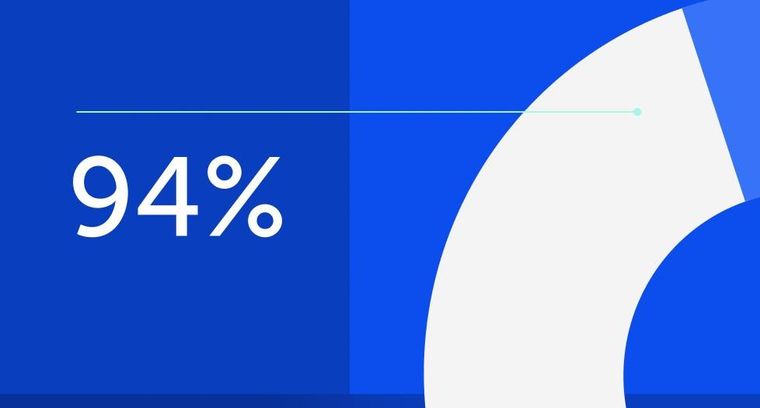
94% of researchers rate our articles as excellent or good
Learn more about the work of our research integrity team to safeguard the quality of each article we publish.
Find out more
REVIEW article
Front. Immunol., 04 February 2015
Sec. T Cell Biology
Volume 6 - 2015 | https://doi.org/10.3389/fimmu.2015.00037
This article is part of the Research TopicRecent Advances in γδ T-Cell Biology: New Ligands, New Functions, Translational PerspectiveView all 28 articles
In comparison to conventional αβT cells, γδT cells are considered as specialized T cells based on their contributions in regulating immune response. γδT cells sense early environmental signals and initiate local immune-surveillance. The development of functional subtypes of γδT cells takes place in the thymus but they also exhibit plasticity in response to the activating signals and cytokines encountered in the extrathymic region. Thymic development of Tγδ1 requires strong TCR, CD27, and Skint-1 signals. However, differentiation of IL17-producing γδT cells (Tγδ17) is independent of Skint-1 or CD27 but requires notch signaling along with IL6 and TGFβ cytokines in the presence of weak TCR signal. In response to cytokines like IL23, IL6, and IL1β, Tγδ17 outshine Th17 cells for early activation and IL17 secretion. Despite expressing similar repertoire of lineage transcriptional factors, cytokines, and chemokine receptors, Tγδ17 cells differ from Th17 in spatial and temporal fashion. There are compelling reasons to consider significant role of Tγδ17 cells in regulating inflammation and thereby disease outcome. Tγδ17 cells regulate mobilization of innate immune cells and induce keratinocytes to secrete anti-microbial peptides thus exhibiting protective functions in anti-microbial immunity. In contrast, dysregulated Tγδ17 cells inhibit Treg cells, exacerbate autoimmunity, and are also known to support carcinogenesis by enhancing angiogenesis. The mechanism associated with this dual behavior of Tγδ17 is not clear. To exploit, Tγδ17 cells for beneficial use requires comprehensive analysis of their biology. Here, we summarize the current understanding on the characteristics, development, and functions of Tγδ17 cells in various pathological scenarios.
Decades have passed since the accidental discovery of T cells expressing γ and δ chains (1), yet it is hard to define γδT cells like αβT cells. Ambiguity in understanding the functions of γδT cells is attributed to their unparalleled characteristics as compared to αβT cells. Current understanding of T cell biology has emerged extensively from studies on αβT cells; however, recent findings have underlined the crucial role of γδT cells in shaping the immune response in infections, inflammatory diseases, and cancer. They are involved in early immune response like innate cells, produce proinflammatory cytokines (IFNγ, IL17, and TNFα), and activate adaptive immune cells. The cytokines secreted by γδT cells determine their effector functions. In humans, the major cytokine produced by γδT cells is IFNγ, contributing to its role in anti-viral, anti-bacterial, and anti-tumor immunity (2–4). However, upon activation γδT cells can be skewed toward IL17, IL4, or TGFβ producing phenotype governed by the polarizing cytokines present in the surrounding milieu (5). Recent investigations in mice and human have highlighted the role of IL17-producing γδT cells (hereafter referred as Tγδ17) in bacterial infection, inflammatory disease, and cancer (6–8). They are the primary source of IL17 in early disease condition and are pivotal in progression and disease outcome (9, 10). To understand the functional significance of Tγδ17 in pathological conditions, many efforts have made in mouse models but there is scanty literature available on human Tγδ17 cells. In this review, we will discuss the recent findings of Tγδ17 differentiation, mechanisms regulating IL17 production, and their relevance in pathological conditions.
Survival of γδT cells over strong evolutionary selection pressure highlights their exclusive importance and disparate properties from conventional αβT cells. Initially, γδT cells were considered as cells of innate immunity owing to their ability to recognize conserved non-peptide antigens expressed by stressed cells. In addition to this, they recognize pathogen-associated molecular pattern (PAMP) or danger-associated molecular pattern (DAMP) through pattern recognition receptors (PRR) expressed by them (11). Like adaptive immune cells, human γδT cells undergo clonal expansion and exhibit antigen-specific memory (12). Thus, γδT cells link innate and adaptive immunity thereby enhancing the immune response against invading pathogen or danger signal posed by “self” cells. Antigen recognition by murine or human γδT cells does not require antigen presentation by major histocompatibility complex (MHC) class I or class II (13) and the crystal structure of γδTCR has revealed its close homology with immunoglobulins suggesting that antigen recognition by γδT cells is similar to antigen–antibody interaction (14). However, diversity of antigens recognized by γδT cells brands it different from B cells. The antigens exclusively recognized by γδT cells are not peptides of protein antigens rather are small mono- and pyrophosphates of linear C5 isoprenoids called as phosphoantigens (13). These prenyl pyrophospahtes are metabolites of cholesterol biosynthesis and are recognized through complementarity determining regions (CDRs) of γδT cells (15). In humans, during cholesterol biosynthesis, phosphorylated precursors such as isopentenyl pyrophosphate (IPP) and DMAPP (dimethylallyl pyrophosphate) are synthesized by mevalonate pathway (16). However, microbial pathogens use non-mevalonate pathway to produce these phosphorylated precursors (17). γδT cells respond to these natural or synthetic stimulators with varying degree. Based on this, stimulators are classified either as weak or potent stimulators. HMBPP [(E)-4-hydroxy-3-methyl-but-2-enyl pyrophosphate], a metabolite of non-mevalonate pathway of bacteria Mycobacterium tuberculosis is 104 times more potent stimulator of human γδT cells than IPP (18). The exclusive response of γδT cells to these phosphoantigens has a potential therapeutic significance and synthetic pyrophosphates can be used to harness the cytotoxic potential of γδT cells.
Murine and human γδT cells also recognize phycoerythrin (PE) – fluorescent molecule of cyanobacteria and red algae. PE is directly recognized by γδT cells but there is no sequence similarity between PE-specific murine and human γδ TCR (19). Naturally occurring primary alkyl amines activate human Vγ2Vδ2 T cells and enhance immunity against certain microbes and plant-derived antigens (20, 21). Similar to natural killer (NK) cells, human γδT cells also recognize the stress-induced MHC class I-related molecules MICA, MICB, and the UL16-binding proteins that are upregulated on malignant or stressed cells (22, 23). The stress-related molecules are ligands for NKG2D expressed by γδT cells and this engagement also enhances γδT cells’ response to non-peptide antigens (24). Human and murine γδT cells recognize lipid antigens presented by CD1 molecules, a classical ligand for NK T cell suggesting the phenomenon similar to MHC-restricted antigen recognition by αβT cells (25–27). The murine γδT cells also recognize non-classical MHC class I molecules like T10 and T22 (β2 microglobulin-associated molecules lacking peptide binding groove) (28, 29). In addition to non-protein and MHC related antigens, murine and human γδT cells also recognize small peptides such as heat shock proteins (HSPs) (30–32). However, they do not require antigen-presenting cells (APCs) and recognition of antigen is MHC unrestricted, resembling B cells (33). Thus, the broad spectrum antigen responsiveness of γδT cells helps them to mount faster immune response.
Like αβT cells, γδT cells develop in the thymus from CD4−CD8− (double negative, DN) thymocytes (34); however, they precede αβT cells in T cells ontogeny. γδ TCR rearrangements can be traced in early embryonic stages in mice as well as in humans (35, 36). This highlights their role in neonatal protection as conventional T cells are functionally impaired and APCs are immature in newborns (37). During thymic development, the decision of γδ versus αβ T cell commitment is determined by TCR signal strength or notch signaling (38). In mice, the strong TCR signaling in absence of notch signal induces γδT cells lineage commitment whereas low TCR signal strength in presence of strong notch signaling promotes αβ T cell lineage (39–41). However, notch signaling alone is insufficient to decide γδ/αβ T cell commitment. The intrinsic signals from T cell receptor complex and trans-conditioning by different subsets of thymocytes also determine thymic development of γδT cells (42). In humans, notch has opposite role in αβ versus γδT cell lineage decision, sustained notch signaling is required for the development of γδT cells (43) which is determined by differential notch receptor–ligand interaction importantly Jagged2/Notch3 signaling (44). In human, γδT cells differentiate along two pathways, a notch-independent DN pathway, generating mature DN and CD8αα+ SP (single positive) TCRγδ+ cells. In the notch-dependent DP (double positive) pathway, immature CD4+ SP, and subsequently DP TCRγδ+ cells are generated. Human postnatal thymus thus exhibits a scenario of DN, DP, and SP TCRγδ+ population, which highlights heterogeneity in human γδT cell development (45). The activated extrathymic γδT cells, in humans, express notch receptors, which regulate their effector functions. Inhibiting notch signaling in γδT cells dampened their anti-tumor cytotoxic potential (46). Thus, validates the requirement of notch signaling in both thymic development and functions of human γδT cells. The diversity of human γδ T cell repertoire at birth (majorly contributed by Vδ1+ subset of γδT cells in cord blood) is restricted in adulthood especially to Vγ9Vδ2, a circulating subset of γδT cells. The absolute numbers of Vγ9Vδ2 T cells increase from minor population at birth to more than 75% of γδT cells pool in peripheral blood (35), which constitute around 1–10% of total T cells in humans. The γδT cells exit the thymus as mature T cells and express markers that are associated with antigen-experienced T cells (47).
The other important feature of γδT cells apart from antigen recognition is their tissue tropism. In humans, the first γδT cells to arise from thymus are Vδ1+ (paired with various Vγ chains), which preferentially populate in epithelial tissue and constitute larger proportion of intraepithelial lymphocytes (IELs) (48). They rapidly and innately recognize stressed cells found to be enriched in various tumor tissues (4). The Vγ9Vδ2 is a lymphoid homing subset of γδT cells, which continually expand in response to microbial antigen in circulation and exhibit characteristics of adaptive immune system (49). These cells recognize, expand, and secrete cytokines in response to non-peptide antigens associated with microbes in circulation. In mouse, a substantial proportion of γδT cells reside as the IEL in the skin, intestine, and genitourinary tract. In response to the chemokine signals, Vγ5Vδ1+ T cells leave the fetal thymus, reside in the epidermis, and form dendritic-like network similar to Langerhans cells. These cells are called as dendritic epidermal T cells (DETCs) and constitute more than 90% of epidermal T cells (50). Vγ6+ T cells home to tongue and reproductive tract whereas Vγ7+ T cells home to intestinal tract suggesting that distinct TCR repertoire are present at different anatomical site and respond to antigens unique to their resident tissues (51–53). However, the functions of IELs are determined by the environment at the anatomical site (54) and hence specific γδ T cell subset could be used in tissue repair and generation of effective immune response at different epithelial sites.
γδT cells perform diverse effector functions determined by the TCR expressed, tissue localization, and activation status. Apart from these, MHC-independent recognition of antigens, production of IFNγ, and expression of cytotoxic granules classify γδT cells as potential cytotoxic cells (55). They can kill activated, infected, stressed, and transformed cells using various strategies such as engagement of death-inducing receptors, such as FAS and TNF-related apoptosis-inducing ligand receptors (TRAILR) and the release of cytotoxic effector molecules such as perforin and granzyme (56, 57). Human γδT cells also recognize HSP (HSP60/70) expressed on tumor cells and enhance its cytolytic activity against the tumors (31, 58). γδT cells support the maturation and activation of other lymphocytes, NK cells, and macrophages with the help of secreted chemokines (CCL3, CCL4, CXCL10) (55). Another chemokine CXC–chemokine ligand 13 (CXCL13) produced by Vγ9Vδ2 cells can regulate B cell organization within lymphoid tissues and help B cells to produce antibodies (59). Human γδT cells can also crosstalk with dendritic cells (DCs) influencing each other functions like the antigen presentation by DCs, activation, and secretion of IL12 and IFNγ by γδT cells, which result in DC maturation (11, 60). These properties of γδT cells aid in generation of the effective immune response in the appropriate condition. Not only this, activated Vγ9Vδ2 cells can take up and process the soluble antigens, opsonize target cells, and can migrate to lymph nodes through CC-chemokine receptor 7 (CCR7) where they upregulate expression of MHCs and co-stimulatory receptors CD80 and CD86 (61, 62). Activated Vγ9Vδ2 cells has also been licensed to act as APC and activate CD4 and CD8 T cells (63). Collectively, these observations highlight the multi-talented role of γδT cells, having both Th- and Tc-like properties along with acting as APC. The special trait of γδT cells is their ability to recognize phosphorylated non-protein antigens and mediate its effector function in spatial and temporal manner making them a robust cell type, which can be manipulated to develop a promising tool for novel immunotherapies against certain types of diseases. However, care should be adapted while designing such immunotherapies because these cells have capacity to secrete various cytokines under different conditions.
