- 1Department of Translational Medical Sciences, “Federico II” University, Naples, Italy
- 2Department of Biochemistry and Medical Biotechnology, CEINGE Institute, “Federico II” University, Naples, Italy
T cell ontogeny is a sophisticated process, which takes place within the thymus through a series of well-defined discrete stages. The process requires a proper lympho-stromal interaction. In particular, cortical and medullary thymic epithelial cells (cTECs, mTECs) drive T cell differentiation, education, and selection processes, while the thymocyte-dependent signals allow thymic epithelial cells (TECs) to maturate and provide an appropriate thymic microenvironment. Alterations in genes implicated in thymus organogenesis, including Tbx1, Pax1, Pax3, Pax9, Hoxa3, Eya1, and Six1, affect this well-orchestrated process, leading to disruption of thymic architecture. Of note, in both human and mice, the primordial TECs are yet unable to fully support T cell development and only after the transcriptional activation of the Forkhead-box n1 (FOXN1) gene in the thymic epithelium this essential function is acquired. FOXN1 is a master regulator in the TEC lineage specification in that it down-stream promotes transcription of genes, which, in turn, regulate TECs differentiation. In particular, FOXN1 mainly regulates TEC patterning in the fetal stage and TEC homeostasis in the post-natal thymus. An inborn null mutation in FOXN1 leads to Nude/severe combined immunodeficiency (SCID) phenotype in mouse, rat, and humans. In Foxn1−/− nude animals, initial formation of the primordial organ is arrested and the primordium is not colonized by hematopoietic precursors, causing a severe primary T cell immunodeficiency. In humans, the Nude/SCID phenotype is characterized by congenital alopecia of the scalp, eyebrows, and eyelashes, nail dystrophy, and a severe T cell immunodeficiency, inherited as an autosomal recessive disorder. Aim of this review is to summarize all the scientific information so far available to better characterize the pivotal role of the master regulator FOXN1 transcription factor in the TEC lineage specifications and functionality.
Introduction
The thymus is the primary lymphoid organ with the unique function to produce and to maintain the pool of mature and functional T cells. This process is strictly dependent on specialized functions of thymic stromal cells (TSCs) and requires the thymus peculiar tridimensional (3D) architecture, which allows a proper intercellular cross talk (1). For a long time, the difficulty in the isolation and characterization of the thymic cellular components has limited studies on the peculiar role of individual stromal components. Novel experimental tools, including stromal cell isolation by phenotype-based cell sorting (2), dissociation and reaggregation of stromal cell subsets (3, 4), or global gene expression analysis and the evaluation of the pattern of self-antigen expression within the individual thymic epithelial cells (TECs) subset (5), allowed to acquire important knowledge on the cellular and molecular basis of thymus organogenesis and TECs functionality.
The recent discovery of disease models associated to genetic alterations of molecules implicated in thymus specification and TECs differentiation, provided new and conclusive insights regarding the pathways, the genes, and the molecular mechanism governing these processes and stromal functionality.
The Thymus Architecture: Requirement of a 3D Structure for a Proper Lympho-Epithelial Crosstalk
The thymus provides the microenvironment essential for the development of T cells. T cell progenitors originate in the bone marrow, enter into the thymus (6, 7) and, through a series of well defined and coordinated developmental stages, differentiate, undergo selection process, and mature into functional T cells. The steps in this process are tightly regulated through a complex network of transcriptional events, specific receptor-ligand interactions, and sensitization to trophic factors, which mediate the homing, proliferation, survival, and differentiation of developing T cells (1, 8, 9).
The thymus is organized in two lobes, which are already present in mice at 21 days of thymic organogenesis and is completely organized at 1 month of post-natal life. The lobes are divided in three areas: a cortical and the dark cortical area, with a high number of lymphoid cells and epithelial cells, cortical thymic epithelial cells (cTECs); a light medullary area with a low number of mature T cells, named medullary TECs (mTECs), Hassall’s bodies (HB), macrophages, dendritic cells (DCs), B lymphocytes, and rarely myoid cells. Eventually, there is a transitional area, named cortico-medullary junction (CMJ), characterized by abundant blood vessels (10).
The unique function of the thymus in the establishment and maintenance of the T cell pool is intimately linked to this peculiar thymus architecture and to the specialized functions of the TSCs.
Lympho-Epithelial Cross-Talk Required for Thymocyte and TECs Differentiation
An important feature of the thymic microenvironment is its 3D organization, consisting of an ordered architecture of TSCs, that represents a heterogeneous mixture of distinct cell types, including cTECs, mTECs, fibroblasts, endothelial cells, DCs, and macrophages (11). Among these stromal elements, TECs are the most abundant cell types, which form a delicate 3D cellular network spanning throughout both the thymic cortex and the medulla. The requirement for the 3D-supporting stroma appears to be unique to the T cell development, as the in vitro differentiation program of other hematopoietic lineages, including B and NK cells, does not require a 3D structure (12).
Thymocyte development is not a cell-autonomous process, and the transition to the next stage in development relies on the proper interaction of HSCs with thymic stroma. The 3D configuration of the thymus maximizes this interaction, allowing intercellular cross-talk integral to the development of both T cells and TSCs (13). Paralleling the T cell precursor proliferation and differentiation program, immature TECs undergo a developmental sequence, resulting in the establishment of mature cTECs and mTECs organized in this 3D network. Several studies on mutant mice with an abnormal organization of thymic epithelium substantiated the concept that a reciprocal signaling between thymocytes and TSCs is required, not only for the production of mature T cells but also for the development and organization of the thymic microenvironment in a bi-directional fashion (14, 15). Mice showing a blockage of the T cell development process, in the absence of T cell receptor (TCR)-expressing cells, have a defective organization of the thymic medulla, as well (16, 17). Of note, under this condition, thymic medullary organization can be restored by the addition of mature T cells, which follows stem cell transplantation (17, 18). In adult CD3etg26 mice, lacking intra-thymic T cell precursors, a severe alteration of the cortical thymic architecture has been documented (19), even though a restoration of the architecture and TEC development in these mice can occur. Recently, the injection of either fetal or adult T-committed precursors into adult CD3etg26 mice leads to the reconstitution of thymic microenvironment, as indicated by thymocyte differentiation, organization of functional cortical and medullary areas, and generation of Foxp3+ Treg and Aire+ mTECs (20). These data suggest that adult TECs maintain the receptivity to cross talk with thymocytes despite a prolonged absence of T cell precursors. Moreover, the absence of both thymocytes and of the 3D framework may result in changes of the keratin genes expression, thus inducing the cTECs and mTECs to undergo a de-differentiation process and to reacquire the precursor K5+K8+ cellular phenotype. Taken together, these findings suggest that signals from early CD4–CD8– DN T cell precursors and/or their immediate progeny provide necessary signals to promote the formation of the thymic cortex, while, later in ontogeny, the differentiation of TECs into a medullary phenotype are clearly dependent on the presence of CD4+CD8− and CD4−CD8+ single positive (SP) thymocytes (21–23). However, the precise molecular nature of the signals provided by developing thymocytes, which lead to the generation of the thymic stromal compartment are still incompletely defined.
Eventually, a better understanding of the developmental process through which a normal thymus structure is built, is essential for a better comprehension of the intimate mechanisms which take place within the thymus to promote the T cell development in vivo. This knowledge may also be useful in designing future therapeutic strategies, as alterations of the thymus structure and function may result in serious health consequences, including immunodeficiency or autoimmunity.
mTECS and cTECS are Specialized Cells Playing a Different Role in the T Cell Education Process
T cell ontogeny is a sophisticated process, which takes place through discrete stages during which developing thymocytes dynamically relocate in different thymic areas, following a cortico-medullary gradient.
The initial colonization of the thymus anlagen by migrant lymphoid progenitors occurs at an early stage, embryonic day 11.5 (E11.5) in mice and 8 week of gestation in humans (24, 25). Studies documented that chemokines CC ligand (CCL)21 and CCL25 play a major role in the early stage of fetal thymus colonization (26, 27). Indeed, mice deficient for these chemokines or for the cognate receptors, showed a significant reduction in the number of thymocytes compared to normal mice (28). In post-natal thymus, lymphoid progenitor cells through their cell surface adhesion molecules, such as platelet-selectin glycoprotein ligand 1, interact with P-selectin, expressed on the TECs, and thanks to this interaction they are allowed to migrate from the blood into the thymic parenchyma, in correspondence of the area around the CMJ [Figure 1; (29)].
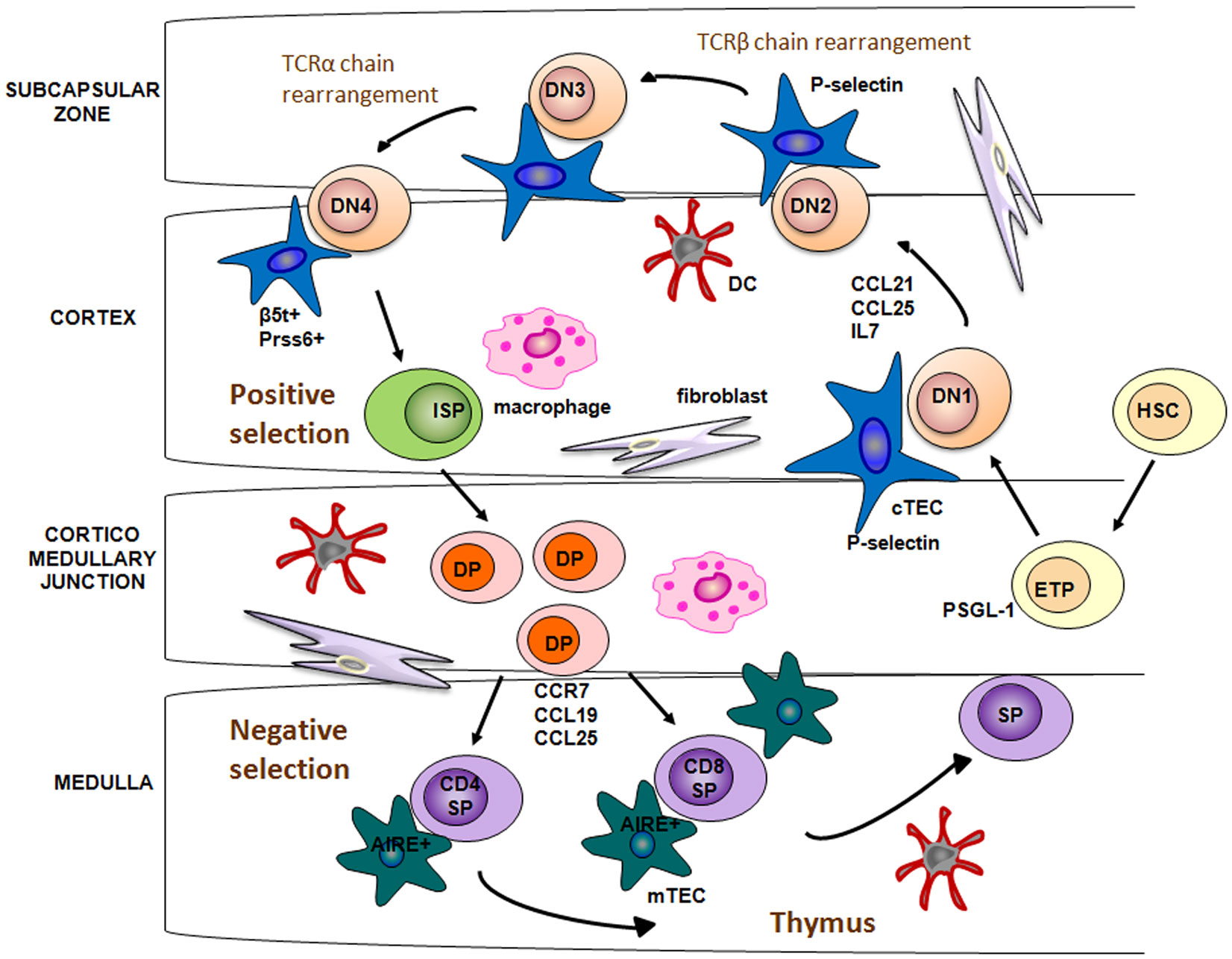
Figure 1. Lympho-stromal interactions and T cell development. Bone marrow HSCs enter into the thymus through CMJ, a process mediated by CCL12 and CCL25 in the embryonic thymus and by the interaction between P-selectin and its cognate ligand PSGL-1 in adult thymus. Stimulation by IL-7 allows the relocation of DN thymocytes from the cortex to the subcapsular region. DP thymocytes bearing TCR and capable of binding to self-MHC ligands are positively selected. This process is regulated by Pssr6 and b5t, which are expressed in cTECs. Developing thymocytes are relocated from cortex to the medulla by chemotactic attraction between CCR7 and the ligands CCL19/CCL21, expressed on the mTECs. Into medulla, self-reactive thymocytes are deleted through the negative selection, a process mediated by dendritic cells and Aire-expressing mTECs.
