- Department of Dermatology, University Medical Center, Johannes Gutenberg-University, Mainz, Germany
Regulatory T cells (Treg) control immune cell function as well as non-immunological processes. Their far-reaching regulatory activities suggest their functional manipulation as a means to sustainably and causally intervene with the course of diseases. Preclinical tools and strategies are however needed to further test and develop interventional strategies outside the human body. “Humanized” mouse models consisting of mice engrafted with human immune cells and tissues provide new tools to analyze human Treg ontogeny, immunobiology, and therapy. Here, we summarize the current state of humanized mouse models as a means to study human Treg function at the molecular level and to design strategies to harness these cells for therapeutic purposes.
The Emergency of Treg as Therapeutic Target
The notion that T cells can be actively involved in immunological tolerance goes back to the seventies of the last century when it was shown that T cells (thymus-derived lymphocytes) are required for tolerance induction and could transfer tolerance to naïve recipients (1, 2). Tolerance induction by T cells has thereafter been convincingly demonstrated by numerous investigators using a variety of experimental systems (3, 4). Despite decades of research, it was not possible to unequivocally identify the responsible T cell population. In the 90s, Simon Sakaguchi and colleagues were able to prove that a small population of constitutively CD25-expressing CD4+ T cells prevents autoimmune disease in mice (5). The discovery of the X chromosome encoded transcription factor Foxp3 (forkhead box protein 3) (6–9) as a specification and maintenance factor (10, 11) subsequently confirmed CD4+CD25+ T cells as a unique thymus-derived lineage. A population of phenotypically and functionally similar T cells could also be corroborated in humans (12, 13). Examining their role in the murine immune system revealed that regulatory T cells (Treg) – as they had been called in foresight – take a central role in immune homeostasis: genetic defects that turn Treg dysfunctional result in multiorgan autoimmune disease (14) and their depletion induces autoimmunity (15). The investigation of their suppressive activity was greatly helped by major improvements in molecular profiling techniques and revealed a number of potential suppressive mechanisms (16). More recently, several Treg effector molecules were found to play a role in Treg peripheral expansion and activation rather than suppressive activity (17) or contribute to Treg function only under particular conditions (18). Moreover, only few mechanisms have been confirmed in mice and man (19–21).
While Treg were initially investigated mainly in regard to their effects on T cells, their far-reaching regulatory activity affects the activation, differentiation, and survival of all types of immune cells. Although their main role appears to be the prevention of autoimmune reactions their broad immune-regulatory potential and bystander activation in immune responses affects a wide range of conditions such as infection, cancer, transplantation, allergy, and inflammatory diseases, but also responses toward foreign antigens, as well as fetal antigens during pregnancy (22–28).
Murine and human Treg, like all T cells, consist of numerous differentiated subpopulations (29–38). While in some cases, the functional properties of subpopulations have been described in detail, a systematic assignment of phenotypes and functions is missing in both species. Strikingly, beyond their role in immune homeostasis and immune cell regulation, specialized Treg subpopulations also interfere with non-immunological processes such as metabolism (39) and wound healing (36, 40, 41). So far, the latter populations have so far exclusively been described in mice.
To date, the vast majority of data concerning Treg were obtained from mouse experiments. The reason for this is the fact that human tissue cannot always be investigated. However, human Treg studies are also technically limited by the lack of a reliable and specific Treg marker molecule (21, 42). Whereas in healthy subjects, the main human Treg population in blood can at least be approximated as CD45RO+TCR+CD4+CD25highFoxp3+CD127neg cells (43), their analysis and isolation remains affected by similarly phenotyped non-regulatory T helper cells under conditions of T cell activation such as in inflammatory disease (44, 45). Today, the most effective, reliable, and objective criteria for the identification and quantification of Treg in diagnostic or therapeutic settings is in quantitative real-time PCR-based methylation analysis of an evolutionarily conserved element within the Foxp3 locus (46, 47). This method however only indirectly contributes to Treg isolation from human samples due to associated cell destruction.
Their potent and far-reaching regulatory activities have helped Treg to become a major subject in virtually all fields of medical research. With regard to the lack of a comprehensive understanding of human Treg composition and function, a cautionary approach to Treg modulation in patients appears to be warranted, even more so, as animal models failed to predict effects of Treg modulating agents in humans (48). The latter, in particular, has made clear that human Treg function as well as Treg targeted immune intervention need to be examined more closely. As these studies cannot be performed in man, a model organism is required in which the function of human cells can be examined outside the human body. In recent years, humanized mouse models have emerged as a suitable tool to study human Treg function and to develop strategies for their therapeutic application.
Treg in Autoimmune Disease and Cancer
The main physiological role of Treg appears to be the prevention of autoimmune responses (49) as evidenced by fulminate autoimmune reactions in their absence (50) (Table 1). In turn, their autoprotective activity suggests that autoimmune diseases may result from their functional impairment. While their peripheral number remains unchanged in the majority of autoimmune diseases (51–53), their suppressive function seems to be indeed altered (51, 52, 54–56). However, retrospective studies in patients are confused with the problem that Treg dysfunctionality can be either intrinsic or reflect changes in other immune components (57–61). The autoimmune phenotype of scurfy mice, for example, results from both Treg dysfunction and an increased population of self-reactive T cells emerging from progenitor cells which did not properly develop into Treg. Thus, to reveal the functional state of Treg and to understand their role and possible effects in disease, causes and effects need to be carefully discerned. As shown by Trinschek et al. (62), humanized mouse models can help to elucidate disease mechanisms by enabling to individually manipulate and combine diseased and unaffected Treg from patients and healthy donors. It needs to be mentioned that in addition to the thymus-derived Treg population non-regulatory CD4+ T cells can acquire a similar regulatory function and phenotype outside of the thymus, typically under the influence of particular forms of inflammation (63). Here, we focus on thymus-derived Treg since they are the most widely studied in humanized mice.
Regardless of numerous genetic changes, tumors are primarily the body’s own tissue (77). Their destruction by the immune system therefore represents – at least in part – an autoimmune response. Since Treg appear to be in part self-reactive (78), it is not surprising that increased Treg activity is causally involved in tumor-induced tolerance (52, 79). Numerous studies reported elevated numbers of Treg in the tumor tissue, lymphoid tissues, and peripheral blood of cancer patients (43, 52, 79–81), also the infiltrating of Treg into the tumor site correlates with poor prognoses in many tumor types (82). Some mechanisms of tumor-associated Treg recruitment and activation have been proposed (83–85), but may vary between tumor types and/or stages. Whereas Treg depletion increases all inflammatory responses including antitumor immunity (86), it is effective only in early stages of tumor growth (87, 88). Despite its proven genuine potential as a strategy to overcome tumor tolerance, Treg depletion is not yet a therapeutic option in cancer patients. While animal experiments strongly indicate that only a complete and selective Treg destruction may affect antitumor immunity, Treg depletion in cancer patients is hampered by inefficient non-specific depleting agents, which cause unwanted side effects (89). On the other hand, current treatment options such as immune checkpoint blockade seem to affect the Treg compartment (90). Thus, further investigation on the interaction of Treg and tumor cells in humans are needed and reagents for effective and selective Treg destruction need to be identified.
Humanized Mouse Models: Challenges and Limitations to Study Human Treg Biology
The simplest way to equip mice with human immune cells (mainly PBMC) is their intravenous or intraperitoneal injection (Figure 1). In order to accept the human graft, the recipients need to be immunodeficient and the degree of deficiency strongly influences the survival and function of transplanted human cells. Initial experiments by McCune and Mosier in severe combined immunodeficient (SCID) mice lacking T and B cells revealed that human immune cells can survive in mice for several weeks (91, 92). However, due to the remaining innate immunity and leakiness in adaptive cell development in aged SCID mice, transplanted human cells are only transiently accepted. Reduced natural killer cell (NK cell) activity in non-obese diabetic (NOD)-SCID mice improve engraftment. Profound and lasting impairment of adaptive and innate immunity by targeted mutation of the IL-2R gamma-chain gene in NOD-SCID IL-2Rγ−/− (NSG) or BALB/c recombinase activating gene (Rag)2−/− IL-2Rγ−/− mice eventually enable a stable long-term survival of transplanted human cells and tissues (93–99).