Unlike αβ T cells, in mice, which leave thymus as naïve cells and are primed in the peripheral compartment, γδT cells undergo subset commitment in the thymus itself. However, in humans, upon activation with different cytokines, Vγ9Vδ2 cells can be polarized toward different effector subtypes like γδ1, γδ2 (64), γδ17 (65, 66), and γδTreg (67, 68). This functional plasticity of γδT cells assists them to tackle the distinct disease conditions and play important role in the early responses to invasive pathogens. The recent findings have stated that γδT cells are major IL17 producers and have shown their involvement in early onset of immune activation (69). Similar to Th17 cells, Tγδ17 cell express RORγt as a lineage determination transcriptional factor (70). Healthy adult human peripheral blood Vγ9Vδ2 T cells distinctively express Th1 signature and 50–80% produce IFNγ but <5% produce IL17 (6). However, Tγδ17cells have been demonstrated to be involved in the pathogenesis of transplantation rejection (71), autoimmune disease (72), allergy (73), and cancer (74) in humans. The biology of Tγδ17 is so naive that it compels us to cross-examine its genesis, functions, and clinical relevance to understand its therapeutic potential.
The molecular mechanism of IL17-producing γδT cells remains an enigma. Most of the studies carried out to understand the differentiation mechanisms of Tγδ17cells are based on the murine models. γδT cells preferentially localized to barrier tissues are the initial source of IL17 and are likely to originate from the fetal thymus. These are called as the natural IL17-secreting γδT cells. γδT cells that make IL17 within 24 h fall in this category (75). γδT cells acquire IL17-secreting phenotype in secondary lymphoid tissues after antigen exposure, which is referred to as induced Tγδ17 cells (76, 77).
During development of T cells in thymus, murine γδT cells branch off at the transition of thymocytes from DN3 stage to DN4 stage (34). It is also reported that γδT cells develop from DN2 stage and specifically produce IL17 whereas IFNγ-producing γδT cells can develop from both DN2 and DN3 precursors (78) (Figure 1). This suggests that γδT cells do not develop like αβT cells and follow evolutionary ancient path of T cell development. However, the precise DN stage from which γδT cells develop is elusive (79). Fetal thymic γδ T-cell development occurs in successive waves by using the different Vγ and Vδ segments during the embryonic development (34, 80). Successful gene rearrangement of γδ T cells from early thymic precursors (CD44hi) lead to the development of naïve γδ T cell characterized by CD44lo CD27+CD62L+ phenotype. This phenotype can either leave the thymus to populate in secondary lymphoid organs or it can undergo further intrathymic differentiation that results in the development of multiple γδ T cell subtypes such as dendritic epidermal γδT cell (DETCs), Tγδ17, or NK 1.1+ γδ cell (γδNKT cells) (80, 81). Recently, it was described that when thymic lobes of mice at E14 were colonized with DN1a cells from mice at E13 and E18, respectively. It was observed that although both populations (E13 DN1a cells and E18 DN1a cells) generated similar number of γδT cells, only E13 DN1a cells generated Vγ3+ DETCs. These observations indicate that precursor lineage of DETCs may be different and needs further investigation (82). DETCs develop at embryonic day 13 (E13) to approximately E17 and readily secrete IFNγ when activated. After the development of DETCs, the next functional developmental wave consists of Tγδ17 cells. Tγδ17 cells are heterogeneous in using TCR chains that mainly include Vγ6+ and Vγ4+ but also use Vγ1+ chain. Vγ6+ cells develop by E14 to around birth and finally Vγ1 and Vγ4 cells develop E16 onward (81). The other subtypes of γδ T cells, which develop in thymus, are γδ NKT cells, which are similar to invariant TCRαβ+ NKT cells (83, 84).
Figure 1. Overview of Tγδ17 cells development. The figure illustrates the differentiation of Tγδ17 cells from T cell progenitors in the murine thymus (A–C) and from naïve γδT cells in periphery in human (D). Progenitor T cells differentiate through double negative stage 1 (DN1) to DN stage 4 (A). The decision of αβ or γδ TCR expression takes place at early T cells precursor (from DN2 or DN3 stage) as showed by dashed line. The thymocytes expressing αβ TCR develop into double-positive thymocytes, which support differentiation of functional subtypes of γδT cells called as transconditioning. DP thymocytes secrete LTβL, which support differentiation of Tγδ17. The DP αβ thymocytes then exit the thymus as mature single positive T cells (either CD4+ or CD8+ T cells) (A). The functional programing of γδT cells is determined by TCR signal and/or other related signals. TCR signal, interaction with Skint-1 from epithelial cells, downregulation of SOX13, and signaling through CD27/CD70 divert γδ thymocytes toward IFNγ-producing phenotype (Tγδ1), which migrate to periphery (B). Conversely, signaling through Notch receptor maintain Sox13 levels with increase in Hes1 and RORγt expression induce γδ thymocytes to produce IL17. Progression of γδ thymocytes to Tγδ17 cells is independent of signaling through Skint-1 and/or CD27 but require inputs from IL6 and TGFβ. The natural Tγδ17 cells developed in thymus migrate to tissue or periphery (C). In human, naïve γδT cells, which exit thymus, can also differentiate into Tγδ17 cells in presence of TCR signal and cytokines such as IL6, IL1β, IL23, and TGFβ (D).
There are different thymic signaling processes, which determine functional phenotype of γδT cells in thymus before migration to periphery and contribute to the balance between IFNγ committed versus IL17-commited subtypes (85). This biasness toward IL17 or IFNγ depends on the antigen experience in thymus. The γδ T cells that have encountered the cognate antigen interaction in thymus, gain the potential to differentiate into the IFNγ-producing functional phenotype while antigen naïve γδ T cells develop into IL17-producing γδT cells (86). This skewedness also reflects in their distribution outside the thymus. Most of Tγδ17 cells reside in lymph nodes whereas IFNγ-producing γδT cells are mainly found in the spleen and the mechanism for this distribution is not clear (86). Similar distribution is also found in αβT cells and it seems to be logical as the lymph nodes serve as the site of initial exposure to foreign antigens and propagate the wave of inflammation, thus are suited for the earliest source of the IL17 secretion (87).
Besides the γδ TCR signaling (86), expression of tumor necrosis factor receptor family member, CD27, determines the IL17 versus IFNγ production by γδT cells (88). CD27+ γδT cells differentiate into IFNγ producing cells whereas IL17 production was restricted to CD27− T cells (89) (Figure 1). Thus thymic “imprinting” of the γδT cells as CD27+ or CD27− regulates effector functions of γδT cells and is preserved in the periphery (89). CD27 is not only associated with IFNγ production but also aids γδT cells to interact with its ligand CD70 expressed on DCs, thymic epithelial cells, and double-positive thymocytes thus acting as a costimulatory receptor (89). Therefore, CD27 conveys an intrathymic message that licenses the CD27+ γδ T cells for the production of IFNγ (47). Another signaling pathway that influences the differentiation of Tγδ17 is the signaling through lymphotoxin-β receptor (LTβR), a member of the tumor necrosis factor receptor family (90). Signaling through LTβR leads to the activation of the alternative nuclear factor (NF)-κB pathway via RelB. Ligands for LTβR regulating this developmental process are produced by CD4+CD8+ thymocytes (91). The homeostasis of this functional phenotypic differentiation, influenced by other thymic progenitors is known as transconditioning (91), which highlights coordination between different signaling pathways in thymus that occur in physically separate thymic niche (92). LTβR signaling pathway controls Tγδ17 development by regulating transcription factors RORγt and RORα4, required for IL17 expression in γδ thymocytes (93). The role of LTβR signaling, however, remains controversial as LTβR is present downstream to CD27 signaling, which is associated with the IFN-γ production (89).
The maturation of Tγδ17 cells from its precursors requires TCR signaling as mice with reduced ZAP70 show decreased number of Tγδ17 cells (94). However, TCR signaling alone is not sufficient as it also requires other signals (95). An src family kinase, Blk (B lymphoid kinase), is required for Tγδ17 cells development in thymus as Blk-deficient mice was reported to have less number of IL17-producing γδ T cells (96). Similarly, high-mobility group (HMG) box transcription factors, SOX4 and SOX13 are positive regulators of Tγδ17 development (95, 97). These transcription factors expressed in immature T cells (98) highlight that the development of Tγδ17 is from early precursors (DN2) (78, 95). Other thymic determinant, which is responsible for the functional dichotomy in Tγδ17 and Tγδ1, is Skint-1, a thymic epithelial cell determinant. The interaction between Skint-1+ cells and γδ thymocytes (Vγ5+Vδ1+) induce an Egr3-mediated pathway, leading to differentiation toward IFNγ-producing γδ T cells. Further, it suppresses Sox13 and an RORγt transcription factor-associated Tγδ17 cells lineage differentiation suggesting that the functions of the earliest T cells are substantially preprogramed in the thymus (99). Notch signaling is known to be involved in thymic determination and development of Tγδ17 cells. Hes1, one of the basic helix–loop–helix (bHLH) proteins induced by Notch signaling is critical for the IL17 expression by γδ T cells and its thymic development (100–102). Further, the specific expression of Hes1 in CD25+ and CD27− γδ T cells and decreased levels of Tγδ17 in Hes1-deficient mice highlights the critical role of Notch–Hes1 pathway in Tγδ17 development in thymus as well as in periphery (101). The thymic development of Tγδ17 is independent of STAT3 but partly dependent on RORγt (101) and most peripheral IL17-producing γδ cells express RORγt and respond rapidly to IL23 (103).
Developmental process of Tγδ17 also requires signaling through different cytokines. TGFβ signaling is necessary for Tγδ17 development (104). It has been shown that in absence of TGFβ1 or Smad3 (a component of the TGFβ signaling), the number of Tγδ17 thymocytes reduced drastically relative to that of wild-type mice (104). As compared to TGFβ, requirement of IL6 for Tγδ17 development is not well understood as there are contrasting reports on its role (72, 105). It is also reported that IL6 does not act directly on uncommitted γδ thymocytes but instead it acts indirectly by regulating the expression of Delta-like ligand 4, a ligand for notch receptor, expressed by thymic epithelial cells that promote the differentiation of Tγδ17 (101, 106). Moreover, IL23 and IL1 produced by DCs are crucial for IL17 production by γδT cells. IL23−/− and IL23R−/− mice showed the significant reduction in Tγδ17 cells after L. monocytogenes infection supporting earlier observation (107–110).
Thymic development of human Tγδ17 cells is poorly investigated. Around 80% circulating human Vγ9Vδ2 T cells are IFN-γ producers and express CD27 whereas CD27 negative cells are IL17-producing γδ T cells are <5% (65). Interaction of CD70 with CD27 promotes the expansion of Th1-biased Vγ9Vδ2 T cells in periphery (111). However, such role in their thymic development is unknown. Human Vγ9Vδ2 T cells can be polarized to Tγδ17 cells in periphery upon IPP activation and in the presence of cytokines like TGFβ, IL1β, IL6, and IL23, followed by a week of culture in differentiation medium supplemented with IL2 can induce IL17 in these cells (65, 66). In humans, there are contrasting reports on role of IL6 and IL23 in differentiation of Tγδ17. It has been shown that IL6 is required for differentiation of neonatal Tγδ17, and IL23 is required for the generation of adult IL17-producing γδT cells (65). In another study, it is reported that in the presence of TCR signaling, IL23 promotes the induction of IL17 in neonatal (but not adult) γδT cells (112). However, it appears that IL23 induces γδT cells to coproduce IL17 and IFNγ in adults but support development of Tγδ17 cells in neonates. In addition to the above-mentioned cytokines, IL7 selectively promotes the mouse and human IL17-producing γδT cells. IL7 activates STAT3 preferentially in γδT cells competent to produce IL17 (113). However, the increased IL17 production by γδT cells upon TCR stimulation in presence of IL7 is observed only in case of cord blood cells but not with peripheral lymphocytes. Thus, it is important to note that the antigen naïve γδT cells only can be reprogramed in vitro toward Tγδ17 phenotype (66, 113).
The kinetic study of IL17 production by γδT cells has shown that murine γδT cells secrete IL17 within few hours after stimulation (70). This phenomenon can be reasoned by the thymic development of murine Tγδ17 cells and constitutive presence of transcriptional regulators for IL17 production. However, human γδT cells in thymus are functionally immature and can attain their functional differentiation in periphery in presence of cytokines (114). This supports the kinetics of IL17 production by human γδT cells that mRNA expression of IL17 and RORγt peaks by day 3–6 and decrease by day 9 onward, after stimulation. The expression of cytokine receptors (IL1βR, IL6R, TGFβR, and IL23R) on Vγ9Vδ2 T cells peaks on day 3 and decrease by day 6 (66). Thus, coordinated combination of TCR and cytokine stimulation could be necessary for the sustained secretion of IL17 by γδT cells, which highlights the difference in kinetics of IL17 secretion by murine and human Tγδ17 cells. This underscores that human γδ T cells can be “reprogramed” in the periphery into different functional lineages.
Upon antigenic challenge, T cells differentiate to memory phenotype; either central memory (TCM) or effector memory (TEM) (115). Human Tγδ17 cells present in non-lymphoid environment belong to CD27− CD45RA± effector (74) or terminally differentiated (TEMRA) (66) memory phenotype. Similarly, murine Tγδ17 cells also show effector memory phenotype with CD44high, CD45RBlow, and CD62Llow (116). Thus, Tγδ17 cells differentiated either in thymus or in periphery, belong to memory phenotype, and licensed to patrol the blood, lymphoid organs, and peripheral tissues.
