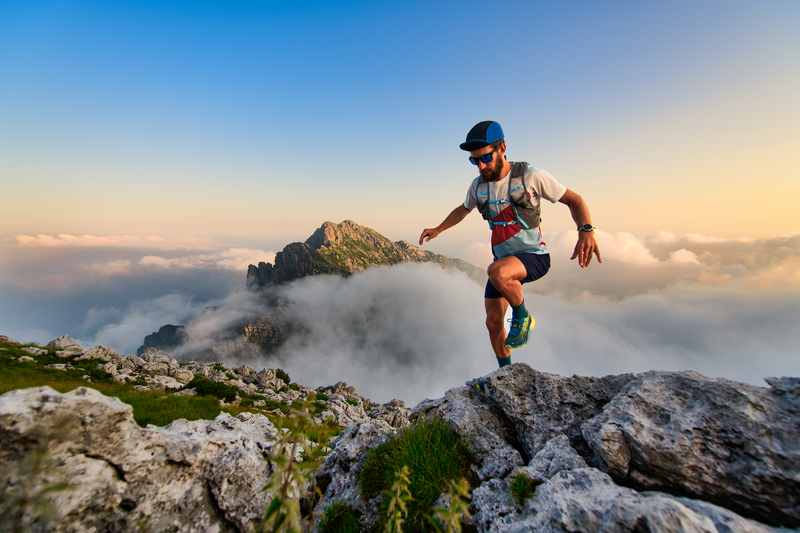
95% of researchers rate our articles as excellent or good
Learn more about the work of our research integrity team to safeguard the quality of each article we publish.
Find out more
MINI REVIEW article
Front. Immunol. , 17 August 2012
Sec. Cytokines and Soluble Mediators in Immunity
Volume 3 - 2012 | https://doi.org/10.3389/fimmu.2012.00266
This article is part of the Research Topic Arrest chemokines View all 10 articles
Leukocyte functions are linked to their migratory responses, which, in turn, are largely determined by the expression profile of classical chemokine receptors. Upon binding their cognate chemokines, these G-protein-coupled receptors (GPCRs) initiate signaling cascades and downstream molecular and cellular responses, including integrin activation and cell locomotion. Chemokines also bind to an alternative subset of chemokine receptors, which have serpentine structure characteristic for GPCRs but lack DRYLAIV consensus motive required for coupling to G-proteins. Duffy antigen receptor for chemokines (DARC) is a member of this atypical receptor subfamily. DARC binds a broad range of inflammatory CXC and CC chemokines and is expressed by erythrocytes, venular endothelial cells, and cerebellar neurons. Erythrocyte DARC serves as blood reservoir of cognate chemokines but also as a chemokine sink, buffering potential surges in plasma chemokine levels. Endothelial cell DARC internalizes chemokines on the basolateral cell surface resulting in subsequent transcytosis of chemokines and their immobilization on the tips of apical microvilli. These DARC-mediated endothelial cell interactions allow chemokines produced in the extravascular tissues to optimally function as arrest chemokines on the luminal endothelial cell surface.
The Duffy antigen receptor for chemokines (DARC) has recently become the focus of studies investigating interactions of inflammatory chemokines with erythrocytes during systemic inflammatory responses as well as with venular endothelial cells during chemokine-induced leukocyte adhesion and emigration. These studies uncovered new functional facets of this rather “old” molecule. DARC was first described in 1950 as the Duffy blood group antigen (Cutbush and Mollison, 1950; Cutbush et al., 1950). An antibody termed anti-Fya present in the plasma of a polytransfused hemophiliac, Mr. Duffy, was found to cause a delayed hemolytic transfusion reaction. In the following year, an antibody to the antithetic antigen, Fyb, was found in a multigravida exposed to fetal Fyb erythrocytes (Ikin et al., 1951). Subsequently, three “Duffy-positive” phenotypes were described: Fy(a+b–), Fy(a–b+), and Fy(a+b+), arising from combinations of the antithetical co-dominant FYA and FYB genes (Klein and Anstee, 2005). However, some individuals, designated “Duffy-negative,” express neither Fya nor Fyb antigens, Fy(a–b–). This phenotype results from a polymorphic form of the FYB gene, FYB(ES) “erythroid silent”, present in up to 99% of West Africans and the majority of African Americans (68%; Mourant et al., 1976). The DARC (Fy) gene is located on chromosome 1, position 1922 and segregates with Un locus, having been the first to be assigned to an autosome in humans (Iwamoto et al., 1996). The two main alleles FYA and FYB differ in a single base substitution (125 G to A) in codon 42 in the NH2 extracellular domain, encoding glycine in Fya and aspartic acid in Fyb (Chaudhuri et al., 1995; Iwamoto et al., 1995). The FYB(ES) allele has a single T to C substitution at nucleotide –67 within the erythroid GATA-1 promoter region, 33 bp upstream from the erythroid transcription starting point and 46 bp upstream from the start of the major transcript translation codon, thus preventing DARC transcription in erythroid cells only (Tournamille et al., 1995).