Upon engraftment of human PBMC into immunodeficient mice, a population of human T cells reacts against murine MHC molecules, supposedly on murine antigen-presenting cells, resulting in T cell-driven lethal xenogeneic graft-versus-host disease (GvHD) (92, 94, 100). In contrast to T cells, other transferred immune compartments such as myeloid cells, dendritic cells, or B cells rapidly vanish or at least become irretrievable. In adult NOD-SCID, sublethal irradiation or depletion of innate murine immune cells (called “conditioning”) accelerates GvHD onset. In contrast, GvHD can be reliably induced in newborn NOD-SCID and gamma-chain-deficient recipients without conditioning.
Graft-versus-host disease upon transfer of human PBMC is characterized by weight loss (or lack of weight gain in pubs), reduced mobility and a total mortality >95% (20). At the tissue level, it is accompanied by a massive infiltration of human T cells into all mouse organs leading to peribronchial and perivascular inflammation and increased mucus production, colitis, skin rashes, and increased glutamate pyruvate transaminase (GPT) serum levels indicative of hepatitis. When performed in newborn NOD-SCID mice, GvHD leads to death within 30–90 days, depending on the number of transferred PBMCs. Interestingly, the number of transferred T cells and their functional state influences disease onset: both increased cell numbers and cells from autoimmune patients accelerate disease onset (62). While increased cell numbers contain more xenoreactive cells, the reason for the latter effect is not completely clear. Supposedly, disease-mediated alterations in the T cell compartment, cross-reactivity or increased inflammatory cytokine production may act beneficial.
Owing to its simplicity, the xenogeneic GvHD provides a robust means to functionally evaluate Treg outside the human body (Figure 2): Without further treatment, the limited number of Treg transferred within the PBMC do not affect GvHD onset. However, transfer of additional Treg in ratios between 4:1 and 10:1 (PBMC:Tregs) suppresses all GvHD symptoms in a dose-dependent manner (20, 101). Since the suppressive activity of Treg is not antigen specific, either syngenic or allogeneic Treg may be used. Treg ratios lower than 10:1 appear ineffective, however, allowing to test biologicals with modest Treg-activating potential (102).
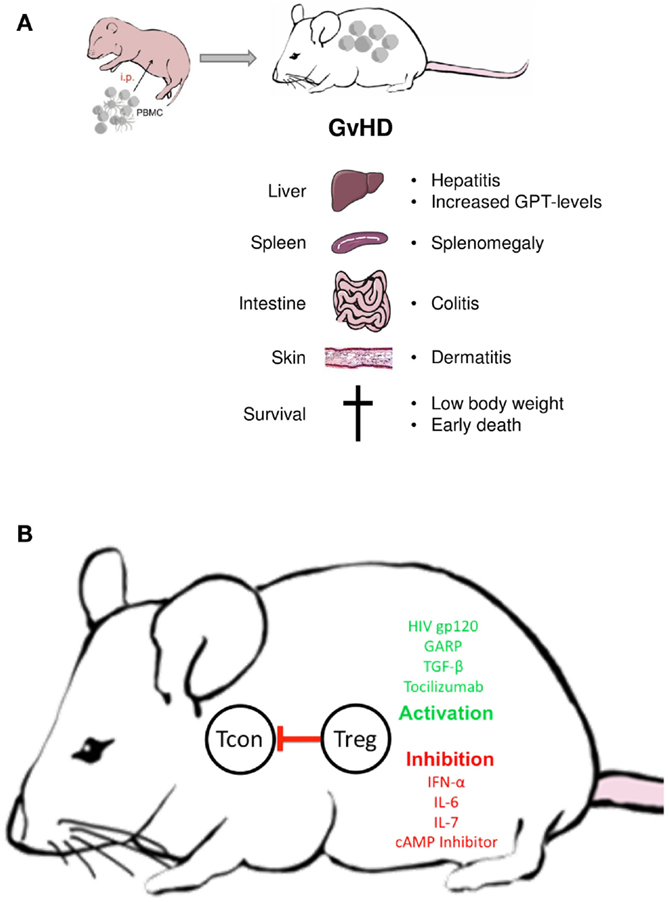
Figure 2. Treg manipulation in the xenogeneic GvHD model. (A) Disease symptoms; (B) examples of biologicals with demonstrated Treg activating or inactivating activity in the GvHD model; Tcon = conventional non-regulatory T cell.
Differences exist between mouse strains in regard to the persistence of Treg-mediated GvHD suppression. While in NOD-SCID mice, Treg-induced tolerance remains stable for weeks (20), it is only transient in NOD-SCIDγc−/− or Rag2γc−/− mice (102, 103). Thus, while all three strains can be used to investigate human Treg activation in vivo, a long-term evaluation of the functional state is only possible in the later model. Not only Treg numbers but also certain biologicals have been shown to selectively increase or repress Treg function in the transfer model (20, 104–106). Particularly, strong stimuli can prevent the xenogeneic response without increasing the number of Treg in PBMC (20). Alternatively, Treg can be separately pretreated with modulating agents or pathway inhibitors before transfer (107, 108). The latter approach is especially helpful in testing compounds which lack Treg specificity. Similar to in vitro assays, the GvHD model can be used to discern patient-associated functional alterations in Treg or T effector cells by combining either cells from patients or healthy donors (62).
Without Treg activation, the xenogeneic GvHD cannot be prevented. It is however delayed by the transfer of very low cell numbers and greatly reduced in MHC class I or class II knockout NOD-SCIDγc−/− or Rag2γc−/− mice (100, 109, 110). In this manner and by the use of PBMC from patients’ inflammatory responses such as allergy-induced inflammation and its regulation by Treg can be studied (102, 111–114). In the same manner, the transfer model has been used to analyze the mechanism of wound healing, tissue remodeling, and allograft rejection after tissue transplantation. Several investigators reported that the transfer of Treg prevented the rejection of human skin, islets, bronchus, and arterial grafts (96, 115–119). In addition to the general effect of Treg on the disease, these models can be used to compare differently isolated or expanded Treg populations (28, 120). So far, almost only total CD4+CD25+ populations have been used, followed by approval of disease repression, but without looking at their further development and survival.
Since current animal models may not accurately identify cancer immunotherapies with clinical potential, reliable preclinical tools are needed to test these drugs directly against human cancers. Here again, in its simplest form, a humanized mouse tumor model can be set up by cotransplantation of human immune cells and tumors. The first difficulty in setting up such a model consist in the constitutive dependency of primary human tumor cells on host growth factors, which greatly limits their ability to grow in a different species. Alternatively, long-term established tumor cell lines engraft well and are readily available. Depending on their origin and methodology of cultivation human tumor cell lines can phenocopy primary tumors (121, 122). Their growth in immunodeficient mice therefore provides a robust cell line model system that potentially predicts patient responses to drugs or biologicals (123, 124).
Adding human immune cells either by transplantation of immune cells or stem cells, does however, complicates the setting. Cotransplantation of human immune cells and allogeneic tumor cell lines creates the need to distinguish between xenogeneic, allogeneic, and potential tumor-specific responses. Although tumor-specific responses may be selectively followed by MHC complex multimers, they will be cross-influenced by strong inflammatory xenogeneic and/or allogeneic background activities. Therefore, a more promising approach consists in a syngeneic approach. In a syngeneic setting, CD8+ CTL have been shown to effectively combat patient tumor cells in immunodeficient mice (125, 126), and such models may help to identify tumor rejection antigens and T cell receptors for redirecting immunity to tumors in a patient-individualized manner. Although more complicated to set up, they may also provide a basis to study the interaction between patient-derived Treg and tumor cells and to develop reagents for effective and selective Treg impairment.