Tγδ17 cells can rapidly produce IL17 upon Toll-like receptors (TLR) or cytokine stimulation alone even in absence of antigen presentation. The general proinflammatory functions of IL17 [reviewed in Ref. (117, 118)] could be associated with γδT cells as they are major producers of IL17. Studies carried out in various infection models showed that Tγδ17 cells are protective against infection. During mycobacterial infection, IL17 produced by Vγ4+ and Vγ6+ cells induce pulmonary granuloma formation by recruitment of granulocytes and monocytes. The IL17 participates in maturation of granuloma by promoting tight cell to cell binding via ICAM1 and LFA1 induction (119). Mycobacteria-infected DCs secrete IL23, which regulate IL17 production by γδT cells emphasizing that the early activation of Tγδ17 cells is important for initiating inflammation and recruiting innate immune cells to the site of infection thereby enhancing bacterial clearance from host (120, 121). Tγδ17 cells also support cell-mediated immunity by inducing Th1cells against pulmonary mycobacterial infection (122).
In Escherichia coli infection model also, γδT cells were reported to be the major producers of IL17, which enhanced neutrophil infiltration to the peritoneum. The infiltration of cells diminished after antibody depletion of resident Vδ1+ subtype of γδT cells highlighting its involvement in IL17 secretion in response to IL23 (9). Thus, IL23 and Tγδ17 cells play a dominant role as first line of defense in infection before CD4 T cell activation. In case of L. monocytogenes infection, a large number of γδ T cells accumulate in the lymph organs shortly after infection and begin to produce IL17A, signifying the role of Tγδ17 cells in the Listeria infection (123). IL17 was also shown to promote proliferation of CD8+ cytotoxic T lymphocytes by enhancing DC cross-presentation in vitro. DCs stimulated with IL17 showed upregulation of MHC-I molecule H2Kb and enhanced secretion of cytokines (IL12, IL6, and IL1β). CD8α+ DCs from Il17a−/− mice also produced less IL12 and are less potent in activating naive CD8+ T cells (123). This indicate that Tγδ17 cells not only induce innate response but also critical for optimal adaptive cytotoxic response against intracellular bacterial infection. The alliance of IL23 and Tγδ17 is also demonstrated to have a protective role during infections such as Klebsiella pneumonia (124), Citrobacter rodentium (125, 126), Salmonella enterica (127, 128), and Toxoplasma gondii (129). The Tγδ17 cells also play a vital role in clearing fungal infections. The rapid production of IL17A was reported in the lungs at a very early stage after intravenous infection with C. albicans. Lung resident γδ T cells were the major source of early IL17A production regulated by IL23 and TLR2/MyD88-dependent pathway (130). Presence of Tγδ17 cells were also reported in the lungs of neutropenic mice during C. neoformans infection. These Tγδ17 cells played an important role in the chemotaxis of leukocytes and induction of protective immune response (131). Tγδ17 cells thus orchestrate the protective immunity by acting at the early onset in infection models (108).
Relatively few studies have evaluated the role of Tγδ17 cells in human microbial immunity. In patients with tuberculosis (TB), elevated levels of Tγδ17 cells were found in peripheral blood and were major producers of IL17 (6). As a protective role, in response to bacterial antigens, IL17-producing Vγ9Vδ2 T cells induce neutrophil migration through secretion of CXCL8 and promote their phagocytic activity (66). Tγδ17 cells also induce epithelial cells to secrete anti-microbial peptides like β-defensins in response to bacterial antigens (66). This signifies the modulatory effects of Tγδ17 cells on keratinocytes and other immune cells in anti-microbial defense. In children with bacterial meningitis, the population of IL17+ Vγ9Vδ2 T cells significantly increase in peripheral blood and at the site of infection (cerebrospinal fluid). The reversal of this pattern after successful anti-bacterial therapy clearly suggests the anti-microbial role of Tγδ17 cells (66). Collectively, these studies provide new insight into the functions of γδ T cells as the first line of host defense against bacterial and fungal infection in human and may pave a path in designing newer treatment modalities.
γδT cells express various chemokine receptors, cytokine receptors, and PRRs, which regulate IL17 production. TLRs are the well-studied PRRs expressed by DCs, macrophages, and γδT cells. The unique microbial molecules called as PAMP are recognized by TLRs, which orchestrate the anti-microbial response in γδT cells (11). In malarial infection, MyD88 deficiency results in severe impairment of IL17A producing γδT cells levels, but not IFNγ producing γδT cells highlighting differential control by innate signaling through TLRs in infections (132). Murine Tγδ17 cells specifically express TLR1 and TLR2 but not TLR4. High number of Tγδ17 cells were induced upon in vivo stimulation with Pam3CSK4 (ligand for TLR2) but not with LPS (TLR4 ligand) or CpG (TLR9 ligand) (70). Interestingly, it has been shown that TLR4 indirectly controls IL17 generation by γδT cells through IL23 secreted by TLR4 expressing macrophages in response to HMG Box 1 (HMGB1, a damage-associated protein and TLR4 ligand) (133). Moreover, Tγδ17 cells promote experimental intraocular neovascularization (134) as well as early acute allograft rejection (135) in response to HMGB1. Signaling through TLR2 is indispensable for Tγδ17 in anti-microbial functions. Absence of TLR2 or MyD88 in cutaneous Staphylococcus aureus infection, or in Candida albicans infection, caused an impaired IL17 production and poor microbial clearance in the skin infiltrated with Vγ5+ γδT cells (130, 136). Tγδ17 cells also express DC-associated C-type lectin 1 (dectin 1) and intraperitoneal injection of curdlan (dictin 1 ligand), induced IL17 production by γδT cells (70). In imiquimod (IMQ)-induced psoriasis-like model, dermal γδT cells spontaneously secreted a large amount of IL17 in IMQ-treated skin cells. Thus, it appears that TLR7/8 (receptor of IMQ) may regulate the IL17 production by γδT cells. It is important to note that the modulatory effects of TLRs on γδT cells as showed in in vivo murine models are mediated through IL23 and/or IL1β cytokines. The direct stimulation of CD27− γδT cells by TLR ligands (LPS or PAM) show no effect on IL17 production (132). This suggests that TLR signaling indirectly modulates Tγδ17 function.
The receptor profile of Tγδ17 cells is similar to Th17 cells. In mice, the majority of IL17-producing CD4 cells belong to CCR6+ compartment compared to CCR6− (137). Sorted CCR6+ γδT cells showed increased mRNA expression of IL17, IL22, IL23R, Rorγt, and aryl hydrocarbon receptor (AhR) compared to CCR6− γδT cells (70, 138). This suggests that CCR6 can be a phenotypic surface marker of Tγδ17 cells. Besides CCR6, Tγδ17 cells express various chemokine receptors including CCR1, CCR2, CCR4, CCR5, CCR7, CCR9, CXCR1, CXCR3, CXCR4, CXCR5, and CXCR6 (7). The early onset recruitment of Tγδ17 to the site of inflammation is determined by the type of chemokine receptor on them. Tγδ17 cells expressing CCR6 and CCR9 show selective migration toward allergic inflamed tissue in response to CCL25 (ligand for CCR9). α4β7 integrin expression is indispensable for this migration and transendothelial crossing of Tγδ17 cells. (139). Since migration through CCL2/CCR2 axis is determinant for total γδT cells, CCL25/CCR9-mediated migration seems to be specific for Tγδ17 subtype (140, 141).
In humans, Tγδ17 cells express CCR6 but not CXCR3, CXCR5, CCR3, CCR4, or CCR5. However, they express granzyme B, FASL, and TRAIL but not perforin (66). The lack of granzyme B and perforin coexpression may be responsible for absence of cytolytic activity of Tγδ17 cells. On the contrary, it has been shown that the human colorectal tumor-infiltrating Tγδ17 cells do not express FASL or TRAIL but express CD161 and CCR6 (74). The inconsistency in expression of cytolytic markers and their relevance on Tγδ17 cells needs to be understood in detail. The AhR is indispensable for Tγδ17 cells as it promotes differentiation of naïve Vγ9Vδ2 T cells toward Tγδ17 phenotype (66).
In mouse model, it has been shown that Ahr−/− Tγδ17 cells express IL17 but fail to produce IL22 (70). Moreover, in mouse model of Bacillus subtilis induced pneumonitis, deficiency of Ahr resulted into low IL22 production but IL17 levels were maintained (142). Thus, although Ahr promotes IL17, it is indispensable for IL22 production by Tγδ17 cells.
Th17 cells and Tγδ17cells are essential in disease progression and are pathogenic in autoimmune disease. Dysregulated levels and sustained secretion of proinflammatory cytokines by γδ and/or CD4 T cells have devastating effects on autoimmune disease progression. In a collagen-induced arthritis (CIA) model (resembling human rheumatoid arthritis), IL17-producing Vγ4/Vδ4+ T cells selectively increase in joints and lymph nodes. Depletion of γδ T cells by anti Vγ4 antibody, markedly reduced the disease severity score revealing its pathogenic nature (143). Interestingly, both Th17 and Tγδ17 are present in the joints but Th17 cells localize proximal to the bone, which facilitates its interaction with osteoclast. Selective depletion of Th17 cells abrogated the bone resorption suggesting that Th17 but not Tγδ17 cells are responsible for bone destruction. Thus, Tγδ17 cells may be responsible for enhancing joint inflammation and exacerbate CIA (144). In contrast, absence of Tγδ17 was reported in patients with rheumatoid arthritis and in murine model of autoimmune arthritis (SKG model) (145). The SKG mouse model has defects in the differentiation of Tγδ17 cells (94), which might result into low Tγδ17 cells in the inflamed joints. Thus, the role of Tγδ17 cells in autoimmune arthritis need to be evaluated comprehensively.
Tγδ17 also enhanced experimental autoimmune encephalo- myelitis (EAE) (mouse model for human multiple sclerosis). Upon immunization of mice with myelin oligodendrocyte glycoprotein (MOG) peptide in complete Freund’s adjuvant (CFA), Vγ4+CCR6+IL23+ γδT cells accumulate in the central nervous system (CNS), which expand by 20-fold in absolute number during development of clinical signs of the disease (72). In contrast, IFNγ-producing γδT cells are low in CNS and marginally increase during course of EAE (103). The mechanism behind aggravation of EAE could be attributed to restraining the development of Foxp3+ regulatory T cells (Tregs) functions by Tγδ17 cells. Supernatants from IL23-activated γδT cells inhibited the TGFβ driven conversion of naive Foxp3− αβ T cells into Foxp3 expressing T cells and also reversed the suppressive effect of Treg cells (72). Similar function of Tγδ17 was reported in cardiac transplantation in mice. IL17, majorly produced by γδT cells, accelerates acute rejection of transplanted heart but IL17 deficiency enhanced Treg expansion and prolonged allograft survival (71). In ischemic brain injury, Tγδ17 were reported to be present at the infract areas (146). Tγδ17 rather than Th17 was the major source of IL17 whereas IFNγ was majorly produced by Th1 cells. In mice, genetically deficient for IL17 or IL23, the infract areas were reduced suggesting a role of Tγδ17 as a key contributor of neuroinflammation (146). Overall, this suggests that in chronic inflammatory condition, innate cytokines IL23 and IL1β promote infiltration and generation of IL17-producing γδT cells, which aggravate the disease.
Experimental silicosis is a useful model for depicting chronic lung inflammation, tissue damage, and fibrosis. Tγδ17 along with Th17 accumulated in the lung in response to IL23 expressing macrophages by third day after silica treatment but interestingly did not induce lung fibrosis (73). On the contrary, in allergic lung inflammation, Tγδ17 cells are known to be protective (147, 148). Functional blockage of both IL17 and γδT cells impaired the resolution of airway lung inflammation (148). It is claimed that this protective role is mediated by prostaglandins (PGs), which are abundant at the site of inflammation. PGI2 analog iloprost enhanced IL17 production by γδT cells in the thymus, spleen, and lungs, reducing airway inflammation (147). This highlights the role of PGI2 analogs that can be exploited in the development of immune response in immunotherapeutic approaches. Age-related macular degeneration (AMD) is another chronic inflammation associated disease, characterized by choroidal neovascularization (CNV). In an experimental model, Tγδ17 cells along with Thy-1+ ILCs (innate lymphoid cells) infiltrate the eye after laser treatment and promote neovascularization. This recruitment is in response to IL1β but not IL23 produced by macrophages (134).
The unmatched characteristics of human γδT cells to have MHC unrestricted tumor directed cytotoxicity, release of copious amounts of IFNγ, and recognition of cancer cells through variety of mechanisms render them as potential candidate for cancer immunotherapy (4, 149). Upon activation, γδT cells show cytotoxicity against myeloma (150), lymphoma (151), leukemia (152, 153), and other epithelial carcinomas (57, 154, 155) in vitro. Several clinical trials have been launched using γδT cells based therapies in cancer patients. The hallmark characteristic of γδT cells to be used for therapy is their ability to infiltrate tumors (156). In vivo activation by phosphoantigens or adaptive transfer of preactivated autologous γδT cells have proved successful in cancer treatment (157). However, the role of Tγδ17 cells as anticancer effector cells is not well defined.