Hence FYB(ES) Fy(a–b–) individuals still express DARC at non-erythroid sites, e.g., on endothelial cells and possibly other cells (Peiper et al., 1995; Chaudhuri et al., 1997; Horuk et al., 1997). The Duffy-negative phenotype was first linked with resistance to malaria when Fy(a–b–) volunteers exposed to the bites of Plasmodium vivax-infected mosquitoes, in contrast to Duffy-positives, did not develop malaria (Miller et al., 1976). This confirmed the long standing clinical observation that African populations appeared resistant to this form of malaria, noted also during the treatment of neurosyphilis by therapeutic P. vivax inoculation (O’Leary, 1927; Boyd and Stratman-Thomas, 1933). Further work showed that this parasite requires DARC binding for entry into the erythrocytes (Miller et al., 1975; Horuk et al., 1993a), leading to the hypothesis that the Fy(a–b–) phenotype evolved as a result of selective pressure to protect its carriers from vivax but not falciparum malaria. Geostatistical maps show that in West Africa the areas of prevalence of the Fy(a–b–) phenotype of almost 100% (Howes et al., 2011), overlap with areas of expected but absent p. vivax infection (Guerra et al., 2010). However, this resistance is not complete and some Fy(a–b–) populations, for example in Madagascar, both carry parasites asymptomatically and experience symptomatic vivax malaria (Ménard et al., 2010).
Other rare DARC polymorphisms include a C265T mutation in FYB leading to FYX allele and 90% reduction of DARC expression, the so called “Fyb weak” phenotype, and the G298A polymorphism resulting in the Ala100Thr substitution (Olsson et al., 1998; Tournamille et al., 1998).
Human DARC contains 336 amino acids (molecular weight 35733) and was first predicted to have nine trans-membrane domains (Chaudhuri et al., 1993), but later shown to have seven, akin to other chemokine receptors (Neote et al., 1994). The extracellular amino-terminal domain of 65 amino acids harbors three potential N-glycosylation sites at residues 16, 27, and 33 (Czerwinski et al., 2007), and epitopes Fya, Fyb, and Fy6 (Tournamille et al., 2003). The Fy6 epitope is between Q19 and W26 residues, the binding site of the reticulocyte binding protein of P. vivax. Accordingly, monoclonal anti-Fy6 antibody inhibits the invasion of human erythrocytes by P. vivax (Tournamille et al., 2005).