Entered thymocytes started to intensely proliferate and to acquire T cell hallmarks. In this phase, T cell proliferation and differentiation are triggered by a potent combination of signals provided by cTECs. Delta-like 4 (DL4), which is an essential, non-redundant ligand for Notch1 during thymic T cell development, and IL-7 are critically involved in the activation of signaling pathways, leading to the proliferation and migration of thymocytes (30–32). In particular, these intra-thymic ligands induce the development of DN CD25+ cells, which migrate toward the subcapsular region of thymic cortex (33). Several chemokine receptors have been suggested to guide the migration of immature thymocytes, such as CXCR4, CCR7, and CCR9 [Figure 1; (34)]. In the thymic cortex DN thymocytes begin V(D)J rearrangement of their TCRβ gene. Successfully rearranged TCRβ protein, assembled with the pre-TCRα chains, forms the pre-TCR complex. Membrane expression of pre-TCR complex, along with the Delta-Notch interaction, provides the signal necessary to induce the expression of the co-receptors CD4 and CD8, as well as V-J rearrangement of the TCRα genomic region. Subsequently, DP thymocytes with a functional TCR-αβ receptor are generated [Figure 1; (35)].
Thymic cortex is also the area where takes place the positive selection of DP thymocytes. Positive selection is the process by which developing thymocytes, that recognize and bind with mild avidity peptide-major histocompatibility complex on cTECs surface, get a rescue signal through their TCR and are allowed to further maturate to the CD4+CD8− or CD4−CD8+ SP stage. Only a small fraction (1–5%) of DP cells survive to positive selection. By contrast, the majority of DP cells, that bind with too low affinity to MHC complex, are programed to undergo death by neglect (36, 37).
Cortical thymic epithelial cells have a crucial role in the positive selection process of T cells within thymus cortex (38). Recent studies have found that cTECs exclusively express a specific form of proteasome, referred as thymoproteasome, which contains a peculiar catalytic subunit, the β5-thymus (β5t) (39). β5t subunits exhibit an unique peptidase activity, compared to other β5 subunits found in common immunoproteasome, which leads to the production of a set of self-peptides with a high affinity for class I MHC molecules (40). Moreover, β5t-deficient mice show a severe decrease in the number of CD8+ SP thymocytes, but no alteration in the CD4+ number or in the thymic architecture. In addition, the small fraction of CD8+ T cells, positively selected by β5t-deficient cTECs, show altered immune responses toward several stimuli. Taken together these results suggest that the thymoproteasome is essential for the production of self antigens involved in the positive selection of functional CD4−CD8+ T cells (41).
As for the positive selection of CD4+ T cells, two other proteins predominantly expressed in cTECs, the lysosomal protease Prss16 and Cathepsin L, have been demonstrated to be essential to generate an immunocompetent repertoire of CD4+CD8− T cells [Figure 1; (42, 43)].
TCR engagement by peptide-MHC complex also triggers the expression of the chemokine receptor CCR7 in positively selected thymocytes. Thanks to the chemotactic attraction between CCR7 and its ligands, CCL19 and CCL21, expressed on the mTECs, developing thymocytes are relocated from cortex to the medulla [Figure 1; (44, 45)].
In order to create a repertoire of mature T cells able to recognize foreign antigens and, at the meantime, to ignore self antigens, SP thymocytes have to undergo the negative selection process in the thymic medulla. Both mTECs and DCs, play a pivotal role in this last stage of thymocyte development, which is critical to establish the central tolerance and, eventually, to prevent autoimmunity. In contrast to cTECs, mTECs are characterized by a high expression of clustered tissue-restricted autoantigens (TSAs), the so called promiscuous gene expression (46). To date, the autoimmune regulator (AIRE) transcription factor represents the only molecule, so far identified, which contributes to the mTECs function and, in particular, to the molecular regulation of the promiscuous gene expression [Figure 1; (47)]. However, not all TSAs are regulated in an AIRE-dependent manner, suggesting that other molecular mechanisms, such as epigenetic mechanisms, may be involved in mTECs function regulation. TSAs associated with class II MHC molecules are presented directly by mTECs or indirectly by DCs to developing thymocytes (48). T cells which recognize with a high avidity self antigens are deleted. Remarkably, only a few number of mTECs express a given TSAs (about 50–500 per thymus), and lead to apoptosis by negative selection of a few thymocytes (37, 49, 50). A possible explanation is that the high motility of thymocytes within the thymic medulla during a period of 4–5 days, allows each of them to interact with mTECs (51). DCs play a similar role in the negative selection process. They are attracted in the thymic medulla by the chemochine XCL1 (lymphotactin), produced by mTECs in an AIRE-dependent manner. Differently from mTECs, DCs are not able to produce TSAs and the TSAs expressed mostly derive from the phagocytosis of apoptotic mTECs (52, 53). mTECs and DCs not only contribute to the establishment of central tolerance through the deletion of self-reactive T cells, but, also, through the generation of regulatory T cells (Tregs) (54, 55, 65, 153), which act in the periphery by suppressing autoreactive T cells, which have escaped to the process of the central tolerance.
A body of evidence documents that the expression of an autoreactive TCR leads to the entry of the thymocyte into the Treg lineage. Tregs, that are about 5–10% of peripheral T cells CD4+, constitutively express the CD25 molecule and share several immunological features, in humans and mice (56, 57). These cells specifically express the transcription factor FOXP3 (Foxp3 in mice) that plays a pivotal role in Tregs differentiation and function (58). The Foxp3 promoter region and the conserved non-coding sequence 2 (CNS2) (known as TSDR, the Treg-specific-demethylated-region) are fully methylated in immature thymocytes (59, 155). At the beginning of Treg development, an appropriate TCR/CD28 signal is needed to make available the Foxp3 promoter through shift of the Protein Inhibitors of Activated STAT 1 (PIAS1), a signal cascade, which results in the NF-κB-mediated transcription of genes playing a role in Treg differentiation (60, 61).
Thymic Formation: New Insights in Epithelial Lineages Specification
In the mouse, mTECs and cTECs originated from the third pharyngeal pouch endoderm and the thymus anlage are located next to that of the parathyroid. The expression of Forkhead-box transcription factor n1 (Foxn1) approximately at E11.5 is crucial for the subsequent epithelial differentiation, since in its absence, the colonization of the anlage by T cell progenitors from the bone marrow fails (62) and the subsequent T cell development and TECs formation is aborted, resulting in a severe immunodeficiency (63, 64, 154, 66).
The maturation process of TECs during thymic organogenesis could be divided in two genetic phases. The first stage is independent from the Foxn1 expression and consists in the induction and outgrowth of the thymic epithelial anlage from the third pharyngeal pouch, through the expression of genes including the Eya1 and Six (67), Hoxa3 (68), and Tbx1 (69, 70). During the second genetic phase, epithelial patterning and differentiation take place and the Foxn1 expression drives the immature epithelial cells to differentiate into functional cTECs and mTECs (71).
FOXN1-Indipendent Genetic Stage of TEC Differentiation
In the first phase of the thymus organogenesis an interaction between epithelial and mesenchymal cells occurs, while at the later phase lympho-epithelial interaction predominates (72). In mice, at about E10.5 the mesenchymal cells are able to respond to the endodermic signals, which induce the development of the primordial thymic epithelium (73, 74). Subsequently, at about E12.5, the thymic rudiment is colonized by progenitors come from the fetal liver, thus resulting in a tight epithelial-thymocyte interaction within the mesenchymal derived capsule. This thymic rudiment contains the EpCam+Plet1+ epithelial population (72, 75), which includes a common thymic epithelial precursor (TEPC), from which both cTECs and mTECs will be subsequently generated (72, 76).
Through studies on animal models carrying molecular alterations of distinct genes, the key role of several transcription factors involved in the thymus organogenesis and TEC-sublineage specification process, have thus far been identified (77). In particular, several genes, including Tbx1 (69, 70), Pax1, Pax3, Pax9 (78–80), Hoxa3 (68), Eya1, and Six1 (67) have been shown to play a central role in the thymus ontogeny. Indeed, their molecular alteration affects this well-orchestrated process, leading to disruption of the thymic architecture. Abnormalities of the paired box (Pax) family transcription factors Pax1 or Pax9 result in a blockage of the thymus organogenesis (79, 81). Mutations in the Hox transcription factor family member, Hoxa3, expressed on both thymic epithelium and mesenchymal cells, result in athymia (68). Furthermore, the homozygous loss of Tbx1, related to the DiGeorge syndrome phenotype, leads to thymic a/hypoplasia in humans (69, 82), while mice heterozygous for a null allele of Tbx1 show a mild phenotype without thymus anomalies (83). Therefore, the expression of Tbx1 both in the pharyngeal core mesoderm and in the pharyngeal endoderm is required for a proper thymus development. However, it remains to be elucidated whether the expression of Tbx1 in the TECs occurs and whether the gene participates in the TECs development (4).
