- 1Advanced Biomedical Research Center, Dokuz Eylul University, Izmir, Turkey
- 2Department of Neuroscience, Health Science Institute, Dokuz Eylul University, Izmir, Turkey
- 3Department of Molecular Biology and Genetics, Izmir Institute of Technology, Urla, Turkey
Erythropoietin (EPO) is a neuroprotective cytokine, which has been applied in several animal models presenting neurological disorders. One of the proposed modes of action resulting in neuroprotection is post-transcriptional gene expression regulation. This directly brings to mind microRNAs (miRNAs), which are small non-coding RNAs that regulate gene expression at the post-transcriptional level. It has not yet been evaluated whether miRNAs participate in the biological effects of EPO or whether it, inversely, modulates specific miRNAs in neuronal cells. In this study, we employed miRNA and mRNA arrays to identify how EPO exerts its biological function. Notably, miR-451 and miR-885-5p are downregulated in EPO-treated SH-SY5Y neuronal-like cells. Accordingly, target prediction and transcriptome analysis of cells treated with EPO revealed an alteration of the expression of genes involved in apoptosis, cell survival, proliferation, and migration. Low expression of miRNAs in SH-SY5Y was correlated with high expression of their target genes, vascular endothelial growth factor A, matrix metallo peptidase 9 (MMP9), cyclin-dependent kinase 2 (CDK2), erythropoietin receptor, Mini chromosome maintenance complex 5 (MCM5), B-cell lymphoma 2 (BCL2), and Galanin (GAL). Cell viability, apoptosis, proliferation, and migration assays were carried out for functional analysis after transfection with miRNA mimics, which inhibited some biological actions of EPO such as neuroprotection, anti-oxidation, anti-apoptosis, and migratory effects. In this study, we report for the first time that EPO downregulates the expression of miRNAs (miR-451 and miR-885-5p) in SH-SY5Y neuronal-like cells. The correlation between the over-expression of miRNAs and the decrease in EPO-mediated biological effects suggests that miR-451 and miR-885-5p may play a key role in the mediation of biological function.
Introduction
Erythropoietin (EPO) is a hematopoietic cytokine that regulates erythropoiesis (1). Its primary production sites are the fetal liver and the adult kidney. Nonetheless, EPO is also produced by many non-hematopoietic tissues including the central nervous system (CNS). It has now been well established that different cell types of the CNS (neurons, glial cells, and endothelial cells) produce EPO (2). In addition to producing EPO, these cells also express the erythropoietin receptor (EPOR) on their plasma membranes thus underlining the crucial role that EPO plays in the physiological function of these cells (3).
Recombinant human erythropoietin (rhEPO) is a well-known FDA approved drug that has been used for the treatment of chronic anemia associated with renal failure, cancer, and prematurity since 1989 (1). It has been shown that EPO can cross the blood–brain barrier (BBB) via a receptor-mediated mechanism (4). EPO exerts remarkable neuroprotection for both, in vitro and in vivo models of nervous system disorders. In vitro studies showed that EPO is directly neuroprotective in hypoxic, hypoglycemic, and excitotoxic neuronal injury models (5). Exogenous administration of rhEPO protects the brain against neurodegeneration in a wide variety of experimental neurological disorders including stroke, neonatal hypoxic–ischemic encephalopathy, multiple sclerosis (MS), subarachnoid hemorrhage, traumatic brain injury, epileptic seizure, Parkinson’s disease (PD), Alzheimer’s disease (AD), and spinal cord injury (2, 6). EPO is currently being under investigation in several clinical trials regarding neuropsychiatric diseases (6).
Erythropoietin protects the CNS cells by limiting the production of tissue-injuring molecules such as reactive oxygen species (ROS) and glutamate, attenuation of apoptosis, modulation of inflammation, stimulation of angiogenesis, and induction of neurogenesis (7). However, the tissue-protective mechanisms of EPO are still not fully understood. EPO’s impact on regulation at both the transcriptional and the post-transcriptional levels may play a critical role for its cellular effects. EPO activates various signaling pathways that result in gene expression changes responsible for its biological activities (8). Previous in vitro (9) and in vivo (10–14) studies using mRNA microarrays evaluated genome-wide expression changes induced by EPO.
Some genes regulated by EPO may host microRNAs (miRNAs), which are then automatically regulated as well. This is then further propagated to the miRNA targets whose transcriptions generally increase with downregulation of its associated miRNAs. miRNAs are short, single stranded, RNA molecules that regulate gene expression at the post-transcriptional level (15). Since their discovery about two decades ago (16), they have spawned a great amount of interest and, thus the modulation of gene expression by miRNAs has been highly investigated. These small RNA molecules generally downregulate gene expression usually by binding to complementary sequences in their target mRNAs’ 3′ untranslated region. miRNAs are likely to be involved in most biological processes including development, cell cycle, apoptosis, proliferation, and angiogenesis (15). They have been demonstrated to be essential for brain development and function, and to influence the neuropsychiatric diseases (17). Therefore, it is reasonable to hypothesize that some miRNAs play roles in EPO-related neuroprotective mechanisms in neuronal cells. To date, there is only one study that indicates the importance of miRNAs for the biological effects of EPO on erythropoiesis (18). However, there is currently no study that demonstrates the contribution of miRNAs to the EPO-mediated neuroprotective mechanisms in neuronal cells.
The aim of this study is to determine global expression changes of miRNAs induced by EPO in vitro and the role of specific miRNAs in biological function of EPO in SH-SY5Y neuron-like cells. To achieve this, we have identified specific miRNAs via microarray analysis whose transcriptional levels were further validated by quantitative PCR (qPCR). EPO caused changes in the expression of specific miRNAs and their target genes involved in cell proliferation, migration, cell survival, and redox regulation. Moreover, these results were confirmed with functional studies by using miRNA mimics. Our results provide a new molecular insight into the cellular and molecular mechanism of EPO action in neuronal cells.
Materials and Methods
Cell Culture and Treatment
SH-SY5Y cells were maintained in Dulbecco’s Modified Eagle Medium: nutrient mixture F-12 (DMEM:F12) (Gibco, Gaithersburg, MD, USA) supplemented with heat-inactivated fetal bovine serum (10% v/v), l-glutamine (1% v/v), and penicillin-streptomycin (1% v/v) at 37°C in 5% CO2.
Twenty-four hours after initial plating of the SH-SY5Y cells, EPO was added at 1 U/ml concentration. Samples were collected after 24 h for quantification of miRNAs expression.
MicroRNA Extraction and Microarray Screening
Total RNA was isolated from EPO-treated and -untreated cells using the miRNeasy kit (Qiagen GmbH, Hilden) according to the manufacturer’s protocol. Microarray studies were carried out by FEBIT Company as described before (19). Samples were analyzed with a Geniom Real time Analyzer (GRTA, FEBIT GmbH, Heidelberg, Germany) using the Geniom miRNA Homo sapiens Biochip. Every array included 710 miRNAs and miRNA star sequences (seven replicates) which are annotated in the Sanger miRBase 20.0. The labeling of samples with biotin was performed by microfluidic-based enzymatic on-chip labeling of miRNAs. Hybridization was carried out for 16 h at 42°C, and the biochip was washed automatically. The image data were analyzed with the Geniom Wizard Software. The analyses included data background correction, normalization, and determination of differentially expressed miRNAs. Background correction was performed by subtracting the median of blank controls from median of each spots. Quantile normalization and variance stabilizing normalization were applied for normalization of the data across different arrays. The significant differentially expressed miRNAs were determined by an adjusted p value lower than 0.05 based on the multiple testing using the Benjamini–Hochberg procedures (20, 21).
Real-Time PCR Analysis of miR-451 and miR-885-5p
Quantitative PCR assay was used to confirm the miRNAs (miR-451 and miR-885-5p) that were found to be differentially regulated in the microarray experiment. Total RNA was isolated from cells using Qiagen miRNeasy Mini Kit and RNA quantity was measured with the NanoDrop Spectrophotometer (Thermo Scientific, USA). Then, 2 μg RNA was reverse-transcribed with a specific stem-loop primer using miScript II RT Kit (Qiagen, Valencia, CA, USA). Real-time PCR was performed using miScript SYBR Green PCR Kit on a Lightcycler 1.5 Real-Time PCR System (Roche Diagnostics, Germany). Primers for mature miR-451, miR-885-5p SNORD95, and U6 were purchased from Qiagen (Valencia, CA, USA). The PCR reactions were performed at 95°C for 10 min, followed by 40 cycles at 95°C for 15 s and at 60°C for 30 s. The specificity of the PCR products was analyzed by the melting curve analysis. The relative expression levels of miRNAs were normalized to the average amounts of U6 and SNORD95 snRNA using the 2−ΔΔCt formulation (22).
MicroRNA Target Prediction
miRWalk online prediction software1 was used for searching the validated and predicted targets of the altered miRNAs. miRWalk combines nine established target prediction tools: miRWalk, Diana-microT, miRanda, miRDB, PICTAR), PITA, RNA22, RNAhybrid, and Targetscan. Target prediction was performed separately for miR-451 and miR-885-5p with a p value <0.05. To establish the interaction network of the miRNAs with the Reactome2 pathways, only targets found in miRTarBase3 and Tarbase4 were accepted since they have experimental evidence.
mRNA Array
Microarray analysis was performed by Aros Applied Biotechnology (Denmark) using with the Human-6 v2 Expression BeadChip (Illumina Inc., San Diego, CA, USA). Briefly, total RNA isolated from SH-SY5Y cells treated with or without EPO, by using Qiagen miRNeasy Mini Kit. cDNA was synthesized from 500 ng total RNA, and then hybridized onto an Illumina Beadchip for 16 h at 58°C, then washed, stained, and scanned. Variance stabilization was performed using variance stabilization transformation and the data were normalized by quantile normalization. All the Illumina IDs (probes) were assumed to be differentially expressed if their ANOVA values were ≤0.5. All experiments were performed in triplicate and overall >98% correlation was observed between replicates.
Real-Time qPCR Array
Real-time qPCR array analysis was performed by Aros Applied Biotechnology (Denmark) using same RNA samples in microarray experiments for 26 differentially regulated genes in microarray analysis. Briefly, total RNA isolated from SH-SY5Y cells treated with or without EPO, by using Qiagen miRNeasy Mini Kit. cDNA was synthesized from 500 ng total RNA, then real-time PCR array analysis was performed in a total volume of 20 μl including 2 μl of cDNA, primers, and 10 μl of SYBR Green mix. Reactions were run on a Light cycler 480 using the universal thermal cycling parameters. Expression of target mRNA was normalized with respect to actin using the 2−ΔΔCt method.
Real-Time PCR Analysis of mRNAs
Total RNA was isolated using the Nucleospin RNA II Kit (Macherey-Nagel, Germany) and converted to cDNA with RevertAid First Strand cDNA Synthesis Kit (Thermo Fermentas, ABD). Real-time qPCR was performed using the Lightcycler 1.5 Instrument (Roche, ABD) and the SYBR-Green I kit, following the manufacturer’s instructions. The primers used in the qPCR reactions are listed in Table 1. PCR amplification of the template cDNAs were performed using following conditions for 40 cycles. After initial denaturation at 95°C for 10 min, temperature cycling of denaturation at 95°C for 10 s, annealing at 60°C for 10 s, and extension at 72°C for 20 s was performed. The specificity of the PCR products was analyzed by the melting curve analysis. The relative expression levels of mRNAs were quantified using the 2−ΔΔ Ct method with endogenous normalization to the average amounts of glyceraldehyde 3-phosphate dehydrogenase (GAPDH) and ribosomal protein, large, P0 (RPLP0).
