- Infection and Immunity Group, Department of Comparative Biomedical Sciences, Royal Veterinary College, University of London, London, UK
Age-related regression of the thymus is associated with a decline in naïve T cell output. This is thought to contribute to the reduction in T cell diversity seen in older individuals and linked with increased susceptibility to infection, autoimmune disease, and cancer. Thymic involution is one of the most dramatic and ubiquitous changes seen in the aging immune system, but the mechanisms which underlying this process are poorly understood. However, a picture is emerging, implicating the involvement of both extrinsic and intrinsic factors. In this review we assess the role of the thymic microenvironment as a potential target that regulates thymic involution, question whether thymocyte development in the aged thymus is functionally impaired, and explore the kinetics of thymic involution.
The Impact of Thymic Involution on Peripheral T Cell Senescence
Advance aging correlates with a reduced ability of the immune system to generate antigen specific responses to pathogens and vaccination. This collectively results in a higher incidence of infection, neoplastic, and autoimmune diseases which are preferentially observed in older individuals. These profound changes exhibited by the aging immune system is termed immunosenescence, which affects both innate and adaptive immunity (1–3).
The thymus is responsible for the development of self-restricted, self-tolerant, immunocompetent T cells but has no self-renewal properties relying on the continuous replenishment of new T cell progenitors from the bone marrow. Maturation of these cells occur through a series of proliferation and differentiation stages dependent upon receiving instructions from the specialized thymic microenvironment (4, 5).
One of the most acknowledged changes of the aging immune system is regression, or involution of the thymus (6–8), which seems to occur in almost all vertebrates suggesting that this is an evolutionary ancient and conserved process (9). Age-associated thymic involution involves a decrease in tissue mass and cellularity, together with a loss of tissue organization with the net outcome being a reduction in naïve T cell output [Figure 1; (6–8)]. This decline in naïve T cell output is believed to have a major impact on the properties on the peripheral T cell pool such that with increasing age, these cells exhibit alterations in phenotype and function, loss of diversity, and replicative senescence (10, 11). Moreover, it is these age-related changes in peripheral T cells that are believed to contribute significantly toward the features of immunosenescence (12, 13), suggesting that the altered thymic activity is a key trigger toward the decline of immune function in the aged (14).
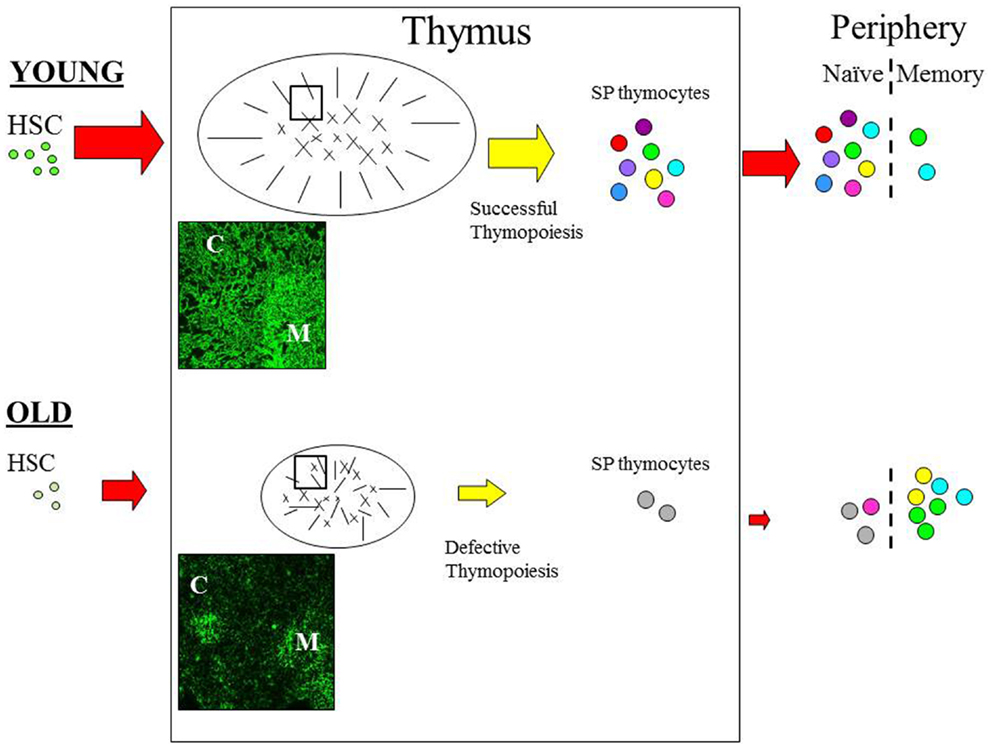
Figure 1. The effect of age on thymic function. Schematic diagram outlining the pathway of T cell development. Aging can impact on variety of pathways during the development of T cells. With increasing age HSC appear to have a reduced lymphoid potential and increased myeloid differential capacity. Age-related involution is associated with reduced thymic mass and altered architecture resulting in reduced thymic output in the aged thymus. In the young, T cell development is functional and the peripheral T cell is pool is diverse; as depicted by the various colors. Furthermore, normal thymopoiesis provides a positive effect on thymic structure; thymic cross-talk. In contrast, T cell output is significantly reduced in the aged thymus resulting in loss of diversity and alteration in the phenotype and function of peripheral T cells; with the majority of cells being in the memory pool. Modifications in the thymic microenvironment are likely to have an impact on thymopoiesis resulting in defective RTE, which in turn disrupt thymic cross-talk leading to further alteration of TEC structure. Immunofluorescence image of young (6 weeks) and old (18 months) murine thymus stained with anti-keratin antibody (55) which detects cortical (C) and medullary (M) TEC. In the young thymus, antibody staining shows the cortical epithelium as a network of long thin processes, while the medullary region reveals a squamous appearance. In contrast, the staining on old thymic section revealed a reduced network of cortical epithelial cells, the medullary region is smaller and more diffuse while the cortical-medullary junction is less distinctive; as also depicted in the schematic. C, cortex; M, medulla. Picture (100× magnification).