FOXN1-Dependent Genetic Stage of TEC Differentiation
In both humans and mice, the primordial TECs are yet unable to fully support T cell development and only after the transcriptional activation of the FOXN1 gene in the thymic epithelium this essential function is acquired. FOXN1 is a master regulator in the TEC lineage development in that it promotes down-stream the transcription of genes implicated in the thymus organogenesis and TECs full differentiation.
Forkhead-box n1 transcription factor belongs to the FOX transcription factor family implicated in a variety of biochemical and cellular processes, including development, metabolism, aging, and cancer (84, 85). During the post-natal life, Foxn1 is selectively expressed only in thymic and skin epithelia, where it regulates the expression of several molecular targets to maintain the balance between growth and differentiation (86, 87). The signals required for FOXN1 expression, and its activity, are still unclear, even though the wingless (Wnt) proteins (88) and bone morphogenetic protein (BMP) signaling have been shown to regulate FOXN1 expression (89). Even though the complete pattern of FOXN1 expression over the time and its role are not yet completely defined, studies on mouse and human model of gene alterations enormously helped unravel important issues on its role. Mutations in Foxn1 gene lead to alymphoid cystic thymic dysgenesis due to a defective TECs differentiation process (63, 90). In both mice and humans FOXN1 abnormalities lead to a hairless phenotype (87, 154).
In the Foxn1-dependent step of thymus organogenesis, precursor epithelial cells differentiate into mature and functional cTECs and mTECs from the same bi-potential TEC progenitor (4, 72, 76). It has been reported, that Foxn1 is differentially expressed during the TE-lineage specification, since it is expressed in all TECs during the pre-natal life, but not in all TECs postnatally, indicating that the gene is highly developmentally regulated. There is a body of evidence documenting different effects of Foxn1 expression in mTEC and cTECs. Particularly, studies on K5- and K18-CreERT-mediated Foxn1-deleted mouse models suggested that during the post-natal life, the loss of Foxn1 affected mTECs, characterized by the expression of K5 and K14 keratins type. Conversely, the loss of Foxn1 did not affect cTECs, which express the keratins K8 and K18 (91, 92). Taken together, these data suggest that cTECs and mTECs are not equally Foxn1-dependent in the post-natal life.
Recent reports highlighted a central role for Foxn1 in TECs homeostasis in the adult thymus and its necessary role for the functionality and survival of adult TEC progenitors (92), expressing K5+ and K14+ markers. This role in adult thymus seems to be exerted in cooperation with other stem cell-related genes, such as p63. Of note, the transcription factor p63, encoding for multiple isoforms (93), plays a pivotal for the development of stratified epithelia of several tissues, such as epidermis, breast, prostate, and thymus (94). In the thymus, the p63 protein drives the proliferation of epithelial progenitor cells (94, 95). Therefore, it has been hypothesized that p63 and Foxn1 could act synergistically through the formation of a p63-Foxn1 regulatory axis aimed at regulating TECs homeostasis. However, the molecular mechanism through which the proliferation regulator p63 and differentiation regulator Foxn1 collaborate in this axis are still unclear.
FOXN1-Mediated Gene Expression for TEC Differentiation
Forkhead-box n1 is directly or indirectly implicated in the transcriptional regulation of a panel of genes involved in thymus development and function.
Pax1 is a key regulator of TEC differentiation/survival balance. Pax1 is expressed in the third pharyngeal pouch from E9.5 during the thymus ontogeny, while in the post-natal thymus only in cTEC (96). Even though the regulation of Pax1 is still unclear, from E11.0 its expression requires Hoxa3 (68). Of note, the loss of Hoxa3 impairs the intrinsic ability of the neural crest cell population to differentiate and/or to lead to the differentiation of the tissues of pharyngeal arch and pouch. Indeed, in Hoxa3 mutant mice the thymus is absent and thyroid hypoplasia has been documented (68). Moreover, the first step of thymus development is the expansion of mesenchymal neural crest in the posterior part of the third pharyngeal pouch. Prior to this event, in the Hoxa3 mutant embryos a marked reduction in Pax1 expression has been shown. Similarly, Pax1 mutant mice also show thymic hypoplasia, suggesting a role for Hoxa3 in maintaining Pax1 expression in these cells (68). In the thymic primordium, Pax1 expression is under the control of Foxn1 (71). This finding indicates that Foxn1 and Hoxa3 are both involved in the network of molecular signals that regulates Pax1 expression, thus demonstrating the existence of a molecular and/or functional interaction between Hoxa3 and Foxn1 [Figure 2; (71)]. In keeping with this, Hoxa3+/−Pax1−/− compound mutant mice display a few phenotypic hallmarks of the Foxn1R/R mouse model, which expresses low-dose of Foxn1, such as hypomorphic post-natal thymus, and reduced levels of MHC class II expression on the TECs surface (80). These data suggest two alternative hypothesis: Hoxa3 may regulate Foxn1, which, in turn, regulates Pax1 expression in the thymic primordium, in a Foxn1-dependent manner, or Hoxa3 and Foxn1 induce Pax1 expression in the third pharyngeal pouch and in early thymus primordium.
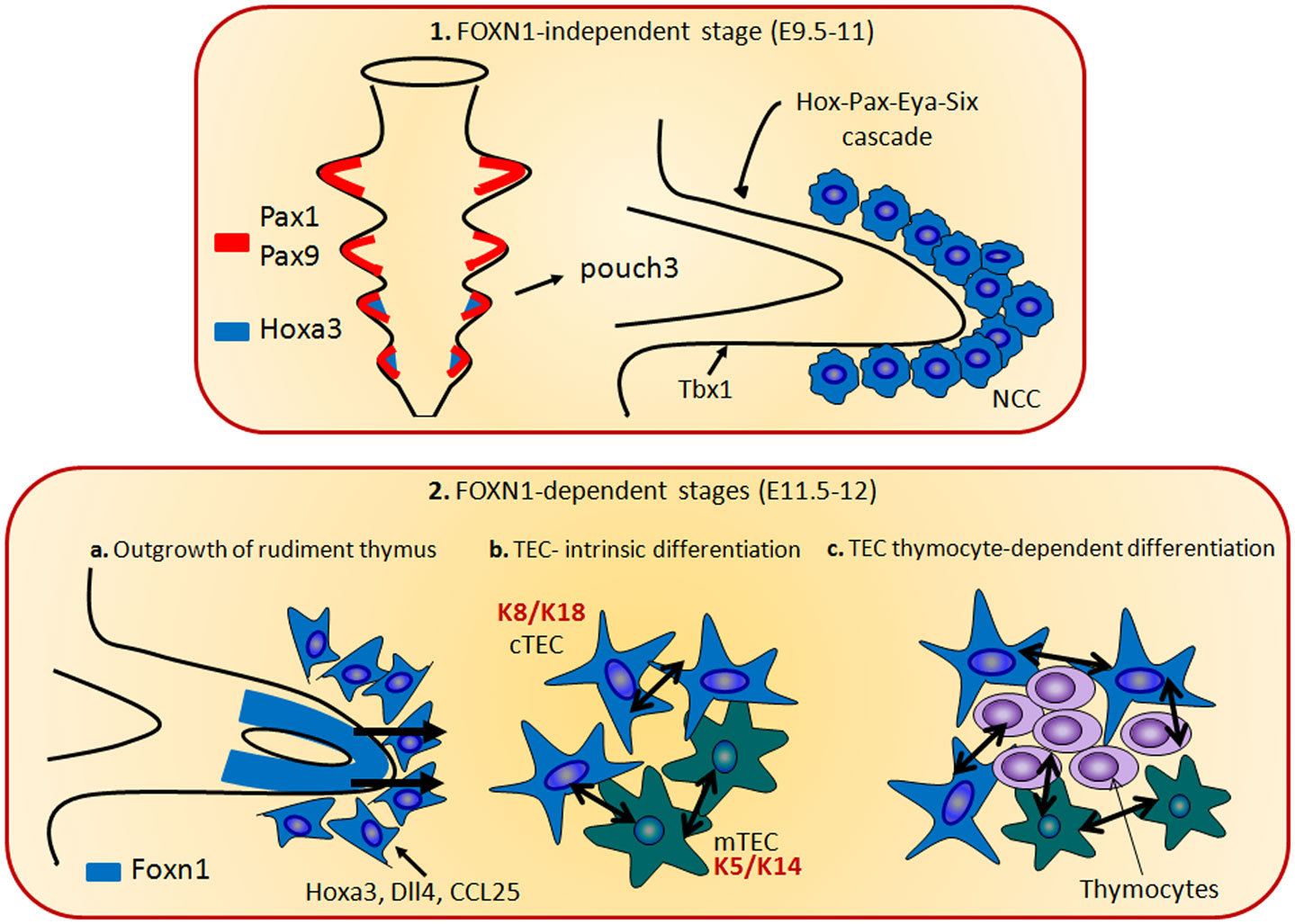
Figure 2. The thymus organogenesis. The thymus organogenesis is characterized by two genetic phases. The first stage is independent from the Foxn1 expression (1) and consists in the induction and outgrowth of the thymic epithelial anlage from the third pharyngeal pouch, through the expression of genes including the Eya1, Six, Hoxa3, and Tbx1. Hoxa3 and Eya1 are also required in neural crest cells (NCCs). In the phase 2, Foxn1 regulates the expression of CCL25, Dll4, and Hoxa3, necessary for the thymocytes and TECs differentiation. During this step, cTECs (expressing K8 and K18 keratin type) and mTECs (expressing K5 and K14 keratin type) originate from the same bi-potential TEC progenitor. The crosstalk between TECs and developing thymocytes is required to generate fully mature TECs and functional T cells.
It has also been shown that Foxn1 regulates the expression of CCL25 and Dll4 (Figure 2). These genes play a pivotal role in the thymocyte development, since CCL25 regulates the colonization of the fetal thymus (97), while the Notch ligand Dll4 is involved in the commitment of hematopoietic progenitors to the T cell lineage (30). In both early fetal TEC and in the post-natal thymus, Dll4 expression is directly related to the Foxn1 expression (71). Furthermore, these molecules are absent in the Foxn1 null thymus, even though there is evidence indicating that their expression may occur in a Foxn1-independent manner in TECs (98, 99). Eventually, in a recent report it has been shown that Foxn1 is upstream of dll4a and ccl25a expression in medaka fish, thus confirming the relationship with this transcription factor (100).
The Human Nude/SCID Phenotype: A Model of Thymic Microenvironment Disruption and Failure of the T Cell Development
The Nude/severe combined immunodeficiency (SCID) phenotype represents the prototype of thymic architecture disruption due to alterations of the FOXN1, which is the master regulator of TE-lineage specification (71).