In a chemotherapeutic approach, Tγδ17 cells are reported to play decisive role in several transplantable tumor models (EG7 thymoma, MCA205 sarcoma, CT26 colon cancer, and TS/A mammary carcinomas). Tγδ17 (Vγ4+/Vγ6+) cells were shown to invade the tumor bed early in response after drug treatment. This was followed by infiltration and induction of IFNγ-producing CD8 (Tc1) cells to the tumor bed. This infiltration of Tγδ17 and Tc1 cells was correlated and associated with tumor regression post radio or chemotherapy (158). Thus, IL17-producing Vγ4+/Vγ6+ cells are critical for the induction of Tc1 response in tumor tissue in response to drug treatment or radiation. Another study in bladder cancer supports the helper function of Tγδ17 cells in cancer treatment. Tγδ17 cells induce neutrophil infiltration to the tumor site and show anti-tumor effect upon Mycobacterium bovis BCG treatment (159).
In contrast to anti-tumor role of Tγδ17 cells, they also promote tumor development. With the notion that IL17 is a proangiogenic cytokine (160), Tγδ17 cells promote angiogenesis in tumor model. In IL17−/− tumor bearing mice, the blood vessel density was markedly decreased compared to wild type. In addition, IL17 induced the expression of Ang-2 (angiopoietin) and VEGF (vascular endothelial growth factor) in tumor cells (8). In ovarian cancer model, it has been reported that CD27− Vγ6+ cells produced higher IL17 and induce VEGF and Ang-2 in peritoneal exudates of tumor bearing mice after 6 weeks of post-tumor inoculation (161). Additionally, Tγδ17 cells induce mobilization of protumor small peritoneal macrophages (SPM) to the tumor bed, which express IL17-dependent proangiogenic profile (Il1b, Il6, vegfa, tgfb, mif, cxcl1, cxcl8, and tie2). SPMs also enhance ovarian cancer growth by stimulating tumor cell proliferation (161). In hepatocellular carcinoma mouse model, it was reported that IL17, majorly produced by Vγ4+γδT cells, induced CXCL5 production by tumor cells, which enhance migration of MDSCs (myeloid-derived suppressor cells) expressing CXCR2 to the tumor site. In addition, IL17 also enhanced suppressive functions of MDSCs by inhibition of T cells proliferation and cytokine (IFNγ and TNFα) production (162). In return, MDSCs induced γδT cells to produce IL17 through IL23 and IL1β secretion forming positive feedback loop for Tγδ17 activation (162). Thus, Tγδ17 cells interact with myeloid cells and counteract tumor immune-surveillance.
In human colorectal cancer, IL8 and GM-CSF secreted by Tγδ17 promote migration of MDSCs while IL17 and GM-CSF enhanced their proliferation. Tγδ17 cells also support survival of MDSCs through IL17, IL8, and TNFα (74). Thus, it is possible to speculate that Tγδ17 cells might be responsible for gradual shift from initial inflammatory to immunosuppressive tumor environment in advanced stage cancer (163). In human colorectal carcinoma, Tγδ17 cells were positively correlated with advancing tumor stages as well as with clinicopathological features including tumor size, tumor invasion, lymphatic and vascular invasion, lymph node metastasis, and serum CEA (Carcinoembryonic antigen) levels suggesting their pathogenic role (74).
Collectively, these findings highlight the apparently opposite roles of Tγδ17 cells in cancer immunity. It seems that during tumor development, inflammatory environment (IL1β and IL23) modulate the cytokine profile of γδT cells from primary IFNγ toward proinflammatory IL17, which support tumor progression.
Despite the small percentage in total T cell population, γδT cells have emerged as an important modulator of early immune responses. The development of functional subtypes of γδT cells require polarizing cues including molecular and cellular interaction and combination of multiple cytokines and chemokine receptors that regulate their distribution. This suggests that the functional determination of γδT cell subtypes is dictated by the local environment (thymus or peripheral blood or the inflamed tissue) in which they are present. Tγδ17 is a special γδT cell subset, distinctly present at early immune response in the tissue and can modulate the functions of other immune and epithelial cells but their relevance in disease outcome remains controversial. In response to microbial antigens, Tγδ17 cells promote infiltration of neutrophils and macrophages and induce production of anti-microbial peptides resulting in clearance of microbial load. Such protective behavior of Tγδ17 cells in infections can be exploited to develop newer approaches to tackle the microbial pathology (Figure 2).
Figure 2. Functions of Tγδ17 cells in pathological conditions. (A) Tγδ17 cells promote infiltration of neutrophils and monocytes/macrophages to the site of inflammation through chemokines. (B) IL17 secreted by Tγδ17 cells induces keratinocytes to produce anti-microbial peptides such as β defensins and protect host in infections. (C) Dysregulated Tγδ17 cells in autoimmune diseases inhibit Treg expansion and its ability to suppress autoreactive cell, thereby exacerbating the disease. (D) The inflammatory condition in arthritis is worsened by IL17, which foster osteoclast formation through induction of RANKL. Tγδ17 cells are involved in bone resorption and enhance joint inflammation. (E) Human Tγδ17 cells support MDSC migration, survival, and promote their suppressive functions through IL17, GMCSF, and IL8. MDSCs also form feedback loop and promote Tγδ17 differentiation through IL23 and IL1β. (F) Tγδ17 cells secrete IL17 and induce tumorigenesis by their proangiogenic activity. (G) Murine Tγδ17 cells recruit small peritoneal macrophages to the tumor bed, which induce angiogenesis.
The opposite side of Tγδ17 functions has revealed its detrimental role in enhancing inflammation in autoimmunity and cancer (Figure 2). The mechanism, which regulates such dual personality of Tγδ17 cells is unknown. It appears that the obvious common role executed by these cells is enhancement of inflammation but due to functional heterogeneity and their complex interdependency on other cells (innate and adaptive); the emerging scenario of their biology is far from complete. This provokes us to consider contextual behavior of Tγδ17 cells in disease pathology. Current progress in understanding the significance of Tγδ17 cells in inflammatory diseases has revealed their novel but debilitating functions such as suppression of Tregs in autoimmunity, induction of angiogenesis, and recruitment and activation of MDSCs in various malignancies. Thus, in inflammatory disorders, Tγδ17 cells can be targeted using various immunotherapeutic approaches. However, need of hour is to expand the understandings of Tγδ17 in humans and develop a protocol for their propagation and activation. The future therapies will rely on regulating the key transcription factor RORγt by designing suitable antagonists that will help in fine tuning Tγδ17 differentiation and eventually their function in chronic inflammation and infection.
The authors declare that the research was conducted in the absence of any commercial or financial relationships that could be construed as a potential conflict of interest.
Department of Atomic Energy-Tata Memorial Centre (DAE-TMC), Council for Scientific and Industrial research (CSIR), and University Grants Commission (UGC) for providing the fellowships to Rushikesh S. Patil, Asif A. Dar, and Sajad A. Bhat, respectively. This work is supported by Department of Biotechnology Centre of Excellence grant, Govt. of India (DBT-COE) to Shubhada V. Chiplunkar (Grant No: BT/01/CEIB/09/V/06).
1. Saito H, Kranz DM, Takagaki Y, Hayday AC, Eisen HN, Tonegawa S. Complete primary structure of a heterodimeric T-cell receptor deduced from cDNA sequences. Nature (1984) 309(5971):757–62. doi:10.1038/309757a0
Pubmed Abstract | Pubmed Full Text | CrossRef Full Text | Google Scholar
2. Qin G, Liu Y, Zheng J, Xiang Z, Ng IH, Malik Peiris JS, et al. Phenotypic and functional characterization of human gammadelta T-cell subsets in response to influenza A viruses. J Infect Dis (2012) 205(11):1646–53. doi:10.1093/infdis/jis253
Pubmed Abstract | Pubmed Full Text | CrossRef Full Text | Google Scholar
3. Zheng J, Liu Y, Lau YL, Tu W. Gammadelta-T cells: an unpolished sword in human anti-infection immunity. Cell Mol Immunol (2013) 10(1):50–7. doi:10.1038/cmi.2012.43
Pubmed Abstract | Pubmed Full Text | CrossRef Full Text | Google Scholar
4. Hannani D, Ma Y, Yamazaki T, Dechanet-Merville J, Kroemer G, Zitvogel L. Harnessing gammadelta T cells in anticancer immunotherapy. Trends Immunol (2012) 33(5):199–206. doi:10.1016/j.it.2012.01.006
5. Caccamo N, Todaro M, Sireci G, Meraviglia S, Stassi G, Dieli F. Mechanisms underlying lineage commitment and plasticity of human gammadelta T cells. Cell Mol Immunol (2013) 10(1):30–4. doi:10.1038/cmi.2012.42
Pubmed Abstract | Pubmed Full Text | CrossRef Full Text | Google Scholar
6. Peng MY, Wang ZH, Yao CY, Jiang LN, Jin QL, Wang J, et al. Interleukin 17-producing gamma delta T cells increased in patients with active pulmonary tuberculosis. Cell Mol Immunol (2008) 5(3):203–8. doi:10.1038/cmi.2008.25
Pubmed Abstract | Pubmed Full Text | CrossRef Full Text | Google Scholar
7. Cai Y, Shen X, Ding C, Qi C, Li K, Li X, et al. Pivotal role of dermal IL-17-producing gammadelta T cells in skin inflammation. Immunity (2011) 35(4):596–610. doi:10.1016/j.immuni.2011.08.001
Pubmed Abstract | Pubmed Full Text | CrossRef Full Text | Google Scholar
8. Wakita D, Sumida K, Iwakura Y, Nishikawa H, Ohkuri T, Chamoto K, et al. Tumor-infiltrating IL-17-producing gammadelta T cells support the progression of tumor by promoting angiogenesis. Eur J Immunol (2010) 40(7):1927–37. doi:10.1002/eji.200940157
Pubmed Abstract | Pubmed Full Text | CrossRef Full Text | Google Scholar
9. Shibata K, Yamada H, Hara H, Kishihara K, Yoshikai Y. Resident Vdelta1+ gammadelta T cells control early infiltration of neutrophils after Escherichia coli infection via IL-17 production. J Immunol (2007) 178(7):4466–72. doi:10.4049/jimmunol.178.7.4466
Pubmed Abstract | Pubmed Full Text | CrossRef Full Text | Google Scholar
10. Kuang DM, Zhao Q, Wu Y, Peng C, Wang J, Xu Z, et al. Peritumoral neutrophils link inflammatory response to disease progression by fostering angiogenesis in hepatocellular carcinoma. J Hepatol (2011) 54(5):948–55. doi:10.1016/j.jhep.2010.08.041
Pubmed Abstract | Pubmed Full Text | CrossRef Full Text | Google Scholar
11. Dar AA, Patil RS, Chiplunkar SV. Insights into the relationship between toll like receptors and gamma delta T cell responses. Front Immunol (2014) 5:366. doi:10.3389/fimmu.2014.00366
Pubmed Abstract | Pubmed Full Text | CrossRef Full Text | Google Scholar
12. Morita CT, Jin C, Sarikonda G, Wang H. Nonpeptide antigens, presentation mechanisms, and immunological memory of human Vgamma2Vdelta2 T cells: discriminating friend from foe through the recognition of prenyl pyrophosphate antigens. Immunol Rev (2007) 215:59–76. doi:10.1111/j.1600-065X.2006.00479.x
Pubmed Abstract | Pubmed Full Text | CrossRef Full Text | Google Scholar
13. Born WK, Kemal Aydintug M, O’Brien RL. Diversity of gammadelta T-cell antigens. Cell Mol Immunol (2013) 10(1):13–20. doi:10.1038/cmi.2012.45
14. Li H, Lebedeva MI, Llera AS, Fields BA, Brenner MB, Mariuzza RA. Structure of the Vdelta domain of a human gammadelta T-cell antigen receptor. Nature (1998) 391(6666):502–6. doi:10.1038/35172
Pubmed Abstract | Pubmed Full Text | CrossRef Full Text | Google Scholar
15. Wang H, Fang Z, Morita CT. Vgamma2Vdelta2 T cell receptor recognition of prenyl pyrophosphates is dependent on all CDRs. J Immunol (2010) 184(11):6209–22. doi:10.4049/jimmunol.1000231
Pubmed Abstract | Pubmed Full Text | CrossRef Full Text | Google Scholar
16. Buhaescu I, Izzedine H. Mevalonate pathway: a review of clinical and therapeutical implications. Clin Biochem (2007) 40(9–10):575–84. doi:10.1016/j.clinbiochem.2007.03.016
Pubmed Abstract | Pubmed Full Text | CrossRef Full Text | Google Scholar
17. Hunter WN. The non-mevalonate pathway of isoprenoid precursor biosynthesis. J Biol Chem (2007) 282(30):21573–7. doi:10.1074/jbc.R700005200
Pubmed Abstract | Pubmed Full Text | CrossRef Full Text | Google Scholar
18. Hintz M, Reichenberg A, Altincicek B, Bahr U, Gschwind RM, Kollas AK, et al. Identification of (E)-4-hydroxy-3-methyl-but-2-enyl pyrophosphate as a major activator for human gammadelta T cells in Escherichia coli. FEBS Lett (2001) 509(2):317–22. doi:10.1016/S0014-5793(01)03191-X
Pubmed Abstract | Pubmed Full Text | CrossRef Full Text | Google Scholar
19. Zeng X, Wei YL, Huang J, Newell EW, Yu H, Kidd BA, et al. gammadelta T cells recognize a microbial encoded B cell antigen to initiate a rapid antigen-specific interleukin-17 response. Immunity (2012) 37(3):524–34. doi:10.1016/j.immuni.2012.06.011
Pubmed Abstract | Pubmed Full Text | CrossRef Full Text | Google Scholar
20. Bukowski JF, Morita CT, Brenner MB. Human gamma delta T cells recognize alkylamines derived from microbes, edible plants, and tea: implications for innate immunity. Immunity (1999) 11(1):57–65. doi:10.1016/S1074-7613(00)80081-3
Pubmed Abstract | Pubmed Full Text | CrossRef Full Text | Google Scholar
21. Shanoj N, Banerjee G, Shinkar VP, Chiplunkar SV. Gamma delta T cell stimulatory activity of tea and Indian herb extracts. Int J Tea Sci (2013) 9(2/3):26–35.