Another way to humanize mice is to transfer hematopoietic stem cells (HSC) either alone or in combination with human tissues required in lymphocyte development (Figure 1). Upon transfer of HSC into newborn or adult mice, multiple lineages of human hematopoietic cells (including T and B cells, plasmacytoid cells, myeloid cells, and NK cells) develop, resulting in a mixed murine/human CD45+ cells compartment. Even greater levels of human cell engraftment are achieved when human fetal tissue is cotransplanted. In the BLT (bone marrow/liver/thymus) model, human fetal liver and autologous thymus fragments are implanted under the renal capsule and HSC cells isolated from the same fetal liver are separately transferred to engraft the bone marrow.
Whereas in HSC-humanized mice, human T cells populate the murine thymus and become H2 restricted, they appear to remain HLA restricted in the BLT model (99, 127–129). To achieve HLA-restriction in the former, multiple HLA-transgenic NSG mice have recently been developed (110, 128, 130–133). HSC-based humanized mouse models have also been further modified by replacing distinct murine genes with the corresponding human alleles using knock-in technology, or the transcription activator like effector nuclease (TALEN) technology (134, 135). Each human factor improves the development of certain human hematopoietic lineages. Administration of human IL-15, for example, promotes human NK cell development, while human IL-7 enhances the maintenance of immature thymocytes (136–138). Similarly, transient expression of human GM-CSF and IL-4, macrophage colony-stimulating factor, or erythropoietin and IL-3 (SGM3) increase the reconstitution of DC, monocytes/macrophages, and erythrocytes, respectively (135, 136, 139). In membrane-bound human stem cell factor (SCF)-expressing NSG mice, the differentiation of immature and mature granulocytes including c-Kit+ human mast cells has been described (140).
In stem cell-transplanted humanized mice, the developing human T cell compartment includes CD4+CD25+Foxp3+ Treg. Interestingly, the transfer of human CD34+ HSC into NSG-SGM3 mice-expressing transgenes for human SCF, GM-CSF, and IL-3 not only led to elevated human myeloid cell frequencies in blood, spleen, bone marrow, and liver compared with non-transgenic NSG recipients, but also to a significant increase in the CD4+CD25+Foxp3+ Treg population. Furthermore, the authors demonstrated Treg expansion in the periphery and not in the thymus as the numbers of CD4+Foxp3+ thymocytes were similar in both mouse strains. Human DC subsets capable of regulating Treg cell frequencies were suggested as one possible mechanism (141, 142). In these models, Treg have been shown to suppress polyclonally activated T cell proliferation (141) and can be engaged to prevent fibrosis (143).
Unfortunately, like in the transfer models, HSC-humanized mice develop GvHD (144–146). Onset of GvHD is not prevented by the lack of murine MHC molecules and develops despite the presence of human Treg. The development of GvHD in apparently all models limits the experimental window of other immune responses and shows that the possible involvement of GvHD, even if it cannot be detected based on established parameters and its possible influence on other immune responses needs to be taken into account.
Considering the many differences between the species, the growth and survival of human cells in mice is still a surprising find. Many, if not the majority of murine cytokines and growth factors, do not bind or insufficiently interact with the respective human receptors, and the cells need to adapt to this situation. In fact, on closer characterization, proliferating xenoresponsive human T cells differentiate into unusual subsets in the xenogeneic environment (147, 148). Moreover, phenotypical and functional analyses of lymphoid lineages in humanized mice >20 weeks posthematopoietic stem cell transplantation reveals that CD8+ T cells and NK cells exhibited functional impairments (149). These observations remind that in humanized mouse models, the mere presence of certain cell types cannot be taken for their functional property. It is, for example, not known whether the cellular and molecular mechanisms that govern the differentiation of the Treg population in mice also applies to HSC-based models (150). While in the mouse, the composition of the Treg population is significantly influenced by commensals, nothing is known in the humanized mouse models (151). Thus, in order to translate potential Treg therapy into the clinic, the origin, fate, and function of Treg in humanized mice must be examined in greater detail.
In summary, in their current state, transfer models provide a robust means to evaluate the interaction of Treg with T cells, particularly in conjunction with Treg activating or inactivating reagents. HSC-based humanized mouse models offer greater possibilities but are difficult to set up and less well explored with regard to Treg development and function. Observations in SGM3 mice are encouraging, and further outfitting mice with additional human factors may help to overcome existing limitations, which might lead to models in where human Treg function and development can be studied in a physiological manner.
Author Contributions
SH and BT: substantial contributions to the conception and design of the work; IB and CB: substantial contributions to the conception of the work.
Conflict of Interest Statement
The authors declare that the research was conducted in the absence of any commercial or financial relationships that could be constructed as a potential conflict of interest.
Acknowledgments
We thank PD Dr. Andrea Tüttenberg, Prof. Kerstin Steinbrink, Dr. Verena Raker, and Natascha Luther for discussion and critically reading the manuscript. This work was supported by the Deutsche Forschungsgemeinschaft BE 4504/2-2 to IB and by funds from the German Federal Ministry of Education and Research (BMBF 01EO1003) to CB.
References
1. Gershon RK, Kondo K. Cell interactions in the induction of tolerance: the role of thymic lymphocytes. Immunology (1970) 18(5):723–37.
3. Dorf ME, Benacerraf B. Suppressor cells and immunoregulation. Annu Rev Immunol (1984) 2:127–57. doi: 10.1146/annurev.iy.02.040184.001015
4. Herzenberg LA, Tokuhisa T, Hayakawa K. Epitope-specific regulation. Annu Rev Immunol (1983) 1:609–32. doi:10.1146/annurev.iy.01.040183.003141
5. Sakaguchi S, Sakaguchi N, Asano M, Itoh M, Toda M. Immunologic self-tolerance maintained by activated T cells expressing IL-2 receptor alpha-chains (CD25). Breakdown of a single mechanism of self-tolerance causes various autoimmune diseases. J Immunol (1995) 155:1151–64.