In humans, as in mice and rats, mutations in the “nude” Foxn1 gene induce the hairless phenotype, associated with a rudimentary thymus gland (T cell related primary immunodeficiency). The human Nude/SCID phenotype (MIM 601705; Pignata Guarino Syndrome) was first identified in 1996, after more than 30 years from the initial mouse description, in two sisters originated from a small community with a high grade of inbreeding, who showed congenital alopecia of the scalp, eyebrows, and eyelashes, nail dystrophy, and a severe T cell immunodeficiency, inherited as an autosomal recessive disorder (154). This phenotype was associated with a C792T transition in the FOXN1 gene, which resulted in the nonsense mutation R255X in the exon 4 (formerly exon 5), with a complete absence of a functional protein similar to the previously described rat and mouse Foxn1 mutations (101–103).
In the absence of Foxn1 expression, thymic development is halted at a rudimentary stage. As a consequence, in the affected patients the thymic lobe is still present but intra-thymic lymphopoiesis is completely blocked (63, 104) leading to severe primary T cell immunodeficiency (105–107) and to death in early childhood from severe infections (105, 108–112, 154). Foxn1 is also involved in morphogenesis and maintenance of the 3D thymic micro-structure, which is necessary for a fully functional thymus (113, 114). In fact, evidence is available that in an in vitro 2D culture system consisting of a monolayer of mouse bone marrow stromal OP9 cells it is possible to generate mature T cells, only if these cells are transduced with the Notch ligand Delta-like 1 (OP9-DL1) (115, 116), whose pathway exerts a pivotal and necessary role in promoting the induction of T cell-lineage commitment (117–119). Of note, in all these co-culture systems, the stromal cells are enforced to overexpress Notch ligands, and their expression by TECs seems to be maintained only in a 3D thymus structure (120). In human Nude/SCID, the T cell defect is characterized by the absence of proliferative response to the common mitogens and a severe blockage of the T cell differentiation (154). Recent studies revealed the presence of some circulating T cells of non-maternal origin in patients carrying alterations of FOXN1 gene. These cells have been shown to be predominantly double-negative αβ T cells (CD3+CD4−CD8−, DN) and to exhibit a regulatory like T cell phenotype (FoxP3+). This finding raised important issues regarding the site of differentiation of these cells. One hypothesis is the persistence of a thymic rudiment, which allows a partial T cell development (109). Alternatively, a T cell differentiation, even though partial and ineffective to result in a productive immunity, could occur at an extra-thymic site. In both pre-natal and post-natal life, the TCRBV spectratype repertoire in Nude/SCID patients is oligoclonal, thus confirming the immaturity of the process and, at the same time, that developmental events do take place at some extent (111, 112).
For many years, the human counterpart of the nude mouse phenotype has been erroneously considered the DiGeorge syndrome, which occurs spontaneously and is mainly characterized by thymic hypo/aplasia and a mild T cell defect. However, several lines of evidence argue against the analogy between these two disorders. In fact, the DiGeorge syndrome is often associated with neonatal tetany and major anomalies of great vessels. These defects are due to malformation of the parathyroid and heart, derived from a major embryologic defect in the third and fourth pharyngeal pouch from which the thymus primordium emerges. In addition, in this syndrome hairlessness is missing and gross abnormalities of skin annexa are not found. Children with DiGeorge syndrome may also have lymphopenia, with a mild reduction of T cells, that are however usually responsive to common mitogens.
In Nude/SCID patients, skin is tighter than usual and is characterized by basal hyperplasia and dysmaturity. Alopecia is primitive in nature, in that it can be observed at birth and persists after bone marrow transplantation, thus ruling out the acquired nature of the disorder. In keeping with this, in athymic mice, completely lacking body hair, restoration of the thymus did not lead to hair growth, indicating a direct participation of FOXN1 to hair follicle development (87). The most frequent phenotypic alteration affecting the nails is koilonychia (“spoon nail”), characterized by a concave surface and raised edges of the nail plate, associated with significant thinning of the plate itself; canaliform dystrophy and a transverse groove of the nail plate (Beau line) may also be observed (121). However, the most specific phenotypic alteration is leukonychia, characterized by a typical arciform pattern resembling a half-moon and involving the proximal part of the nail plate. These alterations of digits and nails were also reported in a few strains of nude mice. Of note, nail dystrophy has also been observed in heterozygous subjects carrying FOXN1 alterations (121). FOXN1 is known to be selectively expressed in the nail matrix, where the nail plate originates, thus confirming that this transcription factor is involved in the maturation process of nails and suggesting nail dystrophy as an indicative sign of heterozygosity for this molecular alteration (121).
Autoptical study of a fetus homozygous for R255X mutation revealed multiple-site neural tube defects, including anencephaly and spina bifida. This finding may help explaining the high rate of mortality in utero observed in the population where the first patients were identified (105). Intriguingly, the other forms of SCID become clinically evident only during the post-natal life, when the protection of the newborn transferred from the mother immune system declines. This observation, suggests that other causes different from immunodeficiency, are responsible for the high rate of mortality in utero and led to consider the Nude/SCID mutation and anencephaly causally related. Of note, in a recent study, the mouse Foxn1 gene was found to be expressed also in epithelial cells of the developing choroids plexus, a structure filling the lateral, third and fourth ventricles of the embryonic brain (105). Moreover abnormality in the development of corpus callosum were also found in another FOXN1 mutated fetus even in the absence of anencephaly, indicating that the transcription factor may play a role as a co-factor in the brain ontogeny (105).
Altogether these findings suggest that FOXN1 may also be implicated as co-factor in the development of vital systems required for a proper fetus development, thus explaining the mortality in the first trimester in fetuses carrying the genetic alterations, which is not justified by the SCID per se.
FOXN1 Mutation Prevents the Pre-Natal T Cell Development in Humans
It is now clear that FOXN1 acts as a transcription factor implicated in the differentiation of thymic and skin epithelial cells, even though many of its molecular targets still remain to be discovered. Most of the knowledge so far available has been achieved in humans in the post-natal life, while little is known about FOXN1 role during the pre-natal life.
Of note, other FOX family members, including Foxq1 and Foxm1b, are important during embryogenesis, being involved in a variety of biological processes (122). Approximately 50% of Foxq1−/− murine embryos die in utero, thus suggesting the requirement of this gene during embryogenesis (123). Similarly, Foxm1b is important during liver regeneration (124).
Studies on thymus organogenesis revealed that Foxn1 is expressed in all TECs during fetal stages. Of note, Foxn1−/− mice showed undifferentiated TECs responsible for a blockage of thymopoiesis and severe immunodeficiency (125). Recently, the identification of a human FOXN1−/− fetus gave the unique opportunity to study in humans the T cell development in utero, in the absence of a functional thymus. Vigliano et al. documented a total blockage of the CD4+ T cell maturation and a severe impairment of CD8+ cells, with an apparent bias toward TCRγδ+ cells (112). In this case in the congenital absence of the thymus was due to R255X missense mutation in the FOXN1 gene. In particular, it has been reported that in the absence of FOXN1 a few not functional CD8+ cells, mostly bearing TCRγδ in the absence of CD3, presumably of extra-thymic origin could develop in both humans and mice (126–128). Further analysis of the fetal RNA, performed to evaluate the variable-domain β-chain (Vβ) families’ usage among T lymphocytes, revealed that the generation of TCR diversity occurred at some extent in the FOXN1−/− fetus, but was abnormal. Thus, these data provided a further evidence of the crucial role for FOXN1 in the early pre-natal stages of T cell development and not in the B and NK-cell differentiation, these populations being normally present in the Nude/SCID fetus (112). A similar impairment of the T cell differentiation with a selective blockage of CD4 differentiation but not of CD8, was detected in murine models characterized by the absence of the nuclear high-mobility group (HMG) box protein TOX (107).
The identification of a limited number of CD8+ cells bearing the TCRγδ suggests that this cell population may develop at extra-thymic sites in a FOXN1-independent manner, even though they are unable to sustain a productive immune response into the periphery. Indeed, evidence exists indicating that T cells may also differentiate at extra-thymic sites, as intestine and liver (129–133). Of note, the majority of thymus-derived T lymphocytes bears the αβ chains of TCR and a few of them express the γδ heterodimer (134), while the T cell pool developed outside the thymus is characterized by a higher proportion of TCRγδ+ T cells expressing the CD8αα homodimer, instead of the CD8αβ (135, 136). Moreover, also DN T cells (CD3+CD4–CD8–) and lymphocytes expressing CD7 and CD2 in the absence of CD3 (CD2+CD3–CD7+) are generally considered of extra-thymic origin (135–137).
In spite of the well documented knowledge on the role of the primary lymphoid organ to foster T cell development, some still unsolved issues in human athymic conditions indicate that an in-depth information of the overall process is still to be achieved and, in particular, the involvement of different tissues in T cell ontogeny must be definitively clarified. Since FOXN1 is selectively expressed in the thymus and skin, one possibility to explain the presence of the few non-functional CD8+TCRγδ+ cells in Nude/SCID fetus is that skin epithelial cells could play a partial role in T cell ontogeny, as already shown in in vitro models (138, 139).
Thymus Transplantation: A Promising Treatment to Athymic Disorders
Forkhead-box n1 deficiency is a very rare immunodeficiency with unfortunately poor chance of curative treatments. Recently, thymus transplantation has emerged as a promising treatment for children affected with congenital athymia (140–143), as that observed in complete DiGeorge anomaly and in FOXN1 deficiency. Conceptually, the thymus transplant seems to be in principle the more appropriate therapeutic strategy, taking into account that bone marrow transplantation performed in one child with FOXN1 deficiency, failed to induce a long-term sustained immune reconstitution. In particular, in this patient no reconstitution of the naïve T cell pool was observed (144).
Thymus transplantation has been first used in children affected with complete DiGeorge anomaly, with excellent clinical and immunologic results (141). In order to achieve immune reconstitution, cultured post-natal allogeneic thymus tissue slices were transplanted into the quadriceps muscles of the athymic host (145). The migration of host bone marrow stem cells to the donor graft allow them to develop into naive T cells, which then emigrate out of the engrafted thymic tissue into the peripheral blood. Thymopoiesis is observed in biopsies of the transplanted thymus within 2 months of transplantation (140) and naive T cells are detected in the peripheral blood approximately 3–5 months after transplantation (146, 147). Taking advantage from this previous experience, a few years ago an allogeneic thymus transplantation has been used for the first time in two unrelated infants with Nude/SCID phenotype due to a deficiency of the transcription factor FOXN1 (111). The clinical phenotype of the two subjects was characterized by the absence of naïve T cells, total alopecia, nail dystrophy, and severe infections, as disseminated Bacillus Calmette–Guérin in subject 1 and severe respiratory infections in subject 2. Molecular analysis, performed to confirm the clinical suspect of the Nude/SCID phenotype, revealed the presence of a homozygous R255X mutation in the FOXN1 gene in subject 1, the same of that previously described (107), and a homozygous R320W novel missense mutation in the subject 2. Moreover, subject 1 showed, like a small percentage of complete DiGeorge patients, referred as atypical complete DiGeorge, circulating oligoclonal T cells of non-maternal origins, which were predominantly double-negative T cells, and a T cell proliferative response to PHA within the normal range. Because of that, before thymus transplantation subject 1 have required immunosuppression regimen to prevent graft rejection. Differently, immunosuppression was not used for the subject 2, who had, like typical complete DiGeorge patients, very few T cells (141, 146).