While animal models show that the maintenance of naïve peripheral T cells in the adult do indeed require the release of cells from the thymus (15, 16). In humans, however the relationship between thymic activity and naïve T cell homeostasis is a matter of debate, with the recent observations that peripheral proliferation and not thymic output contributes to the maintenance of naïve T cells in young adults (17). Nevertheless, using signal-joint T cell receptor (TCR) excision circles (sjTREC) as a measurement of thymic function, numerous studies have shown lower sjTREC levels in elderly individuals are associated with a reduction of naïve T cells (18–20).
Moreover, a direct correlation between thymic function and naïve T cell number comes from studies examining the peripheral immune system of thymectomized individuals (21). In one such study which looked at patients 20+ years after thymectomy, the authors observed a decreased proportion of naïve T cells, reduction in TCR diversity and noted that such changes were more marked in individuals infected with Cytomegalovirus (22). Furthermore, thymectomized individuals exhibited a delayed primary response to tick-borne encephalitis vaccination (23). Interestingly, these and other studies seem to suggest that the thymus may play a role in maintaining immune efficacy in the adult (21). Indeed, reports, using mice, have demonstrated the need for the continual production of naïve T cells to mount an effective immune response against bacterial (24), viral (25), and fungal infections (26); with the latter study showing that mice thymectomized at 5 weeks of age exhibited a delayed response to Pneumocystis infection. Furthermore, amongst HIV-infected patients under highly active antiretroviral therapy, those individuals that show enhanced T cell output appear to demonstrate a better prognosis (27, 28). Furthermore, a recent study proposed that thymic function is a key marker in determining mortality in elderly humans (29). Thus, the notion that thymus activity may play an important role in host defense of the adult is interesting and clearly merits further investigation.
Changes in Thymocyte Development with Age
Although the exact mechanisms involved in age-associated thymic involution are not fully understood, a picture is emerging suggesting defects are present within both developing thymocytes and thymic stroma (30). Thymopoiesis involves a series of sequential developmental steps. Briefly, bone marrow progenitors enter into the thymus and are identified by a lack of both CD4 and CD8. Referred to as double negative (DN) thymocytes, these cells differentiate to become double positive (DP), expressing both CD4 and CD8, and subsequently mature into either single positive (SP) CD4 or SP CD8 T cells, through the process of positive and negative selection, and then exit into the periphery (4, 5).
Given that the thymus requires the continual input of bone marrow progenitors, any age-related alterations in hematopoietic stem cells (HSC) function could conceivably contribute toward thymic involution. Studies have demonstrated that aged HSC appear to exhibit an increased bias toward myeloid differentiation together with a reduced capacity toward lymphoid maturation; which has been observed in mice and human (31, 32). Such alterations in HSC function may manifest within early thymocyte progenitor (ETP) activity. Indeed, aged mice have fewer numbers of ETP, which exhibit reduced proliferation and differentiation potential (33, 34). ETP obtained from young mice are able to differentiate into all the stages of T cell development when seeded into fetal thymic organ culture, in contrast aged ETP showed a reduction of T cell differentiation activity (33). Furthermore, ETP from aged mice show an increased frequency of cells undergoing apoptosis together with a reduced number of Ki67+ cells (34). ETP are contained within the earliest stages of DN thymocytes and other studies have highlighted further age-related changes within the later stages of DN thymocyte development; with the observation of a decrease in proportion of CD44+CD25+ (DN2) and CD44−CD25+ (DN3) cells (35–38). Additionally, a population of CD44+CD24−CD3+ DN cells has been shown to accumulate in the thymus of older mice (35, 39–41). Interestingly, a similar population has been identified in adult murine bone marrow which appears to be associated with a role in reducing hematopoiesis (42), giving rise to the possibility that the accumulation of such cells in the aging thymus might have a negative impact on thymopoiesis thereby contributing to thymic involution.
Further stages in thymocyte maturation also exhibit phenotypical alterations with age; in particular, studies have demonstrated an age-associated decline of CD3 expression on DP and SP thymocytes (40, 41, 43). Such changes may result in impaired TCR-dependent stimulation. Indeed, it has been demonstrated that aged thymocytes, in comparison to young cells, showed reduced Concanavalin A-induced proliferation (37, 40, 41, 44), with the observation that aged cells failed to enter into the G2M phase of the cell cycle (41).