Duffy blood group antigen was designated DARC after it was shown to mediate the promiscuous binding of inflammatory CC and CXC chemokines to erythrocytes (Horuk et al., 1993b; Tournamille et al., 1997; Lee et al., 2003a; Pruenster and Rot, 2006; Ulvmar et al., 2011). DARC’s extracellular N-terminal domain, which bears the blood group determinants, is linked with the fourth extracellular domain via a disulphide bond. These domains together create an active chemokine-binding pocket (Tournamille et al., 1997, 2003). Given the absence of a DRYLAIV motif, which is required G-protein coupling, no detectable chemokine-induced cell signaling has been recorded in either the form of calcium flux (Neote et al., 1994), GTPase activity (Horuk et al., 1993b), or gene transcription (Lee et al., 2003a). Thus, DARC is classified as an atypical chemokine receptor (Nibbs et al., 2003; Pruenster and Rot, 2006; Ulvmar et al., 2011; Graham et al., 2012). However, some intracellular responses take place following DARC ligation by cognate chemokines. It was demonstrated in heterologous transfectants that chemokine binding induces redistribution of DARC from the basolateral cell membrane, via an intracellular vesicular compartment onto the apical membrane and that chemokine cargo is translocated together with DARC (Pruenster et al., 2009). Such chemokine in situ binding mirroring exactly the ligand specificity of DARC (Hub and Rot, 1998) as well as chemokine transcytosis and luminal surface presentation (Middleton et al., 1997) have been shown to place in venular endothelial cells in vivo and ex vivo in intact viable tissues. Unlike other atypical chemokine receptors, D6 in particular, no degradation of chemokines occurs after their internalization by DARC. Accordingly, neutrophil and monocyte migration toward cognate chemokines was enhanced across cellular monolayers expressing DARC (Lee et al., 2003a; Pruenster et al., 2009). Also in vivo, chemokine injections into transgenic mice, which over-express DARC on the endothelium, induced significantly greater leukocyte recruitment (Pruenster et al., 2009). Thus endothelial DARC mediates abluminal internalization and transcellular transport of chemokines. This activity of DARC prevents the escape of soluble tissue-derived chemokine molecules into circulation and allows them to associate with the tips of luminal microvilli and stimulate firm adhesion of leukocytes. Inflammation can further up-regulate DARC expression in postcapillary venules and veins, and induce DARC to appear in vascular segments usually devoid of it (Liu et al., 1999; Segerer et al., 2000; Patterson et al., 2002; Lee et al., 2003b; Bruhl et al., 2005; Gardner et al., 2006; Geleff et al., 2010). It is not clear whether DARC over-expression is the consequence of the development of the inflammatory lesions or their pre-requisite. Primary lymphatic vessels do not express DARC although a small segment, the podoplanin-dull pre-collectors, do express DARC, suggesting that chemokines mediated cell migration may occur at this site (Wick et al., 2008).
Despite the fact that chemokine internalization by DARC does not lead to their degradation, DARC may physically remove chemokines from extracellular environments and thus, e.g., negatively influence angiogenesis induced by extravascular pro-inflammatory chemokines. This was shown in mice over-expressing endothelial DARC, which have reduced angiogenic responses to CXCL2 (Du et al., 2002) and in the context of tumor angiogenesis (Horton et al., 2007). Also, DARC-deficient mice used in a transgenic model of prostate cancer developed tumors with increased vessel density, greater intratumor angiogenic chemokine levels, and augmented growth (Shen et al., 2006). CD82, a tetraspanin which was identified as a prostate cancer metastasis suppressor gene, apparently directly interacts with DARC which thus can inhibit tumor cell proliferation and induce senescence (Bandyopadhyay et al., 2006). It appears therefore that DARC may negatively affect tumor development and metastatic spread either directly by binding to CD82 or by removing angiogenic chemokines from perivascular spaces. Additionally, DARC has been shown to heterodimerize with a classical chemokine receptor CCR5, and through this interaction down-modulate CCR5 mediated signaling responses (Chakera et al., 2008).
Erythrocyte DARC was originally described as a chemokine “sink” (Darbonne et al., 1991) and this function was further supported when DARC was shown to reduce the levels of circulating inflammatory chemokines, thus dampening systemic leukocyte activation (Dawson et al., 2000). Chemokines in circulation can induce the desensitization of their cognate receptors. By protecting circulating leukocytes from chemokine excess, DARC may preserve subsequent leukocyte responsiveness to chemokine signals present on the endothelial surface or in the tissues. Conversely, systemic pre-exposure to chemokines may prime leukocytes for enhanced chemokine-induced migration (Brandt et al., 1998) or other effector functions (Green et al., 1996; Hauser et al., 1999). These two opposing potential outcomes may explain the following apparently conflicting observations in DARC-deficient mice exposed to various inflammatory stimuli (Dawson et al., 2000; Reutershan et al., 2009; Vielhauer et al., 2009; Mei et al., 2010; Zarbock et al., 2010). In an initial study DARC knockout (KO) animals received systemic LPS and responded by a marked increase in neutrophil infiltrate in the lungs and livers as compared to the