Results obtained with thymus transplantation were encouraging in both FOXN1-deficient subjects, and led to a full T and B cell reconstitution and functional rescue. Indeed, both subjects developed naïve T cells, diverse TCR repertoires and an in vitro proliferative T cell responses against different antigens. Eventually they reached normal serum Ig levels with generation of protective antibody specific titers. Of note, HLA matching for class I and II did not seem to interfere with T cell counts after thymus transplantation, being subject 2 transplanted without any HLA matches. However, CD8+ T cell number, although apparently functional, was disproportionally low compared to CD4+ T cells (111). A poor CD8 recovery has also been described in complete DiGeorge patients, who underwent HLA-mismatched thymic transplantation (141, 148). Possible explanations are that the phenomenon is related to the HLA mismatch between host hematopoietic precursors and allograft thymic epithelia or to alterations in the thymic graft due to transplantation procedures.
Functionality of the thymic allograft has been assessed for the first time through signal joint (sj) and DβJβ T cell receptor rearrangement excision circle (TREC) analyses (109). The sj/βTREC represents a ratio between early and late products of TCR rearrangements, which directly correlate with thymic output and provide an indirect measurement of thymocyte division-rate (149–151). The sj/βTREC ratio quantification, conducted in subject 1 with R255X mutation, was very low during the peri-transplant period and comparable to those observed in healthy children at 2.5 years post-transplant. Of note, 4 years post-transplantation a decrease of sj/βTREC ratio associated with a reduction in sjTREC levels and in the number of naïve cells were found, suggesting the decline in thymic allograft output (109). This decline might be due to the reduced longevity of the thymus allograft or to peripheral homeostasis of the T cell pool maintenance following its replenishment. Overall, the thymus transplantation seems to be a promising curative strategy for subjects with athymia due to FOXN1 deficiency or complete DiGeorge syndrome in the perspective of long-term clinical benefit.
Conclusion
The integrity of the thymic epithelial architecture allows the growth, the differentiation, and TCR repertoire selection of immature T cells, thus originating fully mature and functional T cells. Of note, the failure to generate or to maintain the proper 3D thymic architecture leads to severe immunodeficiency or autoimmunity. The unique function of the thymus in the establishment/maintenance of the T cell pool is related not only to the peculiar 3D structure, but also to the specialized functions of the thymic stroma. Indeed, lympho-stromal interactions within the multicellular thymic microenvironment play a crucial role in the regulation of the T cell development. Moreover, these interactions are based on a bilateral crosstalk between stromal cells and traveling thymocytes, which, in turn, are able to provide important signals for the TECs differentiation.
Thymus organogenesis and T cell development are sophisticated biological processes, which require the activation of a wide panel of genes. There is evidence that the master regulator of the thymus development is the Foxn1 gene, since it is required at multiple intermediate stages of the TE-lineage specification either in the fetal and adult thymus, through the direct or indirect regulation of genes involved in the thymus development and function. These genes include Pax1, Hoxa3, CCL25, Dll4, p63.
Studies on the animal and human model of the Nude/SCID phenotype have provided an enormous contribution in identifying the crucial role of Foxn1 to drive the thymus development, even though many issues regarding the transcriptional regulation of the TECs specification and homeostasis still remain to be solved. The development in vitro of cellular models of TEC lineage differentiation, by using the technology of nuclear reprograming, will be certainly useful to better characterize the discrete stages of the TECs differentiation and the molecular mechanism involved in the process.
Eventually, the in vitro re-build of a thymic environment capable to reproduce tissue features of primary lymphoid organs (139, 152) could be a promising and valuable tool for the treatment of congenital athymia, including FOXN1 deficiency, along with the thymus transplantation, which is emerged as a potential treatment for these disorders.
Conflict of Interest Statement
The authors declare that the research was conducted in the absence of any commercial or financial relationships that could be construed as a potential conflict of interest.
References
1. Anderson G, Jenkinson EJ. Lymphostromal interactions in thymic development and function. Nat Rev Immunol (2001) 1:31–40. doi:10.1038/35095500
2. Gray DH, Chidgey AP, Boyd RL. Analysis of thymic stromal cell populations using flow cytometry. J Immunol Methods (2002) 260:15–28. doi:10.1016/S0022-1759(01)00493-8
3. Jenkinson EJ, Anderson G. Fetal thymic organ cultures. Curr Opin Immunol (1994) 6:293–7. doi:10.1016/0952-7915(94)90104-X
4. Rodewald HR. Thymus organogenesis. Annu Rev Immunol (2008) 26:355–88. doi:10.1146/annurev.immunol.26.021607.090408
5. Kyewski B, Derbinski J, Gotter J, Klein L. Promiscuous gene expression and central T-cell tolerance: more than meets the eye. Trends Immunol (2002) 23:364–71. doi:10.1016/S1471-4906(02)02248-2
6. Martin CH, Aifantis I, Scimone ML, von Andrian UH, Reizis B, von Boehmer H, et al. Efficient thymic immigration of B220+ lymphoid-restricted bone marrow cells with T precursor potential. Nat Immunol (2003) 4:866–73. doi:10.1038/ni965
7. Martins VC, Ruggiero E, Schlenner SM, Madan V, Schmidt M, Fink PJ, et al. Thymus-autonomous T cell development in the absence of progenitor import. J Exp Med (2012) 209:1409–17. doi:10.1084/jem.20120846
8. Petrie HT, Zúñiga-Pflücker JC. Zoned out: functional mapping of stromal signaling microenvironments in the thymus. Annu Rev Immunol (2007) 25:649–79. doi:10.1146/annurev.immunol.23.021704.115715
9. Farley AM, Morris LX, Vroegindeweij E, Depreter ML, Vaidya H, Stenhouse FH, et al. Dynamics of thymus organogenesis and colonization in early human development. Development (2013) 140:2015–26. doi:10.1242/dev.087320
10. Duijvestijn AM, Hoefsmit EC. Ultrastructure of the rat thymus: the micro-environment of T-lymphocyte maturation. Cell Tissue Res (1981) 218:279–92. doi:10.1007/BF00210344
11. van Ewijk W. T-cell differentiation is influenced by thymic microenvironments. Ann Rev Immunol (1991) 9:591–615. doi:10.1146/annurev.iy.09.040191.003111
12. Dorshkind K. Regulation of hemopoiesis by bone marrow stromal cells and their products. Annu Rev Immunol (1990) 8:111–37. doi:10.1146/annurev.immunol.8.1.111
13. Manley NR, Blackburn CC. A developmental look at thymus organogenesis: where do the non-hematopoietic cells in the thymus come from? Curr Opin Immunol (2003) 15:225–32. doi:10.1016/S0952-7915(03)00006-2
14. van Ewijk W, Shores EW, Singer A. Crosstalk in the mouse thymus. Immunol Today (1994) 15:214–7. doi:10.1016/0167-5699(94)90246-1
15. van Ewijk W, Wang B, Hollander G, Kawamoto H, Spanopoulou E, Itoi M, et al. Thymic microenvironments, 3-D versus 2-D? Semin Immunol (1999) 11:57–64. doi:10.1006/smim.1998.0158
16. Palmer DB, Viney JL, Ritter MA, Hayday AC, Owen MJ. Expression of the alpha beta T-cell receptoris necessary for the generation of the thymic medulla. Dev Immunol (1993) 3:175–9. doi:10.1155/1993/56290
17. Shores EW, van Ewijk W, Singer A. Maturation of medullary thymic epithelium requires thymocytes expressing fully assembled CD3-TCR complexes. Int Immunol (1994) 6:1393–402. doi:10.1093/intimm/6.9.1393
18. Surh CD, Ernst B, Sprent J. Growth of epithelial cells in the thymic medulla is under the control of mature T cells. J Exp Med (1992) 176:611–6. doi:10.1084/jem.176.2.611
19. Hollander GA, Wang B, Nichogiannopoulou A, Platenburg PP, van Ewijk W, Burakoff SJ, et al. Developmental control point in induction of thymic cortex regulated by a subpopulation of prothymocytes. Nature (1995) 373:350–3. doi:10.1038/373350a0
20. Roberts NA, Desanti GE, Withers DR, Scott HR, Jenkinson WE, Lane PJL, et al. Absence of thymus crosstalk in the fetus does not preclude hematopoietic induction of a functional thymus in the adult. Eur J Immunol (2009) 39:2395–402. doi:10.1002/eji.200939501
21. Germeraad WT, Kawamoto H, Itoi M, Jiang Y, Amagai T, Katsura Y, et al. Development of thymic microenvironments in vitro is oxygen-dependent and requires permanent presence of T-cell progenitors. J Histochem Cytochem (2003) 51:1225–35. doi:10.1177/002215540305100913
22. Gray DH, Ueno T, Chidgey AP, Malin M, Goldberg GL, Takahama Y, et al. Controlling the thymic microenvironment. Curr Opin Immunol (2005) 17:137–43. doi:10.1016/j.coi.2005.02.001
23. van Ewijk W, Hollander G, Terhorst C, Wang B. Stepwise development of thymic microenvironments in vivo is regulated by thymocyte subsets. Development (2000) 127:1583–91.