WST-8 Assay
Cell viability was assessed by the ability of viable cells to reduce WST-8 reagent [2-(2-methoxy-4-nitrophenyl)-3-(4-nitrophenyl)-5-(2,4-disulfophenyl)-2H-tetrazolium, monosodium salt] to the highly water soluble formazan dye. Cells were seeded in 96-well plate at a density of 2 × 104 cells per well. After treatment with EPO and/or a toxic agent, 10 μl of WST-8 reagent was added to each well containing 100 μl of medium. The plate was then incubated for 4 h at 37°C and the absorbance of each well was measured on a microplate reader (Biotek, USA) at 450 nm with a reference wavelength of 630 nm. The relative cell viability was calculated as the percentage of untreated cells.
Trypan Blue Staining
Cell viability was also evaluated by trypan blue dye exclusion. Cells were seeded on a 24-well plate at a density of 5 × 105 cells per well. Following incubation, cells were stained with trypan blue stain for 1 min and fixed with 2% paraformaldehyde for 20 min. Stained cells were photographed by using an inverted microscope (Olympus CKX41, Japan).
DNA Fragmentation Assay
The Cell Death Detection ELISA Kit was used to evaluate DNA fragmentation in the cytoplasm of apoptotic cells. SH-SY5Y cells were seeded in a 96-well plate at a density of 2 × 104 cells per well. SH-SY5Y cells were pretreated with EPO (1 U/ml) for 24 h. Then toxic agents were added to the cell culture medium. Staurosporine (STS) was used at a concentration of 25 nM for 6 h; CoCl2 was used at a concentration of 250 μM for 24 h. Following treatment, cells were incubated in the lysis buffer for 30 min. Thereafter, the samples were centrifuged at 200×g for 10 min and 20 μl of the supernatant was transferred to a streptavidin coated microplate. Then the HRP-conjugated antibody cocktail was added to each well. After 2 h of incubation at room temperature, ABTS (2,2-azino-di-3-ethylbenzthiazoline sulfonate) was added to the wells. Subsequent to 15-min incubation, the plates were read at a wavelength of 405 nm in a microplate reader. The results are represented as fold increase in respect to optical density as compared with control cells.
Annexin-V Immunostaining
Annexin-V-FITC apoptosis detection kit I (Becton Dickinson, USA) was used to detect apoptotic cells. At the end of incubation, cells were stained with Annexin-V according to the manufacturer’s instructions. After staining procedure, cells were fixed with 4% paraformaldehyde for 20 min. Stained cells were photographed by using a fluorescence microscope (Olympus BX50, Japan).
Cell Proliferation Assay
SH-SY5Y cells were seeded in a 24-well plate at a density of 75 × 103 cells per well and treated with or without EPO. After 24 h of EPO treatment, cell culture plates were first washed three times with PBS, the cells then harvested by trypsinization and the cell number was determined using a hemocytometer.
Intracellular ROS Quantification
Intracellular ROS quantification was measured by 2′,7′-dichlorodihydrofluorescein, acetyl ester (CM-H2DCFDA) (Invitrogen). Cells were seeded into black 96-well plate at 2 × 104 cells per well and were treated with EPO for 24 h. Then, 100 μl PBS containing 10 μM CM-H2DCFDA was added to each well and incubated for 1 h. Afterward, CM-H2DCFDA was removed from each well and cells were treated with CoCl2 (250 μM) or both CoCl2 (250 μM) and EPO for 24 h. Fluorescence levels were determined following excitation with a wavelength of 492 nm at an emission wavelength of 527 nm by using a microplate reader (BioTek, USA).
Migration Assay
The SH-SY5Y cells were seeded at a density of 2 × 105 cells/well in 24-well plates, and incubated in DMEM/F12 containing 10% FBS for 24 h to confluence. A confluent monolayer of each well was scratched with a 200 μl pipette tip and cells were washed twice with PBS and replaced with fresh growth medium containing 1% FBS, with or without EPO. Immediately after the scratch (0 h) and at 24 h, four wounded area were marked in each well and images obtained using phase-contrast inverted microscope (Olympus, CKX41; 10×). The same wounded area was selected for the measurements at each time of study. The number of cells that migrated over the margins of the wounds was counted by using ImageJ 1.425. All experiments were performed in triplicate, and each experiment was repeated at least three times.
Neurit Outgrowth and Count
SH-SY5Y cells were transfected with miR-451 and miR-885-5p mimics and negative control oligomers as described before (Qiagen, USA). After transfection, cells were treated with 1 U/ml Epo for 24 h, then, cells were photographed by using an inverted microscope (Eclipse TS120, Nikon) using phase-contrast objectives with a 40× magnification.
For quantifying the number of neurites per cell, neurite-bearing cells were counted from at least three randomly selected microscopic fields with an average of 35 cells per field.
Additionally, neurite length was measured from all neurite-bearing cells and the length of each neurite was quantified by using ImageJ 1.425. Neurite lengths that counted from three different images and at least 30 neurites per condition were quantified. Independent cell culture experiments were conducted in triplicate.
Transfection of miRNAs Mimics
MicroRNA mimics and negative controls were purchased from Qiagen. SH-SY5Y cells were transfected 24 h after seeding in cell culture plates. Transfection of cells with miR-451 and miR-885-5p mimics and negative control oligomers (Qiagen) was performed using the HiPerFect transfection reagent (Qiagen) according to the manufacturer’s protocol. The final concentration of the mimics was 50 nM. Samples were collected after 48 h of miRNA mimic transfections for quantification of miRNA and target gene expression.
Statistical Analysis
Statistical analyses were performed with SPSS 18.0. Values represent the average ± SEM. Differences among averages were analyzed by Student’s t-test or Mann–Whitney U test. p values smaller than 0.05 were considered to be statistically significant. The correlation between the results of mRNA array and qPCR array was investigated by Pearson’s correlation analysis test.
Results
Profiling microRNA Expression Changes Following EPO Treatment
MicroRNA microarrays were used to identify miRNAs with altered expression due to treatment with EPO. SH-SY5Y cells were treated with 1 U/ml EPO for 24 h. Analysis of the microRNA expression using microRNA arrays showed that miR-451 and miR-885-5p expression decreased in response to treatment with EPO in SHSY-5Y cells according to the criteria of a fold change ≤−1.5 or ≥1.5 (P < 0.05) (Table S1 in Supplementary Material).
These results were validated by quantitative qPCR, using miR-451 and miR-885-5p specific primers, which recognize their mature forms. Consistent with the microarray results, both miR-451 and miR-885-5p were downregulated following 24 h of treatment with EPO (Figures 1A,B). In addition, 48 h EPO treatment also decreases expression of miR-451 and miR-885-5p in SH-SY5Y cells (Figures 1A,B).
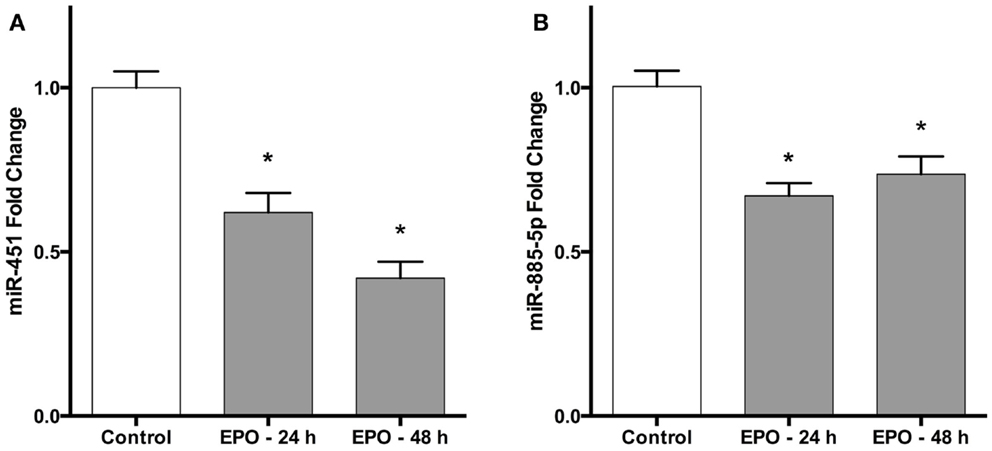
Figure 1. Erythropoietin decreases miR-451 and miR-885-5p expression in SH-SY5Y cells. Cells were treated with 1 U/ml EPO during 24 and 48 h. Expression levels of miR-451 (A) and miR-885-5p (B) in both treated and non-treated cells were quantified by qRT-PCR. EPO; erythropoietin. The data are presented as mean ± SE, n = 5 (*p < 0.05).
Target Gene Prediction
To search the validated and predicted targets for differentially expressed miRNAs miRWalk software1 was used. An mRNA was considered a potential target if it appeared in at least three of the algorithms utilized. Employing that criterion, we obtained 1831 predicted and 117 validated target genes for miR-451 and miR-885-5p.
MiRTarBase (23) and TarBase (24) are two databases, which contain miRNA targets with the restriction that each target must have experimental evidence. We therefore used these two databases to determine the targets for construction of a regulatory network intersected with Reactome pathways (25). miR-451 has Anti-Müllerian hormone (AMH), Calcium-binding protein 39 (CAB39), ATP-binding cassette sub-family B member 1 (ABCB1), AKT1, IMP2 inner mitochondrial membrane peptidase-like (IMMP2L), B-cell lymphoma 2 (BCL2), cAMP-regulated phosphoprotein 19 (ARPP19), Fructose-1,6-bisphosphatase 1 (FBP1), matrix metallopeptidase 9 (MMP9), myc, transmembrane emp24 protein transport domain containing 7 (TMED7), and ubiquitin-conjugating enzyme E2H (UBE2H) as confirmed targets. miR-885-5p has the experimentally supported targets cyclin-dependent kinase 2 (CDK2), and minichromosome maintenance complex component 5 (MCM5). While miR-451 is part of a lincRNA (ENSG00000264066), miR-885 is located in an intron of plasma membrane calcium-transporting ATPase 2 (ATP2B2). Overall, miR-451 has 10 targets, which are involved in 154 distinct reactome pathways (Figure 2; Table S2 in Supplementary Material). miR-885-5p has two confirmed targets, which are involved in 50 distinct reactome pathways (Figure 3; Table S3 in Supplementary Material).
