Natl Acad Sci U S A (2010) 107(33):14721–6. doi:10.1073/pnas.1009220107
105. McCarthy AL, Malik Peiris JS, Taylor CE, Green MA, Sviland L, Pearson AD, et al. Increase in severity of graft versus host disease by cytomegalovirus. J Clin Pathol (1992) 45(6):542–4. doi:10.1136/jcp.45.6.542
106. Hakki M, Riddell SR, Storek J, Carter RA, Stevens-Ayers T, Sudour P, et al. Immune reconstitution to cytomegalovirus after allogeneic hematopoietic stem cell transplantation: impact of host factors, drug therapy, and subclinical reactivation. Blood (2003) 102(8):3060–7. doi:10.1182/blood-2002-11-3472
107. Özdemir E, St. John LS, Gillespie G, Rowland-Jones S, Champlin RE, Molldrem JJ, et al. Cytomegalovirus reactivation following allogeneic stem cell transplantation is associated with the presence of dysfunctional antigen-specific CD8+ T cells. Blood (2002) 100(10):3690–7. doi:10.1182/blood-2002-05-1387
108. Marek A, Stern M, Chalandon Y, Ansari M, Ozsahin H, Gungor T, et al. The impact of T-cell depletion techniques on the outcome after haploidentical hematopoietic SCT. Bone Marrow Transplant (2014) 49(1):55–61. doi:10.1038/bmt.2013.132
109. Ciceri F, Bonini C, Stanghellini MT, Bondanza A, Traversari C, Salomoni M, et al. Infusion of suicide-gene-engineered donor lymphocytes after family haploidentical haemopoietic stem-cell transplantation for leukaemia (the TK007 trial): a non-randomised phase I-II study. Lancet Oncol (2009) 10(5):489–500. doi:10.1016/S1470-2045(09)70074-9
110. Weissinger EM, Borchers S, Silvani A, Provasi E, Radrizzani M, Beckmann IK, et al. Long term follow up of patients after allogeneic stem cell transplantation and transfusion of HSV-TK transduced T-cells. Front Pharmacol (2015) 6:76. doi:10.3389/fphar.2015.00076
111. Na I-K, Wittenbecher F, Dziubianau M, Herholz A, Mensen A, Kunkel D, et al. Rabbit antithymocyte globulin (Thymoglobulin(®)) impairs the thymic output of both conventional and regulatory CD4(+) T cells after allogeneic hematopoietic stem cell transplantation in adult patients. Haematologica (2013) 98(1):23–30. doi:10.3324/haematol.2012.067611
112. Juliusson G, Theorin N, Karlsson K, Frodin U, Malm C. Subcutaneous alemtuzumab vs ATG in adjusted conditioning for allogeneic transplantation: influence of Campath dose on lymphoid recovery, mixed chimerism and survival. Bone Marrow Transplant (2006) 37(5):503–10. doi:10.1038/sj.bmt.1705263
113. Cwynarski K, Ainsworth J, Cobbold M, Wagner S, Mahendra P, Apperley J, et al. Direct visualization of cytomegalovirus-specific T-cell reconstitution after allogeneic stem cell transplantation. Blood (2001) 97(5):1232–40. doi:10.1182/blood.V97.5.1232
114. Jansen J, Hanks S, Thompson JM, Dugan MJ, Akard LP. Transplantation of hematopoietic stem cells from the peripheral blood. J Cell Mol Med (2005) 9(1):37–50. doi:10.1111/j.1582-4934.2005.tb00335.x
115. Barrett AJ. Mechanisms of the graft-versus-leukemia reaction. Stem Cells (1997) 15(4):248–58. doi:10.1002/stem.150248
116. Chakraverty R, Eom H-S, Sachs J, Buchli J, Cotter P, Hsu R, et al. Host MHC class II+ antigen-presenting cells and CD4 cells are required for CD8-mediated graft-versus-leukemia responses following delayed donor leukocyte infusions. Blood (2006) 108(6):2106–13. doi:10.1182/blood-2006-03-007427
117. Nikiforow S, Alyea EP. Maximizing GVL in allogeneic transplantation: role of donor lymphocyte infusions. Hematology Am Soc Hematol Educ Program (2014) 2014(1):570–5. doi:10.1182/asheducation-2014.1.570
118. Kolb H, Schattenberg A, Goldman J, Hertenstein B, Jacobsen N, Arcese W, et al. Graft-versus-leukemia effect of donor lymphocyte transfusions in marrow grafted patients. European group for blood and marrow transplantation working party chronic leukemia. Blood (1995) 86(5):2041–50.