6. Chatila TA, Blaeser F, Ho N, Lederman HM, Voulgaropoulos C, Helms C, et al. JM2, encoding a fork head-related protein, is mutated in X-linked autoimmunity-allergic disregulation syndrome. J Clin Invest (2000) 106(12):R75–81. doi:10.1172/JCI11679
7. Brunkow ME, Jeffery EW, Hjerrild KA, Paeper B, Clark LB, Yasayko SA, et al. Disruption of a new forkhead/winged-helix protein, scurfin, results in the fatal lymphoproliferative disorder of the scurfy mouse. Nat Genet (2001) 27(1):68–73. doi:10.1038/83784
8. Bennett CL, Christie J, Ramsdell F, Brunkow ME, Ferguson PJ, Whitesell L, et al. The immune dysregulation, polyendocrinopathy, enteropathy, X-linked syndrome (IPEX) is caused by mutations of FOXP3. Nat Genet (2001) 27(1):20–1. doi:10.1038/83713
9. Wildin RS, Ramsdell F, Peake J, Faravelli F, Casanova JL, Buist N, et al. X-linked neonatal diabetes mellitus, enteropathy and endocrinopathy syndrome is the human equivalent of mouse scurfy. Nat Genet (2001) 27(1):18–20. doi:10.1038/83707
10. Hori S, Nomura T, Sakaguchi S. Control of regulatory T cell development by the transcription factor Foxp3. Science (2003) 299(5609):1057–61. doi:10.1126/science.1079490
11. Fontenot JD, Gavin MA, Rudensky AY. Foxp3 programs the development and function of CD4+CD25+ regulatory T cells. Nat Immunol (2003) 4(4):330–6. doi:10.1038/ni904
12. Dieckmann D, Bruett CH, Ploettner H, Lutz MB, Schuler G. Human CD4(+)CD25(+) regulatory, contact-dependent T cells induce interleukin 10-producing, contact-independent type 1-like regulatory T cells [corrected]. J Exp Med (2002) 196(2):247–53. doi:10.1084/jem.20020642
13. Jonuleit H, Schmitt E, Kakirman H, Stassen M, Knop J, Enk AH. Infectious tolerance: human CD25(+) regulatory T cells convey suppressor activity to conventional CD4(+) T helper cells. J Exp Med (2002) 196(2):255–60. doi:10.1084/jem.20020394
14. Moraes-Vasconcelos D, Costa-Carvalho BT, Torgerson TR, Ochs HD. Primary immune deficiency disorders presenting as autoimmune diseases: IPEX and APECED. J Clin Immunol (2008) 28(Suppl 1):S11–9. doi:10.1007/s10875-008-9176-5
15. Kim JM, Rasmussen JP, Rudensky AY. Regulatory T cells prevent catastrophic autoimmunity throughout the lifespan of mice. Nat Immunol (2007) 8(2):191–7. doi:10.1038/ni1428
16. Tang Q, Bluestone JA. The Foxp3+ regulatory T cell: a jack of all trades, master of regulation. Nat Immunol (2008) 9(3):239–44. doi:10.1038/ni1572
17. Paterson AM, Lovitch SB, Sage PT, Juneja VR, Lee Y, Trombley JD, et al. Deletion of CTLA-4 on regulatory T cells during adulthood leads to resistance to autoimmunity. J Exp Med (2015) 212(10):1603–21. doi:10.1084/jem.20141030
18. Rubtsov YP, Rasmussen JP, Chi EY, Fontenot J, Castelli L, Ye X, et al. Regulatory T cell-derived interleukin-10 limits inflammation at environmental interfaces. Immunity (2008) 28(4):546–58. doi:10.1016/j.immuni.2008.02.017
19. Bopp T, Becker C, Klein M, Klein-Hessling S, Palmetshofer A, Serfling E, et al. Cyclic adenosine monophosphate is a key component of regulatory T cell-mediated suppression. J Exp Med (2007) 204(6):1303–10. doi:10.1084/jem.20062129
20. Becker C, Taube C, Bopp T, Becker C, Michel K, Kubach J, et al. Protection from graft-versus-host disease by HIV-1 envelope protein gp120-mediated activation of human CD4+CD25+ regulatory T cells. Blood (2009) 114(6):1263–9. doi:10.1182/blood-2009-02-206730
21. Ziegler SF. FOXP3: of mice and men. Annu Rev Immunol (2006) 24:209–26. doi:10.1146/annurev.immunol.24.021605.090547
22. Aluvihare VR, Kallikourdis M, Betz AG. Regulatory T cells mediate maternal tolerance to the fetus. Nat Immunol (2004) 5(3):266–71. doi:10.1038/ni1037
23. Kahn DA, Baltimore D. Pregnancy induces a fetal antigen-specific maternal T regulatory cell response that contributes to tolerance. Proc Natl Acad Sci U S A (2010) 107(20):9299–304. doi:10.1073/pnas.1003909107
24. Kullberg MC, Jankovic D, Gorelick PL, Caspar P, Letterio JJ, Cheever AW, et al. Bacteria-triggered CD4(+) T regulatory cells suppress Helicobacter hepaticus-induced colitis. J Exp Med (2002) 196(4):505–15. doi:10.1084/jem.20020556
25. Karlsson MR, Rugtveit J, Brandtzaeg P. Allergen-responsive CD4+CD25+ regulatory T cells in children who have outgrown cow’s milk allergy. J Exp Med (2004) 199(12):1679–88. doi:10.1084/jem.20032121
26. Planas AM, Chamorro A. Regulatory T cells protect the brain after stroke. Nat Med (2009) 15(2):138–9. doi:10.1038/nm0209-138
27. Oldenhove G, de Heusch M, Urbain-Vansanten G, Urbain J, Maliszewski C, Leo O, et al. CD4+ CD25+ regulatory T cells control T helper cell type 1 responses to foreign antigens induced by mature dendritic cells in vivo. J Exp Med (2003) 198(2):259–66. doi:10.1084/jem.20030654
28. Putnam AL, Safinia N, Medvec A, Laszkowska M, Wray M, Mintz MA, et al. Clinical grade manufacturing of human alloantigen-reactive regulatory T cells for use in transplantation. Am J Transplant (2013) 13(11):3010–20. doi:10.1111/ajt.12433
29. Stassen M, Fondel S, Bopp T, Richter C, Muller C, Kubach J, et al. Human CD25+ regulatory T cells: two subsets defined by the integrins alpha 4 beta 7 or alpha 4 beta 1 confer distinct suppressive properties upon CD4+ T helper cells. Eur J Immunol (2004) 34(5):1303–11. doi:10.1002/eji.200324656
30. Valmori D, Merlo A, Souleimanian NE, Hesdorffer CS, Ayyoub M. A peripheral circulating compartment of natural naive CD4 Tregs. J Clin Invest (2005) 115(7):1953–62. doi:10.1172/JCI23963
31. Ermann J, Hoffmann P, Edinger M, Dutt S, Blankenberg FG, Higgins JP, et al. Only the CD62L+ subpopulation of CD4+CD25+ regulatory T cells protects from lethal acute GVHD. Blood (2005) 105(5):2220–6. doi:10.1182/blood-2004-05-2044
32. Fritzsching B, Oberle N, Pauly E, Geffers R, Buer J, Poschl J, et al. Naive regulatory T cells: a novel subpopulation defined by resistance toward CD95L-mediated cell death. Blood (2006) 108(10):3371–8. doi:10.1182/blood-2006-02-005660
33. Baecher-Allan C, Wolf E, Hafler DA. MHC class II expression identifies functionally distinct human regulatory T cells. J Immunol (2006) 176(8):4622–31. doi:10.4049/jimmunol.176.8.4622
34. Battaglia A, Buzzonetti A, Monego G, Peri L, Ferrandina G, Fanfani F, et al. Neuropilin-1 expression identifies a subset of regulatory T cells in human lymph nodes that is modulated by preoperative chemoradiation therapy in cervical cancer. Immunology (2008) 123(1):129–38. doi:10.1111/j.1365-2567.2007.02737.x
35. Nagata S, Ise T, Pastan I. Fc receptor-like 3 protein expressed on IL-2 nonresponsive subset of human regulatory T cells. J Immunol (2009) 182(12):7518–26. doi:10.4049/jimmunol.0802230