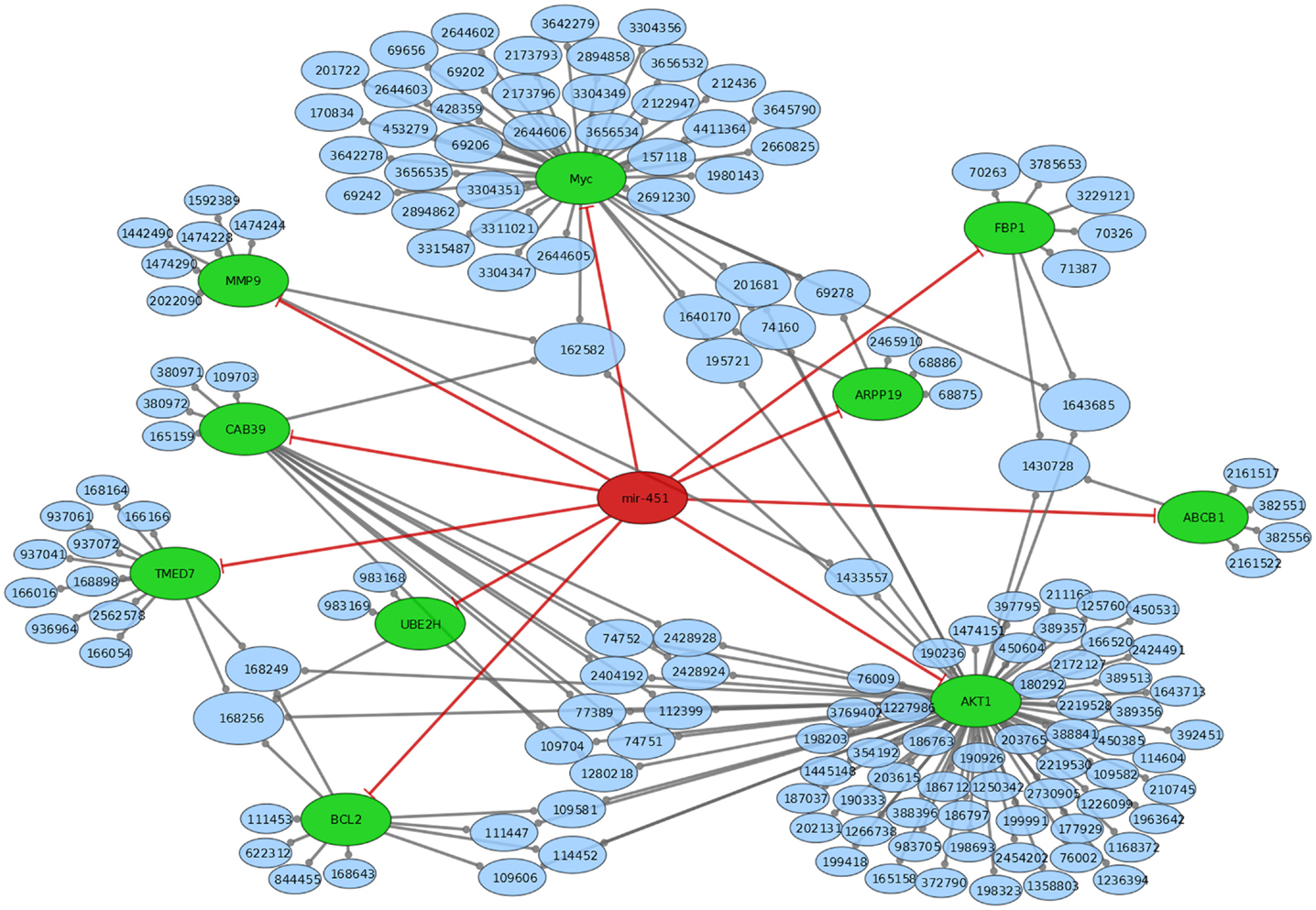
Figure 2. Mir-451 comes from a link RNA (not shown in graph). Mir-451 has 10 known targets (green). These targets are part of a number of reactome pathways (blue). The number in the blue nodes can be used to access the associated reactome pathway, and Table S3 in Supplementary Material provides further information for clarity. Size of blue nodes reflects the number of mir-885 targets it contains. Edges ending in circles (gray) indicate a part of relationship (e.g., FBP1 is a part of the “glycogen storage diseases” pathway, which has the reactome accession number 3229121). Edges ending in straight lines (red) indicate inhibition of the target (e.g., mir-451 inhibits AKT1).
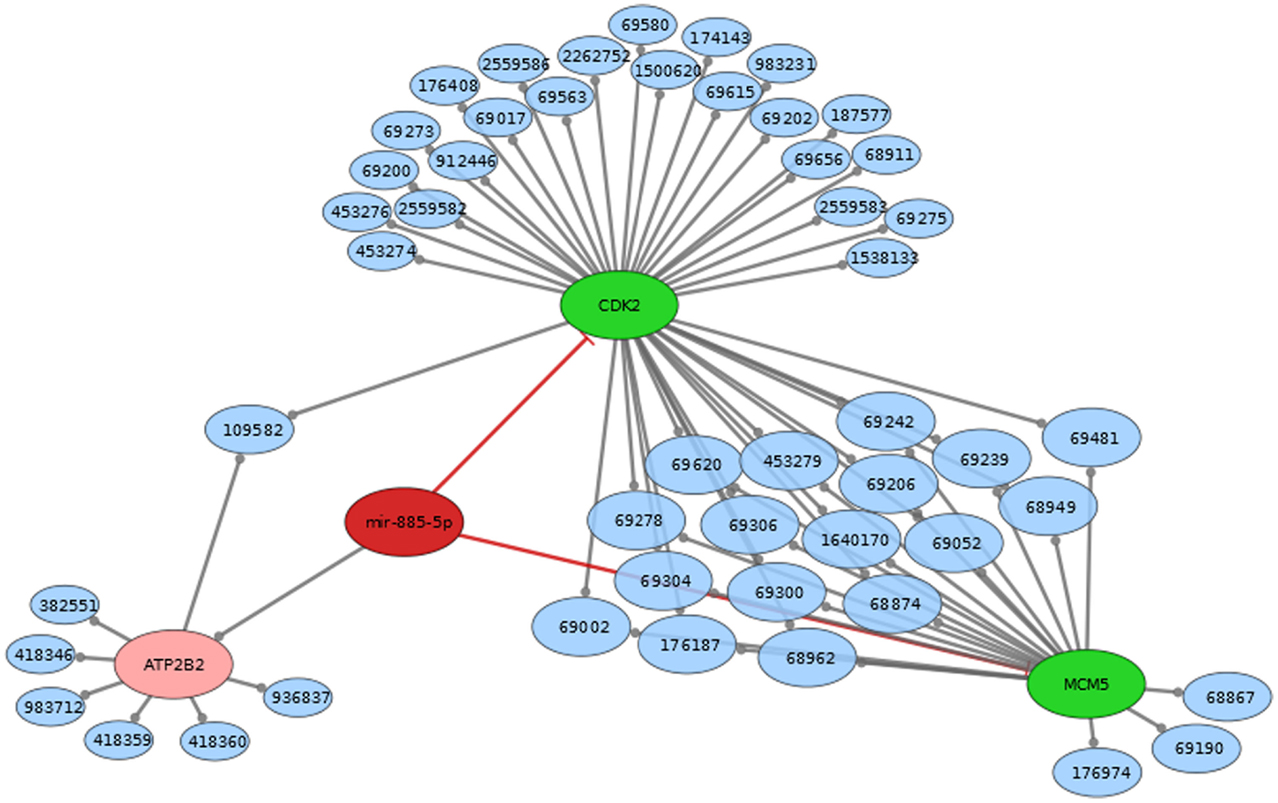
Figure 3. Mir-885 comes from an intron in ATP2B2 (both red). Mir-885 has two known targets (green). These targets are part of a number of reactome pathways (blue). The Number in the blue nodes can be used to access the associated reactome pathway and Table S2 in Supplementary Material provides further information for clarity. Size of blue nodes reflects the number of mir-885 targets it contains. Edges ending in circles (gray) indicate a part of relationship (e.g., CDK2 is a part of the “Activation of the pre-replicative complex” pathway, which has the reactome accession number 68962). Edges ending in straight lines (red) indicate inhibition of the target (e.g., mir-885 inhibits MCM5).
Profiling mRNA Expression Changes Following EPO Treatment
We performed high-density oligonucleotide microarray analyses (n = 3) to determine the changes in gene expression induced by EPO in SH-SY5Y cells. Our data demonstrate EPO treatment upregulated 51 genes and downregulated 23 genes considering a fold change >1.5 (p value <0.05) (Tables 2 and 3).
qPCR Array
We used qPCR array to validate gene expression changes of 26 genes. Aliquots of the same RNA sample were used for all the experiments in both the microarray and qPCR array measurements. Figure 4 shows that the qPCR findings were highly correlated with the microarray data (Pearson’s R = 0.95, p = 0.000). Data from the mRNA microarray experiment are shown in Tables 2 and 3 and qPCR results are shown in Tables S4 and S5 in Supplementary Material.
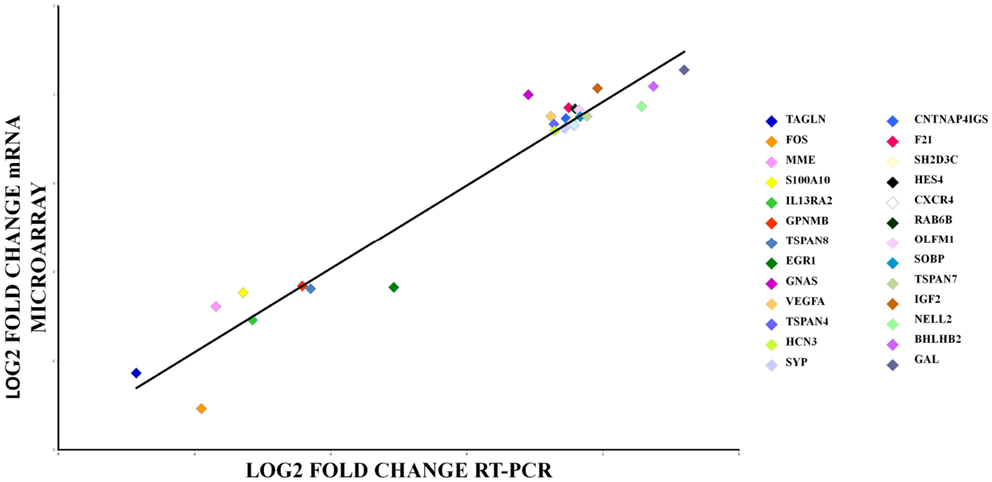
Figure 4. qRT-PCR array validation of the microarray data for the selected genes. The RT-PCR data were normalized to the housekeeping gene β-actin. Pearson’s coefficient, r = 0.95, p = 0.000.
qPCR Analysis of Selected Target Genes at Different Time Point of EPO Treatment
We examined the gene expression levels of these miRNA target genes at different time points after EPO treatment. We found that EPO upregulates matrix metallo peptidase 9 (MMP9), B-celllymphoma 2 (BCL2), EPOR, and Galanin (GAL) expression at 24 and 48 h, vascular endothelial growth factor A (VEGFA), mini chromosome maintenance complex 5 (MCM5) at 48 h, and cyclin-dependent kinase 2 (CDK2) expression at 3 h (Table 4; Figure 5).