Arguably, these age-related changes in thymopoiesis are likely be acquired by RTE; leading to the possibility that such cells will exhibit reduced immunocompetence. Indeed, several studies have showed that aged RTE undergo phenotypic maturation with delayed kinetics, exhibit decreased proliferative capacity, defective calcium signaling following TCR stimulation, and reduced helper and memory activity (45–47). Furthermore, peripheral T cells from older mice exhibit increased resistance to apoptosis which again may be acquired during thymocyte development as it has been demonstrated that thymocytes from older animals are more resistant to apoptosis (41, 44, 48). It is unlikely that the impairment of aged RTE is acquired in the periphery, but is imprinted during their development in the aged thymus and propose that such flawed cells are also likely to contribute further to peripheral immunosenescence. Moreover, these studies also question, the notion regarding whether T cell development is functionally active in the aged and in light of these studies, this often held view may need to be revised (40).
Age-Associated Changes in the Thymic Stromal Environment
The thymic stroma plays a crucial role in thymopoiesis by providing the signals necessary to promote proliferation and differentiation due primarily to the influence of cortical and medullary epithelial cells (4); thus age-related changes in the thymic niches could potentially promote thymic involution. In fact, we have argued that the extrinsic defects within the aged microenvironment contribute significantly to age-associated thymic involution (1, 14, 49). Several studies have demonstrated that with age, the thymic microenvironment undergoes structural, phenotypical, and architectural changes (50). This include down regulation of various thymic epithelial cell (TEC) markers such keratin, MHC class II together with alterations of cortical and medullary markers (37, 51–55). Furthermore, the structural integrity of the thymic niche is disrupted with age, including disorganization of the cortical and medullary junction; together with increase fibrosis, adipose tissue, and the accumulation of senescent cells in the aged thymus (40, 55–57).
The age-associated changes in thymopoiesis would principally imply intrinsic defects, however, closer examination reveal that perhaps such alterations could be due, in part, to extrinsic defects within the aged thymic stromal niche resulting in impaired T cell development. For instance, studies have revealed that the production of IL-7, which is necessary for thymopoiesis (58), decreases with age (59). This may be due to the observed loss of MHC class II+ TEC in the aged thymus which has been identified as the cell type responsible for producing IL-7 (54). Moreover, IL-7 administered in older mice (60) and rhesus macaques (61) was shown to increased thymic output. Interestingly, bone marrow from young mice injected into lethally irradiated older mice failed to restore thymic architecture and was still accompanied by a reduction in quantitative thymic function (62). In an elegant study addressing the repopulation potential of thymic progenitors, Zhu and colleagues transplanted fetal thymic lobes under the kidney capsule of 1-month-old and 18-months-old mice and observed that the total number and proportion of developing thymocytes in the grafts were similar in older and younger host mice (56, 63). Similar results were obtained when transplanting RAG deficient thymic lobes in that the ability of wild-type thymic progenitors to develop stromal patterning was not dependent on the age of recipients (63). In contrast, it was observed that intrathymic injection of young ETP fail to develop in older animals but did so in the thymus of young recipients (63). Furthermore, recent studies revealed that age-associated thymic involution results primarily with changes in gene expression profile in thymic stromal cells (64).
Above all, these studies suggest that the thymic stroma is a key factor in regulating thymic involution and perhaps the acquired intrinsic defects in aged thymocytes could be due to the inability of the aged thymic microenvironment to support and maintain thymopoiesis (56). Furthermore, the inter-dependency of both thymocyte and TEC to maintain a functional thymic structure (i.e., thymic cross-talk), is also likely to be a contributing factor toward thymic involution (65). Indeed, disrupting the integrity of TEC in the adult thymus has been shown to mimic thymic involution. The transcription factor Foxn1, which is essential for TEC development (66), has been shown to be important for maintaining TEC activity and reducing Foxn1 expression in the postnatal thymus mimics features of thymic involution (67, 68). In contrast, over expression of Foxn1 delays age-associated thymic involution (69). Moreover, rejuvenation of the aging thymus has been successful when targeting TEC, with the administration of exogenous keratin growth factor being shown to enhance thymic cellularity, restore thymic architecture, and improve immune function in aged mice (70). Similar results have also been seen when using growth hormone (71), sex steroid ablation (72), ghrelin (73), and IL-22 (74). However, although such treatment have been effective in directly enhancing thymic activity in the aged, in some instances, this may also be due, in part, by promoting hematopoiesis in the bone marrow (71, 75).
In addition to the age-related changes observed in TEC, there is an accumulation of adipose tissue particularly in the human thymus and there is increasing evidence indicating that thymic adiposity may inhibit thymic function (57). In mice, Yang and colleagues demonstrated that inducing obesity in mice accelerated thymic involution (76). In contrast, in another study, the same group observed that caloric restriction resulted in reduced thymic adiposity and delayed thymic involution (77). Although it is unclear how increase thymic adiposity alters thymic function, it has been proposed that this is due to the cytokines produced by adipocytes (57) and while involution occurs before fat deposition, suggesting that it is not initiating thymic involution, it may however exacerbate the impact of age on thymic function.