22. Groh V, Steinle A, Bauer S, Spies T. Recognition of stress-induced MHC molecules by intestinal epithelial gammadelta T cells. Science (1998) 279(5357):1737–40. doi:10.1126/science.279.5357.1737
Pubmed Abstract | Pubmed Full Text | CrossRef Full Text | Google Scholar
23. Groh V, Rhinehart R, Secrist H, Bauer S, Grabstein KH, Spies T. Broad tumor-associated expression and recognition by tumor-derived gamma delta T cells of MICA and MICB. Proc Natl Acad Sci U S A (1999) 96(12):6879–84. doi:10.1073/pnas.96.12.6879
Pubmed Abstract | Pubmed Full Text | CrossRef Full Text | Google Scholar
24. Das H, Groh V, Kuijl C, Sugita M, Morita CT, Spies T, et al. MICA engagement by human Vgamma2Vdelta2 T cells enhances their antigen-dependent effector function. Immunity (2001) 15(1):83–93. doi:10.1016/S1074-7613(01)00168-6
Pubmed Abstract | Pubmed Full Text | CrossRef Full Text | Google Scholar
25. Russano AM, Agea E, Corazzi L, Postle AD, De Libero G, Porcelli S, et al. Recognition of pollen-derived phosphatidyl-ethanolamine by human CD1d-restricted gamma delta T cells. J Allergy Clin Immunol (2006) 117(5):1178–84. doi:10.1016/j.jaci.2006.01.001
Pubmed Abstract | Pubmed Full Text | CrossRef Full Text | Google Scholar
26. Dieude M, Striegl H, Tyznik AJ, Wang J, Behar SM, Piccirillo CA, et al. Cardiolipin binds to CD1d and stimulates CD1d-restricted gammadelta T cells in the normal murine repertoire. J Immunol (2011) 186(8):4771–81. doi:10.4049/jimmunol.1000921
Pubmed Abstract | Pubmed Full Text | CrossRef Full Text | Google Scholar
27. Spada FM, Grant EP, Peters PJ, Sugita M, Melian A, Leslie DS, et al. Self-recognition of CD1 by gamma/delta T cells: implications for innate immunity. J Exp Med (2000) 191(6):937–48. doi:10.1084/jem.191.6.937
Pubmed Abstract | Pubmed Full Text | CrossRef Full Text | Google Scholar
28. Adams EJ, Chien YH, Garcia KC. Structure of a gammadelta T cell receptor in complex with the nonclassical MHC T22. Science (2005) 308(5719):227–31. doi:10.1126/science.1106885
Pubmed Abstract | Pubmed Full Text | CrossRef Full Text | Google Scholar
29. Crowley MP, Reich Z, Mavaddat N, Altman JD, Chien Y. The recognition of the nonclassical major histocompatibility complex (MHC) class I molecule, T10, by the gammadelta T cell, G8. J Exp Med (1997) 185(7):1223–30. doi:10.1084/jem.185.7.1223
Pubmed Abstract | Pubmed Full Text | CrossRef Full Text | Google Scholar
30. Laad AD, Thomas ML, Fakih AR, Chiplunkar SV. Human gamma delta T cells recognize heat shock protein-60 on oral tumor cells. Int J Cancer (1999) 80(5):709–14. doi:10.1002/(SICI)1097-0215(19990301)80:5<709::AID-IJC14>3.0.CO;2-R
Pubmed Abstract | Pubmed Full Text | CrossRef Full Text | Google Scholar
31. Thomas ML, Samant UC, Deshpande RK, Chiplunkar SV. Gammadelta T cells lyse autologous and allogenic oesophageal tumours: involvement of heat-shock proteins in the tumour cell lysis. Cancer Immunol Immunother (2000) 48(11):653–9. doi:10.1007/s002620050014
Pubmed Abstract | Pubmed Full Text | CrossRef Full Text | Google Scholar
32. O’Brien RL, Fu YX, Cranfill R, Dallas A, Ellis C, Reardon C, et al. Heat shock protein Hsp60-reactive gamma delta cells: a large, diversified T-lymphocyte subset with highly focused specificity. Proc Natl Acad Sci U S A (1992) 89(10):4348–52. doi:10.1073/pnas.89.10.4348
Pubmed Abstract | Pubmed Full Text | CrossRef Full Text | Google Scholar
33. Born WK, Zhang L, Nakayama M, Jin N, Chain JL, Huang Y, et al. Peptide antigens for gamma/delta T cells. Cell Mol Life Sci (2011) 68(14):2335–43. doi:10.1007/s00018-011-0697-3
Pubmed Abstract | Pubmed Full Text | CrossRef Full Text | Google Scholar
34. Prinz I, Sansoni A, Kissenpfennig A, Ardouin L, Malissen M, Malissen B. Visualization of the earliest steps of gammadelta T cell development in the adult thymus. Nat Immunol (2006) 7(9):995–1003. doi:10.1038/ni1371
Pubmed Abstract | Pubmed Full Text | CrossRef Full Text | Google Scholar
35. McVay LD, Carding SR. Generation of human gammadelta T-cell repertoires. Crit Rev Immunol (1999) 19(5–6):431–60.
36. Carding SR, Kyes S, Jenkinson EJ, Kingston R, Bottomly K, Owen JJ, et al. Developmentally regulated fetal thymic and extrathymic T-cell receptor gamma delta gene expression. Genes Dev (1990) 4(8):1304–15. doi:10.1101/gad.4.8.1304
Pubmed Abstract | Pubmed Full Text | CrossRef Full Text | Google Scholar
37. Velilla PA, Rugeles MT, Chougnet CA. Defective antigen-presenting cell function in human neonates. Clin Immunol (2006) 121(3):251–9. doi:10.1016/j.clim.2006.08.010
Pubmed Abstract | Pubmed Full Text | CrossRef Full Text | Google Scholar
38. Washburn T, Schweighoffer E, Gridley T, Chang D, Fowlkes BJ, Cado D, et al. Notch activity influences the alphabeta versus gammadelta T cell lineage decision. Cell (1997) 88(6):833–43. doi:10.1016/S0092-8674(00)81929-7
Pubmed Abstract | Pubmed Full Text | CrossRef Full Text | Google Scholar
39. Haks MC, Lefebvre JM, Lauritsen JP, Carleton M, Rhodes M, Miyazaki T, et al. Attenuation of gammadeltaTCR signaling efficiently diverts thymocytes to the alphabeta lineage. Immunity (2005) 22(5):595–606. doi:10.1016/j.immuni.2005.04.003
Pubmed Abstract | Pubmed Full Text | CrossRef Full Text | Google Scholar
40. Hayes SM, Li L, Love PE. TCR signal strength influences alphabeta/gamma delta lineage fate. Immunity (2005) 22(5):583–93. doi:10.1016/j.immuni.2005.03.014
Pubmed Abstract | Pubmed Full Text | CrossRef Full Text | Google Scholar
41. Zarin P, Wong GW, Mohtashami M, Wiest DL, Zuniga-Pflucker JC. Enforcement of gammadelta-lineage commitment by the pre-T-cell receptor in precursors with weak gammadelta-TCR signals. Proc Natl Acad Sci U S A (2014) 111(15):5658–63. doi:10.1073/pnas.1312872111
Pubmed Abstract | Pubmed Full Text | CrossRef Full Text | Google Scholar
42. Hayday AC, Pennington DJ. Key factors in the organized chaos of early T cell development. Nat Immunol (2007) 8(2):137–44. doi:10.1038/ni1436
Pubmed Abstract | Pubmed Full Text | CrossRef Full Text | Google Scholar
43. Garcia-Peydro M, de Yebenes VG, Toribio ML. Sustained Notch1 signaling instructs the earliest human intrathymic precursors to adopt a gammadelta T-cell fate in fetal thymus organ culture. Blood (2003) 102(7):2444–51. doi:10.1182/blood-2002-10-3261
Pubmed Abstract | Pubmed Full Text | CrossRef Full Text | Google Scholar
44. Van de Walle I, Waegemans E, De Medts J, De Smet G, De Smedt M, Snauwaert S, et al. Specific Notch receptor-ligand interactions control human TCR-alphabeta/gammadelta development by inducing differential Notch signal strength. J Exp Med (2013) 210(4):683–97. doi:10.1084/jem.20121798
Pubmed Abstract | Pubmed Full Text | CrossRef Full Text | Google Scholar
45. Van Coppernolle S, Vanhee S, Verstichel G, Snauwaert S, van der Spek A, Velghe I, et al. Notch induces human T-cell receptor gammadelta+ thymocytes to differentiate along a parallel, highly proliferative and bipotent CD4 CD8 double-positive pathway. Leukemia (2012) 26(1):127–38. doi:10.1038/leu.2011.324
Pubmed Abstract | Pubmed Full Text | CrossRef Full Text | Google Scholar
46. Gogoi D, Dar AA, Chiplunkar SV. Involvement of Notch in activation and effector functions of gammadelta T cells. J Immunol (2014) 192(5):2054–62. doi:10.4049/jimmunol.1300369
Pubmed Abstract | Pubmed Full Text | CrossRef Full Text | Google Scholar
47. Korn T, Petermann F. Development and function of interleukin 17-producing gammadelta T cells. Ann N Y Acad Sci (2012) 1247:34–45. doi:10.1111/j.1749-6632.2011.06355.x
48. Hayday A, Theodoridis E, Ramsburg E, Shires J. Intraepithelial lymphocytes: exploring the third way in immunology. Nat Immunol (2001) 2(11):997–1003. doi:10.1038/ni1101-997
Pubmed Abstract | Pubmed Full Text | CrossRef Full Text | Google Scholar
49. Bonneville M, O’Brien RL, Born WK. Gammadelta T cell effector functions: a blend of innate programming and acquired plasticity. Nat Rev Immunol (2010) 10(7):467–78. doi:10.1038/nri2781
Pubmed Abstract | Pubmed Full Text | CrossRef Full Text | Google Scholar
50. Bergstresser PR, Sullivan S, Streilein JW, Tigelaar RE. Origin and function of Thy-1+ dendritic epidermal cells in mice. J Invest Dermatol (1985) 85:85s–90s. doi:10.1111/1523-1747.ep12275516
51. Itohara S, Nakanishi N, Kanagawa O, Kubo R, Tonegawa S. Monoclonal antibodies specific to native murine T-cell receptor gamma delta: analysis of gamma delta T cells during thymic ontogeny and in peripheral lymphoid organs. Proc Natl Acad Sci U S A (1989) 86(13):5094–8. doi:10.1073/pnas.86.13.5094
Pubmed Abstract | Pubmed Full Text | CrossRef Full Text | Google Scholar
52. Girardi M. Immunosurveillance and immunoregulation by gammadelta T cells. J Invest Dermatol (2006) 126(1):25–31. doi:10.1038/sj.jid.5700003
Pubmed Abstract | Pubmed Full Text | CrossRef Full Text | Google Scholar
53. Janeway CA Jr, Jones B, Hayday A. Specificity and function of T cells bearing gamma delta receptors. Immunol Today (1988) 9(3):73–6. doi:10.1016/0167-5699(88)91267-4
54. Goodman T, Lefrancois L. Intraepithelial lymphocytes. Anatomical site, not T cell receptor form, dictates phenotype and function. J Exp Med (1989) 170(5):1569–81. doi:10.1084/jem.170.5.1569
Pubmed Abstract | Pubmed Full Text | CrossRef Full Text | Google Scholar
55. Vantourout P, Hayday A. Six-of-the-best: unique contributions of gammadelta T cells to immunology. Nat Rev Immunol (2013) 13(2):88–100. doi:10.1038/nri3384
Pubmed Abstract | Pubmed Full Text | CrossRef Full Text | Google Scholar
56. Qin G, Mao H, Zheng J, Sia SF, Liu Y, Chan PL, et al. Phosphoantigen-expanded human gammadelta T cells display potent cytotoxicity against monocyte-derived macrophages infected with human and avian influenza viruses. J Infect Dis (2009) 200(6):858–65. doi:10.1086/605413
Pubmed Abstract | Pubmed Full Text | CrossRef Full Text | Google Scholar
57. Dhar S, Chiplunkar SV. Lysis of aminobisphosphonate-sensitized MCF-7 breast tumor cells by Vgamma9Vdelta2 T cells. Cancer Immun (2010) 10:10.