36. Burzyn D, Kuswanto W, Kolodin D, Shadrach JL, Cerletti M, Jang Y, et al. A special population of regulatory T cells potentiates muscle repair. Cell (2013) 155(6):1282–95. doi:10.1016/j.cell.2013.10.054
37. Afzali B, Mitchell PJ, Edozie FC, Povoleri GA, Dowson SE, Demandt L, et al. CD161 expression characterizes a subpopulation of human regulatory T cells that produces IL-17 in a STAT3-dependent manner. Eur J Immunol (2013) 43(8):2043–54. doi:10.1002/eji.201243296
38. Ulges A, Klein M, Reuter S, Gerlitzki B, Hoffmann M, Grebe N, et al. Protein kinase CK2 enables regulatory T cells to suppress excessive TH2 responses in vivo. Nat Immunol (2015) 16(3):267–75. doi:10.1038/ni.3083
39. Feuerer M, Herrero L, Cipolletta D, Naaz A, Wong J, Nayer A, et al. Lean, but not obese, fat is enriched for a unique population of regulatory T cells that affect metabolic parameters. Nat Med (2009) 15(8):930–9. doi:10.1038/nm.2002
40. Ehrentraut H, Clambey ET, McNamee EN, Brodsky KS, Ehrentraut SF, Poth JM, et al. CD73+ regulatory T cells contribute to adenosine-mediated resolution of acute lung injury. FASEB J (2013) 27(6):2207–19. doi:10.1096/fj.12-225201
41. Hofmann U, Beyersdorf N, Weirather J, Podolskaya A, Bauersachs J, Ertl G, et al. Activation of CD4+ T lymphocytes improves wound healing and survival after experimental myocardial infarction in mice. Circulation (2012) 125(13):1652–63. doi:10.1161/CIRCULATIONAHA.111.044164
42. Banham AH, Powrie FM, Suri-Payer E. FOXP3+ regulatory T cells: current controversies and future perspectives. Eur J Immunol (2006) 36(11):2832–6. doi:10.1002/eji.200636459
43. Correll A, Tuettenberg A, Becker C, Jonuleit H. Increased regulatory T-cell frequencies in patients with advanced melanoma correlate with a generally impaired T-cell responsiveness and are restored after dendritic cell-based vaccination. Exp Dermatol (2010) 19(8):e213–21. doi:10.1111/j.1600-0625.2009.01055.x
44. Powell DJ Jr, Parker LL, Rosenberg SA. Large-scale depletion of CD25+ regulatory T cells from patient leukapheresis samples. J Immunother (2005) 28(4):403–11. doi:10.1097/01.cji.0000170363.22585.5a
45. Hoffmann P, Eder R, Boeld TJ, Doser K, Piseshka B, Andreesen R, et al. Only the CD45RA+ subpopulation of CD4+CD25high T cells gives rise to homogeneous regulatory T-cell lines upon in vitro expansion. Blood (2006) 108(13):4260–7. doi:10.1182/blood-2006-06-027409
46. Baron U, Floess S, Wieczorek G, Baumann K, Grutzkau A, Dong J, et al. DNA demethylation in the human FOXP3 locus discriminates regulatory T cells from activated FOXP3(+) conventional T cells. Eur J Immunol (2007) 37(9):2378–89. doi:10.1002/eji.200737594
47. Wieczorek G, Asemissen A, Model F, Turbachova I, Floess S, Liebenberg V, et al. Quantitative DNA methylation analysis of FOXP3 as a new method for counting regulatory T cells in peripheral blood and solid tissue. Cancer Res (2009) 69(2):599–608. doi:10.1158/0008-5472.CAN-08-2361
48. St Clair EW. The calm after the cytokine storm: lessons from the TGN1412 trial. J Clin Invest (2008) 118(4):1344–7. doi:10.1172/JCI35382
49. Asano M, Toda M, Sakaguchi N, Sakaguchi S. Autoimmune disease as a consequence of developmental abnormality of a T cell subpopulation. J Exp Med (1996) 184(2):387–96. doi:10.1084/jem.184.2.387
50. Hill JA, Benoist C, Mathis D. Treg cells: guardians for life. Nat Immunol (2007) 8(2):124–5. doi:10.1038/ni0207-124
51. Brusko TM, Putnam AL, Bluestone JA. Human regulatory T cells: role in autoimmune disease and therapeutic opportunities. Immunol Rev (2008) 223:371–90. doi:10.1111/j.1600-065X.2008.00637.x
52. von Boehmer H, Daniel C. Therapeutic opportunities for manipulating T(Reg) cells in autoimmunity and cancer. Nat Rev Drug Discov (2013) 12(1):51–63. doi:10.1038/nrd3683
53. Zozulya AL, Wiendl H. The role of regulatory T cells in multiple sclerosis. Nat Clin Pract Neurol (2008) 4(7):384–98. doi:10.1038/ncpneuro0832
54. Haas J, Hug A, Viehover A, Fritzsching B, Falk CS, Filser A, et al. Reduced suppressive effect of CD4+CD25high regulatory T cells on the T cell immune response against myelin oligodendrocyte glycoprotein in patients with multiple sclerosis. Eur J Immunol (2005) 35(11):3343–52. doi:10.1002/eji.200526065
55. Baecher-Allan C, Hafler DA. Suppressor T cells in human diseases. J Exp Med (2004) 200(3):273–6. doi:10.1084/jem.20040812
56. Long SA, Buckner JH. CD4+FOXP3+ T regulatory cells in human autoimmunity: more than a numbers game. J Immunol (2011) 187(5):2061–6. doi:10.4049/jimmunol.1003224
57. Neurath MF, Finotto S. IL-6 signaling in autoimmunity, chronic inflammation and inflammation-associated cancer. Cytokine Growth Factor Rev (2011) 22(2):83–9. doi:10.1016/j.cytogfr.2011.02.003
58. Goodman WA, Cooper KD, McCormick TS. Regulation generation: the suppressive functions of human regulatory T cells. Crit Rev Immunol (2012) 32(1):65–79. doi:10.1615/CritRevImmunol.v32.i1.40
59. Jin Y, Chen X, Podolsky R, Hopkins D, Makala LH, Muir A, et al. APC dysfunction is correlated with defective suppression of T cell proliferation in human type 1 diabetes. Clin Immunol (2009) 130(3):272–9. doi:10.1016/j.clim.2008.10.005
60. Trinschek B, Luessi F, Haas J, Wildemann B, Zipp F, Wiendl H, et al. Kinetics of IL-6 production defines T effector cell responsiveness to regulatory T cells in multiple sclerosis. PLoS One (2013) 8(10):e77634. doi:10.1371/journal.pone.0077634
61. Wehrens EJ, Mijnheer G, Duurland CL, Klein M, Meerding J, van Loosdregt J, et al. Functional human regulatory T cells fail to control autoimmune inflammation due to PKB/c-akt hyperactivation in effector cells. Blood (2011) 118(13):3538–48. doi:10.1182/blood-2010-12-328187
62. Trinschek B, Luessi F, Gross CC, Wiendl H, Jonuleit H. Interferon-beta therapy of multiple sclerosis patients improves the responsiveness of T cells for immune suppression by regulatory T cells. Int J Mol Sci (2015) 16(7):16330–46. doi:10.3390/ijms160716330
63. Curotto de Lafaille MA, Lafaille JJ. Natural and adaptive foxp3+ regulatory T cells: more of the same or a division of labor? Immunity (2009) 30(5):626–35. doi:10.1016/j.immuni.2009.05.002
64. Haas J, Fritzsching B, Trubswetter P, Korporal M, Milkova L, Fritz B, et al. Prevalence of newly generated naive regulatory T cells (Treg) is critical for Treg suppressive function and determines Treg dysfunction in multiple sclerosis. J Immunol (2007) 179(2):1322–30. doi:10.4049/jimmunol.179.2.1322