119. Kolb H-J, Schmid C, Barrett AJ, Schendel DJ. Graft-versus-leukemia reactions in allogeneic chimeras. Blood (2004) 103(3):767–76. doi:10.1182/blood-2003-02-0342
120. Meyer RG, Britten CM, Wehler D, Bender K, Hess G, Konur A, et al. Prophylactic transfer of CD8-depleted donor lymphocytes after T-cell-depleted reduced-intensity transplantation. Blood (2007) 109(1):374–82. doi:10.1182/blood-2006-03-005769
121. Meyer RG, Wagner EM, Konur A, Bender K, Schmitt T, Hemmerling J, et al. Donor CD4 T cells convert mixed to full donor T-cell chimerism and replenish the CD52-positive T-cell pool after alemtuzumab-based T-cell-depleted allo-transplantation. Bone Marrow Transplant (2010) 45(4):668–74. doi:10.1038/bmt.2009.212
122. Zhou W, Longmate J, Lacey SF, Palmer JM, Gallez-Hawkins G, Thao L, et al. Impact of donor CMV status on viral infection and reconstitution of multifunction CMV-specific T cells in CMV-positive transplant recipients. Blood (2009) 113(25):6465–76. doi:10.1182/blood-2009-02-203307
123. Ljungman P, Hakki M, Boeckh M. Cytomegalovirus in hematopoietic stem cell transplant recipients. Hematol Oncol Clin North Am (2011) 25(1):151–69. doi:10.1016/j.hoc.2010.11.011
124. Boeckh M, Ljungman P. How we treat cytomegalovirus in hematopoietic cell transplant recipients. Blood (2009) 113(23):5711–9. doi:10.1182/blood-2008-10-143560
125. Mori T, Mori S, Kanda Y, Yakushiji K, Mineishi S, Takaue Y, et al. Clinical significance of cytomegalovirus (CMV) antigenemia in the prediction and diagnosis of CMV gastrointestinal disease after allogeneic hematopoietic stem cell transplantation. Bone Marrow Transplant (2003) 33(4):431–4. doi:10.1038/sj.bmt.1704369
126. Eid AJ, Bakri SJ, Kijpittayarit S, Razonable RR. Clinical features and outcomes of cytomegalovirus retinitis after transplantation. Transpl Infect Dis (2008) 10(1):13–8. doi:10.1111/j.1399-3062.2007.00241.x
127. Travi G, Pergam SA. Cytomegalovirus pneumonia in hematopoietic stem cell recipients. J Intensive Care Med (2014) 29(4):200–12. doi:10.1177/0885066613476454
128. Ljungman P, Perez-Bercoff L, Jonsson J, Avetisyan G, Sparrelid E, Aschan J, et al. Risk factors for the development of cytomegalovirus disease after allogeneic stem cell transplantation. Haematologica (2006) 91(1):78–83.
129. Pourgheysari B, Piper KP, McLarnon A, Arrazi J, Bruton R, Clark F, et al. Early reconstitution of effector memory CD4+ CMV-specific T cells protects against CMV reactivation following allogeneic SCT. Bone Marrow Transplant (2008) 43(11):853–61. doi:10.1038/bmt.2008.403
130. Gärtner BC, Schäfer H, Marggraff K, Eisele G, Schäfer M, Roemer K, et al. Evaluation of use of Epstein-Barr viral load in patients after allogeneic stem cell transplantation to diagnose and monitor posttransplant lymphoproliferative disease. J Clin Microbiol (2002) 40(2):351–8. doi:10.1128/JCM.40.2.351-358.2002
131. Peric Z, Cahu X, Chevallier P, Brissot E, Malard F, Guillaume T, et al. Features of Epstein-Barr virus (EBV) reactivation after reduced intensity conditioning allogeneic hematopoietic stem cell transplantation. Leukemia (2011) 25(6):932–8. doi:10.1038/leu.2011.26
132. Uhlin M, Wikell H, Sundin M, Blennow O, Maeurer M, Ringden O, et al. Risk factors for Epstein-Barr virus-related post-transplant lymphoproliferative disease after allogeneic hematopoietic stem cell transplantation. Haematologica (2014) 99(2):346–52. doi:10.3324/haematol.2013.087338
133. Maschmeyer G, Ljungman P. Infections in hematopoietic stem cell transplant recipients. In: Safdar A, editor. Principles and Practice of Cancer Infectious Diseases. Springer (2011). p. 17–25.