Studies have also noted an increase in the proportion of fibroblasts in the aging thymus of several species including mice (1, 54), human (52), and fish (78); suggesting that this may be a common feature. Several tissues such as heart (79), kidney (80), and liver (81) also show increased fibrosis with age which is associated with senescence and impairment of tissue function. Reports have implicated a role for TGFβ (82) and metalloproteinases (80) in the accumulation of fibroblasts in various tissues, which may be activated in response to inflammation as a result of wounding (83). It is currently unknown whether similar events also occur in the thymus, but may exacerbate the aforementioned alterations seen with age.
Kinetics of Age-Associated Thymic Involution
An often held view is that thymic involution is triggered during puberty. This is based on studies showing that sex steroids have a detrimental effect on thymocytes and that chemical or surgical castration in older rodents is able to restore thymic size (34, 38, 64). While sex hormones are likely to contribute to thymic regression, the role of these steroids being responsible for initiating thymic involution is now being questioned (84). Indeed, several studies using a variety of thymic indices (cellularity, epithelial space, number of recent thymic emigrants) have observed that thymic involution occurs early in life, prior to puberty and that the rate of decline is not linear, but appears to be phasic. In mice, thymic cellularity begins to decrease within the first few weeks after birth (37, 45, 53, 85) and a similar picture is evident in human (51, 52, 86), equine (87), and zebrafish (88) thymus.
After this rapid early decline, involution appears to proceed at a steady rate, with studies examining human thymus suggesting a rate of 3% of thymic tissue is lost per year until middle age, followed by a rate of 1% per year (6, 89); which perhaps may cease in later life with studies showing TREC levels being barely detectable in individuals over the age of 85 years (18, 19).
Overall, these studies strongly suggest that the kinetics of age-associated thymic involution is not uniform throughout life, but characterized by distinct phases and perhaps controlled by different mechanisms. Indeed, the onset of thymic involution occurs much earlier than most acknowledged features of aging and interestingly, microarray analysis of the aged thymic revealed limited overlap with genes normally associated with aging (7). Thus, we propose that there are at least two phases in thymic involution: the first occurring in early life which would be referred to as “growth-dependent thymic involution,” as it is associated with this period of physiological growth and development and another termed “age-dependent thymic involution” linked to the age-related changes that are occurring in various body systems (85).
Concluding Remarks
Age-associated thymic involution represents one of the most recognizable features of the aging immune system and is believed to contribute significantly toward immunosenescence. Although the molecular triggers that instigate involution remain to be fully elucidated, both intrinsic and extrinsic factors are thought to contribute toward this process. Moreover, TEC offers a potential target for rejuvenation and requires further exploration. Given the alterations in thymic development in the aged, the evidence suggests that the RTE from the aging thymus are intrinsically defective and could further exacerbate peripheral immunosenescence. Finally, additional factors that are known to modulate thymic function such as pregnancy, infection, inflammatory status, and early life events; i.e., life history is also likely to have an impact on the rate of thymic involution (9, 90).
Conflict of Interest Statement
The author declares that the research was conducted in the absence of any commercial or financial relationships that could be construed as a potential conflict of interest.
Acknowledgments
I would like to thank Professor Julian Dyson for critical reading of the manuscript.
References
1. Aw D, Silva AB, Palmer DB. Immunosenescence: emerging challenges for an ageing population. Immunology (2007) 120:435–46. doi: 10.1111/j.1365-2567.2007.02555.x
2. Shaw AC, Joshi S, Greenwood H, Panda A, Lord JM. Aging of the innate immune system. Curr Opin Immunol (2010) 22:507–13. doi:10.1016/j.coi.2010.05.003
3. McElhaney JE, Zhou X, Talbot HK, Soethout E, Bleackley RC, Granville DJ, et al. The unmet need in the elderly: how immunosenescence, CMV infection, co-morbidities and frailty are a challenge for the development of more effective influenza vaccines. Vaccine (2012) 30:2060–7. doi:10.1016/j.vaccine.2012.01.015
4. Anderson G, Jenkinson EJ. Lymphostromal interactions in thymic development and function. Nat Rev Immunol (2001) 1:31–40. doi:10.1038/35095500
5. Ma D, Wei Y, Liu F. Regulatory mechanisms of thymus and T cell development. Dev Comp Immunol (2013) 39:91–102. doi:10.1016/j.dci.2011.12.013
6. George AJ, Ritter MA. Thymic involution with ageing: obsolescence or good housekeeping? Immunol Today (1996) 17:267–72. doi:10.1016/0167-5699(96)80543-3
7. Taub DD, Longo DL. Insights into thymic aging and regeneration. Immunol Rev (2005) 205:72–93. doi:10.1111/j.0105-2896.2005.00275.x
8. Lynch HE, Goldberg GL, Chidgey A, Van den Brink MR, Boyd R, Sempowski GD. Thymic involution and immune reconstitution. Trends Immunol (2009) 30:366–73. doi:10.1016/j.it.2009.04.003
9. Shanley DP, Aw D, Manley NR, Palmer DB. An evolutionary perspective on the mechanisms of immunosenescence. Trends Immunol (2009) 30:374–81. doi:10.1016/j.it.2009.05.001