65. Venken K, Hellings N, Hensen K, Rummens JL, Medaer R, D’hooghe MB, et al. Secondary progressive in contrast to relapsing-remitting multiple sclerosis patients show a normal CD4+CD25+ regulatory T-cell function and FOXP3 expression. J Neurosci Res (2006) 83(8):1432–46. doi:10.1002/jnr.20852
66. Kleinewietfeld M, Hafler DA. Regulatory T cells in autoimmune neuroinflammation. Immunol Rev (2014) 259(1):231–44. doi:10.1111/imr.12169
67. Cooles FA, Isaacs JD, Anderson AE. Treg cells in rheumatoid arthritis: an update. Curr Rheumatol Rep (2013) 15(9):352. doi:10.1007/s11926-013-0352-0
68. Alunno A, Bartoloni E, Nocentini G, Bistoni O, Ronchetti S, Petrillo MG, et al. Role of regulatory T cells in rheumatoid arthritis: facts and hypothesis. Auto Immun Highlights (2010) 1(1):45–51. doi:10.1007/s13317-010-0008-2
69. Brusko T, Atkinson M. Treg in type 1 diabetes. Cell Biochem Biophys (2007) 48(2–3):165–75. doi:10.1007/s12013-007-0018-5
70. Clough LE, Wang CJ, Schmidt EM, Booth G, Hou TZ, Ryan GA, et al. Release from regulatory T cell-mediated suppression during the onset of tissue-specific autoimmunity is associated with elevated IL-21. J Immunol (2008) 180(8):5393–401. doi:10.4049/jimmunol.180.8.5393
71. Muzes G, Molnar B, Sipos F. Regulatory T cells in inflammatory bowel diseases and colorectal cancer. World J Gastroenterol (2012) 18(40):5688–94. doi:10.3748/wjg.v18.i40.5688
72. Baumgart DC, Sandborn WJ. Crohn’s disease. Lancet (2012) 380(9853):1590–605. doi:10.1016/S0140-6736(12)60026-9
73. Crispin JC, Martinez A, Alcocer-Varela J. Quantification of regulatory T cells in patients with systemic lupus erythematosus. J Autoimmun (2003) 21(3):273–6. doi:10.1016/S0896-8411(03)00121-5
74. Zhang L, Yang XQ, Cheng J, Hui RS, Gao TW. Increased Th17 cells are accompanied by FoxP3(+) Treg cell accumulation and correlated with psoriasis disease severity. Clin Immunol (2010) 135(1):108–17. doi:10.1016/j.clim.2009.11.008
75. Hirahara K, Liu L, Clark RA, Yamanaka K, Fuhlbrigge RC, Kupper TS. The majority of human peripheral blood CD4+CD25highFoxp3+ regulatory T cells bear functional skin-homing receptors. J Immunol (2006) 177(7):4488–94. doi:10.4049/jimmunol.177.7.4488
76. Sather BD, Treuting P, Perdue N, Miazgowicz M, Fontenot JD, Rudensky AY, et al. Altering the distribution of Foxp3(+) regulatory T cells results in tissue-specific inflammatory disease. J Exp Med (2007) 204(6):1335–47. doi:10.1084/jem.20070081
77. Pardoll D. Does the immune system see tumors as foreign or self? Annu Rev Immunol (2003) 21:807–39. doi:10.1146/annurev.immunol.21.120601.141135
78. Nishikawa H, Kato T, Tawara I, Saito K, Ikeda H, Kuribayashi K, et al. Definition of target antigens for naturally occurring CD4(+) CD25(+) regulatory T cells. J Exp Med (2005) 201(5):681–6. doi:10.1084/jem.20041959
79. Beyer M, Schultze JL. Regulatory T cells in cancer. Blood (2006) 108(3):804–11. doi:10.1182/blood-2006-02-002774
80. Duan MC, Zhong XN, Liu GN, Wei JR. The Treg/Th17 paradigm in lung cancer. J Immunol Res (2014) 2014:730380. doi:10.1155/2014/730380
81. Whiteside TL. Regulatory T cell subsets in human cancer: are they regulating for or against tumor progression? Cancer Immunol Immunother (2014) 63(1):67–72. doi:10.1007/s00262-013-1490-y
82. Wilke CM, Wu K, Zhao E, Wang G, Zou W. Prognostic significance of regulatory T cells in tumor. Int J Cancer (2010) 127(4):748–58. doi:10.1002/ijc.25464
83. Curiel TJ, Coukos G, Zou L, Alvarez X, Cheng P, Mottram P, et al. Specific recruitment of regulatory T cells in ovarian carcinoma fosters immune privilege and predicts reduced survival. Nat Med (2004) 10(9):942–9. doi:10.1038/nm1093
84. Ishida T, Ishii T, Inagaki A, Yano H, Komatsu H, Iida S, et al. Specific recruitment of CC chemokine receptor 4-positive regulatory T cells in Hodgkin lymphoma fosters immune privilege. Cancer Res (2006) 66(11):5716–22. doi:10.1158/0008-5472.CAN-06-0261
85. Cao X, Cai SF, Fehniger TA, Song J, Collins LI, Piwnica-Worms DR, et al. Granzyme B and perforin are important for regulatory T cell-mediated suppression of tumor clearance. Immunity (2007) 27(4):635–46. doi:10.1016/j.immuni.2007.08.014
86. Sutmuller RP, van Duivenvoorde LM, van Elsas A, Schumacher TN, Wildenberg ME, Allison JP, et al. Synergism of cytotoxic T lymphocyte-associated antigen 4 blockade and depletion of cd25(+) regulatory T cells in antitumor therapy reveals alternative pathways for suppression of autoreactive cytotoxic T lymphocyte responses. J Exp Med (2001) 194(6):823–32. doi:10.1084/jem.194.6.823
87. Turk MJ, Guevara-Patino JA, Rizzuto GA, Engelhorn ME, Houghton AN. Concomitant tumor immunity to a poorly immunogenic melanoma is prevented by regulatory T cells. J Exp Med (2004) 200(6):771–82. doi:10.1084/jem.20041130
88. Klages K, Mayer CT, Lahl K, Loddenkemper C, Teng MW, Ngiow SF, et al. Selective depletion of Foxp3+ regulatory T cells improves effective therapeutic vaccination against established melanoma. Cancer Res (2010) 70(20):7788–99. doi:10.1158/0008-5472.CAN-10-1736
89. Barnett B, Kryczek I, Cheng P, Zou W, Curiel TJ. Regulatory T cells in ovarian cancer: biology and therapeutic potential. Am J Reprod Immunol (2005) 54(6):369–77. doi:10.1111/j.1600-0897.2005.00330.x
90. Peggs KS, Quezada SA, Chambers CA, Korman AJ, Allison JP. Blockade of CTLA-4 on both effector and regulatory T cell compartments contributes to the antitumor activity of anti-CTLA-4 antibodies. J Exp Med (2009) 206(8):1717–25. doi:10.1084/jem.20082492
91. McCune JM, Namikawa R, Kaneshima H, Shultz LD, Lieberman M, Weissman IL. The SCID-hu mouse: murine model for the analysis of human hematolymphoid differentiation and function. Science (1988) 241(4873):1632–9. doi:10.1126/science.2971269
92. Mosier DE, Gulizia RJ, Baird SM, Wilson DB. Transfer of a functional human immune system to mice with severe combined immunodeficiency. Nature (1988) 335(6187):256–9. doi:10.1038/335256a0
93. Brehm MA, Jouvet N, Greiner DL, Shultz LD. Humanized mice for the study of infectious diseases. Curr Opin Immunol (2013) 25(4):428–35. doi:10.1016/j.coi.2013.05.012
94. Ito M, Hiramatsu H, Kobayashi K, Suzue K, Kawahata M, Hioki K, et al. NOD/SCID/gamma(c)(null) mouse: an excellent recipient mouse model for engraftment of human cells. Blood (2002) 100(9):3175–82. doi:10.1182/blood-2001-12-0207
95. Ito R, Takahashi T, Katano I, Ito M. Current advances in humanized mouse models. Cell Mol Immunol (2012) 9(3):208–14. doi:10.1038/cmi.2012.2
96. King M, Pearson T, Shultz LD, Leif J, Bottino R, Trucco M, et al. A new Hu-PBL model for the study of human islet alloreactivity based on NOD-scid mice bearing a targeted mutation in the IL-2 receptor gamma chain gene. Clin Immunol (2008) 126(3):303–14. doi:10.1016/j.clim.2007.11.001