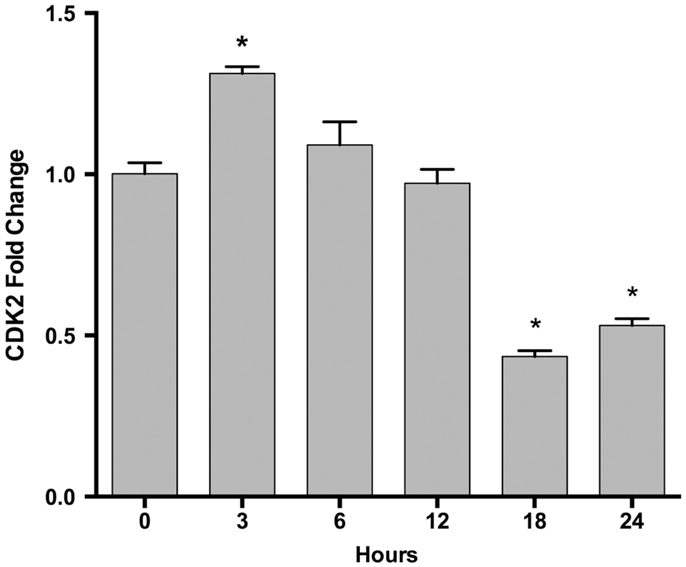
Figure 5. Time-dependent CDK2 gene expression changes following EPO treatment in SH-SY5Y cells. Cells were treated with 1 U/ml EPO during 0–24 h time intervals. Expression levels of CDK2 were quantified by qRT-PCR. CDK2; cell cycle dependent kinase 2. The data are presented as mean ± SE, n = 5 (*p < 0.05).
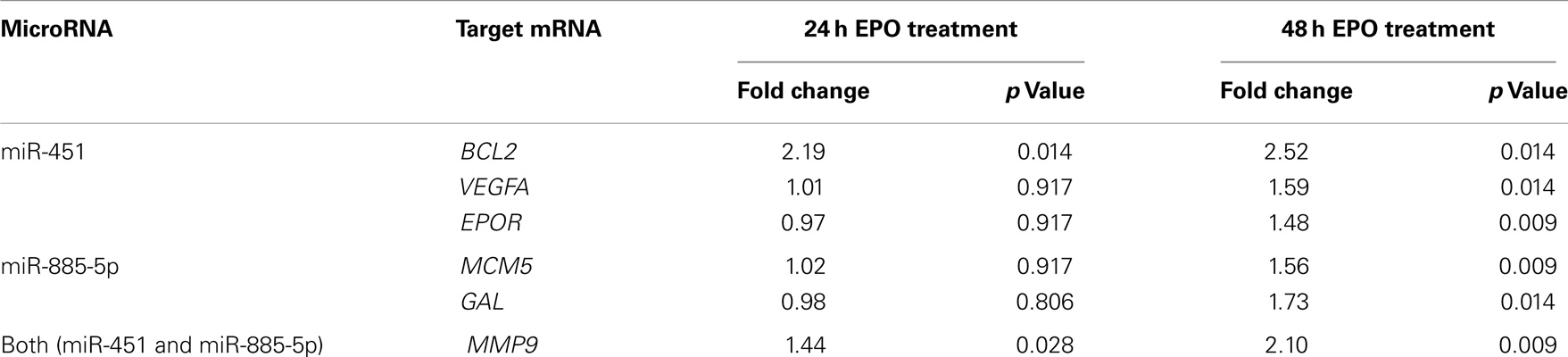
Table 4. Selected target mRNA expression changes of miR-451 and miR-885-5p in EPO-treated SH-SY5Y cells.
Upregulation of miR-451 and miR-885-5p Expression Can Inhibit Their Target Gene Expression
To determine whether differentially expressed miRNA can regulate their target genes’ expression in SH-SY5Y cells, we investigated the modulation of MMP9, BCL2, VEGFA, EPOR, MCM5, and GAL mRNA levels in cells transfected with mimics of miR-451 and miR-885-5p by qPCR. The result of real-time qPCR revealed that miR-451 and miR-885-5p mimics can significantly decrease the basal expression of MMP9, BCL2, VEGFA, EPOR, MCM5, and GAL, suggesting that mimics efficiently introduced into the cells and decreased the expression of their target genes (Figure 6A).
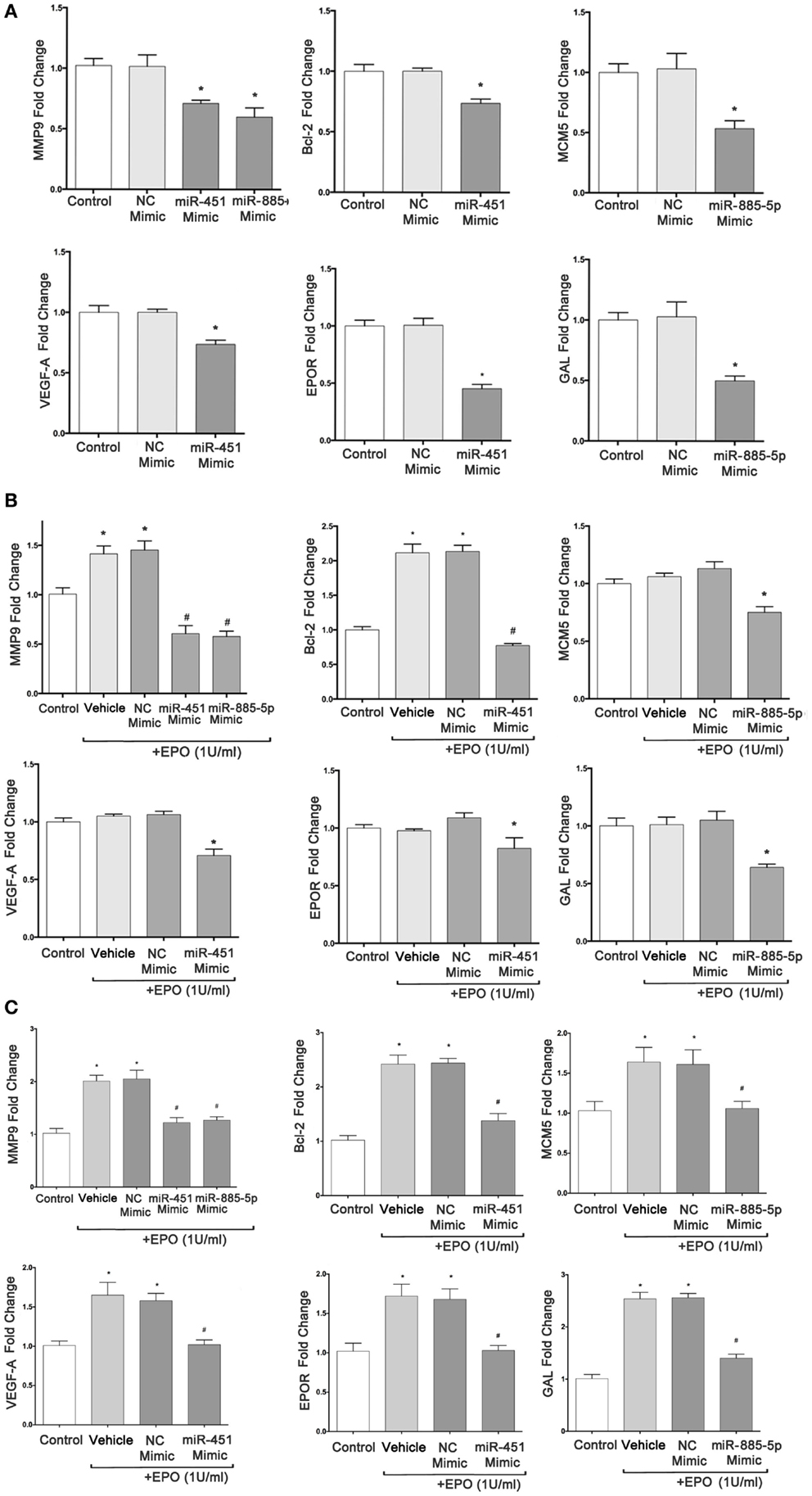
Figure 6. miR-451 and miR-885-5p significantly downregulate expression of target genes in SH-SY5Y cells. qRT-PCR was performed to determine the mRNA levels of target genes after transfection of SH-SY5Y cells with miR-451, miR-885-5p mimics, and negative control (NC) miRNAs without EPO treatment (A), with 24 h EPO treatment (B) and 48 h EPO treatment (C). Control: cells without any transfection. The data are presented as mean ± SE, n = 5 (*p < 0.05).
We found that cells transiently transfected with miR-451 and miR-885-5p mimics had significantly reduced EPO-induced target genes expression at 24 h (MMP9, BCL2) and at 48 h (MMP9, BCL2, VEGFA, EPOR, MCM5, and GAL) (Figures 6B,C).
Downregulation of miR-451 and miR-885-5p Contributes to the Neuroprotective Effects of EPO
To evaluate the role of miR-451 and miR-885-5p in the protective effect of EPO, SH-SY5Y cells were transfected with mimics to investigate the effect of over-expression of these miRNAs. The EPO responsiveness in respect to cell death induced by CoCl2 was examined with the WST-8 assay. Cell viability significantly decreased with CoCl2, which can be reversed by EPO pretreatment. After upregulation of miR-451 and miR-885-5p levels with mimics, the cell viability levels in EPO-treated cells did not reach the levels in the negative control mimic group (Figures 7A–C). This finding suggests that the protective effect of EPO was in part dependent on the expression levels of miR-451 and miR-885-5p.