10. Hohensinner PJ, Goronzy JJ, Weyand CM. Telomere dysfunction, autoimmunity and aging. Aging Dis (2011) 2:524–37.
11. Akbar AN, Henson SM. Are senescence and exhaustion intertwined or unrelated processes that compromise immunity? Nat Rev Immunol (2011) 11:289–95. doi:10.1038/nri2959
12. Haynes L, Swain SL. Why aging T cells fail: implications for vaccination. Immunity (2006) 24:663–6. doi:10.1016/j.immuni.2006.06.003
13. Goronzy JJ, Weyand CM. Understanding immunosenescence to improve responses to vaccines. Nat Rev Immunol (2013) 14:428–36. doi:10.1038/ni.2588
15. Cicin-Sain L, Messaoudi I, Park B, Currier N, Planer S, Fsicher M, et al. Dramatic increase in naive T cell turnover is linked to loss of naive T cells from old primates. Proc Natl Acad Sci U S A (2007) 104:19960–5. doi:10.1073/pnas.0705905104
16. Bourgeois C, Hao Z, Rajewsky K, Potocnik AJ, Stockinger B. Ablation of thymic export causes accelerated decay of naive CD4 T cells in the periphery because of activation by environmental antigen. Proc Natl Acad Sci U S A (2008) 105:8691–6. doi:10.1073/pnas.0803732105
17. den Braber I, Mugwagwa T, Vrisekoop N, Westera L, Mögling R, de Boer AB, et al. Maintenance of peripheral naive T cells is sustained by thymus output in mice but not humans. Immunity (2012) 36:288–97. doi:10.1016/j.immuni.2012.02.006
18. Nasi M, Troiano L, Lugli E, Pinti M, Ferraresi R, Monterastelli E, et al. Thymic output and functionality of the IL-7/IL-7 receptor system in centenarians: implications for the neolymphogenesis at the limit of human life. Aging Cell (2006) 5:167–75. doi:10.1111/j.1474-9726.2006.00204.x
19. Mitchell WA, Lang PO, Aspinall R. Tracing thymic output in older individuals. Clin Exp Immunol (2010) 161:497–503. doi:10.1111/j.1365-2249.2010.04209.x
20. Ferrando-Martínez S, Ruiz-Mateos E, Hernández A, Gutiérrez E, Rodríguez-Méndez MM, Ordoñez A, et al. Age-related deregulation of naive T cell homeostasis in elderly humans. Age (Dordr) (2011) 33:197–207. doi:10.1007/s11357-010-9170-8
21. Sauce D, Appay V. Altered thymic activity in early life: how does it affect the immune system in young adults? Curr Opin Immunol (2011) 23:543–8. doi:10.1016/j.coi.2011.05.001
22. Sauce D, Larsen M, Fastenackels S, Duperrier A, Keller M, Grubeck-Loebenstein B, et al. Evidence of premature immune aging in patients thymectomized during early childhood. J Clin Invest (2009) 119:3070–8. doi:10.1172/JCI39269
23. Prelog M, Wilk C, Keller M, Karall T, Orth D, Geiger R, et al. Diminished response to tick-borne encephalitis vaccination in thymectomized children. Vaccine (2008) 26:595–600. doi:10.1016/j.vaccine.2007.11.074
24. Reiley WW, Wittmer ST, Ryan LM, Eaton SM, Haynes L, Winslow GM, et al. Maintenance of peripheral T cell responses during Mycobacterium tuberculosis infection. J Immunol (2012) 189:4451–8. doi:10.4049/jimmunol.1201153
25. Vezys V, Masopust D, Kemball CC, Barber DL, O’Mara LA, Larsen CP, et al. Continuous recruitment of naive T cells contributes to heterogeneity of antiviral CD8 T cells during persistent infection. J Exp Med (2006) 203:2263–9. doi:10.1084/jem.20060995
26. Shi X, Zhang P, Sempowski GD, Shellito JE. Thymopoietic and bone marrow response to murine Pneumocystis pneumonia. Infect Immun (2011) 79:2031–42. doi:10.1128/IAI.01213-10
27. Douek DC, McFarland RD, Keiser PH, Gage EA, Massey JM, Haynes BF, et al. Changes in thymic function with age and during the treatment of HIV infection. Nature (1998) 396:690–5. doi:10.1038/25374
28. de la Rosa R, Leal M. Thymic involvement in recovery of immunity among HIV-infected adults on highly active antiretroviral therapy. J Antimicrob Chemother (2003) 52:155–8. doi:10.1093/jac/dkg311
29. Ferrando-Martínez S, Romero-Sánchez MC, Solana R, Delgado J, de la Rosa R, Muñoz-Fernández MA, et al. Thymic function failure and C-reactive protein levels are independent predictors of all-cause mortality in healthy elderly humans. Age (Dordr) (2013) 35:251–9. doi:10.1007/s11357-011-9341-2
30. Linton PJ, Dorshkind K. Age-related changes in lymphocyte development and function. Nat Immunol (2004) 5:133–9.
31. Beerman I, Maloney WJ, Weissmann IL, Rossi DJ. Stem cells and the aging hematopoietic system. Curr Opin Immunol (2010) 22:500–6. doi:10.1016/j.coi.2010.06.007
32. Van Zant G, Liang Y. Concise review: hematopoietic stem cell aging, life span, and transplantation. Stem Cells Transl Med (2012) 9:651–7. doi:10.5966/sctm.2012-0033
33. Min H, Montecino-Rodriguez E, Dorshkind K. Reduction in the developmental potential of intrathymic T cell progenitors with age. J Immunol (2004) 173:245–50.