97. Macchiarini F, Manz MG, Palucka AK, Shultz LD. Humanized mice: are we there yet? J Exp Med (2005) 202(10):1307–11. doi:10.1084/jem.20051547
98. Shultz LD, Pearson T, King M, Giassi L, Carney L, Gott B, et al. Humanized NOD/LtSz-scid IL2 receptor common gamma chain knockout mice in diabetes research. Ann N Y Acad Sci (2007) 1103:77–89. doi:10.1196/annals.1394.002
99. Shultz LD, Brehm MA, Garcia-Martinez JV, Greiner DL. Humanized mice for immune system investigation: progress, promise and challenges. Nat Rev Immunol (2012) 12(11):786–98. doi:10.1038/nri3311
100. King MA, Covassin L, Brehm MA, Racki W, Pearson T, Leif J, et al. Human peripheral blood leucocyte non-obese diabetic-severe combined immunodeficiency interleukin-2 receptor gamma chain gene mouse model of xenogeneic graft-versus-host-like disease and the role of host major histocompatibility complex. Clin Exp Immunol (2009) 157(1):104–18. doi:10.1111/j.1365-2249.2009.03933.x
101. Mutis T, van Rijn RS, Simonetti ER, arts-Riemens T, Emmelot ME, van Bloois L, et al. Human regulatory T cells control xenogeneic graft-versus-host disease induced by autologous T cells in RAG2-/-gammac-/- immunodeficient mice. Clin Cancer Res (2006) 12(18):5520–5. doi:10.1158/1078-0432.CCR-06-0035
102. Hahn SA, Stahl HF, Becker C, Correll A, Schneider FJ, Tuettenberg A, et al. Soluble GARP has potent antiinflammatory and immunomodulatory impact on human CD4(+) T cells. Blood (2013) 122(7):1182–91. doi:10.1182/blood-2012-12-474478
103. Klein M, Vaeth M, Scheel T, Grabbe S, Baumgrass R, Berberich-Siebelt F, et al. Repression of cyclic adenosine monophosphate upregulation disarms and expands human regulatory T cells. J Immunol (2012) 188(3):1091–7. doi:10.4049/jimmunol.1102045
104. Kinter A, McNally J, Riggin L, Jackson R, Roby G, Fauci AS. Suppression of HIV-specific T cell activity by lymph node CD25+ regulatory T cells from HIV-infected individuals. Proc Natl Acad Sci U S A (2007) 104(9):3390–5. doi:10.1073/pnas.0611423104
105. Tran DQ, Andersson J, Hardwick D, Bebris L, Illei GG, Shevach EM. Selective expression of latency-associated peptide (LAP) and IL-1 receptor type I/II (CD121a/CD121b) on activated human FOXP3+ regulatory T cells allows for their purification from expansion cultures. Blood (2009) 113(21):5125–33. doi:10.1182/blood-2009-01-199950
106. Wang Y, Zhu M, Yu P, Fu YX. Promoting immune responses by LIGHT in the face of abundant regulatory T cell inhibition. J Immunol (2010) 184(3):1589–95. doi:10.4049/jimmunol.0901582
107. Bacher N, Raker V, Hofmann C, Graulich E, Schwenk M, Baumgrass R, et al. Interferon-alpha suppresses cAMP to disarm human regulatory T cells. Cancer Res (2013) 73(18):5647–56. doi:10.1158/0008-5472.CAN-12-3788
108. Klein L, Jovanovic K. Regulatory T cell differentiation: turning harmful into useful. Immunity (2012) 37(3):441–3. doi:10.1016/j.immuni.2012.09.002
109. Pino S, Brehm MA, Covassin-Barberis L, King M, Gott B, Chase TH, et al. Development of novel major histocompatibility complex class I and class II-deficient NOD-SCID IL2R gamma chain knockout mice for modeling human xenogeneic graft-versus-host disease. Methods Mol Biol (2010) 602:105–17. doi:10.1007/978-1-60761-058-8_7
110. Shultz LD, Saito Y, Najima Y, Tanaka S, Ochi T, Tomizawa M, et al. Generation of functional human T-cell subsets with HLA-restricted immune responses in HLA class I expressing NOD/SCID/IL2r gamma(null) humanized mice. Proc Natl Acad Sci U S A (2010) 107(29):13022–7. doi:10.1073/pnas.1000475107
111. Eschborn M, Weigmann B, Reissig S, Waisman A, Saloga J, Bellinghausen I. Activated glycoprotein A repetitions predominant (GARP)-expressing regulatory T cells inhibit allergen-induced intestinal inflammation in humanized mice. J Allergy Clin Immunol (2015) 136(1):159–68. doi:10.1016/j.jaci.2015.04.020
112. Martin H, Reuter S, Dehzad N, Heinz A, Bellinghausen I, Saloga J, et al. CD4-mediated regulatory T-cell activation inhibits the development of disease in a humanized mouse model of allergic airway disease. J Allergy Clin Immunol (2012) 129(2):521–8. doi:10.1016/j.jaci.2011.09.038
113. Weigmann B, Schughart N, Wiebe C, Sudowe S, Lehr HA, Jonuleit H, et al. Allergen-induced IgE-dependent gut inflammation in a human PBMC-engrafted murine model of allergy. J Allergy Clin Immunol (2012) 129(4):1126–35. doi:10.1016/j.jaci.2011.11.036
114. Bellinghausen I, Reuter S, Martin H, Maxeiner J, Luxemburger U, Tureci O, et al. Enhanced production of CCL18 by tolerogenic dendritic cells is associated with inhibition of allergic airway reactivity. J Allergy Clin Immunol (2012) 130(6):1384–93. doi:10.1016/j.jaci.2012.08.039
115. Issa F, Hester J, Goto R, Nadig SN, Goodacre TE, Wood K. Ex vivo-expanded human regulatory T cells prevent the rejection of skin allografts in a humanized mouse model. Transplantation (2010) 90(12):1321–7. doi:10.1097/TP.0b013e3181ff8772
116. Nadig SN, Wieckiewicz J, Wu DC, Warnecke G, Zhang W, Luo S, et al. In vivo prevention of transplant arteriosclerosis by ex vivo-expanded human regulatory T cells. Nat Med (2010) 16(7):809–13. doi:10.1038/nm.2154
117. Racki WJ, Covassin L, Brehm M, Pino S, Ignotz R, Dunn R, et al. NOD-scid IL2rgamma(null) mouse model of human skin transplantation and allograft rejection. Transplantation (2010) 89(5):527–36. doi:10.1097/TP.0b013e3181c90242
118. Wu DC, Hester J, Nadig SN, Zhang W, Trzonkowski P, Gray D, et al. Ex vivo expanded human regulatory T cells can prolong survival of a human islet allograft in a humanized mouse model. Transplantation (2013) 96(8):707–16. doi:10.1097/TP.0b013e31829fa271
119. Sommer W, Knofel AK, Madrahimov N, Avsar M, Jonigk D, Salman J, et al. Allogeneic CD4+CD25high T cells regulate obliterative bronchiolitis of heterotopic bronchus allografts in both porcinized and humanized mouse models. Transplantation (2015) 99(3):482–91. doi:10.1097/TP.0000000000000632
120. Noyan F, Lee YS, Hardtke-Wolenski M, Knoefel AK, Taubert R, Baron U, et al. Donor-specific regulatory T cells generated on donor B cells are superior to CD4+CD25high cells in controlling alloimmune responses in humanized mice. Transplant Proc (2013) 45(5):1832–7. doi:10.1016/j.transproceed.2013.01.073
121. Wistuba II, Behrens C, Milchgrub S, Syed S, Ahmadian M, Virmani AK, et al. Comparison of features of human breast cancer cell lines and their corresponding tumors. Clin Cancer Res (1998) 4(12):2931–8.