wild type controls (Dawson et al., 2000). Another study showed that DARC KO mice have significantly less leukocyte infiltrate in the bronchoalveolar lavage in response to chemokine instilled into pulmonary airspace (Lee et al., 2003a). These experiments used DARC KOs lacking this receptor on all cells. Subsequently, bone marrow chimeras were constructed allowing the examination of respective roles of DARC on erythrocytes and endothelium (Lee et al., 2006). Here, mice lacking erythrocyte DARC had significantly fewer airspace neutrophils following intratracheal LPS instillation, suggesting that erythrocyte DARC supports leukocyte emigration. The lack of DARC in the lungs was associated with higher chemokine concentrations in the airspaces compared with mice lacking DARC on erythrocytes. In a model of LPS-inhalation-induced acute lung injury neutrophil migration into the alveolar spaces was increased in DARC KO animals, along with elevated levels of CXC chemokines (Reutershan et al., 2009). In chimeric animals, the absence of erythrocyte DARC was the most significant factor determining leukocyte trafficking. Difference between the outcomes in these two studies may be due to the divergent dose of LPS administered. With higher LPS concentrations the role for erythroid DARC as a sink may become more significant (Reutershan et al., 2009). Of note is that during severe systemic inflammation erythrocyte-bound chemokines amounted to 30% of plasma chemokine concentrations, suggesting only a limited sink effect of erythrocyte DARC during severe inflammation (Reutershan et al., 2009). Conversely, in humans, following endotoxin challenge several hundred fold increases in chemokine levels in erythrocyte lysates were noted (Mayr et al., 2008). Further investigation into the role of DARC in acute lung inflammation revealed that a lack of DARC in mice results in down-regulation of CXCR2 on neutrophils because of high levels of circulating chemokines during severe inflammation (Zarbock et al., 2010). It this study DARC was essential for chemokine-mediated leukocyte recruitment, whereby DARC KO animals were protected from acid-induced lung injury and experienced preserved oxygenation. This occurred as a result of impaired leukocyte arrest on endothelial cells and consequently reduced pulmonary neutrophil recruitment. Adoptive transfer of neutrophils showed that the latter effect is dependent on neutrophils and independent of endothelial cells and erythrocytes, suggesting that the contribution of DARC is in the maintenance of receptor expression in the environments with excess ligands. Because neutrophils, which are activated by chemokines in the systemic circulation (Colditz et al., 2007), may be passively trapped in the lung circulation and contribute to the lung damage (Rot, 1991), inflammatory models in other organs may be more revealing in dissecting local vs. systemic effects of DARC on chemokine-driven leukocyte emigration. Renal models of inflammation have shown that DARC-deficient mice are protected from ischemic and LPS-induced acute renal injury (Zarbock et al., 2007). Furthermore, chemokine presentation on renal endothelial cells was absent, and renal neutrophil recruitment was impaired, in the context of lower inflammatory chemokine levels during systemic inflammation (Zarbock et al., 2007). In contrast, Vielhauer et al. (2009) studied tubule-interstitial inflammation and glomerulonephritis in DARC-deficient mice and demonstrated that in these models macrophage and T lymphocytes were recruited equally well in DARC KO and wild type mice.
Both human and murine studies suggest that DARC can sustain inflammatory chemokines levels on erythrocytes and in plasma (Jilma-Stohlawetz et al., 2001; Fukuma et al., 2003), but the biological purpose of this reservoir function is not clear. Basal plasma CCL2 levels are one-third lower in DARC KO mice than in control wild type animals (Fukuma et al., 2003). When CCL11 or hCXCL1 were administered intravenously, these chemokines disappeared more rapidly from the plasma of DARC KOs (Fukuma et al., 2003). Duffy-negative humans were noted to have significantly lower basal CCL2 levels than Duffy-positives (Jilma-Stohlawetz et al., 2001) and following endotoxin administration, higher levels of plasma CCL2 were observed in Duffy-positive individuals as compared to the Duffy-negative ones (Mayr et al., 2008). Also CCL2 and CXCL1 levels, but not CXCL8 or CCL4 levels were higher in erythrocyte lysates of Duffy-positive individuals at baseline, whereas following endotoxin administration CCL2 and CXCL8, but not CCL4, levels increased significantly in erythrocyte lysates of Duffy-positive subjects. Given that chemokine plasma levels, including of CXCL8 (Wong et al., 2008) and CCL2 (Bozza et al., 2007) have been shown to be predictive of survival and correlate with sepsis severity, it is tempting to speculate that the loss of DARC expression may affect the outcome in sepsis. It has been recently suggested that chemokines with different binding affinities for DARC can modify the levels of other erythrocyte-bound and free plasma chemokines, affecting resultant leukocyte responses (Mei et al., 2010). In addition, heparin and activated coagulation factors can elute chemokines off erythrocyte DARC (Schnabel et al., 2010). Thus chemokines with range of affinities for DARC and other factors may significantly interfere with the ability of DARC to bind any particular chemokine introducing further complexity into mechanistic understanding of erythrocyte DARC function.