34. Heng TS, Goldberg GL, Gray DH, Sutherland JS, Chidgey AP, Boyd RL. Effects of castration on thymocyte development in two different models of thymic involution. J Immunol (2005) 175:2982–93.
35. Thoman ML. The pattern of T lymphocyte differentiation is altered during thymic involution. Mech Ageing Dev (1995) 82:155–70. doi:10.1016/0047-6374(95)01597-S
36. Aspinall R. Age-associated thymic atrophy in the mouse is due to a deficiency affecting rearrangement of the TCR during intrathymic T cell development. J Immunol (1997) 158:3037–45.
37. Li L, Hsu HC, Grizzle WE, Stockard CR, Ho KJ, Lott P, et al. Cellular mechanism of thymic involution. Scand J Immunol (2003) 57:410–42. doi:10.1046/j.1365-3083.2003.01206.x
38. Sutherland JS, Goldberg GL, Hammett MV, Uldrich AP, Berzins SP, Heng TS, et al. Activation of thymic regeneration in mice and humans following androgen blockade. J Immunol (2005) 175:2741–53.
39. Fowlkes BJ, Pardoll DM. Molecular and cellular events of T cell development. Adv Immunol (1989) 44:207–64. doi:10.1016/S0065-2776(08)60643-4
40. Aw D, Silva AB, Palmer DB. Is thymocyte development functional in the aged? Aging (Albany NY) (2009) 1:146–53.
41. Aw D, Silva AB, Palmer DB. The effect of age on the phenotype and function of developing thymocytes. J Comp Pathol (2010) 142(Suppl 1):S45–59. doi:10.1016/j.jcpa.2009.10.004
42. Sykes M. Unusual T cell populations in adult murine bone marrow. Prevalence of CD3+CD4-CD8- and alpha beta TCR+NK1.1+ cells. J Immunol (1990) 145:3209–15.
43. Lau LL, Spain LM. Altered aging-related thymic involution in T cell receptor transgenic, MHC-deficient, and CD4-deficient mice. Mech Ageing Dev (2000) 114:101–21. doi:10.1016/S0047-6374(00)00091-9
44. Leposavic G, Pesic V, Kosec D, Radojevic K, Arsenovic-Ranin N, Pilipovic I, et al. Age-associated changes in CD90 expression on thymocytes and in TCR-dependent stages of thymocyte maturation in male rats. Exp Gerontol (2006) 41:574–89. doi:10.1016/j.exger.2006.03.006
45. Hale JS, Boursalian TE, Turk GL, Fink PJ. Thymic output in aged mice. Proc Natl Acad Sci U S A (2006) 103:8447–52. doi:10.1073/pnas.0601040103
46. Clise-Dwyer K, Huston GE, Buck AL, Duso DK, Swain SL. Environmental and intrinsic factors lead to antigen unresponsiveness in CD4(+) recent thymic emigrants from aged mice. J Immunol (2007) 178:1321–31.
47. Eaton SM, Maue AC, Swain SL, Haynes L. Bone marrow precursor cells from aged mice generate CD4 T cells that function well in primary and memory responses. J Immunol (2008) 181:4825–31.
48. Provinciali M, Di Stefano G, Stronati S. Flow cytometric analysis of CD3/TCR complex, zinc, and glucocorticoid-mediated regulation of apoptosis and cell cycle distribution in thymocytes from old mice. Cytometry (1998) 32:1–8. doi:10.1002/(SICI)1097-0320(19980501)32:1<1::AID-CYTO1>3.3.CO;2-N
49. Su D-M, Aw D, Palmer DB. Immunosenescence: a product of the environment? Curr Opin Immunol (2013) 25:498–503. doi:10.1016/j.coi.2013.05.018
50. Chinn IK, Blackburn CC, Manley NR, Sempowski GD. Changes in primary lymphoid organs with aging. Semin Immunol (2012) 24:309–20. doi:10.1016/j.smim.2012.04.005
51. Steinmann GG, Klaus B, Muller-Hermelink HK. The involution of the ageing human thymic epithelium is independent of puberty. A morphometric study. Scand J Immunol (1985) 22:563–75. doi:10.1111/j.1365-3083.1985.tb01916.x
52. Bertho JM, Demarquay C, Moulain N, Van Der Meeren A, Berrih-Aknin S, Gourmelon P. Phenotypic and immunohistological analyses of the human adult thymus: evidence for an active thymus during adult life. Cell Immunol (1997) 179:30–40. doi:10.1006/cimm.1997.1148
53. Ortman CL, Dittmar KA, Witte PL, Le PT. Molecular characterization of the mouse involuted thymus: aberrations in expression of transcription regulators in thymocyte and epithelial compartments. Int Immunol (2002) 14:813–22. doi:10.1093/intimm/dxf042
54. Gray DH, Seach N, Ueno T, Milton MK, Liston A, Lew AM, et al. Developmental kinetics, turnover, and stimulatory capacity of thymic epithelial cells. Blood (2006) 108:3777–85. doi:10.1182/blood-2006-02-004531