122. Ince TA, Sousa AD, Jones MA, Harrell JC, Agoston ES, Krohn M, et al. Characterization of twenty-five ovarian tumour cell lines that phenocopy primary tumours. Nat Commun (2015) 6:7419. doi:10.1038/ncomms8419
123. Kubach J, Hubo M, Amendt C, Stroh C, Jonuleit H. IgG1 anti-epidermal growth factor receptor antibodies induce CD8-dependent antitumor activity. Int J Cancer (2015) 136(4):821–30. doi:10.1002/ijc.29037
124. Sanmamed MF, Rodriguez I, Schalper KA, Onate C, Azpilikueta A, Rodriguez-Ruiz ME, et al. Nivolumab and urelumab enhance antitumor activity of human T lymphocytes engrafted in Rag2-/-IL2Rgammanull immunodeficient mice. Cancer Res (2015) 75(17):3466–78. doi:10.1158/0008-5472.CAN-14-3510
125. Lacerda JF, Ladanyi M, Louie DC, Fernandez JM, Papadopoulos EB, O’Reilly RJ. Human Epstein-Barr virus (EBV)-specific cytotoxic T lymphocytes home preferentially to and induce selective regressions of autologous EBV-induced B cell lymphoproliferations in xenografted C.B-17 scid/scid mice. J Exp Med (1996) 183(3):1215–28. doi:10.1084/jem.183.3.1215
126. Distler E, Albrecht J, Brunk A, Khan S, Schnurer E, Frey M, et al. Patient-individualized CD8 cytolytic T-cell therapy effectively combats minimal residual leukemia in immunodeficient mice. Int J Cancer (2015). doi:10.1002/ijc.29854
127. Marsden MD, Zack JA. Studies of retroviral infection in humanized mice. Virology (2015) 47(9–480):297–309. doi:10.1016/j.virol.2015.01.017
128. Rongvaux A, Takizawa H, Strowig T, Willinger T, Eynon EE, Flavell RA, et al. Human hemato-lymphoid system mice: current use and future potential for medicine. Annu Rev Immunol (2013) 31:635–74. doi:10.1146/annurev-immunol-032712-095921
129. Wege AK, Melkus MW, Denton PW, Estes JD, Garcia JV. Functional and phenotypic characterization of the humanized BLT mouse model. Curr Top Microbiol Immunol (2008) 324:149–65. doi:10.1007/978-3-540-75647-7_10
130. Brehm MA, Shultz LD, Luban J, Greiner DL. Overcoming current limitations in humanized mouse research. J Infect Dis (2013) 208(Suppl 2):S125–30. doi:10.1093/infdis/jit319
131. Ishikawa F, Yasukawa M, Lyons B, Yoshida S, Miyamoto T, Yoshimoto G, et al. Development of functional human blood and immune systems in NOD/SCID/IL2 receptor {gamma} chain(null) mice. Blood (2005) 106(5):1565–73. doi:10.1182/blood-2005-02-0516
132. Jaiswal S, Pazoles P, Woda M, Shultz LD, Greiner DL, Brehm MA, et al. Enhanced humoral and HLA-A2-restricted dengue virus-specific T-cell responses in humanized BLT NSG mice. Immunology (2012) 136(3):334–43. doi:10.1111/j.1365-2567.2012.03585.x
133. Watanabe Y, Takahashi T, Okajima A, Shiokawa M, Ishii N, Katano I, et al. The analysis of the functions of human B and T cells in humanized NOD/shi-scid/gammac(null) (NOG) mice (hu-HSC NOG mice). Int Immunol (2009) 21(7):843–58. doi:10.1093/intimm/dxp050
134. Bogdanove AJ, Voytas DF. TAL effectors: customizable proteins for DNA targeting. Science (2011) 333(6051):1843–6. doi:10.1126/science.1204094
135. Willinger T, Rongvaux A, Takizawa H, Yancopoulos GD, Valenzuela DM, Murphy AJ, et al. Human IL-3/GM-CSF knock-in mice support human alveolar macrophage development and human immune responses in the lung. Proc Natl Acad Sci U S A (2011) 108(6):2390–5. doi:10.1073/pnas.1019682108
136. Chen Q, Khoury M, Chen J. Expression of human cytokines dramatically improves reconstitution of specific human-blood lineage cells in humanized mice. Proc Natl Acad Sci U S A (2009) 106(51):21783–8. doi:10.1073/pnas.0912274106
137. Huntington ND, Legrand N, Alves NL, Jaron B, Weijer K, Plet A, et al. IL-15 trans-presentation promotes human NK cell development and differentiation in vivo. J Exp Med (2009) 206(1):25–34. doi:10.1084/jem.20082013
138. van Lent AU, Dontje W, Nagasawa M, Siamari R, Bakker AQ, Pouw SM, et al. IL-7 enhances thymic human T cell development in “human immune system” Rag2-/-IL-2Rgammac-/- mice without affecting peripheral T cell homeostasis. J Immunol (2009) 183(12):7645–55. doi:10.4049/jimmunol.0902019
139. Rathinam C, Poueymirou WT, Rojas J, Murphy AJ, Valenzuela DM, Yancopoulos GD, et al. Efficient differentiation and function of human macrophages in humanized CSF-1 mice. Blood (2011) 118(11):3119–28. doi:10.1182/blood-2010-12-326926
140. Takagi S, Saito Y, Hijikata A, Tanaka S, Watanabe T, Hasegawa T, et al. Membrane-bound human SCF/KL promotes in vivo human hematopoietic engraftment and myeloid differentiation. Blood (2012) 119(12):2768–77. doi:10.1182/blood-2011-05-353201
141. Billerbeck E, Barry WT, Mu K, Dorner M, Rice CM, Ploss A. Development of human CD4+FoxP3+ regulatory T cells in human stem cell factor-, granulocyte-macrophage colony-stimulating factor-, and interleukin-3-expressing NOD-SCID IL2Rgamma(null) humanized mice. Blood (2011) 117(11):3076–86. doi:10.1182/blood-2010-08-301507
142. Yamazaki S, Steinman RM. Dendritic cells as controllers of antigen-specific Foxp3+ regulatory T cells. J Dermatol Sci (2009) 54(2):69–75. doi:10.1016/j.jdermsci.2009.02.001
143. Nunoya J, Washburn ML, Kovalev GI, Su L. Regulatory T cells prevent liver fibrosis during HIV type 1 infection in a humanized mouse model. J Infect Dis (2014) 209(7):1039–44. doi:10.1093/infdis/jit548
144. Lockridge JL, Zhou Y, Becker YA, Ma S, Kenney SC, Hematti P, et al. Mice engrafted with human fetal thymic tissue and hematopoietic stem cells develop pathology resembling chronic graft-versus-host disease. Biol Blood Marrow Transplant (2013) 19(9):1310–22. doi:10.1016/j.bbmt.2013.06.007
145. Covassin L, Jangalwe S, Jouvet N, Laning J, Burzenski L, Shultz LD, et al. Human immune system development and survival of non-obese diabetic (NOD)-scid IL2rgamma(null) (NSG) mice engrafted with human thymus and autologous haematopoietic stem cells. Clin Exp Immunol (2013) 174(3):372–88. doi:10.1111/cei.12180
146. Sonntag K, Eckert F, Welker C, Muller H, Muller F, Zips D, et al. Chronic graft-versus-host-disease in CD34(+)-humanized NSG mice is associated with human susceptibility HLA haplotypes for autoimmune disease. J Autoimmun (2015) 62:55–66. doi:10.1016/j.jaut.2015.06.006
147. Ali N, Flutter B, Sanchez Rodriguez R, Sharif-Paghaleh E, Barber LD, Lombardi G, et al. Xenogeneic graft-versus-host-disease in NOD-scid IL-2Rgammanull mice display a T-effector memory phenotype. PLoS One (2012) 7(8):e44219. doi:10.1371/journal.pone.0044219
148. Billerbeck E, Labitt RN, Vega K, Frias-Staheli N, Dorner M, Xiao JW, et al. Insufficient interleukin-12 signalling favours differentiation of human CD4(+) and CD8(+) T cells into GATA-3(+) and GATA-3(+) T-bet(+) subsets in humanized mice. Immunology (2014) 143(2):202–18. doi:10.1111/imm.12304
149. Andre MC, Erbacher A, Gille C, Schmauke V, Goecke B, Hohberger A, et al. Long-term human CD34+ stem cell-engrafted nonobese diabetic/SCID/IL-2R gamma(null) mice show impaired CD8+ T cell maintenance and a functional arrest of immature NK cells. J Immunol (2010) 185(5):2710–20. doi:10.4049/jimmunol.1000583
150. Hsieh CS, Lee HM, Lio CW. Selection of regulatory T cells in the thymus. Nat Rev Immunol (2012) 12(3):157–67. doi:10.1038/nri3155
Keywords: humanized mice, Treg function, therapy, autoimmune disease risk, tolerance
Citation: Hahn SA, Bellinghausen I, Trinschek B and Becker C (2015) Translating Treg Therapy in Humanized Mice. Front. Immunol. 6:623. doi: 10.3389/fimmu.2015.00623
Received: 21 September 2015; Accepted: 30 November 2015;
Published: 14 December 2015
Edited by:
Nurit Hollander, Tel Aviv University, IsraelReviewed by:
Urszula Krzych, Walter Reed Army Institute of Research, USAAna Claudia Zenclussen, Otto-von-Guericke University, Germany
Copyright: © 2015 Hahn, Bellinghausen, Trinschek and Becker. This is an open-access article distributed under the terms of the Creative Commons Attribution License (CC BY). The use, distribution or reproduction in other forums is permitted, provided the original author(s) or licensor are credited and that the original publication in this journal is cited, in accordance with accepted academic practice. No use, distribution or reproduction is permitted which does not comply with these terms.
*Correspondence: Susanne A. Hahn, c3VzYW5uZS5oYWhuQHVuaW1lZGl6aW4tbWFpbnouZGU=