134. Borchers S, Luther S, Lips U, Hahn N, Kontsendorn J, Stadler M, et al. Tetramer monitoring to assess risk factors for recurrent cytomegalovirus reactivation and reconstitution of antiviral immunity post allogeneic hematopoietic stem cell transplantation. Transpl Infect Dis (2011) 13(3):222–36. doi:10.1111/j.1399-3062.2011.00626.x
135. Chalandon Y, Degermann S, Villard J, Arlettaz L, Kaiser L, Vischer S, et al. Pretransplantation CMV-specific T cells protect recipients of T-cell-depleted grafts against CMV-related complications. Blood (2006) 107(1):389–96. doi:10.1182/blood-2005-07-2746
136. Borchers S, Bremm M, Lehrnbecher T, Dammann E, Pabst B, Wölk B, et al. Sequential anti-cytomegalovirus response monitoring may allow prediction of cytomegalovirus reactivation after allogeneic stem cell transplantation. PLoS One (2012) 7(12):e50248. doi:10.1371/journal.pone.0050248
137. Borchers S, Ogonek J, Varanasi PR, Tischer S, Bremm M, Eiz-Vesper B, et al. Multimer monitoring of CMV-specific T cells in research and in clinical applications. Diagn Microbiol Infect Dis (2014) 78(3):201–12. doi:10.1016/j.diagmicrobio.2013.11.007
138. Odendahl M, Grigoleit GU, Bönig H, Neuenhahn M, Albrecht J, Anderl F, et al. Clinical-scale isolation of ‘minimally manipulated’ cytomegalovirus-specific donor lymphocytes for the treatment of refractory cytomegalovirus disease. Cytotherapy (2014) 16(9):1245–56. doi:10.1016/j.jcyt.2014.05.023
139. Kumaresan P, Figliola M, Moyes JS, Huls MH, Tewari P, Shpall EJ, et al. Automated cell enrichment of cytomegalovirus-specific T cells for clinical applications using the cytokine-capture system. J Vis Exp (2015) (104):e52808. doi:10.3791/52808
140. Lacey SF, Diamond DJ, Zaia JA. Assessment of cellular immunity to human cytomegalovirus in recipients of allogeneic stem cell transplants. Biol Blood Marrow Transplant (2004) 10(7):433–47. doi:10.1016/j.bbmt.2003.12.004
141. Tuthill M, Chen F, Paston S, De La Peña H, Rusakiewicz S, Madrigal A. The prevention and treatment of cytomegalovirus infection in haematopoietic stem cell transplantation. Cancer Immunol Immunother (2009) 58(9):1481–8. doi:10.1007/s00262-009-0722-7
142. Gratama JW, van Esser JWJ, Lamers CHJ, Tournay C, Löwenberg B, Bolhuis RLH, et al. Tetramer-based quantification of cytomegalovirus (CMV)-specific CD8+ T lymphocytes in T-cell-depleted stem cell grafts and after transplantation may identify patients at risk for progressive CMV infection. Blood (2001) 98(5):1358–64. doi:10.1182/blood.V98.5.1358
143. Lilleri D, Fornara C, Chiesa A, Caldera D, Alessandrino EP, Gerna G. Human cytomegalovirus-specific CD4+ and CD8+ T-cell reconstitution in adult allogeneic hematopoietic stem cell transplant recipients and immune control of viral infection. Haematologica (2008) 93(2):248–56. doi:10.3324/haematol.11912
144. Imai C, Iwamoto S, Campana D. Genetic modification of primary natural killer cells overcomes inhibitory signals and induces specific killing of leukemic cells. Blood (2005) 106(1):376–83. doi:10.1182/blood-2004-12-4797
145. Foster AE, Gottlieb DJ, Sartor M, Hertzberg MS, Bradstock KF. Cytomegalovirus-specific CD4+ and CD8+ T-cells follow a similar reconstitution pattern after allogeneic stem cell transplantation. Biol Blood Marrow Transplant (2002) 8(9):501–11. doi:10.1053/bbmt.2002.v8.pm12374455
146. Altman JD, Moss PA, Goulder PJ, Barouch DH, McHeyzer-Williams MG, Bell JI, et al. Phenotypic analysis of antigen-specific T lymphocytes. Science (1996) 274(5284):94–6. doi:10.1126/science.274.5284.94
147. Aubert G, Hassan-Walker AF, Madrigal JA, Emery VC, Morte C, Grace S, et al. Cytomegalovirus-specific cellular immune responses and viremia in recipients of allogeneic stem cell transplants. J Infect Dis (2001) 184(8):955–63. doi:10.1086/323354
148. Chen FE, Aubert G, Travers P, Dodi IA, Madrigal JA. HLA tetramers and anti-CMV immune responses: from epitope to immunotherapy. Cytotherapy (2002) 4(1):41–8. doi:10.1080/146532402317251518