55. Aw D, Silva AB, Maddick MT, von Zglinicki T, Palmer DB. Architectural changes in the thymus of aging mice. Aging Cell (2008) 7:158–67. doi:10.1111/j.1474-9726.2007.00365.x
56. Gui J, Zhu X, Dohkan J, Cheng L, Barnes PF, Su D-M. The aged thymus shows normal recruitment of lymphohematopoietic progenitors but has defects in thymic epithelial cells. Int Immunol (2007) 19:1201–11. doi:10.1093/intimm/dxm095
57. Dixit VD. Thymic fatness and approaches to enhance thymopoietic fitness in aging. Curr Opin Immunol (2010) 22:521–8. doi:10.1016/j.coi.2010.06.010
58. Hong C, Luckey MA, Park JH. Intrathymic IL-7: the where, when, and why of IL-7 signaling during T cell development. Semin Immunol (2012) 24:151–8. doi:10.1016/j.smim.2012.02.002
59. Aspinall R, Andrew D. Thymic atrophy in the mouse is a soluble problem of the thymic environment. Vaccine (2000) 18:1629–37. doi:10.1016/S0264-410X(99)00498-3
60. Pido-Lopez J, Imami N, Andrew D, Aspinall R. Molecular quantitation of thymic output in mice and the effect of IL-7. Eur J Immunol (2002) 32:2827–36. doi:10.1002/1521-4141(2002010)32:10<2827::AID-IMMU2827>3.0.CO;2-X
61. Aspinall R, Pido-Lopez J, Imami N, Henson SM, Ngom PT, Morre M, et al. Old rhesus macaques treated with interleukin-7 show increased TREC levels and respond well to influenza vaccination. Rejuvenation Res (2007) 10:5–17. doi:10.1089/rej.2006.9098
62. Mackall CL, Punt JA, Morgan P, Farr AG, Gress RE. Thymic function in young/old chimeras: substantial thymic T cell regenerative capacity despite irreversible age-associated thymic involution. Eur J Immunol (1998) 28:1886–93. doi:10.1002/(SICI)1521-4141(199806)28:06<1886::AID-IMMU1886>3.0.CO;2-M
63. Zhu X, Gui J, Dohkan J, Cheng L, Barnes PF, Su D-M. Lymphohematopoietic progenitors do not have a synchronized defect with age-related thymic involution. Aging Cell (2007) 6:663–72. doi:10.1111/j.1474-9726.2007.00325.x
64. Griffith AV, Fallahi M, Venables T, Petrie HT. Persistent degenerative changes in thymic organ function revealed by an inducible model of organ regrowth. Aging Cell (2012) 11:169–77. doi:10.1111/j.1474-9726.2011.00773.x
65. Palmer DB, Viney JL, Ritter MA, Hayday AC, Owen MJ. Expression of the alpha beta T-cell receptor is necessary for the generation of the thymic medulla. Dev Immunol (1993) 3:175–9. doi:10.1155/1993/56290
66. Nehls M, Pfeifer D, Schorpp M, Hedrich H, Boehm T. New member of the winged-helix protein family disrupted in mouse and rat nude mutations. Nature (1994) 1994(372):103–7. doi:10.1038/372103a0
67. Chen L, Xiao S, Manley NR. Foxn1 is required to maintain the postnatal thymic microenvironment in a dosage-sensitive manner. Blood (2009) 113:567–74. doi:10.1182/blood-2008-05-156265
68. Cheng L, Guo J, Sun L, Fu J, Barnes PF, Metzger D, et al. Postnatal tissue-specific disruption of transcription factor FoxN1 triggers acute thymic atrophy. J Biol Chem (2010) 285:5836–47. doi:10.1074/jbc.M109.072124
69. Zook EC, Krishack PA, Zhang S, Zeleznik-Le NJ, Firulli AB, Witte PL, et al. Overexpression of Foxn1 attenuates age-associated thymic involution and prevents the expansion of peripheral CD4 memory T cells. Blood (2011) 118:5723–31. doi:10.1182/blood-2011-03-342097
70. Min D, Panoskaltsis-Mortari A, Kuro OM, Hollander GA, Blazar BR, Weinberg KI. Sustained thymopoiesis and improvement in functional immunity induced by exogenous KFG administration in murine models of aging. Blood (2007) 109:2529–37. doi:10.1182/blood-2006-08-043794
71. French RA, Broussard SR, Meier WA, Minshall C, Arkins S, Zachary JF, et al. Age-associated loss of bone marrow hematopoietic cells is reversed by GH and accompanies thymic reconstitution. Endocrinology (2002) 143:690–9. doi:10.1210/en.143.2.690
72. Olsen NJ, Olson G, Viselli SM, Gu X, Kovacs WJ. Androgen receptors in thymic epithelium modulate thymus size and thymocyte development. Endocrinology (2001) 142:1278–83. doi:10.1210/en.142.3.1278
73. Dixit VD, Yang H, Sun Y, Weeraratna AT, Youm YH, Smith RG, et al. Ghrelin promotes thymopoiesis during aging. J Clin Invest (2007) 117:2778–90. doi:10.1172/JCI30248