58. Zhang H, Hu H, Jiang X, He H, Cui L, He W. Membrane HSP70: the molecule triggering gammadelta T cells in the early stage of tumorigenesis. Immunol Invest (2005) 34(4):453–68. doi:10.1080/08820130500265349
Pubmed Abstract | Pubmed Full Text | CrossRef Full Text | Google Scholar
59. Ansel KM, Ngo VN, Hyman PL, Luther SA, Forster R, Sedgwick JD, et al. A chemokine-driven positive feedback loop organizes lymphoid follicles. Nature (2000) 406(6793):309–14. doi:10.1038/35018581
Pubmed Abstract | Pubmed Full Text | CrossRef Full Text | Google Scholar
60. Conti L, Casetti R, Cardone M, Varano B, Martino A, Belardelli F, et al. Reciprocal activating interaction between dendritic cells and pamidronate-stimulated gammadelta T cells: role of CD86 and inflammatory cytokines. J Immunol (2005) 174(1):252–60. doi:10.4049/jimmunol.174.1.252
Pubmed Abstract | Pubmed Full Text | CrossRef Full Text | Google Scholar
61. Brandes M, Willimann K, Bioley G, Levy N, Eberl M, Luo M, et al. Cross-presenting human gammadelta T cells induce robust CD8+ alphabeta T cell responses. Proc Natl Acad Sci U S A (2009) 106(7):2307–12. doi:10.1073/pnas.0810059106
Pubmed Abstract | Pubmed Full Text | CrossRef Full Text | Google Scholar
62. Himoudi N, Morgenstern DA, Yan M, Vernay B, Saraiva L, Wu Y, et al. Human gammadelta T lymphocytes are licensed for professional antigen presentation by interaction with opsonized target cells. J Immunol (2012) 188(4):1708–16. doi:10.4049/jimmunol.1102654
Pubmed Abstract | Pubmed Full Text | CrossRef Full Text | Google Scholar
63. Brandes M, Willimann K, Moser B. Professional antigen-presentation function by human gammadelta T Cells. Science (2005) 309(5732):264–8. doi:10.1126/science.1110267
Pubmed Abstract | Pubmed Full Text | CrossRef Full Text | Google Scholar
64. Wesch D, Glatzel A, Kabelitz D. Differentiation of resting human peripheral blood gamma delta T cells toward Th1- or Th2-phenotype. Cell Immunol (2001) 212(2):110–7. doi:10.1006/cimm.2001.1850
Pubmed Abstract | Pubmed Full Text | CrossRef Full Text | Google Scholar
65. Ness-Schwickerath KJ, Jin C, Morita CT. Cytokine requirements for the differentiation and expansion of IL-17A- and IL-22-producing human Vgamma2Vdelta2 T cells. J Immunol (2010) 184(12):7268–80. doi:10.4049/jimmunol.1000600
Pubmed Abstract | Pubmed Full Text | CrossRef Full Text | Google Scholar
66. Caccamo N, La Mendola C, Orlando V, Meraviglia S, Todaro M, Stassi G, et al. Differentiation, phenotype, and function of interleukin-17-producing human Vgamma9Vdelta2 T cells. Blood (2011) 118(1):129–38. doi:10.1182/blood-2011-01-331298
Pubmed Abstract | Pubmed Full Text | CrossRef Full Text | Google Scholar
67. Casetti R, Agrati C, Wallace M, Sacchi A, Martini F, Martino A, et al. Cutting edge: TGF-beta1 and IL-15 Induce FOXP3+ gammadelta regulatory T cells in the presence of antigen stimulation. J Immunol (2009) 183(6):3574–7. doi:10.4049/jimmunol.0901334
Pubmed Abstract | Pubmed Full Text | CrossRef Full Text | Google Scholar
68. Li X, Kang N, Zhang X, Dong X, Wei W, Cui L, et al. Generation of human regulatory gammadelta T cells by TCRgammadelta stimulation in the presence of TGF-beta and their involvement in the pathogenesis of systemic lupus erythematosus. J Immunol (2011) 186(12):6693–700. doi:10.4049/jimmunol.1002776
Pubmed Abstract | Pubmed Full Text | CrossRef Full Text | Google Scholar
69. Keijsers RR, Joosten I, van Erp PE, Koenen HJ, van de Kerkhof PC. Cellular sources of il-17: a paradigm shift? Exp Dermatol (2014) 23(11):799–803. doi:10.1111/exd.12487
70. Martin B, Hirota K, Cua DJ, Stockinger B, Veldhoen M. Interleukin-17-producing gammadelta T cells selectively expand in response to pathogen products and environmental signals. Immunity (2009) 31(2):321–30. doi:10.1016/j.immuni.2009.06.020
Pubmed Abstract | Pubmed Full Text | CrossRef Full Text | Google Scholar
71. Itoh S, Kimura N, Axtell RC, Velotta JB, Gong Y, Wang X, et al. Interleukin-17 accelerates allograft rejection by suppressing regulatory T cell expansion. Circulation (2011) 124(11 Suppl):S187–96. doi:10.1161/CIRCULATIONAHA.110.014852
Pubmed Abstract | Pubmed Full Text | CrossRef Full Text | Google Scholar
72. Petermann F, Rothhammer V, Claussen MC, Haas JD, Blanco LR, Heink S, et al. gammadelta T cells enhance autoimmunity by restraining regulatory T cell responses via an interleukin-23-dependent mechanism. Immunity (2010) 33(3):351–63. doi:10.1016/j.immuni.2010.08.013
Pubmed Abstract | Pubmed Full Text | CrossRef Full Text | Google Scholar
73. Lo Re S, Dumoutier L, Couillin I, Van Vyve C, Yakoub Y, Uwambayinema F, et al. IL-17A-producing gammadelta T and Th17 lymphocytes mediate lung inflammation but not fibrosis in experimental silicosis. J Immunol (2010) 184(11):6367–77. doi:10.4049/jimmunol.0900459
Pubmed Abstract | Pubmed Full Text | CrossRef Full Text | Google Scholar
74. Wu P, Wu D, Ni C, Ye J, Chen W, Hu G, et al. Gammadeltat17 cells promote the accumulation and expansion of myeloid-derived suppressor cells in human colorectal cancer. Immunity (2014) 40(5):785–800. doi:10.1016/j.immuni.2014.03.013
Pubmed Abstract | Pubmed Full Text | CrossRef Full Text | Google Scholar
75. Price AE, Reinhardt RL, Liang HE, Locksley RM. Marking and quantifying IL-17A-producing cells in vivo. PLoS One (2012) 7(6):e39750. doi:10.1371/journal.pone.0039750
Pubmed Abstract | Pubmed Full Text | CrossRef Full Text | Google Scholar
76. Chien YH, Zeng X, Prinz I. The natural and the inducible: interleukin (IL)-17-producing gammadelta T cells. Trends Immunol (2013) 34(4):151–4. doi:10.1016/j.it.2012.11.004
Pubmed Abstract | Pubmed Full Text | CrossRef Full Text | Google Scholar
77. Prinz I, Fohse L. Gammadelta T cells are not alone. Immunol Cell Biol (2012) 90(4):370–1. doi:10.1038/icb.2011.61
78. Shibata K, Yamada H, Nakamura M, Hatano S, Katsuragi Y, Kominami R, et al. IFN-gamma-producing and IL-17-producing gammadelta T cells differentiate at distinct developmental stages in murine fetal thymus. J Immunol (2014) 192(5):2210–8. doi:10.4049/jimmunol.1302145
Pubmed Abstract | Pubmed Full Text | CrossRef Full Text | Google Scholar
79. Pennington DJ, Silva-Santos B, Hayday AC. Gammadelta T cell development – having the strength to get there. Curr Opin Immunol (2005) 17(2):108–15. doi:10.1016/j.coi.2005.01.009
Pubmed Abstract | Pubmed Full Text | CrossRef Full Text | Google Scholar
80. Turchinovich G, Pennington DJ. T cell receptor signalling in gammadelta cell development: strength isn’t everything. Trends Immunol (2011) 32(12):567–73. doi:10.1016/j.it.2011.09.005
Pubmed Abstract | Pubmed Full Text | CrossRef Full Text | Google Scholar
81. Prinz I, Silva-Santos B, Pennington DJ. Functional development of gammadelta T cells. Eur J Immunol (2013) 43(8):1988–94. doi:10.1002/eji.201343759
82. Ramond C, Berthault C, Burlen-Defranoux O, de Sousa AP, Guy-Grand D, Vieira P, et al. Two waves of distinct hematopoietic progenitor cells colonize the fetal thymus. Nat Immunol (2014) 15(1):27–35. doi:10.1038/ni.2782
Pubmed Abstract | Pubmed Full Text | CrossRef Full Text | Google Scholar
83. Vicari AP, Mocci S, Openshaw P, O’Garra A, Zlotnik A. Mouse gamma delta TCR+NK1.1+ thymocytes specifically produce interleukin-4, are major histocompatibility complex class I independent, and are developmentally related to alpha beta TCR+NK1.1+ thymocytes. Eur J Immunol (1996) 26(7):1424–9. doi:10.1002/eji.1830260704
Pubmed Abstract | Pubmed Full Text | CrossRef Full Text | Google Scholar
84. Azuara V, Levraud JP, Lembezat MP, Pereira P. A novel subset of adult gamma delta thymocytes that secretes a distinct pattern of cytokines and expresses a very restricted T cell receptor repertoire. Eur J Immunol (1997) 27(2):544–53. doi:10.1002/eji.1830270228
Pubmed Abstract | Pubmed Full Text | CrossRef Full Text | Google Scholar
85. Haas JD, Ravens S, Duber S, Sandrock I, Oberdorfer L, Kashani E, et al. Development of interleukin-17-producing gammadelta T cells is restricted to a functional embryonic wave. Immunity (2012) 37(1):48–59. doi:10.1016/j.immuni.2012.06.003
Pubmed Abstract | Pubmed Full Text | CrossRef Full Text | Google Scholar
86. Jensen KD, Su X, Shin S, Li L, Youssef S, Yamasaki S, et al. Thymic selection determines gammadelta T cell effector fate: antigen-naive cells make interleukin-17 and antigen-experienced cells make interferon gamma. Immunity (2008) 29(1):90–100. doi:10.1016/j.immuni.2008.04.022
Pubmed Abstract | Pubmed Full Text | CrossRef Full Text | Google Scholar
87. Forster R, Schubel A, Breitfeld D, Kremmer E, Renner-Muller I, Wolf E, et al. CCR7 coordinates the primary immune response by establishing functional microenvironments in secondary lymphoid organs. Cell (1999) 99(1):23–33. doi:10.1016/S0092-8674(00)80059-8
Pubmed Abstract | Pubmed Full Text | CrossRef Full Text | Google Scholar
88. Ribot JC, Silva-Santos B. Differentiation and activation of gammadelta T Lymphocytes: focus on CD27 and CD28 costimulatory receptors. Adv Exp Med Biol (2013) 785:95–105. doi:10.1007/978-1-4614-6217-0_11
Pubmed Abstract | Pubmed Full Text | CrossRef Full Text | Google Scholar
89. Ribot JC, deBarros A, Pang DJ, Neves JF, Peperzak V, Roberts SJ, et al. CD27 is a thymic determinant of the balance between interferon-gamma- and interleukin 17-producing gammadelta T cell subsets. Nat Immunol (2009) 10(4):427–36. doi:10.1038/ni.1717
Pubmed Abstract | Pubmed Full Text | CrossRef Full Text | Google Scholar
90. Muller JR, Siebenlist U. Lymphotoxin beta receptor induces sequential activation of distinct NF-kappa B factors via separate signaling pathways. J Biol Chem (2003) 278(14):12006–12. doi:10.1074/jbc.M210768200
Pubmed Abstract | Pubmed Full Text | CrossRef Full Text | Google Scholar
91. Silva-Santos B, Pennington DJ, Hayday AC. Lymphotoxin-mediated regulation of gammadelta cell differentiation by alphabeta T cell progenitors. Science (2005) 307(5711):925–8. doi:10.1126/science.1103978
Pubmed Abstract | Pubmed Full Text | CrossRef Full Text | Google Scholar
92. Hayes SM, Laird RM. Genetic requirements for the development and differentiation of interleukin-17-producing gammadelta T cells. Crit Rev Immunol (2012) 32(1):81–95. doi:10.1615/CritRevImmunol.v32.i1.50
Pubmed Abstract | Pubmed Full Text | CrossRef Full Text | Google Scholar
93. Powolny-Budnicka I, Riemann M, Tanzer S, Schmid RM, Hehlgans T, Weih F. RelA and RelB transcription factors in distinct thymocyte populations control lymphotoxin-dependent interleukin-17 production in gammadelta T cells. Immunity (2011) 34(3):364–74. doi:10.1016/j.immuni.2011.02.019
Pubmed Abstract | Pubmed Full Text | CrossRef Full Text | Google Scholar
94. Wencker M, Turchinovich G, Di Marco Barros R, Deban L, Jandke A, Cope A, et al. Innate-like T cells straddle innate and adaptive immunity by altering antigen-receptor responsiveness. Nat Immunol (2014) 15(1):80–7. doi:10.1038/ni.2773
Pubmed Abstract | Pubmed Full Text | CrossRef Full Text | Google Scholar
95. Malhotra N, Narayan K, Cho OH, Sylvia KE, Yin C, Melichar H, et al. A network of high-mobility group box transcription factors programs innate interleukin-17 production. Immunity (2013) 38(4):681–93. doi:10.1016/j.immuni.2013.01.010
Pubmed Abstract | Pubmed Full Text | CrossRef Full Text | Google Scholar
96. Laird RM, Laky K, Hayes SM. Unexpected role for the B cell-specific Src family kinase B lymphoid kinase in the development of IL-17-producing gammadelta T cells. J Immunol (2010) 185(11):6518–27. doi:10.4049/jimmunol.1002766