Recently, differences in plasma and serum chemokine levels were reported in persons with DARC Fya and Fyb (Schnabel et al., 2010), although the mechanism for this is not apparent. Further work revealed that Fyb erythrocytes have reduced surface DARC expression as compared to Fya erythrocytes; however, the binding affinity of DARC for chemokines was not appreciably different between these two phenotypes (Xiong et al., 2011). As discussed above, endothelial cells of post-capillary and collective venules and small veins express DARC, which functions here as a transcytosis receptor transporting chemokines from the basolateral to the apical side (Pruenster et al., 2009) where they are immobilized on the luminal surface. It is attractive to speculate that that individuals of alternative Fya vs. Fyb DARC phenotypes may also show differences in chemokine-binding specificity and patterning by the endothelium, though to date there is no data to support this notion.
Since the discovery of its chemokine-binding properties, DARC has been mainly considered as a “decoy” receptor scavenging its ligands. Recent research shed new light on much more multifaceted activities of DARC. On erythrocytes, DARC acts on the one hand as a blood chemokine sink and, on the other, as a reservoir of cognate chemokines buffering the bursts in their blood levels, and maintaining these, respectively. Both of these functions are absent in individuals with FYB(ES) DARC “negative” polymorphism. Future work should uncover molecular and cellular mechanisms explaining how the lack of erythrocyte chemokine sink and depot functions in these DARC-negative individuals affects pathomechanisms in various inflammatory diseases and cancer. In endothelial cells DARC functions as a transcytosis receptor leading to correct patterning of chemokines on the tissue–blood interface in venules and veins, thus supporting optimal chemokine-induced leukocyte endothelial cell adhesion and subsequent leukocyte emigration.
The authors declare that the research was conducted in the absence of any commercial or financial relationships that could be construed as a potential conflict of interest.
Supported by the MRC grants G0802838 and G9818340, and Wellcome Trust grant WT090962MA.
Bandyopadhyay, S., Zhan, R., Chaudhuri, A., Watabe, M., Pai, S. K., Hirota, S., Hosobe, S., Tsukada, T., Miura, K., Takano, Y., Saito, K., Pauza, M. E., Hayashi, S., Wang, Y., Mohinta, S., Mashimo, T., Iiizumi, M., Furuta, E., and Watabe, K. (2006). Interaction of KAI1 on tumor cells with DARC on vascular endothelium leads to metastasis suppression. Nat. Med. 12, 933–938.
Boyd, M. F., and Stratman-Thomas, W. K. (1933). Studies on benign tertian malaria. 4. On the refractoriness of negroes to inoculation with Plasmodium vivax. Am. J. Hyg. 18, 485–489.
Bozza, F., Salluh, J. I., Japiassu, A. M., Soares, M., Assis, E. F., Gomes, R. N., Bozza, M. T., Castro-Faria-Neto, H. C., and Bozza, P. T. (2007). Cytokine profiles as markers of disease severity in sepsis: a multiplex analysis. Crit. Care 11, R49.
Brandt, E., Muller-Alouf, H., Desreumaux, P., Woerly, G., Colombel, J. F., and Capron, M. (1998). Circulating growth-regulator oncogene alpha contributes to neutrophil priming and interleukin-8-directed mucosal recruitment into chronic lesions of patients with Crohn’s disease. Eur. Cytokine Netw. 9, 647–653.
Bruhl, H., Vielhauer, V., Weiss, M., Mack, M., Schlondorff, D., and Segerer, S. (2005). Expression of DARC, CXCR3 and CCR5 in giant cell arteritis. Rheumatology (Oxford) 44, 309–313.
Chakera, A., Seeber, R. M., John, A. E., Eidne, K. A., and Greaves, D. R. (2008). The duffy antigen/receptor for chemokines exists in an oligomeric form in living cells and functionally antagonizes CCR5 signaling through hetero-oligomerization. Mol. Pharmacol. 73, 1362–1370.