149. Lacey SF, Villacres MC, La Rosa C, Wang Z, Longmate J, Martinez J, et al. Relative dominance of HLA-B*07 restricted CD8+ T-lymphocyte immune responses to human cytomegalovirus pp65 in persons sharing HLA-A*02 and HLA-B*07 alleles. Hum Immunol (2003) 64(4):440–52. doi:10.1016/S0198-8859(03)00028-4
150. Akiyama Y, Kuzushima K, Tsurumi T, Yamaguchi K. Analysis of HLA-A24-restricted CMVpp65 peptide-specific CTL with HLA-A*2402-CMVpp65 tetramer. Immunol Lett (2004) 95(2):199–205. doi:10.1016/j.imlet.2004.07.010
151. Bunde T, Kirchner A, Hoffmeister B, Habedank D, Hetzer R, Cherepnev G, et al. Protection from cytomegalovirus after transplantation is correlated with immediate early 1-specific CD8 T cells. J Exp Med (2005) 201(7):1031–6. doi:10.1084/jem.20042384
152. Lilleri D, Gerna G, Fornara C, Lozza L, Maccario R, Locatelli F. Prospective simultaneous quantification of human cytomegalovirus-specific CD4+ and CD8+ T-cell reconstitution in young recipients of allogeneic hematopoietic stem cell transplants. Blood (2006) 108(4):1406–12. doi:10.1182/blood-2005-11-012864
153. Gratama JW, Brooimans RA, van der Holt B, Sintnicolaas K, van Doornum G, Niesters HG, et al. Monitoring cytomegalovirus IE-1 and pp65-specific CD4+ and CD8+ T-cell responses after allogeneic stem cell transplantation may identify patients at risk for recurrent CMV reactivations. Cytometry B Clin Cytom (2008) 74(4):211–20. doi:10.1002/cyto.b.20420
154. Koehl U, Dirkwinkel E, Koenig M, Erben S, Soerensen J, Bader P, et al. Reconstitution of cytomegalovirus specific T cells after pediatric allogeneic stem cell transplantation: results from a pilot study using a multi-allele CMV tetramer group. Klin Padiatr (2008) 220(6):348–52. doi:10.1055/s-0028-1086029
155. Giest S, Grace S, Senegaglia AC, Pasquini R, Gonzalo-Daganzo RM, Fernandez MN, et al. Cytomegalovirus-specific CD8(+) T cells targeting different HLA/peptide combinations correlate with protection but at different threshold frequencies. Br J Haematol (2010) 148(2):311–22. doi:10.1111/j.1365-2141.2009.07969.x
156. Gratama JW, Boeckh M, Nakamura R, Cornelissen JJ, Brooimans RA, Zaia JA, et al. Immune monitoring with iTAg MHC Tetramers for prediction of recurrent or persistent cytomegalovirus infection or disease in allogeneic hematopoietic stem cell transplant recipients: a prospective multicenter study. Blood (2010) 116(10):1655–62. doi:10.1182/blood-2010-03-273508
Keywords: hematopoietic stem cell transplantation, immune reconstitution, infection, graft-versus-leukemia effect, graft-versus-host disease
Citation: Ogonek J, Kralj Juric M, Ghimire S, Varanasi PR, Holler E, Greinix H and Weissinger E (2016) Immune Reconstitution after Allogeneic Hematopoietic Stem Cell Transplantation. Front. Immunol. 7:507. doi: 10.3389/fimmu.2016.00507
Received: 05 August 2016; Accepted: 02 November 2016;
Published: 17 November 2016
Edited by:
Anne Mary Dickinson, Newcastle University, UKReviewed by:
Ignacio Anegon, University of Nantes, FranceCopyright: © 2016 Ogonek, Kralj Juric, Ghimire, Varanasi, Holler, Greinix and Weissinger. This is an open-access article distributed under the terms of the Creative Commons Attribution License (CC BY). The use, distribution or reproduction in other forums is permitted, provided the original author(s) or licensor are credited and that the original publication in this journal is cited, in accordance with accepted academic practice. No use, distribution or reproduction is permitted which does not comply with these terms.
*Correspondence: Eva Weissinger, bWlzY2hhay13ZWlzc2luZ2VyLmV2YUBtaC1oYW5ub3Zlci5kZQ==
†Justyna Ogonek, Mateja Kralj Juric, and Sakhila Ghimire equally contributed to this manuscript.
‡Hildegard Greinix and Eva Weissinger equally contributed as senior authorship.
Disclaimer: All claims expressed in this article are solely those of the authors and do not necessarily represent those of their affiliated organizations, or those of the publisher, the editors and the reviewers. Any product that may be evaluated in this article or claim that may be made by its manufacturer is not guaranteed or endorsed by the publisher.
Research integrity at Frontiers
Learn more about the work of our research integrity team to safeguard the quality of each article we publish.