74. Dudakov JA, Hanash AM, Jenq RR, Young LF, Ghosh A, Singer NV, et al. Interleukin-22 drives endogenous thymic regeneration in mice. Science (2012) 336:91–5. doi:10.1126/science.1218004
75. Dudakov JA, Goldberg GL, Reiseger JJ, Vlahos K, Chidgey AP, Boyd RL. Sex steroid ablation enhances hematopoietic recovery following cytotoxic antineoplastic therapy in aged mice. J Immunol (2009) 183:7084–94. doi:10.4049/jimmunol.0900196
76. Yang H, Youm YH, Vandanmagsar B, Rood J, Kumar KG, Butler AA, et al. Obesity accelerates thymic aging. Blood (2009) 114:3803–12. doi:10.1182/blood-2009-03-213595
77. Yang H, Youm YH, Dixit VD. Inhibition of thymic adipogenesis by caloric restriction is coupled with reduction in age-related thymic involution. J Immunol (2009) 183:3040–52. doi:10.4049/jimmunol.0900562
78. Torroba M, Zapata AG. Aging of the vertebrate immune system. Microsc Res Tech (2003) 62:477–81. doi:10.1002/jemt.10409
79. Khan AS, Sane DC, Wannenburg T, Sonntag WE. Growth hormone, insulin-like growth factor-1 and the aging cardiovascular system. Cardiovasc Res (2002) 54:25–35. doi:10.1016/S0008-6363(01)00533-8
80. Zhang X, Chen X, Hong Q, Lin H, Zhu H, Liu Q, et al. TIMP-1 promotes age-related renal fibrosis through upregulating ICAM-1 in human TIMP-1 transgenic mice. J Gerontol A Biol Sci Med Sci (2006) 61:1130–43. doi:10.1093/gerona/61.11.1130
81. Paradis V, Youssef N, Dargere D, Ba N, Bonvoust F, Deschatrette J, et al. Replicative senescence in normal liver, chronic hepatitis C, and hepatocellular carcinomas. Hum Pathol (2001) 32:327–32. doi:10.1053/hupa.2001.22747
82. Gauldie J, Kolb M, Ask K, Martin G, Bonniaud P, Warburton D. Smad3 signaling involved in pulmonary fibrosis and emphysema. Proc Am Thorac Soc (2006) 3:696–702. doi:10.1513/pats.200605-125SF
83. Mori R, Shaw TJ, Martin P. Molecular mechanisms linking wound inflammation and fibrosis: knockdown of osteopontin leads to rapid repair and reduced scarring. J Exp Med (2008) 205:43–51. doi:10.1084/jem.20071412
84. Montecino-Rodriquez E, Min H, Dorshkind K. Reevaluating current models of thymic involution. Semin Immunol (2005) 17:356–61. doi:10.1016/j.smim.2005.05.006
85. Aw D, Palmer DB. It’s not all equal: a multiphasic theory of thymic involution. Biogerontology (2012) 13:77–81. doi:10.1007/s10522-011-9349-0
86. Jamieson BD, Douek DC, Killian S, Hultin LE, Scripture-Adams DD, Giorgi JV, et al. Generation of functional thymocytes in the human adult. Immunity (1999) 10:569–75. doi:10.1016/S1074-7613(00)80056-4
87. Contreiras EC, Lenz IHL, Meirelles MN, Caputo LF, Calado TJ, Villa-Verde DM, et al. The equine thymus microenvironment: a morphological and immunohistochemical analysis. Dev Comp Immunol (2004) 28:251–64. doi:10.1016/S0145-305X(03)00134-4
88. Lam SH, Chua HL, Gong Z, Wen Z, Lam TJ, Sin YM. Morphologic transformation of the thymus in developing zebrafish. Dev Dyn (2002) 225:87–94. doi:10.1002/dvdy.10127
89. Steinmann GG. Changes in the human thymus during aging. Curr Top Pathol (1986) 75:43–88. doi:10.1007/978-3-642-82480-7_2
Keywords: thymus, immunosenescence, thymic involution, thymic stroma, thymocyte
Citation: Palmer DB (2013) The effect of age on thymic function. Front. Immunol. 4:316. doi: 10.3389/fimmu.2013.00316
Received: 03 May 2013; Accepted: 18 September 2013;
Published online: 07 October 2013.
Edited by:
Dietmar Herndler-Brandstetter, Yale University School of Medicine, USAReviewed by:
James Dooley, VIB – KU Leuven, BelgiumDietmar Herndler-Brandstetter, Yale University School of Medicine, USA
Copyright: © 2013 Palmer. This is an open-access article distributed under the terms of the Creative Commons Attribution License (CC BY). The use, distribution or reproduction in other forums is permitted, provided the original author(s) or licensor are credited and that the original publication in this journal is cited, in accordance with accepted academic practice. No use, distribution or reproduction is permitted which does not comply with these terms.
*Correspondence: Donald B. Palmer, Infection and Immunity Group, Department of Comparative Biomedical Sciences, Royal Veterinary College, University of London, Royal College Street, London NW1 0TU, UK e-mail:ZHBhbG1lckBydmMuYWMudWs=