Chaudhuri, A., Nielsen, S., Elkjaer, M. L., Zbrzezna, V., Fang, F., and Pogo, A. O. (1997). Detection of Duffy antigen in the plasma membranes and caveolae of vascular endothelial and epithelial cells of nonerythroid organs. Blood 89, 701–712.
Chaudhuri, A., Polyakova, J., Zbrzezna, V., and Pogo, A. O. (1995). The coding sequence of the Duffy blood group gene in humans and simians: restriction fragment length polymorphism, antibody and malarial parasite specificities, and expression in nonerythroid tissues in Duffy-negative individuals. Blood 85, 615–621.
Chaudhuri, A., Polyakova, J., Zbrzezna, V., Williams, K., Gulati, S., and Pogo, A. O. (1993). Cloning of glycoprotein D cDNA, which encodes the major subunit of the Duffy blood group system and the receptor for the Plasmodium vivax malaria parasite. Proc. Natl. Acad. Sci. U.S.A. 90, 10793–10797.
Colditz, I. G., Schneider, M. A., Pruenster, M., and Rot, A. (2007). Chemokines at large: in-vivo mechanisms of their transport, presentation and clearance. Thromb. Haemost. 97, 688–693.
Cutbush, M., Mollison, P. L., and Parkin, D. M. (1950). A new human blood group. Nature 165, 188–190.
Czerwinski, M., Kern, J., Grodecka, M., Paprocka, M., Krop-Watorek, A., and Wasniowska, K. (2007). Mutational analysis of the N-glycosylation sites of Duffy antigen/receptor for chemokines. Biochem. Biophys. Res. Commun. 356, 816–821.
Darbonne, W. C., Rice, G. C., Mohler, M. A., Apple, T., Hebert, C. A., Valente, A. J., and Baker, J. B. (1991). Red blood cells are a sink for interleukin 8, a leukocyte chemotaxin. J. Clin. Invest. 88, 1362–1369.
Dawson, T. C., Lentsch, A. B., Wang, Z., Cowhig, J. E., Rot, A., Maeda, N., and Peiper, S. C. (2000). Exaggerated response to endotoxin in mice lacking the Duffy antigen/receptor for chemokines (DARC). Blood 96, 1681–1684.
Du, J., Luan, J., Liu, H., Daniel, T. O., Peiper, S., Chen, T. S., Yu, Y., Horton, L. W., Nanney, L. B., Strieter, R. M., and Richmond, A. (2002). Potential role for Duffy antigen chemokine-binding protein in angiogenesis and maintenance of homeostasis in response to stress. J. Leukoc. Biol. 71, 141–153.
Fukuma, N., Akimitsu, N., Hamamoto, H., Kusuhara, H., Sugiyama, Y., and Sekimizu, K. (2003). A role of the Duffy antigen for the maintenance of plasma chemokine concentrations. Biochem. Biophys. Res. Commun. 303, 137–139.
Gardner, L., Wilson, C., Patterson, A. M., Bresnihan, B., FitzGerald, O., Stone, M. A., Ashton, B. A., and Middleton, J. (2006). Temporal expression pattern of Duffy antigen in rheumatoid arthritis: up-regulation in early disease. Arthritis Rheum. 54, 2022–2026.
Geleff, S., Draganovici, D., Jaksch, P., and Segerer, S. (2010). The role of chemokine receptors in acute lung allograft rejection. Eur. Respir. J. 35, 167–175.
Graham, G. J., Locati, M., Mantovani, A., Rot, A., and Thelen, M. (2012). The biochemistry and biology of the atypical chemokine receptors. Immunol. Lett. 145, 30–38.
Green, S. P., Chuntharapai, A., and Curnutte, J. T. (1996). Interleukin-8 (IL-8), melanoma growth-stimulatory activity, and neutrophil-activating peptide selectively mediate priming of the neutrophil NADPH oxidase through the type A or type B IL-8 receptor. J. Biol. Chem. 271, 25400–25405.