Pubmed Abstract | Pubmed Full Text | CrossRef Full Text | Google Scholar
97. Gray EE, Ramirez-Valle F, Xu Y, Wu S, Wu Z, Karjalainen KE, et al. Deficiency in IL-17-committed Vgamma4(+) gammadelta T cells in a spontaneous Sox13-mutant CD45.1(+) congenic mouse substrain provides protection from dermatitis. Nat Immunol (2013) 14(6):584–92. doi:10.1038/ni.2585
Pubmed Abstract | Pubmed Full Text | CrossRef Full Text | Google Scholar
98. Narayan K, Sylvia KE, Malhotra N, Yin CC, Martens G, Vallerskog T, et al. Intrathymic programming of effector fates in three molecularly distinct gammadelta T cell subtypes. Nat Immunol (2012) 13(5):511–8. doi:10.1038/ni.2247
Pubmed Abstract | Pubmed Full Text | CrossRef Full Text | Google Scholar
99. Turchinovich G, Hayday AC. Skint-1 identifies a common molecular mechanism for the development of interferon-gamma-secreting versus interleukin-17-secreting gammadelta T cells. Immunity (2011) 35(1):59–68. doi:10.1016/j.immuni.2011.04.018
Pubmed Abstract | Pubmed Full Text | CrossRef Full Text | Google Scholar
100. Maekawa Y, Tsukumo S, Chiba S, Hirai H, Hayashi Y, Okada H, et al. Delta1-Notch3 interactions bias the functional differentiation of activated CD4+ T cells. Immunity (2003) 19(4):549–59. doi:10.1016/S1074-7613(03)00270-X
Pubmed Abstract | Pubmed Full Text | CrossRef Full Text | Google Scholar
101. Shibata K, Yamada H, Sato T, Dejima T, Nakamura M, Ikawa T, et al. Notch-Hes1 pathway is required for the development of IL-17-producing gammadelta T cells. Blood (2011) 118(3):586–93. doi:10.1182/blood-2011-02-334995
Pubmed Abstract | Pubmed Full Text | CrossRef Full Text | Google Scholar
102. Keerthivasan S, Suleiman R, Lawlor R, Roderick J, Bates T, Minter L, et al. Notch signaling regulates mouse and human Th17 differentiation. J Immunol (2011) 187(2):692–701. doi:10.4049/jimmunol.1003658
Pubmed Abstract | Pubmed Full Text | CrossRef Full Text | Google Scholar
103. Sutton CE, Lalor SJ, Sweeney CM, Brereton CF, Lavelle EC, Mills KH. Interleukin-1 and IL-23 induce innate IL-17 production from gammadelta T cells, amplifying Th17 responses and autoimmunity. Immunity (2009) 31(2):331–41. doi:10.1016/j.immuni.2009.08.001
Pubmed Abstract | Pubmed Full Text | CrossRef Full Text | Google Scholar
104. Do JS, Fink PJ, Li L, Spolski R, Robinson J, Leonard WJ, et al. Cutting edge: spontaneous development of IL-17-producing gamma delta T cells in the thymus occurs via a TGF-beta 1-dependent mechanism. J Immunol (2010) 184(4):1675–9. doi:10.4049/jimmunol.0903539
Pubmed Abstract | Pubmed Full Text | CrossRef Full Text | Google Scholar
105. Lochner M, Peduto L, Cherrier M, Sawa S, Langa F, Varona R, et al. In vivo equilibrium of proinflammatory IL-17+ and regulatory IL-10+ Foxp3+ RORgamma t+ T cells. J Exp Med (2008) 205(6):1381–93. doi:10.1084/jem.20080034
Pubmed Abstract | Pubmed Full Text | CrossRef Full Text | Google Scholar
106. Suzuki M, Yamamoto M, Sugimoto A, Nakamura S, Motoda R, Orita K. Delta-4 expression on a stromal cell line is augmented by interleukin-6 via STAT3 activation. Exp Hematol (2006) 34(9):1143–50. doi:10.1016/j.exphem.2006.04.027
Pubmed Abstract | Pubmed Full Text | CrossRef Full Text | Google Scholar
107. Meeks KD, Sieve AN, Kolls JK, Ghilardi N, Berg RE. IL-23 is required for protection against systemic infection with Listeria monocytogenes. J Immunol (2009) 183(12):8026–34. doi:10.4049/jimmunol.0901588
Pubmed Abstract | Pubmed Full Text | CrossRef Full Text | Google Scholar
108. Riol-Blanco L, Lazarevic V, Awasthi A, Mitsdoerffer M, Wilson BS, Croxford A, et al. IL-23 receptor regulates unconventional IL-17-producing T cells that control bacterial infections. J Immunol (2010) 184(4):1710–20. doi:10.4049/jimmunol.0902796
Pubmed Abstract | Pubmed Full Text | CrossRef Full Text | Google Scholar
109. McGeachy MJ, Chen Y, Tato CM, Laurence A, Joyce-Shaikh B, Blumenschein WM, et al. The interleukin 23 receptor is essential for the terminal differentiation of interleukin 17-producing effector T helper cells in vivo. Nat Immunol (2009) 10(3):314–24. doi:10.1038/ni.1698
Pubmed Abstract | Pubmed Full Text | CrossRef Full Text | Google Scholar
110. Doisne JM, Soulard V, Becourt C, Amniai L, Henrot P, Havenar-Daughton C, et al. Cutting edge: crucial role of IL-1 and IL-23 in the innate IL-17 response of peripheral lymph node NK1.1- invariant NKT cells to bacteria. J Immunol (2011) 186(2):662–6. doi:10.4049/jimmunol.1002725
Pubmed Abstract | Pubmed Full Text | CrossRef Full Text | Google Scholar
111. DeBarros A, Chaves-Ferreira M, d’Orey F, Ribot JC, Silva-Santos B. CD70-CD27 interactions provide survival and proliferative signals that regulate T cell receptor-driven activation of human gammadelta peripheral blood lymphocytes. Eur J Immunol (2011) 41(1):195–201. doi:10.1002/eji.201040905
Pubmed Abstract | Pubmed Full Text | CrossRef Full Text | Google Scholar
112. Moens E, Brouwer M, Dimova T, Goldman M, Willems F, Vermijlen D. IL-23R and TCR signaling drives the generation of neonatal Vgamma9Vdelta2 T cells expressing high levels of cytotoxic mediators and producing IFN-gamma and IL-17. J Leukoc Biol (2011) 89(5):743–52. doi:10.1189/jlb.0910501
Pubmed Abstract | Pubmed Full Text | CrossRef Full Text | Google Scholar
113. Michel ML, Pang DJ, Haque SF, Potocnik AJ, Pennington DJ, Hayday AC. Interleukin 7 (IL-7) selectively promotes mouse and human IL-17-producing gammadelta cells. Proc Natl Acad Sci U S A (2012) 109(43):17549–54. doi:10.1073/pnas.1204327109
Pubmed Abstract | Pubmed Full Text | CrossRef Full Text | Google Scholar
114. Ribot JC, Ribeiro ST, Correia DV, Sousa AE, Silva-Santos B. Human gammadelta thymocytes are functionally immature and differentiate into cytotoxic type 1 effector T cells upon IL-2/IL-15 signaling. J Immunol (2014) 192(5):2237–43. doi:10.4049/jimmunol.1303119
Pubmed Abstract | Pubmed Full Text | CrossRef Full Text | Google Scholar
115. Sallusto F, Geginat J, Lanzavecchia A. Central memory and effector memory T cell subsets: function, generation, and maintenance. Annu Rev Immunol (2004) 22:745–63. doi:10.1146/annurev.immunol.22.012703.104702
Pubmed Abstract | Pubmed Full Text | CrossRef Full Text | Google Scholar
116. Roark CL, Simonian PL, Fontenot AP, Born WK, O’Brien RL. Gammadelta T cells: an important source of IL-17. Curr Opin Immunol (2008) 20(3):353–7. doi:10.1016/j.coi.2008.03.006
117. Sabat R, Witte E, Witte K, Wolk K. IL-22 and IL-17: an overview. In: Quesniaux V, Ryffel B, Padova F, editors. IL-17, IL-22 and Their Producing Cells: Role in Inflammation and Autoimmunity. Progress in Inflammation Research. Basel: Springer (2013). p. 11–35.
118. Eyerich S, Eyerich K, Cavani A, Schmidt-Weber C. IL-17 and IL-22: siblings, not twins. Trends Immunol (2010) 31(9):354–61. doi:10.1016/j.it.2010.06.004
Pubmed Abstract | Pubmed Full Text | CrossRef Full Text | Google Scholar
119. Okamoto Yoshida Y, Umemura M, Yahagi A, O’Brien RL, Ikuta K, Kishihara K, et al. Essential role of IL-17A in the formation of a mycobacterial infection-induced granuloma in the lung. J Immunol (2010) 184(8):4414–22. doi:10.4049/jimmunol.0903332
120. Lockhart E, Green AM, Flynn JL. IL-17 production is dominated by gammadelta T cells rather than CD4 T cells during Mycobacterium tuberculosis infection. J Immunol (2006) 177(7):4662–9. doi:10.4049/jimmunol.177.7.4662
Pubmed Abstract | Pubmed Full Text | CrossRef Full Text | Google Scholar
121. Curtis MM, Way SS. Interleukin-17 in host defence against bacterial, mycobacterial and fungal pathogens. Immunology (2009) 126(2):177–85. doi:10.1111/j.1365-2567.2008.03017.x
Pubmed Abstract | Pubmed Full Text | CrossRef Full Text | Google Scholar
122. Umemura M, Yahagi A, Hamada S, Begum MD, Watanabe H, Kawakami K, et al. IL-17-mediated regulation of innate and acquired immune response against pulmonary Mycobacterium bovis bacille Calmette-Guerin infection. J Immunol (2007) 178(6):3786–96. doi:10.4049/jimmunol.178.6.3786
Pubmed Abstract | Pubmed Full Text | CrossRef Full Text | Google Scholar
123. Xu S, Han Y, Xu X, Bao Y, Zhang M, Cao X. IL-17A-producing gammadeltaT cells promote CTL responses against Listeria monocytogenes infection by enhancing dendritic cell cross-presentation. J Immunol (2010) 185(10):5879–87. doi:10.4049/jimmunol.1001763
Pubmed Abstract | Pubmed Full Text | CrossRef Full Text | Google Scholar
124. Happel KI, Dubin PJ, Zheng M, Ghilardi N, Lockhart C, Quinton LJ, et al. Divergent roles of IL-23 and IL-12 in host defense against Klebsiella pneumoniae. J Exp Med (2005) 202(6):761–9. doi:10.1084/jem.20050193
Pubmed Abstract | Pubmed Full Text | CrossRef Full Text | Google Scholar
125. Mangan PR, Harrington LE, O’Quinn DB, Helms WS, Bullard DC, Elson CO, et al. Transforming growth factor-beta induces development of the T(H)17 lineage. Nature (2006) 441(7090):231–4. doi:10.1038/nature04754
Pubmed Abstract | Pubmed Full Text | CrossRef Full Text | Google Scholar
126. Zheng Y, Valdez PA, Danilenko DM, Hu Y, Sa SM, Gong Q, et al. Interleukin-22 mediates early host defense against attaching and effacing bacterial pathogens. Nat Med (2008) 14(3):282–9. doi:10.1038/nm1720
Pubmed Abstract | Pubmed Full Text | CrossRef Full Text | Google Scholar
127. Schulz SM, Kohler G, Schutze N, Knauer J, Straubinger RK, Chackerian AA, et al. Protective immunity to systemic infection with attenuated Salmonella enterica serovar enteritidis in the absence of IL-12 is associated with IL-23-dependent IL-22, but not IL-17. J Immunol (2008) 181(11):7891–901. doi:10.4049/jimmunol.181.11.7891
Pubmed Abstract | Pubmed Full Text | CrossRef Full Text | Google Scholar
128. Godinez I, Raffatellu M, Chu H, Paixao TA, Haneda T, Santos RL, et al. Interleukin-23 orchestrates mucosal responses to Salmonella enterica serotype Typhimurium in the intestine. Infect Immun (2009) 77(1):387–98. doi:10.1128/IAI.00933-08
Pubmed Abstract | Pubmed Full Text | CrossRef Full Text | Google Scholar
129. Kelly MN, Kolls JK, Happel K, Schwartzman JD, Schwarzenberger P, Combe C, et al. Interleukin-17/interleukin-17 receptor-mediated signaling is important for generation of an optimal polymorphonuclear response against Toxoplasma gondii infection. Infect Immun (2005) 73(1):617–21. doi:10.1128/IAI.73.1.617-621.2005
Pubmed Abstract | Pubmed Full Text | CrossRef Full Text | Google Scholar
130. Dejima T, Shibata K, Yamada H, Hara H, Iwakura Y, Naito S, et al. Protective role of naturally occurring interleukin-17A-producing gammadelta T cells in the lung at the early stage of systemic candidiasis in mice. Infect Immun (2011) 79(11):4503–10. doi:10.1128/IAI.05799-11
Pubmed Abstract | Pubmed Full Text | CrossRef Full Text | Google Scholar
131. Wozniak KL, Kolls JK, Wormley FL Jr. Depletion of neutrophils in a protective model of pulmonary cryptococcosis results in increased IL-17A production by gammadelta T cells. BMC Immunol (2012) 13:65. doi:10.1186/1471-2172-13-65
Pubmed Abstract | Pubmed Full Text | CrossRef Full Text | Google Scholar
132. Ribot JC, Chaves-Ferreira M, d’Orey F, Wencker M, Goncalves-Sousa N, Decalf J, et al. Cutting edge: adaptive versus innate receptor signals selectively control the pool sizes of murine IFN-gamma- or IL-17-producing gammadelta T cells upon infection. J Immunol (2010) 185(11):6421–5. doi:10.4049/jimmunol.1002283
Pubmed Abstract | Pubmed Full Text | CrossRef Full Text | Google Scholar