24. Haynes BF, Heinly CS. Early human T cell development: analysis of the human thymus at the time of initial entry of hematopoietic stem cells into the fetal thymic microenvironment. J Exp Med (1995) 181:1445–58. doi:10.1084/jem.181.4.1445
25. Owen JJ, Ritter MA. Tissue interaction in the development of thymus lymphocytes. J Exp Med (1969) 129:431–42. doi:10.1084/jem.129.2.431
26. Calderon L, Boehm T. Three chemokine receptors cooperatively regulate homing of hematopoietic progenitors to the embryonic mouse thymus. Proc Natl Acad Sci U S A (2011) 108:7517–22. doi:10.1073/pnas.1016428108
27. Liu C, Saito F, Liu C, Lei Y, Uehara S, Love P, et al. Coordination between CCR7- and CCR9-mediated chemokine signals in prevascular fetal thymus colonization. Blood (2006) 108:2531–9. doi:10.1182/blood-2006-05-024190
28. Wurbel MA, Malissen M, Guy-Grand D, Meffre E, Nussenzweig MC, Richelme M, et al. Mice lacking the CCR9 CC-chemokine receptor show a mild impairment of early T- and B-cell development and a reduction in T-cell receptor gammadelta(+) gut intraepithelial lymphocytes. Blood (2001) 98:2626–32. doi:10.1182/blood.V98.9.2626
29. Rossi FM, Corbel SY, Merzaban JS, Carlow DA, Gossens K, Duenas J, et al. Recruitment of adult thymic progenitors is regulated by P-selectin and its ligand PSGL-1. Nat Immunol (2005) 6:626–34. doi:10.1038/ni1203
30. Koch U, Fiorini E, Benedito R, Besseyrias V, Schuster-Gossler K, Pierres M, et al. Delta-like 4 is the essential, nonredundant ligand for Notch1 during thymic T cell lineage commitment. J Exp Med (2008) 205:2515–23. doi:10.1084/jem.20080829
31. Thompson PK, Zúñiga-Pflücker JC. On becoming a T cell, a convergence of factors kick it up a Notch along the way. Semin Immunol (2011) 23:350–9. doi:10.1016/j.smim.2011.08.007
32. Hozumi K, Mailhos C, Negishi N, Hirano K, Yahata T, Ando K, et al. Delta-like 4 is indispensable in thymic environment specific for T cell development. J Exp Med (2008) 205:2507–13. doi:10.1084/jem.20080134
33. Lind EF, Prockop SE, Porritt HE, Petrie HT. Mapping precursor movement through the postnatal thymus reveals specific microenvironments supporting defined stages of early lymphoid development. J Exp Med (2001) 194:127–34. doi:10.1084/jem.194.2.127
34. Benz C, Heinzel K, Bleul CC. Homing of immature thymocytes to the subcapsular microenvironment within the thymus is not an absolute requirement for T cell development. Eur J Immunol (2004) 34:3652–63. doi:10.1002/eji.200425248
35. Yamasaki S, Saito T. Molecular basis for pre-TCR-mediated autonomous signaling. Trends Immunol (2007) 28:39–43. doi:10.1016/j.it.2006.11.006
36. Goldrath AW, Bevan MJ. Selecting and maintaining a diverse T-cell repertoire. Nature (1999) 402:255–62. doi:10.1038/46218
37. Palmer E. Negative selection – clearing out the bad apples from the T-cell repertoire. Nat Rev Immunol (2003) 3:383–91. doi:10.1038/nri1085
38. Laufer TM, DeKoning J, Markowitz JS, Lo D, Glimcher LH. Unopposed positive selection and autoreactivity in mice expressing class II MHC only on thymic cortex. Nature (1996) 383:81–5. doi:10.1038/383081a0
39. Murata S, Sasaki K, Kishimoto T, Niwa S, Hayashi H, Takahama Y, et al. Regulation of CD8+ T cell development by thymus-specific proteasomes. Science (2007) 316:1349–53. doi:10.1126/science.1141915
40. Ripen AM, Nitta T, Murata S, Tanaka K, Takahama Y. Ontogeny of thymic cortical epithelial cells expressing the thymoproteasome subunit b5t. Eur J Immunol (2011) 41:1278–87. doi:10.1002/eji.201041375
41. Nitta T, Murata S, Sasaki K, Fujii H, Ripen AM, Ishimaru N, et al. Thymoproteasome shapes immunocompetent repertoire of CD8+ T cells. Immunity (2010) 32:29–40. doi:10.1016/j.immuni.2009.10.009
42. Gommeaux J, Gržgoire C, Nguessan P, Richelme M, Malissen M, Guerder S, et al. Thymus-specific serine protease regulates positive selection of a subset of CD4+ thymocytes. Eur J Immunol (2009) 39:956–64. doi:10.1002/eji.200839175
43. Viret C, Lamare C, Guiraud M, Fazilleau N, Bour A, Malissen B, et al. Thymus-specific serine protease contributes to the diversification of the functional endogenous CD4 T cell receptor repertoire. J Exp Med (2011) 208:3–11. doi:10.1084/jem.20100027
44. Nitta T, Nitta S, Lei Y, Lipp M, Takahama Y. CCR7-mediated migration of developing thymocytes to the medulla is essential for negative selection to tissue-restricted antigens. Proc Natl Acad Sci U S A (2009) 106:17129–33. doi:10.1073/pnas.0906956106
45. Ueno T, Saito F, Gray DH, Kuse S, Hieshima K, Nakano H, et al. CCR7 signals are essential for cortex-medulla migration of developing thymocytes. J Exp Med (2004) 200:493–505. doi:10.1084/jem.20040643
46. Derbinski J, Schulte A, Kyewski B, Klein L. Promiscuous gene expression in medullary thymic epithelial cells mirrors the peripheral self. Nature (2001) 2:1032–9.
47. Mathis D, Benoist C. Aire. Annu Rev Immunol (2009) 27:287–312. doi:10.1146/annurev.immunol.25.022106.141532
48. Hubert FX, Kinkel SA, Davey GM, Phipson B, Mueller SN, Liston A, et al. Aire regulates the transfer of antigen from mTECs to dendritic cells for induction of thymic tolerance. Blood (2011) 118:2462–72. doi:10.1182/blood-2010-06-286393
49. Derbinski J, Pinto S, Ršsch S, Hexel K, Kyewski B. Promiscuous gene expression patterns in single medullary thymic epithelial cells argue for a stochastic mechanism. Proc Natl Acad Sci U S A (2008) 105:657–62. doi:10.1073/pnas.0707486105
50. Gillard GO, Farr AG. Features of medullary thymic epithelium implicate postnatal development in maintaining epithelial heterogeneity and tissue-restricted antigen expression. J Immunol (2006) 176:5815–24.
51. Rooke R, Waltzinger C, Benoist C, Mathis D. Targeted complementation of MHC class II deficiency by intrathymic delivery of recombinant adenoviruses. Immunity (1997) 7:123–34. doi:10.1016/S1074-7613(00)80515-4
52. Klein L. Dead man walking: how thymocytes scan the medulla. Nat Immunol (2009) 10:809–11. doi:10.1038/ni0809-809
53. Koble C, Kyewski B. The thymic medulla: a unique microenvironment for intercellular self-antigen transfer. J Exp Med (2009) 206:1505–13. doi:10.1084/jem.20082449
54. Lei Y, Ripen AM, Ishimaru N, Ohigashi I, Nagasawa T, Jeker LT, et al. Aire-dependent production of XCL1 mediates medullary accumulation of thymic dendritic cells and contributes to regulatory T cell development. J Exp Med (2011) 208:383–94. doi:10.1084/jem.20102327
55. Román E, Shino H, Qin FX, Liu YJ. Hematopoietic-derived APCs select regulatory T cells in thymus. J Immunol (2010) 185:3819–23. doi:10.4049/jimmunol.0900665
56. Itoh M, Takahashi T, Sakaguchi N, Kuniyasu Y, Shimizu J, Otsuka F, et al. Thymus and autoimmunity: production of CD25+CD4+ naturally anergic and suppressive T cells as a key function of the thymus in maintaining immunologic self-tolerance. J Immunol (1999) 162:5317–26.
57. Dieckmann D, Plottner H, Berchtold S, Berger T, Schuler G. Ex vivo isolation and characterization of CD4+CD25+ T cells with regulatory properties from human blood. J Exp Med (2001) 193:1303–10. doi:10.1084/jem.193.11.1303
58. Schubert LA, Jeffery E, Zhang Y, Ramsdell F, Ziegler SF. Scurfin (FOXP3) acts as a repressor of transcription and regulates T cell activation. J Biol Chem (2001) 276:37672–9. doi:10.1074/jbc.M104521200
59. Liu B, Tahk S, Yee KM, Fan G, Shuai K. The ligase PIAS1 restricts natural regulatory T cell differentiation by epigenetic repression. Science (2010) 330:521–5. doi:10.1126/science.1193787
60. Yao Z, Kanno Y, Kerenyi M, Stephens G, Durant L, Watford WT, et al. Nonredundant roles for Stat5a/b in directly regulating Foxp3. Blood (2007) 109:4368–75. doi:10.1182/blood-2006-11-055756
61. Schmidt-Supprian M, Tian J, Grant EP, Pasparakis M, Maehr R, Ovaa H, et al. Differential dependence of CD4+CD25+ regulatory and natural killer like T cells on signals leading to NF-kappaB activation. Proc Natl Acad Sci U S A (2004) 101:4566–71. doi:10.1073/pnas.0400885101
62. Bleul CC, Boehm T. Chemokines define distinct microenvironments in the developing thymus. Eur J Immunol (2000) 30:3371–9. doi:10.1002/1521-4141(2000012)30:12<3371::AID-IMMU3371>3.0.CO;2-L
63. Nehls M, Kyewski B, Messerle M, Waldschutz R, Schuddekopf K, Smith AJ, et al. Two genetically separable steps in the differentiation of thymic epithelium. Science (1996) 272:886–9. doi:10.1126/science.272.5263.886
64. Pignata C. A lesson to unraveling complex aspects of novel immunodeficiencies from the human equivalent of the nude/SCID phenotype. J Hematother Stem Cell Res (2002) 11:409–14. doi:10.1089/152581602753658592
65. Pignata C, D’Agostino A, Finelli P, Fiore M, Scotese I, Cosentini E, et al. Progressive deficiencies in blood T cells associated with a 10p12-13 interstitial deletion. Clin Immunol Immopathol (1996) 80:9–15. doi:10.1006/clin.1996.0088
66. Su D, Navarre S, Oh W, Condie BG, Manley NR. A domain of Foxn1 required for crosstalk-dependent thymic epithelial cell differentiation. Nat Immunol (2003) 4:1128–35. doi:10.1038/ni983
67. Zou D, Silvius D, Davenport J, Grifone R, Maire P, Xu P-X. Patterning of the third pharyngeal pouch into thymus/parathyroid by Six and Eya1. Dev Biol (2006) 293:499–512. doi:10.1016/j.ydbio.2005.12.015
68. Manley NR, Capecchi MR. The role of Hoxa-3 in mouse thymus and thyroid development. Development (1995) 121:1989–2003.