Guerra, C. A., Howes, R. E., Patil, A. P., Gething, P. W., Van Boeckel, T. P., Temperley, W. H., Kabaria, C. W., Tatem, A. J., Manh, B. H., Elyazar, I. R., Baird, J. K., Snow, R. W., and Hay, S. I. (2010). The international limits and population at risk of Plasmodium vivax transmission in 2009. PLoS Negl. Trop. Dis. 4, e774. doi: 10.1371/journal.pntd.0000774
Hauser, C. J., Fekete, Z., Goodman, E. R., Kleinstein, E., Livingston, D. H., and Deitch, E. A. (1999). CXCR2 stimulation primes CXCR1 [Ca2+]i responses to IL-8 in human neutrophils. Shock 12, 428–437.
Horton, L. W., Yu, Y., Zaja-Milatovic, S., Strieter, R. M., and Richmond, A. (2007). Opposing roles of murine duffy antigen receptor for chemokine and murine CXC chemokine receptor-2 receptors in murine melanoma tumor growth. Cancer Res. 67, 9791–9799.
Horuk, R., Chitnis, C. E., Darbonne, W. C., Colby, T. J., Rybicki, A., Hadley, T. J., and Miller, L. H. (1993a). A receptor for the malarial parasite Plasmodium vivax: the erythrocyte chemokine receptor. Science 261, 1182–1184.
Horuk, R., Colby, T. J., Darbonne, W. C., Schall, T. J., and Neote, K. (1993b). The human erythrocyte inflammatory peptide (chemokine) receptor. Biochemical characterization, solubilization, and development of a binding assay for the soluble receptor. Biochemistry 32, 5733–5738.
Horuk, R., Martin, A. W., Wang, Z., Schweitzer, L., Gerassimides, A., Guo, H., Lu, Z., Hesselgesser, J., Perez, H. D., Kim, J., Parker, J., Hadley, T. J., and Peiper, S. C. (1997). Expression of chemokine receptors by subsets of neurons in the central nervous system. J. Immunol. 158, 2882–2890.
Howes, R. E., Patil, A. P., Piel, F. B., Nyangiri, O. A., Kabaria, C. W., Gething, P. W., Zimmerman, P. A., Barnadas, C., Beall, C. M., Gebremedhin, A., Ménard, D., Williams, T. N., Weatherall, D. J., and Hay, S. I. (2011). The global distribution of the Duffy blood group. Nat. Commun. 266, 1–10.
Hub, E., and Rot, A. (1998). Binding of RANTES, MCP-1, MCP-3, and MIP-1 alpha to cells in human skin. Am. J. Pathol. 152, 749–757.
Ikin, E. W., Mourant, A. E., Pettenkofer, H. J., and Blumenthal, G. (1951). Discovery of the expected haemagglutinin, anti-Fyb. Nature 168, 1077–1078.
Iwamoto, S., Li, J., Omi, T., Ikemoto, S., and Kajii, E. (1996). Identification of a novel exon and spliced form of Duffy mRNA that is the predominant transcript in both erythroid and postcapillary venule endothelium. Blood 87, 378–385.
Iwamoto, S., Omi, T., Kajii, E., and Ikemoto, S. (1995). Genomic organization of the glycoprotein D gene: Duffy blood group Fya/Fyb alloantigen system is associated with a polymorphism at the 44-amino acid residue. Blood 85, 622–626.
Jilma-Stohlawetz, P., Homoncik, M., Drucker, C., Marsik, C., Rot, A., Mayr, W. R., Seibold, B., and Jilma, B. (2001). Fy phenotype and gender determine plasma levels of monocyte chemotactic protein. Transfusion 41, 378–381.
Klein, H. G., and Anstee, D. J. (2005). Mollison’s Blood Transfusion in Clinical Medicine, 11th Edn. Malden, MA: Blackwell Publishing.
Lee, J. S., Frevert, C. W., Thorning, D. R., Segerer, S., Alpers, C. E., Cartron, J. P., Colin, Y., Wong, V. A., Martin, T. R., and Goodman, R. B. (2003b). Enhanced expression of Duffy antigen in the lungs during suppurative pneumonia. J. Histochem. Cytochem. 51, 159–166.
Lee, J. S., Frevert, C. W., Wurfel, M. M., Peiper, S. C., Wong, V. A., Ballman, K. K., Ruzinski, J. T., Rhim, J. S., Martin, T. R., and Goodman, R. B. (2003a). Duffy antigen facilitates movement of chemokine across the endothelium in vitro and promotes neutrophil transmigration in vitro and in vivo. J. Immunol. 170, 5244–5251.