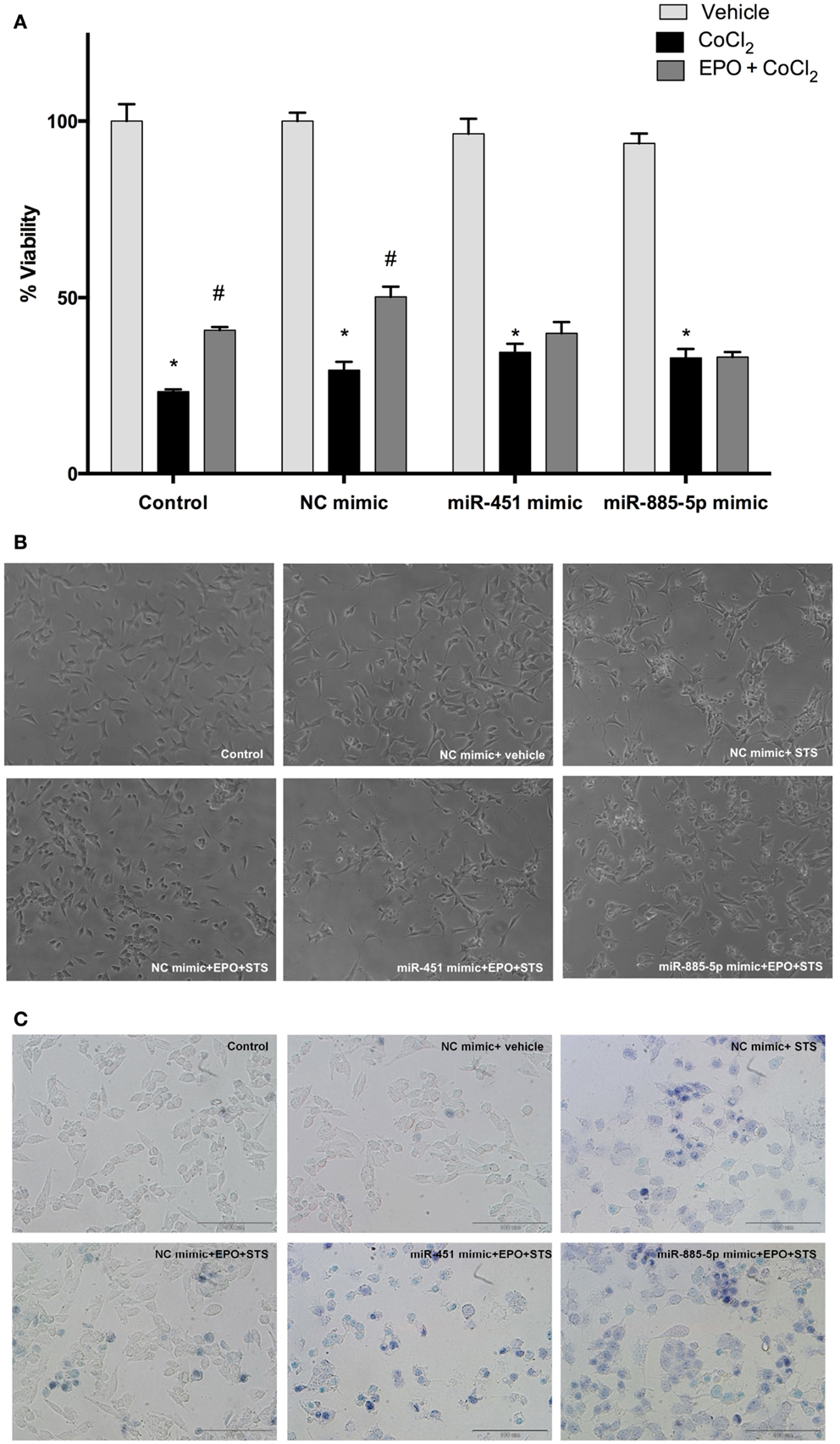
Figure 7. miR-451 and miR-885-5p may mediate the neuroprotective effect of EPO. Transfected and non-transfected cells were treated with EPO and/or CoCl2. (A) Cell viability was analyzed by WST-8 assay, (B) representative phase-contrast microcopy images of SH-SY5Y cells, and (C) representative light microscopy images showing trypan blue stained dead cells. Over-expression of miR-451 and miR-885-5p reverse the neuroprotective effect of EPO induced by CoCl2. CoCl2; cobalt chloride, EPO; erythropoietin. The data are presented as mean ± SE, n = 5 (*p < 0.05 compared to control and #p < 0.05 compared to CoCl2 treated cells).
Over-Expression of miR-451 and miR-885-5p Can Reverse Anti-Apoptotic Effect of EPO
Previous findings indicated that EPO inhibits apoptosis induced by STS. Here, we investigated the effects of EPO on apoptosis of SH-SY5Y cells following an increase in miR-451 and miR-885-5p levels upon transfection with mimics.
Cells were transfected with mimics to simulate over-expression of miR-451 and miR-885-5p. As expected, EPO effects on miR-451 and miR-885-5p expression were diminished in cells transfected with mimics (Figures 8A,B). Consequently, EPO-mediated anti-apoptotic effect was significantly reduced in the mimics transfected cells as compared with cells that were transfected with control mimics. This indicates the importance of miR-451 and miR-885-5p in mediating the anti-apoptotic effect of EPO. We also found that there is an impact of miR-451 and miR-885-5p on EPO’s anti-apoptotic effects in response to CoCl2 induced apoptosis (Figure 8C).
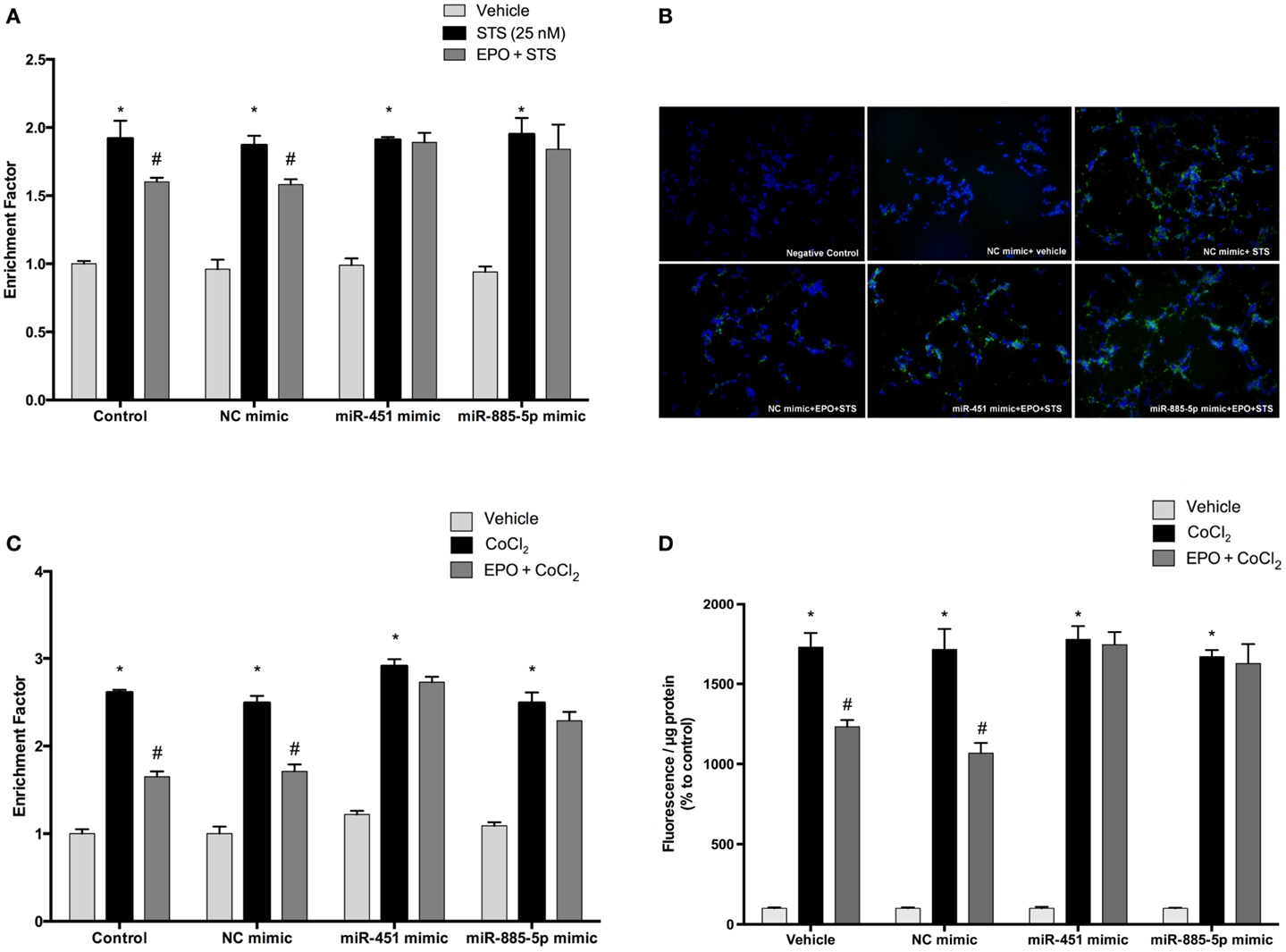
Figure 8. Anti-apoptotic and anti-oxidant effects of EPO in SH-SY5Y cells may be regulated by miR-451 and miR-885-5p. Transfected and non-transfected cells were treated by staurosporine (STS) (25 nM) and 1 U/ml EPO. (A) DNA fragmentation was evaluated by cell death ELISA assay. (B) Apoptotic phosphatidylserine (PS) positive cells were stained by Annexin-V-FITC dye and visualized using immunofluorescence microscopy. Cells were also treated with CoCl2 (250 μM) and 1 U/ml EPO (C) and DNA fragmentation was analyzed by cell death ELISA assay. Transfection of miR-451 and miR-885-5p mimics reduced the anti-apoptotic effect of EPO in both STS and CoCl2 induced apoptosis. (D) Intracellular ROS production was quantified by using the CM-H2DCFDA method. Over-expression of miR-451 and miR-885-5p reversed anti-oxidant effect of EPO induced by CoCl2. STS; staurosporin, EPO; erythropoietin, CM-H2DCFDA; 2′,7′-dichlorodihydrofluorescein, acetyl ester. The data are presented as mean ± SE, n = 5 [*p < 0.05 compared to control and #p < 0.05 compared to toxic agents (STS or CoCl2) treated cells].
Over-Expression of miR-451 and miR-885-5p Can Reverse the Anti-Oxidant Effect of EPO
In order to evaluate the role of miR-451 and miR-885-5p for the anti-oxidant effect of EPO, we investigated the effects of EPO on ROS production induced by CoCl2 in SH-SY5Y cells after transfection with mimics. As shown in Figure 8D, the anti-oxidant effect of EPO was attenuated by mimics of miR-451 and miR-885-5p. Our results indicate the importance of miR-451 and miR-885-5p in mediating the anti-oxidant effect of EPO.
Upregulation of miR-451 and miR-885-5p Affects Proliferation of SH-SY5Y Cells Enhanced by EPO
Cell proliferation was evaluated after transfection with mimics to determine the impact of miR-451 and miR-885-5p on the proliferative effect of EPO. According to our results, EPO increases proliferation of SH-SY5Y cells at 24 and 48 h after treatment (Figures 9A,B). We found that cells transiently transfected with miR-451 and miR-885-5p mimics had significantly reduced proliferation as compared with cells transfected with control mimics.