69. Jerome LA, Papaioannou VE. DiGeorge syndrome phenotype in mice mutant for the T-box gene, Tbx1. Nat Genet (2001) 27:286–91. doi:10.1038/85845
70. Lindsay EA. Chromosomal microdeletions: dissecting del22q11 syndrome. Nat Rev Genet (2001) 2:858–68. doi:10.1038/35098574
71. Nowell CS, Bredenkamp N, Tetelin S, Jin X, Tischner C, Vaidya H, et al. Foxn1 regulates lineage progression in cortical and medullary thymic epithelial cells but is dispensable for medullary sublineage divergence. PLoS Genet (2011) 7:e1002348. doi:10.1371/journal.pgen.1002348
72. Rossi SW, Jenkinson WE, Anderson G, Jenkinson EJ. Clonal analysis reveals a common progenitor for thymic cortical and medullary epithelium. Nature (2006) 441:988–91. doi:10.1038/nature04813
73. Owen JJ, McLoughlin DE, Suniara RK, Jenkinson EJ. The role of mesenchyme in thymus development. Curr Top Microbiol Immunol (2000) 251:133–7. doi:10.1007/978-3-642-57276-0_17
74. Zhang L, Sun L, Zhao Y. Thymic epithelial progenitor cells and thymus regeneration: an update. Cell Res (2007) 17:50–5. doi:10.1038/sj.cr.7310114
75. Bennett AR, Farley A, Blair NF, Gordon J, Sharp L, Blackburn CC. Identification and characterization of thymic epithelial progenitor cells. Immunity (2002) 16:803–14. doi:10.1016/S1074-7613(02)00321-7
76. Bleul C, Corbeaux T, Reuter A, Fisch P, Schulte Monting J, Boehm T. Formation of a functional thymus initiated by a postnatal epithelial progenitor cell. Nature (2006) 441:992–6. doi:10.1038/nature04850
77. Manley NR, Condie BG. Transcriptional regulation of thymus organogenesis and thymic epithelial cell differentiation. Prog Mol Biol Transl Sci (2010) 92:103–20. doi:10.1016/S1877-1173(10)92005-X
78. Dietrich S, Gruss P. Undulated phenotypes suggest a role of Pax-1 for the development of vertebral and extravertebral structures. Dev Biol (1995) 167:529–48. doi:10.1006/dbio.1995.1047
79. Hetzer-Egger C, Schorpp M, Haas-Assenbaum A, Balling R, Peters H, Boehm T. Thymopoiesis requires Pax9 function in thymic epithelial cells. Eur J Immunol (2002) 32:1175–81. doi:10.1002/1521-4141(200204)32:4<1175::AID-IMMU1175>3.0.CO;2-U
80. Su DM, Manley NR. Hoxa3 and pax1 transcription factors regulate the ability of fetal thymic epithelial cells to promote thymocyte development. J Immunol (2000) 164:5753–60.
81. Mansouri A, Goudreau G, Gruss P. Pax genes and their role in organogenesis. Cancer Res (1999) 59:1707s–9.
82. Merscher S, Funke B, Epstein JA, Heyer J, Puech A, Lu MM, et al. TBX1 is responsible for cardiovascular defects in velo-cardio-facial/DiGeorge syndrome. Cell (2001) 104:619–29. doi:10.1016/S0092-8674(01)00247-1
83. Liao J, Kochilas L, Nowotschin S, Arnold JS, Aggarwal VS, Epstein JA, et al. Full spectrum of malformations in velo-cardio-facial syndrome/DiGeorge syndrome mouse models by altering Tbx1 dosage. Hum Mol Genet (2004) 13:1577–85. doi:10.1093/hmg/ddh176
84. Kaufmann E, Knochel W. Five years on the wings of fork head. Mech Dev (1996) 57:3–20. doi:10.1016/0925-4773(96)00539-4
85. Schorpp M, Hofmann M, Dear TN, Boehm T. Characterization of mouse and human nude genes. Immunogenetics (1997) 46:509–15. doi:10.1007/s002510050312
86. Brissette JL, Li J, Kamimura J, Lee D, Dotto GP. The product of the mouse nude locus, Whn, regulates the balance between epithelial cell growth and differentiation. Genes Dev (1996) 10:2212–21. doi:10.1101/gad.10.17.2212
87. Mecklenburg L, Tychsen B, Paus R. Learning from nudity: lessons from the nude phenotype. Exp Dermatol (2005) 14:797–810. doi:10.1111/j.1600-0625.2005.00362.x
88. Balciunaite G, Keller MP, Balciunaite E, Piali L, Zuklys S, Mathieu YD, et al. Wnt glycoproteins regulate the expression of FoxN1, the gene defective in nude mice. Nat Immunol (2002) 3:1102–8. doi:10.1038/ni850
89. Bleul CC, Boehm T. BMP signaling is required for normal thymus development. J Immunol (2005) 175:5213–21.
90. Nehls M, Pfeifer D, Schorpp M, Hedrich H, Boehm T. New member of the winged-helix protein family disrupted in mouse and rat nude mutations. Nature (1994) 372:103–7. doi:10.1038/372103a0
91. Cheng L, Guo J, Sun L, Fu J, Barnes PF, Metzger D, et al. Postnatal tissue-specific disruption of transcription factor FoxN1 triggers acute thymic atrophy. J Biol Chem (2010) 285:5836–47. doi:10.1074/jbc.M109.072124
92. Corbeaux T, Hess I, Swann JB, Kanzler B, Haas-Assenbaum A, Boehm T. Thymopoiesis in mice depends on a Foxn1-positive thymic epithelial cell lineage. Proc Natl Acad Sci U S A (2010) 107:16613–8. doi:10.1073/pnas.1004623107
93. Crum CP, McKeon FD. p63 in epithelial survival, germ cell surveillance, and neoplasia. Annu Rev Pathol (2010) 5:349–71. doi:10.1146/annurev-pathol-121808-102117
94. Candi E, Rufini A, Terrinoni A, Giamboi-Miraglia A, Lena AM, Mantovani R, et al. DeltaNp63 regulates thymic development through enhanced expression of FgfR2 and Jag2. Proc Natl Acad Sci U S A (2007) 104:11999–2004. doi:10.1073/pnas.0703458104
95. Senoo M, Pinto F, Crum CP, McKeon F. p63 is essential for the proliferative potential of stem cells in stratified epithelia. Cell (2007) 129:523–36. doi:10.1016/j.cell.2007.02.045
96. Wallin J, Eibel H, Neubuser A, Wilting J, Koseki H, Balling R. Pax1 is expressed during development of the thymus epithelium and is required for normal T-cell maturation. Development (1996) 122:23–30.
97. Liu H, Leung BP. CD4+CD25+ regulatory T cells in health and disease. Clin Exp Pharmacol Physiol (2006) 33:519–24. doi:10.1111/j.1440-1681.2006.04401.x
98. Itoi M, Tsukamoto N, Amagai T. Expression of Dll4 and CCL25 in Foxn1-negative epithelial cells in the post-natal thymus. Int Immunol (2007) 19:127–32. doi:10.1093/intimm/dxl129
99. Tsukamoto N, Itoi M, Nishikawa M, Amagai T. Lack of Delta like 1 and 4 expressions in nude thymus anlages. Cell Immunol (2005) 234:77–80. doi:10.1016/j.cellimm.2005.06.009
100. Bajoghli B, Aghaallaei N, Hess I, Rode I, Netuschil N, Tay BH, et al. Evolution of genetic networks underlying the emergence of thymopoiesis in vertebrates. Cell (2009) 138:186–97. doi:10.1016/j.cell.2009.04.017
101. Festing MFW, May D, Connors TA, Lovell D, Sparrow S. An athymic nude mutation in the rat. Nature (1978) 274:365–6. doi:10.1038/274365a0
102. Hoffman SR, Ettinger R, Zhou YJ, Gadina M, Lipsky P, Siegel R, et al. Cytokines and their role in lymphoid development, differentiation and homeostasis. Curr Opin Allergy Clin Immunol (2002) 2:495–506. doi:10.1097/00130832-200212000-00004
103. Huth M, Schlake T, Boehm T. Transposon-induced splicing defect in the rat nude gene. Immunogenetics (1997) 45:282–3. doi:10.1007/s002510050206
104. Blackburn CC, Augustine CL, Li R, Harvey RP, Malin MA, Boyd RL, et al. The nu gene acts cell-autonomously and is required for differentiation of thymic epithelial progenitors. Proc Natl Acad Sci U S A (1996) 93:5742–6. doi:10.1073/pnas.93.12.5742
105. Amorosi S, D’Armiento M, Calcagno G, Russo I, Adriani M, Christiano AM, et al. FOXN1 homozygous mutation associated with anencephaly and severe neural tube defect in human athymic Nude/SCID fetus. Clin Genet (2008) 73:380–4. doi:10.1111/j.1399-0004.2008.00977.x
106. Cunningham-Rundles C, Ponda PP. Molecular defects in T- and B-cell primary immunodeficiency diseases. Nat Rev Immunol (2005) 5:880–92. doi:10.1038/nri1713
107. Frank J, Pignata C, Panteleyev AA, Prowse DM, Baden H, Weiner L, et al. Exposing the human nude phenotype. Nature (1999) 398:473–4. doi:10.1038/18997
108. Adriani M, Martinez-Mir A, Fusco F, Busiello R, Frank J, Telese S, et al. Ancestral founder mutation of the nude (FOXN1) gene in congenital severe combined immunodeficiency associated with alopecia in southern Italy population. Ann Hum Genet (2004) 68:265–8. doi:10.1046/j.1529-8817.2004.00091.x
109. Albuquerque A, Marques JG, Silva SL, Ligeiro D, Devline BH, Dutrieux J, et al. Human FOXN1-deficiency is associated with ab double-negative and FoxP3+ T-cell expansions that are distinctly modulated upon thymic transplantation. PLoS ONE (2012) 7:e37042. doi:10.1371/journal.pone.0037042
110. Coffer PJ, Burgering BMT. Forkhead-box transcription factors and their role in the immune system. Nat Rev Immunol (2004) 4:889–99. doi:10.1038/nri1488
111. Markert ML, Marques J, Neven B, Devlin B, McCarthy E, Chinn I, et al. First use of thymus transplantation therapy for Foxn1 deficiency (nude/SCID): a report of two cases. Blood (2011) 117:688–96. doi:10.1182/blood-2010-06-292490
112. Vigliano I, Gorrese M, Fusco A, Vitiello L, Amorosi S, Panico L, et al. FOXN1 mutation abrogates prenatal T-cell development in humans. J Med Genet (2011) 48:413–6. doi:10.1136/jmg.2011.089532
113. Mohtashami M, Zúñiga-Pflücker JC. Cutting edge: three-dimensional architecture of the thymus is required to maintain delta-like expression necessary for inducing T cell development. J Immunol (2006) 176:730–4.