133. Wang X, Sun R, Wei H, Tian Z. High-mobility group box 1 (HMGB1)-Toll-like receptor (TLR)4-interleukin (IL)-23-IL-17A axis in drug-induced damage-associated lethal hepatitis: interaction of gammadelta T cells with macrophages. Hepatology (2013) 57(1):373–84. doi:10.1002/hep.25982
Pubmed Abstract | Pubmed Full Text | CrossRef Full Text | Google Scholar
134. Hasegawa E, Sonoda KH, Shichita T, Morita R, Sekiya T, Kimura A, et al. IL-23-independent induction of IL-17 from gammadeltaT cells and innate lymphoid cells promotes experimental intraocular neovascularization. J Immunol (2013) 190(4):1778–87. doi:10.4049/jimmunol.1202495
Pubmed Abstract | Pubmed Full Text | CrossRef Full Text | Google Scholar
135. Xia Q, Duan L, Shi L, Zheng F, Gong F, Fang M. High-mobility group box 1 accelerates early acute allograft rejection via enhancing IL-17+ gammadelta T-cell response. Transpl Int (2014) 27(4):399–407. doi:10.1111/tri.12264
Pubmed Abstract | Pubmed Full Text | CrossRef Full Text | Google Scholar
136. Cho JS, Pietras EM, Garcia NC, Ramos RI, Farzam DM, Monroe HR, et al. IL-17 is essential for host defense against cutaneous Staphylococcus aureus infection in mice. J Clin Invest (2010) 120(5):1762–73. doi:10.1172/JCI40891
Pubmed Abstract | Pubmed Full Text | CrossRef Full Text | Google Scholar
137. Hirota K, Yoshitomi H, Hashimoto M, Maeda S, Teradaira S, Sugimoto N, et al. Preferential recruitment of CCR6-expressing Th17 cells to inflamed joints via CCL20 in rheumatoid arthritis and its animal model. J Exp Med (2007) 204(12):2803–12. doi:10.1084/jem.20071397
Pubmed Abstract | Pubmed Full Text | CrossRef Full Text | Google Scholar
138. Haas JD, Gonzalez FH, Schmitz S, Chennupati V, Fohse L, Kremmer E, et al. CCR6 and NK1.1 distinguish between IL-17A and IFN-gamma-producing gammadelta effector T cells. Eur J Immunol (2009) 39(12):3488–97. doi:10.1002/eji.200939922
Pubmed Abstract | Pubmed Full Text | CrossRef Full Text | Google Scholar
139. Costa MF, Bornstein VU, Candea AL, Henriques-Pons A, Henriques MG, Penido C. CCL25 induces alpha(4)beta(7) integrin-dependent migration of IL-17(+) gammadelta T lymphocytes during an allergic reaction. Eur J Immunol (2012) 42(5):1250–60. doi:10.1002/eji.201142021
Pubmed Abstract | Pubmed Full Text | CrossRef Full Text | Google Scholar
140. Penido C, Costa MF, Souza MC, Costa KA, Candea AL, Benjamim CF, et al. Involvement of CC chemokines in gammadelta T lymphocyte trafficking during allergic inflammation: the role of CCL2/CCR2 pathway. Int Immunol (2008) 20(1):129–39. doi:10.1093/intimm/dxm128
Pubmed Abstract | Pubmed Full Text | CrossRef Full Text | Google Scholar
141. Kabelitz D, Wesch D. Features and functions of gamma delta T lymphocytes: focus on chemokines and their receptors. Crit Rev Immunol (2003) 23(5–6):339–70. doi:10.1615/CritRevImmunol.v23.i56.10
Pubmed Abstract | Pubmed Full Text | CrossRef Full Text | Google Scholar
142. Simonian PL, Wehrmann F, Roark CL, Born WK, O’Brien RL, Fontenot AP. Gammadelta T cells protect against lung fibrosis via IL-22. J Exp Med (2010) 207(10):2239–53. doi:10.1084/jem.20100061
Pubmed Abstract | Pubmed Full Text | CrossRef Full Text | Google Scholar
143. Roark CL, French JD, Taylor MA, Bendele AM, Born WK, O’Brien RL. Exacerbation of collagen-induced arthritis by oligoclonal, IL-17-producing gamma delta T cells. J Immunol (2007) 179(8):5576–83. doi:10.4049/jimmunol.179.8.5576
Pubmed Abstract | Pubmed Full Text | CrossRef Full Text | Google Scholar
144. Pollinger B, Junt T, Metzler B, Walker UA, Tyndall A, Allard C, et al. Th17 cells, not IL-17+ gammadelta T cells, drive arthritic bone destruction in mice and humans. J Immunol (2011) 186(4):2602–12. doi:10.4049/jimmunol.1003370
Pubmed Abstract | Pubmed Full Text | CrossRef Full Text | Google Scholar
145. Ito Y, Usui T, Kobayashi S, Iguchi-Hashimoto M, Ito H, Yoshitomi H, et al. Gamma/delta T cells are the predominant source of interleukin-17 in affected joints in collagen-induced arthritis, but not in rheumatoid arthritis. Arthritis Rheum (2009) 60(8):2294–303. doi:10.1002/art.24687
Pubmed Abstract | Pubmed Full Text | CrossRef Full Text | Google Scholar
146. Shichita T, Sugiyama Y, Ooboshi H, Sugimori H, Nakagawa R, Takada I, et al. Pivotal role of cerebral interleukin-17-producing gammadeltaT cells in the delayed phase of ischemic brain injury. Nat Med (2009) 15(8):946–50. doi:10.1038/nm.1999
Pubmed Abstract | Pubmed Full Text | CrossRef Full Text | Google Scholar
147. Jaffar Z, Ferrini ME, Shaw PK, FitzGerald GA, Roberts K. Prostaglandin I(2) promotes the development of IL-17-producing gammadelta T cells that associate with the epithelium during allergic lung inflammation. J Immunol (2011) 187(10):5380–91. doi:10.4049/jimmunol.1101261
Pubmed Abstract | Pubmed Full Text | CrossRef Full Text | Google Scholar
148. Murdoch JR, Lloyd CM. Resolution of allergic airway inflammation and airway hyperreactivity is mediated by IL-17-producing {gamma}{delta}T cells. Am J Respir Crit Care Med (2010) 182(4):464–76. doi:10.1164/rccm.200911-1775OC
Pubmed Abstract | Pubmed Full Text | CrossRef Full Text | Google Scholar
149. Gogoi D, Chiplunkar SV. Targeting gamma delta T cells for cancer immunotherapy: bench to bedside. Indian J Med Res (2013) 138(5):755–61.
150. von Lilienfeld-Toal M, Nattermann J, Feldmann G, Sievers E, Frank S, Strehl J, et al. Activated gammadelta T cells express the natural cytotoxicity receptor natural killer p 44 and show cytotoxic activity against myeloma cells. Clin Exp Immunol (2006) 144(3):528–33. doi:10.1111/j.1365-2249.2006.03078.x
Pubmed Abstract | Pubmed Full Text | CrossRef Full Text | Google Scholar
151. Zhou J, Kang N, Cui L, Ba D, He W. Anti-gammadelta TCR antibody-expanded gammadelta T cells: a better choice for the adoptive immunotherapy of lymphoid malignancies. Cell Mol Immunol (2012) 9(1):34–44. doi:10.1038/cmi.2011.16
Pubmed Abstract | Pubmed Full Text | CrossRef Full Text | Google Scholar
152. Lanca T, Correia DV, Moita CF, Raquel H, Neves-Costa A, Ferreira C, et al. The MHC class Ib protein ULBP1 is a nonredundant determinant of leukemia/lymphoma susceptibility to gammadelta T-cell cytotoxicity. Blood (2010) 115(12):2407–11. doi:10.1182/blood-2009-08-237123
Pubmed Abstract | Pubmed Full Text | CrossRef Full Text | Google Scholar
153. Siegers GM, Felizardo TC, Mathieson AM, Kosaka Y, Wang XH, Medin JA, et al. Anti-leukemia activity of in vitro-expanded human gamma delta T cells in a xenogeneic Ph+ leukemia model. PLoS One (2011) 6(2):e16700. doi:10.1371/journal.pone.0016700
Pubmed Abstract | Pubmed Full Text | CrossRef Full Text | Google Scholar
154. Kong Y, Cao W, Xi X, Ma C, Cui L, He W. The NKG2D ligand ULBP4 binds to TCRgamma9/delta2 and induces cytotoxicity to tumor cells through both TCRgammadelta and NKG2D. Blood (2009) 114(2):310–7. doi:10.1182/blood-2008-12-196287
Pubmed Abstract | Pubmed Full Text | CrossRef Full Text | Google Scholar
155. Viey E, Fromont G, Escudier B, Morel Y, Da Rocha S, Chouaib S, et al. Phosphostim-activated gamma delta T cells kill autologous metastatic renal cell carcinoma. J Immunol (2005) 174(3):1338–47. doi:10.4049/jimmunol.174.3.1338
Pubmed Abstract | Pubmed Full Text | CrossRef Full Text | Google Scholar
156. Viey E, Lucas C, Romagne F, Escudier B, Chouaib S, Caignard A. Chemokine receptors expression and migration potential of tumor-infiltrating and peripheral-expanded Vgamma9Vdelta2 T cells from renal cell carcinoma patients. J Immunother (2008) 31(3):313–23. doi:10.1097/CJI.0b013e3181609988
Pubmed Abstract | Pubmed Full Text | CrossRef Full Text | Google Scholar
157. Fournie JJ, Sicard H, Poupot M, Bezombes C, Blanc A, Romagne F, et al. What lessons can be learned from gammadelta T cell-based cancer immunotherapy trials? Cell Mol Immunol (2013) 10(1):35–41. doi:10.1038/cmi.2012.39
Pubmed Abstract | Pubmed Full Text | CrossRef Full Text | Google Scholar
158. Ma Y, Aymeric L, Locher C, Mattarollo SR, Delahaye NF, Pereira P, et al. Contribution of IL-17-producing gamma delta T cells to the efficacy of anticancer chemotherapy. J Exp Med (2011) 208(3):491–503. doi:10.1084/jem.20100269
Pubmed Abstract | Pubmed Full Text | CrossRef Full Text | Google Scholar
159. Takeuchi A, Dejima T, Yamada H, Shibata K, Nakamura R, Eto M, et al. IL-17 production by gammadelta T cells is important for the antitumor effect of Mycobacterium bovis bacillus Calmette-Guerin treatment against bladder cancer. Eur J Immunol (2011) 41(1):246–51. doi:10.1002/eji.201040773
Pubmed Abstract | Pubmed Full Text | CrossRef Full Text | Google Scholar
160. Yang B, Kang H, Fung A, Zhao H, Wang T, Ma D. The role of interleukin 17 in tumour proliferation, angiogenesis, and metastasis. Mediators Inflamm (2014) 2014:623759. doi:10.1155/2014/623759
Pubmed Abstract | Pubmed Full Text | CrossRef Full Text | Google Scholar
161. Rei M, Goncalves-Sousa N, Lanca T, Thompson RG, Mensurado S, Balkwill FR, et al. Murine CD27(-) Vgamma6(+) gammadelta T cells producing IL-17A promote ovarian cancer growth via mobilization of protumor small peritoneal macrophages. Proc Natl Acad Sci U S A (2014) 111(34):E3562–70. doi:10.1073/pnas.1403424111
Pubmed Abstract | Pubmed Full Text | CrossRef Full Text | Google Scholar
162. Ma S, Cheng Q, Cai Y, Gong H, Wu Y, Yu X, et al. IL-17A produced by gammadelta T cells promotes tumor growth in hepatocellular carcinoma. Cancer Res (2014) 74(7):1969–82. doi:10.1158/0008-5472.CAN-13-2534
Pubmed Abstract | Pubmed Full Text | CrossRef Full Text | Google Scholar
163. Fialova A, Partlova S, Sojka L, Hromadkova H, Brtnicky T, Fucikova J, et al. Dynamics of T-cell infiltration during the course of ovarian cancer: the gradual shift from a Th17 effector cell response to a predominant infiltration by regulatory T-cells. Int J Cancer (2013) 132(5):1070–9. doi:10.1002/ijc.27759
Pubmed Abstract | Pubmed Full Text | CrossRef Full Text | Google Scholar
Keywords: γδT cell, IL17, Tγδ17, infection, inflammation, cancer
Citation: Patil RS, Bhat SA, Dar AA and Chiplunkar SV (2015) The Jekyll and Hyde story of IL17-producing γδT cells. Front. Immunol. 6:37. doi: 10.3389/fimmu.2015.00037
Received: 27 October 2014; Accepted: 20 January 2015;
Published online: 04 February 2015.
Edited by:
Julie Dechanet-Merville, Centre National de la Recherche Scientifique, FranceReviewed by:
Maria L. Toribio, Spanish Research Council (CSIC), SpainCopyright: © 2015 Patil, Bhat, Dar and Chiplunkar. This is an open-access article distributed under the terms of the Creative Commons Attribution License (CC BY). The use, distribution or reproduction in other forums is permitted, provided the original author(s) or licensor are credited and that the original publication in this journal is cited, in accordance with accepted academic practice. No use, distribution or reproduction is permitted which does not comply with these terms.
*Correspondence: Shubhada V. Chiplunkar, Chiplunkar Laboratory, Advanced Centre for Treatment, Research and Education in Cancer (ACTREC), Tata Memorial Centre, Sector 22, Navi Mumbai, Kharghar 410210, Maharashtra, India e-mail:c2NoaXBsdW5rYXJAYWN0cmVjLmdvdi5pbg==
Disclaimer: All claims expressed in this article are solely those of the authors and do not necessarily represent those of their affiliated organizations, or those of the publisher, the editors and the reviewers. Any product that may be evaluated in this article or claim that may be made by its manufacturer is not guaranteed or endorsed by the publisher.
Research integrity at Frontiers
Learn more about the work of our research integrity team to safeguard the quality of each article we publish.