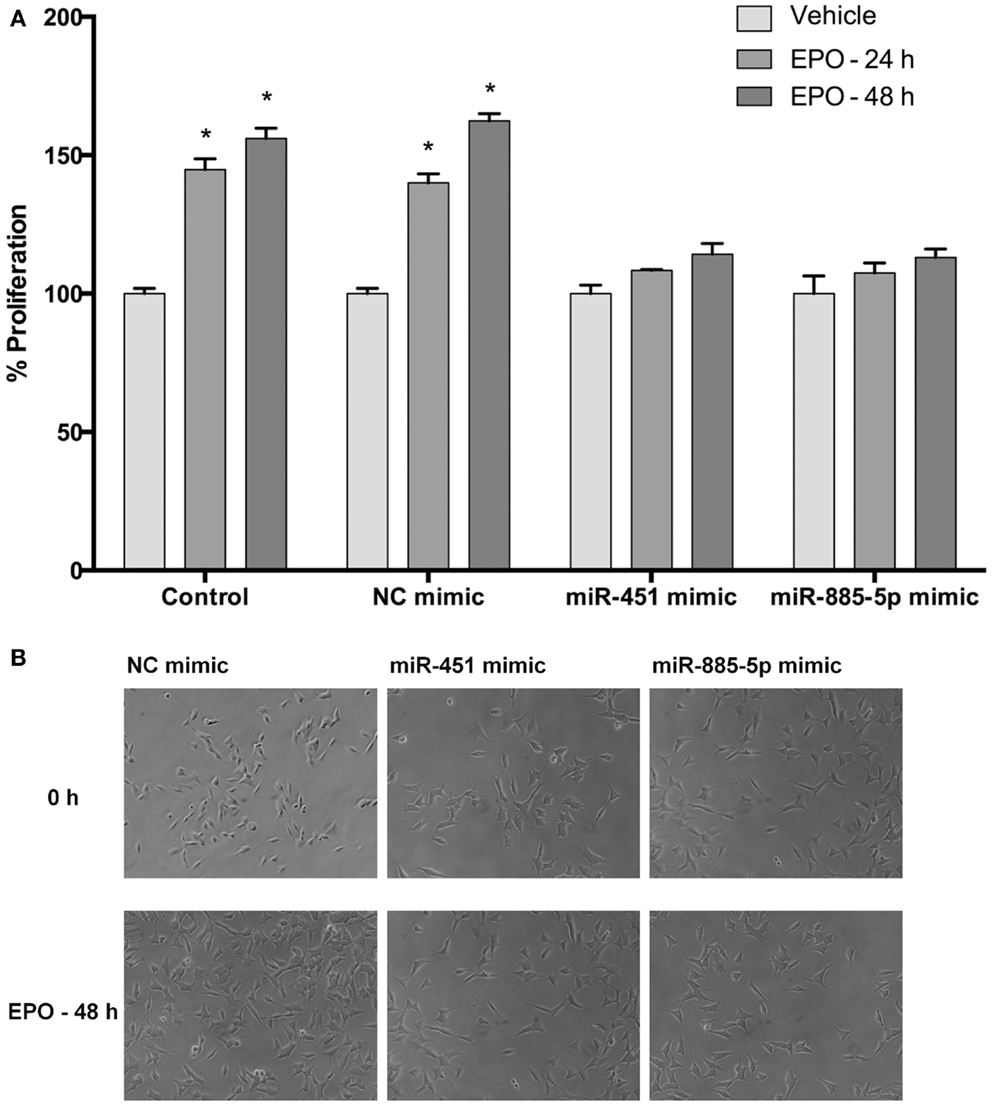
Figure 9. Upregulation of miR-451 and miR-885-5p reduces proliferation of SH-SY5Y cells enhanced by EPO. In miRNA mimic transfected and non-transfected cells, cell proliferation was observed by counting cells using phase-contrast microscopy following either 24 or 48 h EPO treatment. (A) Cell counts and (B) phase-contrast microscopy images. Transfection of miR-451 and miR-885-5p mimics reduces the proliferative effect of EPO. EPO; erythropoietin. The data are presented as mean ± SE, n = 5 (*p < 0.05).
Downregulation of miR-451 and miR-885-5p Contributes to the Neuronal Migratory Effect of EPO
We further investigated the role of miR-451 and miR-885-5p on the migratory effects of EPO. SH-SY5Y cells were transfected with miR-451 and miR-885-5p mimics or control miRNAs for 48 h and then EPO responsiveness on cell migration was examined with scratch assay. We found that the EPO-mediated migratory effect was significantly reduced in the mimics transfected cells as compared with cells that were transfected with control mimics (Figures 10A,B).
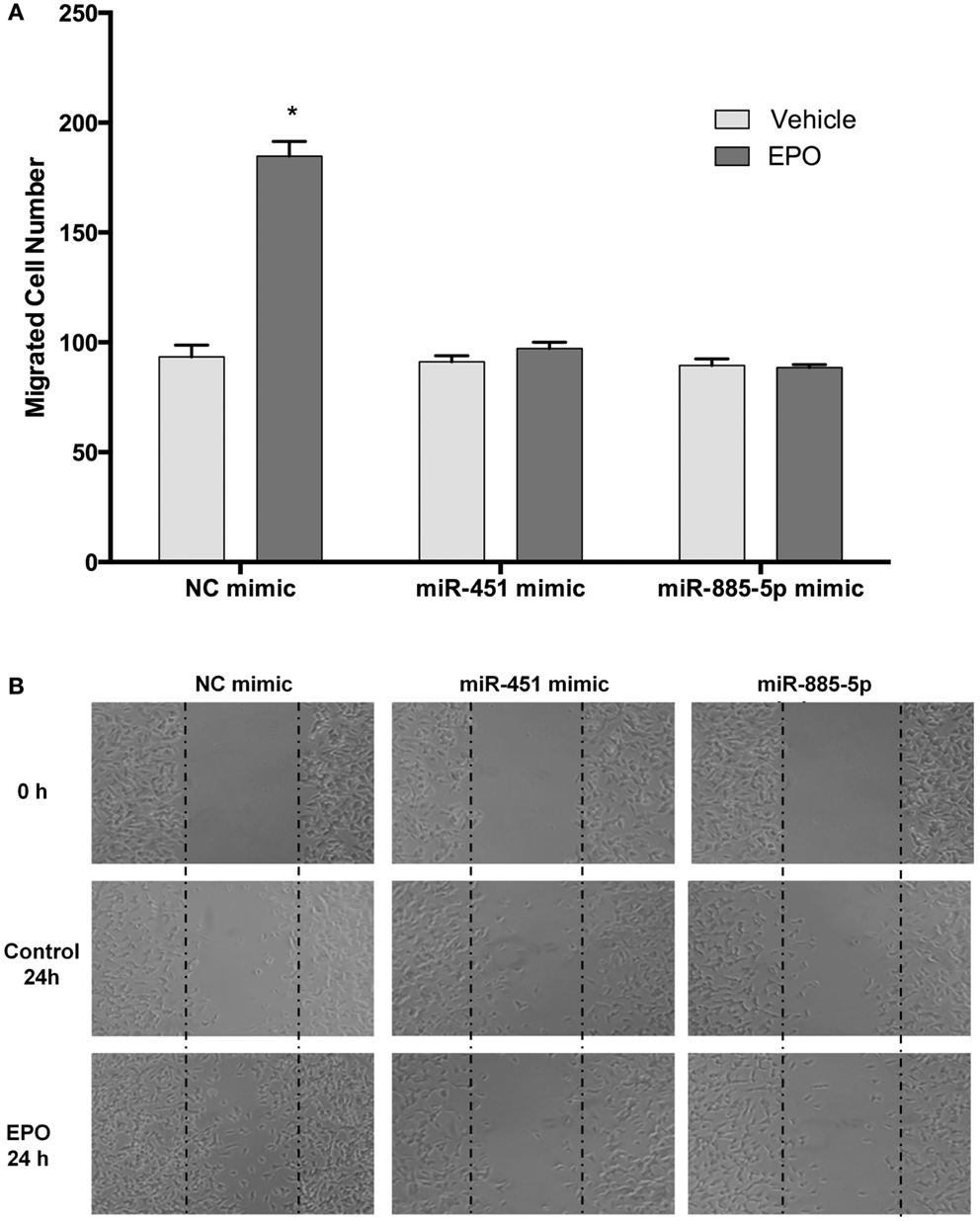
Figure 10. EPO induces migration in SH-SY5Y cells, which can be reversed by mir-451 and mir-885-5p. Transfected and non-transfected cells were treated with 1 U/ml EPO and migration assay was conducted for either condition. (A) Cells were counted by using ImageJ 1.42 (http://imagej.nih.gov/ij/). (B) Representative images of migrated SH-SY5Y cells. Over-expression of miR-451 and miR-885-5p reverse the migratory effect of EPO. EPO, erythropoietin. The data are presented as mean ± SE, n = 3 (*p < 0.05).
Effects of miR-451 and miR-885-5p Upregulation on EPO-Mediated Neurite Outgrowth and Neurite Length in SH-SY5Y Cells
To investigate the role of miR-451 and miR-885-5p upregulation on EPO-mediated neurite outgrowth in SH-SY5Y cells, we used mimics to simulate over-expression of miR-451 and miR-885-5p. EPO significantly increased neurite outgrowth to 69.5 ± 5.3% relative to controls and the increase remained unchanged in negative control mimics transfected cells (73.4 ± 3.9%). However, the increase due to EPO treatment in the percentage of neurite-bearing cells was significantly attenuated in those cells transfected with mimics (Figures 11A,B).
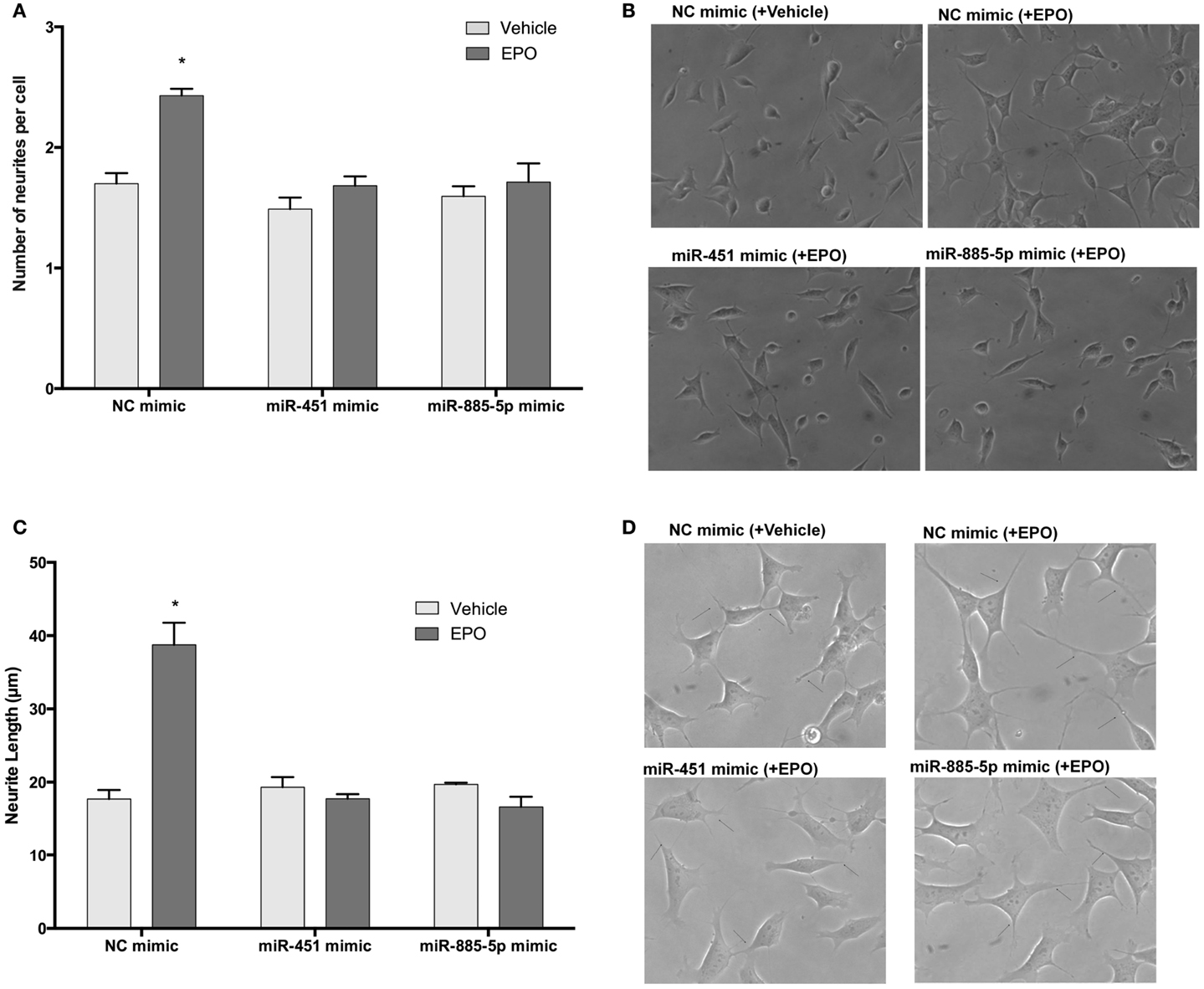
Figure 11. EPO may enhance neurite outgrowth and length via regulation of miR-451 and miR-885-5p. Transfected and non-transfected cells were treated with 1 U/ml EPO. (A) Neurite cell number was counted using ImageJ 1.42 (http://imagej.nih.gov/ij/). Randomly selected fields in microscopic analysis with an average of 35 cells per field were analyzed. (B) Representative phase-contrast microscopy images are shown in order to indicate the increased neurite numbers induced by EPO and reversed by the transfection of mimics of miR-451 and miR-885-5p. (C) The average maximal neurite length for both, the transfected and non-transfected cells were analyzed by ImageJ 1.42 (http://imagej.nih.gov/ij/). At least 30 neurites per condition were measured. (D) Neurite length distribution. Arrowheads indicate the neurites arising from cells. Scale bar, 100 μm. EPO, erythropoietin. The data are presented as mean ± SE, n = 5 (*p < 0.05).