114. Zúñiga-Pflücker JC. T-cell development made simple. Nat Rev Immunol (2004) 4:67–72. doi:10.1038/nri1257
115. Schmitt TM, Zúñiga-Pflücker JC. Induction of T cell development from hematopoietic progenitor cells by delta-like-1 in vitro. Immunity (2002) 17:749–56. doi:10.1016/S1074-7613(02)00474-0
116. La Motte-Mohs RN, Herer E, Zúñiga-Pflücker JC. Induction of T cell development from human cord blood hematopoietic stem cells by Delta-like 1 in vitro. Blood (2004) 105:1431–9. doi:10.1182/blood-2004-04-1293
117. Osborne B, Miele L. Notch and the immune system. Immunity (1999) 11:653–63. doi:10.1016/S1074-7613(00)80140-5
118. MacDonald HR, Wilson A, Radtke F. Notch1 and T-cell development: insights from conditional knockout mice. Trends Immunol (2001) 22:155–60. doi:10.1016/S1471-4906(00)01828-7
119. Pear WS, Radtke F. Notch signaling in lymphopoiesis. Semin Immunol (2003) 15:69–79. doi:10.1016/S1044-5323(03)00003-4
120. Anderson G, Jenkinson EJ. Investigating central tolerance with reaggregate thymus organ cultures. Methods Mol Biol (2007) 380:185–96. doi:10.1007/978-1-59745-395-0_11
121. Auricchio L, Adriani M, Frank J, Busiello R, Christiano A, Pignata C. Nail distrophy associated with a heterozygous mutation of the Nude/SCID human FOXN1 (WHN) gene. Arch Dermatol (2005) 141:647–8. doi:10.1001/archderm.141.5.647
122. Jonsson H, Peng SL. Forkhead transcription factors in immunology. CMLS Cell Mol Life Sci (2005) 62:397–409. doi:10.1007/s00018-004-4365-8
123. Goering W, Adham IM, Pasche B, Manner J, Ochs M, Engel W, et al. Impairment of gastric acid secretion and increase of embryonic lethality in Foxq1-deficient mice. Cytogenet Genome Res (2008) 121:88–95. doi:10.1159/000125833
124. Krupczak-Hollis K, Wang X, Kalinichenko VV, Gusarova GA, Wang IC, Dennewitz MB, et al. The mouse Forkhead Box m1 transcription factor is essential for hepatoblast mitosis and development of intrahepatic bile ducts and vessels during liver morphogenesis. Develop Biol (2004) 276:74–88. doi:10.1016/j.ydbio.2004.08.022
125. Muller SM, Ege M, Pottharst A, Schulz AS, Schwarz K, Friedrich W. Transplacentally acquired maternal T lymphocytes in severe combined immunodeficiency: a study of 121 patients. Blood (2001) 98:1847–51. doi:10.1182/blood.V98.6.1847
126. Rocha B. The extrathymic T-cell differentiation in the murine gut. Immunol Rev (2007) 215:166–77. doi:10.1111/j.1600-065X.2006.00467.x
127. Jensen KD, Shin S, Chien YH. Cutting edge: Γδ intraepithelial lymphocytes of the small intestine are not biased toward thymic antigens. J Immunol (2009) 182:7348–51. doi:10.4049/jimmunol.0900465
128. Guy-Grand D, Azogui O, Celli S, Darche S, Nussenzweig MC, Kourilsky P, et al. Extrathymic T Cell lymphopoiesis: ontogeny and contribution to gut intraepithelial lymphocytes in athymic and euthymic mice. J Exp Med (2003) 197:333–41. doi:10.1084/jem.20021639
129. Blais M, Louis I, Perreault C. T-cell development: an extrathymic perspective. Immunol Rev (2006) 209:103–14. doi:10.1111/j.0105-2896.2006.00341.x
130. Nonaka S, Naito T, Chen H, Yamamoto M, Moro K, Kiyono H, et al. Intestinal gd T cells develop in mice lacking thymus, all lymphonodes, Peyer’s patches, and isolated lymphoid follicles. J Immunol (2005) 174:1906–12.
131. Peaudecerf L, Ribeiro dos Santos P, Boudil A, Ezine S, Pardigon N, Rocha B. The role of the gut as a primary lymphoid organ: CD8 euthymic mice derive from very immature CD44+ thymocyte precursors. Mucosal Immunol (2011) 4:93–101. doi:10.1038/mi.2010.47
132. Suzuki K, Oida T, Hamada H, Hitotsumatsu O, Watanabe M, Hibi T, et al. Gut cryptopatches: direct evidence of extrathymic anatomical sites for intestinal T lymphopoiesis. Immunity (2000) 13:691–702. doi:10.1016/S1074-7613(00)00068-6
133. Torfadottir H, Freysdottir J, Skaftadottir I, Haraldsson A, Sigfusson G, Ogmundsdottir HM. Evidence for extrathymic T cell maturation after thymectomy in infancy. Clin Exp Immunol (2006) 145:407–12. doi:10.1111/j.1365-2249.2006.03139.x
134. Xiong N, Raulet DH. Development and selection of gammadelta T cells. Immunol Rev (2007) 215:15–31. doi:10.1111/j.1600-065X.2006.00478.x
135. Gunther U, Holloway JA, Gordon JG, Knight A, Chance V, Hanley NA, et al. Phenotypic characterization of CD3-7+ cells in developing human intestine and an analysis of their ability to differentiate into T cells. J Immunol (2005) 174:5414–22.
136. Hayday A, Gibbons D. Brokering the peace: the origin of intestinal T cells. Mucosal Immunol (2008) 1:12–4. doi:10.1038/mi.2008.8
137. Eiras P, Leon F, Camarero C, Lombardia M, Roldan E, Bootello A, et al. Intestinal intraepithelial lymphocytes contain a CD3-CD7+ subset expressing natural killer markers and a singular pattern of adhesion molecules. Scand J Immunol (2000) 52:1–6. doi:10.1046/j.1365-3083.2000.00761.x
138. Aliaamad P, Kaye J. Development of all CD4 T lineages requires nuclear factor TOX. J Exp Med (2008) 205:245–56. doi:10.1084/jem.20071944
139. Palamaro L, Guarino V, Scalia G, Antonini D, De Falco L, Bianchino G, et al. Human skin-derived keratinocytes and fibroblasts co-culture on 3D poly e-caprolactone scaffold support in vitro HSCs differentiation into T-lineage committed cells. Int Immunol (2013). doi:10.1093/intimm/dxt035
140. Markert ML. Treatment of infants with complete DiGeorge anomaly. J Allergy Clin Immunol (2008) 121:1063. doi:10.1016/j.jaci.2007.12.1181
141. Markert ML, Devlin BH, Alexieff MJ, Li J, McCarthy EA, Gupton SE, et al. Review of 54 patients with complete DiGeorge anomaly enrolled in protocols for thymus transplantation: outcome of 44 consecutive transplants. Blood (2007) 109:4539–47. doi:10.1182/blood-2006-10-048652
142. Li B, Li J, Devlin BH, Markert ML. Thymic microenvironment reconstitution after postnatal human thymus transplantation. Clin Immunol (2011) 140:244–59. doi:10.1016/j.clim.2011.04.004
143. Chinn IK, Markert ML. Induction of tolerance to parental parathyroid grafts using allogeneic thymus tissue in patients with DiGeorge anomaly. J Allergy Clin (2011) 127:1351–2. doi:10.1016/j.jaci.2011.03.033
144. Pignata C, Gaetaniello L, Masci AM, Frank J, Christiano A, Matrecano E, et al. Human equivalent of the mouse nude/SCID phenotype: long-term evaluation of immunological reconstitution after bone marrow transplantation. Blood (2001) 97:880–5. doi:10.1182/blood.V97.4.880
145. Rice HE, Skinner MA, Mahaffey SM, Oldham KT, Ing RJ, Hale LP, et al. Thymic transplantation for complete DiGeorge syndrome: medical and surgical considerations. J Pediatr Surg (2004) 39:1607–15. doi:10.1016/j.jpedsurg.2004.07.020
146. Markert ML, Alexieff MJ, Li J, Sarzotti M, Ozaki DA, Devlin BH, et al. Postnatal thymus transplantation with immunosuppression as treatment for DiGeorge syndrome. Blood (2003) 104:2574–81. doi:10.1182/blood-2003-08-2984
147. Markert ML, Alexieff MJ, Li J, Sarzotti M, Ozaki DA, Devlin BH, et al. Complete DiGeorge syndrome: development of rash, lymphadenopathy, and oligoclonal T cells in 5 cases. J Allergy Clin Immunol (2004) 113:734–41. doi:10.1016/j.jaci.2004.01.766
148. Markert ML, Devlin BH, Chinn IK, McCarthy EA, Li YJ. Factors affecting success of thymus transplantation for complete DiGeorge anomaly. Am J Transplant (2008) 8:1729–36. doi:10.1111/j.1600-6143.2008.02301.x
149. Dion ML, Poulin JF, Bordi R, Sylvestre M, Corsini R, Kettaf N, et al. HIV infection rapidly induces and maintains a substantial suppression of thymocyte proliferation. Immunity (2004) 21:757–68. doi:10.1016/j.immuni.2004.10.013
150. Dulude G, Cheynier R, Gauchat D, Abdallah A, Kettaf N, Sžkaly RP, et al. The magnitude of thymic output is genetically determined through controlled intrathymic precursor T cell proliferation. J Immunol (2008) 181:7818–24.
151. Morrhaye G, Kermani H, Legros JJ, Baron F, Beguin Y, Moutschen M, et al. Impact of growth hormone (GH) deficiency and GH replacement upon thymus function in adult patients. PLoS ONE (2009) 4:e5668. doi:10.1371/journal.pone.0005668
152. Clark RA, Yamanaka K, Bai M, Dowgiert R, Kupper TS. Human skin cells support thymus-indipendent T cell development. J Clin Invest (2005) 115:3239–49. doi:10.1172/JCI24731
153. Klein L, Jovanovic K. Regulatory T cell lineage commitment in the thymus. Semin Immunol (2011) 23:401–9. doi:10.1016/j.smim.2011.06.003
154. Pignata C, Fiore M, Guzzetta V, Castaldo A, Sebastio G, Porta F, et al. Congenital alopecia and nail dystrophy associated with severe functional T-cell immunodeficiency in two sibs. Am J Med Genet (1996) 65:167–70. doi:10.1002/(SICI)1096-8628(19961016)65:2<167::AID-AJMG17>3.0.CO;2-O
Keywords: Foxn1 gene, TECs, thymus gland, immunodeficiency, Nude/SCID
Citation: Romano R, Palamaro L, Fusco A, Giardino G, Gallo V, Del Vecchio L and Pignata C (2013) FOXN1: a master regulator gene of thymic epithelial development program. Front. Immunol. 4:187. doi: 10.3389/fimmu.2013.00187
Received: 17 April 2013; Accepted: 25 June 2013;
Published online: 12 July 2013.
Edited by:
Ana E. Sousa, Universidade de Lisboa, PortugalReviewed by:
Graham Anderson, University of Birmingham, UKMaria L. Toribio, Spanish National Research Council, Spain
Copyright: © 2013 Romano, Palamaro, Fusco, Giardino, Gallo, Del Vecchio and Pignata. This is an open-access article distributed under the terms of the Creative Commons Attribution License, which permits use, distribution and reproduction in other forums, provided the original authors and source are credited and subject to any copyright notices concerning any third-party graphics etc.
*Correspondence: Claudio Pignata, Department of Translational Medical Sciences, “Federico II” University, Via S. Pansini 5, Naples 80131, Italy e-mail: pignata@unina.it