Similar results were obtained for neurite length analysis. EPO increases neurite length of SH-SY5Y cells after 24 h treatment (Figures 11C,D). We found that cells transiently transfected with miR-451 and miR-885-5p mimics had significantly reduced neurite length compared with that of cells transfected with control mimics. These results show that the miR-451 and miR-885-5p are involved in the induction of neurite outgrowth in SH-SY5Ycells.
Discussion
Erythropoietin is a well-known cytokine growth factor, which exerts pleiotropic effects including neurotrophic, neuroprotective, anti-apoptotic, anti-oxidative, and immune-modulatory activities. For instance, EPO inhibits pro-inflammatory cytokine release and T cell responses in experimental autoimmune encephalomyelitis (EAE) (26, 27). Proposed mechanisms of neuroprotective effects of EPO include gene expression regulation at the post-transcriptional level including alteration of miRNA expression. Here, we analyzed the effect of EPO on miRNA expression in SH-SY5Y neuronal-like cells. MicroRNA microarray experiments showed that miR-451 and miR-885-5p were downregulated by 24 h EPO treatment. Real-time qPCR studies verified the miRNA microarray results.
In this in vitro study, we chose SH-SY5Y human neuroblastoma cells as cell culture model because of their high transfection efficiency. SH-SY5Y cells express EPOR and other tissue-protective EPO receptors such as CD131 (26). In addition, cellular and molecular actions of EPO have been widely studied in this model such as proliferative, anti-apoptotic, anti-oxidant, anti-inflammatory, and neurite outgrowth promoting effects (28–30).
In this study, we demonstrated, for the first time, that select miRNAs are regulated by EPO in neuronal SH-SY5Y cells. Previously, only one study evaluated the effect of EPO on the miRNA expression profile in murine erythroid cells (18). In that study, miR-210, miR-188, and miR-362 were significantly elevated with stimulation of EPO. miR-210 has been demonstrated to be a neuroprotective miRNA against oxygen-glucose deprivation in PC12 cells (31). This miRNA is well known to stimulate angiogenesis and neurogenesis (32). As activity-regulated miRNA, miR-188 shows neurotrophic effects in rat hippocampal neurons (33). We could not detect any change in miR-210 and miR-188 expression profiles in EPO-treated SH-SY5Y cells. This discrepancy may be due to species and cell type differences. Another plausible explanation could be the use of different exposure duration and dose of EPO. In our hand, EPO dose of one unit did not show any change in the expression of miR-210 in time course qPCR experiments (3–48 h, data not shown). Our ongoing studies, especially, focus on whether miR-210 mediates the promoting effect of EPO on angiogenesis and neurogenesis in neural progenitor and cerebral endothelial cells.
We performed mRNA microarray analysis to evaluate mRNA changes in EPO-treated SH-SY5Y cells. EPO treatment in SH-SY5Y neuronal cells upregulated 51 genes and downregulated 23 genes. EPO caused changes in the expression of genes involved in cell proliferation, cell survival, and cell differentiation. There are some contradictory and similar results as compared with previous EPO mRNA array studies in the literature. The discrepancies that discussed above in detail may be due to technical limitations that also interfere with miRNA expression profiling results. Although the comparison of in vitro and in vivo findings may be somewhat problematic, the results of a previous in vivo study by Anderson et al. are comparable with our transcriptome profiling (14). They found that chemokine (C–X–C Motif) receptor 4 (CXCR4), Gal, and IGF2 upregulated at different time points, 24 h–7 days, in the brains of EPO-treated rats. In another experimental rodent infarct model, CXCR4 was found significantly upregulated in EPO-treated rats (34). Although VEGFA upregulation has not been reported in previous mRNA array studies, we found that EPO increased VEGFA expression by qPCR. As a neuroprotectant, neurotrophic, and angiogenic molecule, VEGFA may mediate the effects of EPO in the nervous system (35). Conversely, EPO-mediated VEGFA gene regulation may proceed via downregulation of miR-451, as revealed by our mimic transfection study. Gal, which is a target of miR-885-5p has neuroprotective, neurotrophic, and immunomodulatory effects (36). Future studies using Gal siRNA or GAL receptor blockers may delineate the Gal gene regulation mechanisms induced by EPO. Using cultured rat PC12 cells or rat B104 neuroblastoma cells, other groups found upregulation in anti-apoptotic genes, BCLXL and BCL2, respectively (9, 37). We also found an increase in BCL2 expression by qPCR. We also confirmed the finding that EPO-mediated EPOR expression in SH-SY5Y cells (28, 38). In addition, we also studied cell cycle regulating genes, MCM5 and CDK2 that are targets of miR-885-5p (39). EPO significantly increased their expression and mimics of miR-885-5p reversed the resulting effect, suggesting that EPO regulates cell cycle genes in an miRNA-dependent manner. As validated targets of EPO downregulated miRNAs (miR-451 and miR-885-5p), MMP9 may mediate neuronal migratory and angiogenic effects of EPO.
In the current study, we choose a single time point for mRNA assay. In the literature, some EPO-induced genes such as BCLXL are upregulated as early as at 3 h and the expression of others peaked at a very late time point such as 7 days (MMP9) (9, 14). Thus, we might fail to detect possible expression changes of some EPO-regulated genes such as CDK2. As a powerful technique and gold standard method, qPCR may detect tiny expression changes as revealed in our study. In contrast to mRNA array, qPCR showed upregulation of some EPO-regulated genes (BCL2, EPOR, and MCM5) at 48 h.
We found some genes whose expressions are upregulated by EPO in our mRNA microarray study: IGF2 (neuroprotection), CXCR4 (neural migration, immunomodulation, neuroprotection), and synaptophysin (synaptogenesis). Since differentially regulated miRNAs, miR-451 and miR-885-5p, did not target those genes, we did not focus on miRNA-mediated regulation of them in this study. Future studies may provide insight on the roles of these miRNA–mRNA interactions on the effects of EPO. As shown in non-neuronal cells, miRNAs may be both regulators and mediators of EPO’s action. For instance, miR-125b downregulates EPO and EPOR expression in breast MCF7 cancer cell lines (40). In another study, brain enriched miRNA, miR-132 downregulated the expression of EPO via the inhibition of Nrf2 transcription factor in rat epithelial cells (41).
In this study, we did not focus on the possible mechanism of EPO-mediated miRNA regulation. EPO modulates various signaling pathways and transcription factors and may use these mechanisms to regulate miRNA expression (8). Another interesting point is the differences between EPO and its variant carbamylated EPO (42). First, the effects of EPO variants on miRNA expression profiling should be studied. Then supporting functional studies should be performed using mRNAs mimics and antagomirs. EPO exerts similar cytoprotective effects in non-neuronal cells of nervous system. Thus, the results of miRNA expression profiling studies using cultured astrocytes, microglia, oligodendroglia, and cerebral endothelial cells will also be informative. Here, the expression of pri- and pre-forms of miRNAs was not studied and only mature forms were evaluated. Such studies will give more information on the molecular level(s) in which EPO modulates specific miRNAs. Another issue that should be addressed in future studies is the detection of exosomal miRNAs, especially miR-451, in the EPO-treated supernatant of cultured cells (43, 44).
The extrapolation of in vitro results to in vivo status is not without limitation. Thus, experimental animal studies should also be designed and performed using mimics or antagomirs of selected miRNAs. Complementary behavioral, electrophysiological, and bioinformatics analyses are also a mandatory part of in vivo studies combined miRNAs in situ hybridization/immunohistochemical analyses or sophisticated techniques such as laser captured microdissection microscopy will help to reveal miRNAs expression changes at the cellular level.
In conclusion, to the best of our knowledge, this is the first integrative miRNA–mRNA study using EPO. Such studies will uncover the mechanism of cellular and molecular actions of EPO at different regulation steps. Future studies focusing on miRNA-mediated mechanisms may provide novel insight on how biological effects of EPO are modulated.
Conflict of Interest Statement
The authors declare that the research was conducted in the absence of any commercial or financial relationships that could be construed as a potential conflict of interest.
Acknowledgments
The research was supported by grants from the Dokuz Eylul University (2009-KB-SAG-46 and 2009-KB-SAG-78).
Supplementary Material
The Supplementary Material for this article can be found online at http://www.frontiersin.org/Journal/10.3389/fimmu.2014.00475/abstract
Footnotes
References
1. Jelkmann W. Physiology and pharmacology of erythropoietin. Transfus Med Hemother (2013) 40(5):302–9. doi: 10.1159/000356193
2. Kumral A, Tüzün F, Oner MG, Genç S, Duman N, Ozkan H. Erythropoietin in neonatal brain protection: the past, the present and the future. Brain Dev (2011) 33(8):632–43. doi:10.1016/j.braindev.2010.10.014
3. Ogunshola OO, Bogdanova AY. Epo and non-hematopoietic cells: what do we know? Methods Mol Biol (2013) 982:13–41. doi:10.1007/978-1-62703-308-4_2
4. Brines ML, Ghezzi P, Keenan S, Agnello D, de Lanerolle NC, Cerami C, et al. Erythropoietin crosses the blood-brain barrier to protect against experimental brain injury. Proc Natl Acad Sci U S A (2000) 97(19):10526–31. doi:10.1073/pnas.97.19.10526
5. Genc S, Koroglu TF, Genc K. Erythropoietin and the nervous system. Brain Res (2004) 1000(1–2):19–31. doi:10.1016/j.brainres.2003.12.037
6. Sargin D, Friedrichs H, El-Kordi A, Ehrenreich H. Erythropoietin as neuroprotective and neuroregenerative treatment strategy: comprehensive overview of 12 years of preclinical and clinical research. Best Pract Res Clin Anaesthesiol (2010) 24(4):573–94. doi:10.1016/j.bpa.2010.10.005
7. Maiese K, Chong ZZ, Shang YC, Wang S. Erythropoietin: new directions for the nervous system. Int J Mol Sci (2012) 13(9):11102–29. doi:10.3390/ijms130911102
8. Chong ZZ, Shang YC, Mu Y, Cui S, Yao Q, Maiese K. Targeting erythropoietin for chronic neurodegenerative diseases. Expert Opin Ther Targets (2013) 17(6):707–20. doi:10.1517/14728222.2013.780599
9. Renzi MJ, Farrell FX, Bittner A, Galindo JE, Morton M, Trinh H, et al. Erythropoietin induces changes in gene expression in PC-12 cells. Brain Res Mol Brain Res (2002) 104(1):86–95. doi:10.1016/S0169-328X(02)00323-6
10. Juul SE, McPherson RJ, Bammler TK, Wilkerson J, Beyer RP, Farin FM. Recombinant erythropoietin is neuroprotective in a novel mouse oxidative Injury model. Dev Neurosci (2008) 30(4):231–42. doi:10.1159/000110348
11. Juul SE, Beyer RP, Bammler TK, McPherson RJ, Wilkerson J, Farin FM. Microarray analysis of high-dose recombinant erythropoietin treatment of unilateral brain injury in neonatal mouse hippocampus. Pediatr Res (2009) 65(5):485–92. doi:10.1203/PDR.0b013e31819d90c8
12. Maurer MH, Frietsch T, Waschke KF, Kuschinsky W. Cerebral transcriptome analysis of transgenic mice overexpressing erythropoietin. Neurosci Lett (2002) 327(3):181–4. doi:10.1016/S0304-3940(02)00425-1
13. Mengozzi M, Cervellini I, Villa P, Erbayraktar Z, Gökmen N, Yilmaz O, et al. Erythropoietin-induced changes in brain gene expression reveal induction of synaptic plasticity genes in experimental stroke. Proc Natl Acad Sci U S A (2012) 109(24):9617–22. doi:10.1073/pnas.1200554109
14. Anderson GD, Peterson TC, VonderHaar C, Kantor ED, Farin FM, Bammler TK, et al. Comparison of the effects of erythropoietin and anakinra on functional recovery and gene expression in a traumatic brain injury model. Front Pharmacol (2013) 4:129. doi:10.3389/fphar.2013.00129
15. Tüfekci KU, Meuwissen RL, Genç S. The role of microRNAs in biological processes. Methods Mol Biol (2014) 1107:15–31. doi:10.1007/978-1-62703-748-8_2
16. Lee RC, Feinbaum RL, Ambros V. The C. elegansheterochronic gene lin-4 encodes small RNAs with antisense complementarity to lin-14. Cell (1993) 75(5):843–54. doi:10.1016/0092-8674(93)90529-Y
17. Follert P, Cremer H, Béclin C. MicroRNAs in brain development and function: a matter of flexibility and stability. Front Mol Neurosci (2014) 7:5. doi:10.3389/fnmol.2014.00005
18. Kosaka N, Sugiura K, Yamamoto Y, Yoshioka Y, Miyazaki H, Komatsu N, et al. Identification of erythropoietin-induced microRNAs in haematopoietic cells during erythroid differentiation. Br J Haematol (2008) 142(2):293–300. doi:10.1111/j.1365-2141.2008.07151.x
19. Keller A, Leidinger P, Lange J, Borries A, Schroers H, Scheffler M, et al. Multiple sclerosis: microRNA expression profiles accurately Differentiate patients with relapsing-remitting disease from healthy controls. PLoS One (2009) 4(10):e7440. doi:10.1371/journal.pone.0007440
20. Hochberg Y. A sharper Bonferroni procedure for multiple tests of significance. Biometrica (1988) 75:185–93. doi:10.1093/biomet/75.4.800
21. Benjamini Y, Hochberg Y. Controlling the false discovery rate: a practical and powerful approach to multiple testing. J R Stat Soc B (1995) 57:289–300.
22. Schmittgen TD, Livak KJ. Analyzing real-time PCR data by the comparative C(T) method. Nat Protoc (2008) 3(6):1101–8. doi:10.1038/nprot.2008.73
23. Hsu SD, Lin FM, Wu WY, Liang C, Huang WC, Chan WL, et al. miRTarBase: a database curates experimentally validated microRNA-target interactions. Nucleic Acids Res (2011) 39:D163–9. doi:10.1093/nar/gkq1107
24. Vergoulis T, Vlachos IS, Alexiou P, Georgakilas G, Maragkakis M, Reczko M, et al. TarBase 6.0: capturing the exponential growth of miRNA targets with experimental support. Nucleic Acids Res (2012) 40:D222–9. doi:10.1093/nar/gkr1161
25. D’Eustachio P. Reactome knowledgebase of human biological pathways and processes. Methods Mol Biol (2011) 694:49–61. doi:10.1007/978-1-60761-977-2_4
26. Brines M, Cerami A. The receptor that tames the innate immune response. Mol Med (2012) 18:486–96. doi:10.2119/molmed.2011.00414
27. Nairz M, Sonnweber T, Schroll A, Theurl I, Weiss G. The pleiotropic effects of erythropoietin in infection and inflammation. Microbes Infect (2012) 14(3):238–46. doi:10.1016/j.micinf.2011.10.005
28. Pregi N, Vittori D, Pérez G, Leirós CP, Nesse A. Effect of erythropoietin on staurosporine-induced apoptosis and differentiation of SH-SY5Y neuroblastoma cells. Biochim Biophys Acta (2006) 1763(2):238–46. doi:10.1016/j.bbamcr.2005.12.011
29. Um M, Gross AW, Lodish HF. A “classical” homodimeric erythropoietin receptor is essential for the antiapoptotic effects of erythropoietin on differentiated neuroblastoma SH-SY5Y and pheochromocytoma PC-12 cells. Cell Signal (2007) 19(3):634–45. doi:10.1016/j.cellsig.2006.08.014
30. Wenker SD, Chamorro ME, Vittori DC, Nesse AB. Protective action of erythropoietin on neuronal damage induced by activated microglia. FEBS J (2013) 280(7):1630–42. doi:10.1111/febs.12172
31. Qiu J, Zhou XY, Zhou XG, Cheng R, Liu HY, Li Y. Neuroprotective effects of microRNA-210 against oxygen-glucose deprivation through inhibition of apoptosis in PC12 cells. Mol Med Rep (2013) 7(6):1955–9. doi:10.3892/mmr.2013.1431
32. Zeng L, He X, Wang Y, Tang Y, Zheng C, Cai H, et al. MicroRNA-210 overexpression induces angiogenesis and neurogenesis in the normal adult mouse brain. Gene Ther (2014) 21(1):37–43. doi:10.1038/gt.2013.55
33. Lee K, Kim JH, Kwon OB, An K, Ryu J, Cho K, et al. An activity-regulated microRNA, miR-188, controls dendritic plasticity and synaptic transmission by downregulating neuropilin-2. J Neurosci (2012) 32(16):5678–87. doi:10.1523/JNEUROSCI.6471-11.2012
34. Yuen CM, Leu S, Lee FY, Yen CH, Lin YC, Chua S, et al. Erythropoietin markedly attenuates brain infarct size and improves neurological function in the rat. J Investig Med (2010) 58(7):893–904. doi:10.231/JIM.0b013e3181e80c40
35. Rosenstein JM, Krum JM, Ruhrberg C. VEGF in the nervous system. Organogenesis (2010) 6(2):107–14. doi:10.4161/org.6.2.11687
36. Hobson SA, Bacon A, Elliot-Hunt CR, Holmes FE, Kerr NC, Pope R, et al. Galanin acts as a trophic factor to the central and peripheral nervous systems. EXS (2010) 102:25–38. doi:10.1007/s00018-008-8154-7
37. Mengozzi M, Ermilov P, Annenkov A, Ghezzi P, Pearl F. Definition of a family of tissue-protective cytokines using functional cluster analysis: a proof-of-concept study. Front Immunol (2014) 5:115. doi:10.3389/fimmu.2014.00115
38. Hellewell SC, Yan EB, Alwis DS, Bye N, Morganti-Kossmann MC. Erythropoietin improves motor and cognitive deficit, axonal pathology, and neuroinflammation in a combined model of diffuse traumatic brain injury and hypoxia, in association with upregulation of the erythropoietin receptor. J Neuroinflammation (2013) 10:156. doi:10.1186/1742-2094-10-156
39. Afanasyeva EA, Mestdagh P, Kumps C, Vandesompele J, Ehemann V, Theissen J, et al. MicroRNA miR-885-5p targets CDK2 and MCM5, activates p53 and Inhibits proliferation and survival. Cell Death Differ (2011) 18(6):974–84. doi:10.1038/cdd.2010.164
40. Ferracin M, Bassi C, Pedriali M, Pagotto S, D’Abundo L, Zagatti B, et al. miR-125b targets erythropoietin and its receptor and their expression correlates with metastatic potential and ERBB2/HER2 expression. Mol Cancer (2013) 12(1):130. doi:10.1186/1476-4598-12-130
41. Stachurska A, Ciesla M, Kozakowska M, Wolffram S, Boesch-Saadatmandi C, Rimbach G, et al. Cross-talk between microRNAs, nuclear factor E2-related factor 2, and heme oxygenase-1 in ochratoxin A-induced toxic effects in renal proximal tubular epithelial cells. Mol Nutr Food Res (2013) 57(3):504–15. doi:10.1002/mnfr.201200456
42. Chamorro ME, Wenker SD, Vota DM, Vittori DC, Nesse AB. Signaling pathways of Cell proliferation are involved in the differential effect of erythropoietin and Its carbamylated derivative. Biochim Biophys Acta (2013) 1833(8):1960–8. doi:10.1016/j.bbamcr.2013.04.006
43. Hu G, Drescher KM, Chen XM. Exosomal miRNAs: biological properties and therapeutic potential. Front Genet (2012) 3:56. doi:10.3389/fgene.2012.00056
Keywords: erythropoietin, miRNAs, cell death, proliferation, apoptosis, migration, neurite outgrowth, oxidative stress
Citation: Alural B, Duran GA, Tufekci KU, Allmer J, Onkal Z, Tunali D, Genc K and Genc S (2014) EPO mediates neurotrophic, neuroprotective, anti-oxidant, and anti-apoptotic effects via downregulation of miR-451 and miR-885-5p in SH-SY5Y neuron-like cells. Front. Immunol. 5:475. doi: 10.3389/fimmu.2014.00475
Received: 15 July 2014; Accepted: 17 September 2014;
Published online: 30 September 2014.
Edited by:
Pietro Ghezzi, Brighton and Sussex Medical School, UKReviewed by:
Manuela Mengozzi, Brighton and Sussex Medical School, UKGiovanni Grasso, University of Palermo, Italy
Copyright: © 2014 Alural, Duran, Tufekci, Allmer, Onkal, Tunali, Genc and Genc. This is an open-access article distributed under the terms of the Creative Commons Attribution License (CC BY). The use, distribution or reproduction in other forums is permitted, provided the original author(s) or licensor are credited and that the original publication in this journal is cited, in accordance with accepted academic practice. No use, distribution or reproduction is permitted which does not comply with these terms.
*Correspondence: Kursad Genc, Department of Neuroscience, Health Science Institute, Dokuz Eylul University, Balcova, Izmir 35340, Turkey e-mail:a2VtYWwuZ2VuY0BkZXUuZWR1LnRy