Lee, J. S., Wurfel, M. M., Matute-Bello, G., Frevert, C. W., Rosengart, M. R., Ranganathan, M., Wong, V. W., Holden, T., Sutlief, S., Richmond, A., Peiper, S., and Martin, T. R. (2006). The Duffy antigen modifies systemic and local tissue chemokine responses following lipopolysaccharide stimulation. J. Immunol. 177, 8086–8094.
Liu, X. H., Hadley, T. J., Xu, L., Peiper, S. C., and Ray, P. E. (1999). Up-regulation of Duffy antigen receptor expression in children with renal disease. Kidney Int. 55, 1491–1500.
Mayr, F. B., Spiel, A. O., Leitner, J. M., Firbas, C., Kliegel, T., Jilma-Stohlawetz, P., Derendorf, H., and Jilma, B. (2008). Duffy antigen modifies the chemokine response in human endotoxemia. Crit. Care Med. 36, 159–165.
Mei, J., Liu, Y., Dai, N., Favara, M., Greene, T., Jeyaseelan, S., Poncz, M., Lee, J. S., and Worthen, G. S. (2010). CXCL5 regulates chemokine scavenging and pulmonary host defense to bacterial infection. Immunity 33, 106–117.
Ménard, D., Barnadas, C., Bouchier, C., Henry-Halldin, C., Gray, L. R., Ratsimbasoa, A., Thonier, V., Carod, J. F., Domarle, O., Colin, Y., Bertrand, O., Picot, J., King, C. L., Grimberg, B. T., Mercereau-Puijalon, O., and Zimmerman, P. A. (2010). Plasmodium vivax clinical malaria is commonly observed in Duffy-negative Malagasy people. Proc. Natl. Acad. Sci. U.S.A. 107, 5967–5971.
Middleton, J., Neil, S., Wintle, J., Clark-Lewis, I., Moore, H., Lam, C., Auer, M., Hub, E., and Rot, A. (1997). Transcytosis and surface presentation of IL-8 by venular endothelial cells. Cell 91, 385–395.
Miller, L. H., Mason, S. J., Clyde, D. F., and McGinniss, M. H. (1976). The resistance factor to Plasmodium vivax in blacks. The Duffy-blood-group genotype, FyFy. N. Engl. J. Med. 295, 302–304.
Miller, L. H., Mason, S. J., Dvorak, J. A., McGinniss, M. H., and Rothman, I. K. (1975). Erythrocyte receptors for (Plasmodium knowlesi) malaria: Duffy blood group determinants. Science 189, 561–563.
Mourant, A. E., Kopec, A. C., and Domaniewska-Sobczak, K. (1976). The Distribution of the Human Blood Groups, and Other Polymorphisms, 2nd Edn. London: Oxford University Press.
Neote, K., Mak, J., Kolakowski, L. J., and Schall, T. (1994). Functional and biochemical analysis of the cloned Duffy antigen: identity with the red blood cell chemokine receptor. Blood 84, 44–52.
Nibbs, R., Graham, G., and Rot, A. (2003). Chemokines on the move: control by the chemokine “interceptors” Duffy blood group antigen and D6. Semin. Immunol. 15, 287–294.
O’Leary, P. A. (1927). Treatment of neurosyphilis by malaria: report on the three years’ observation of the first one hundred patients treated. J. Am. Med. Assoc. 89, 95–100.
Olsson, M. L., Smythe, J. S., Hansson, C., Poole, J., Mallinson, G., Jones, J., Avent, N. D., and Daniels, G. (1998). The Fy(x) phenotype is associated with a missense mutation in the Fy(b) allele predicting Arg89Cys in the Duffy glycoprotein. Br. J. Haematol. 103, 1184–1191.
Patterson, A. M., Siddall, H., Chamberlain, G., Gardner, L., and Middleton, J. (2002). Expression of the duffy antigen/receptor for chemokines (DARC) by the inflamed synovial endothelium. J. Pathol. 197, 108–116.
Peiper, S. C., Wang, Z. X., Neote, K., Martin, A. W., Showell, H. J., Conklyn, M. J., Ogborne, K., Hadley, T. J., Lu, Z. H., Hesselgesser, J., and Horuk, R. (1995). The Duffy antigen/receptor for chemokines (DARC) is expressed in endothelial cells of Duffy negative individuals who lack the erythrocyte receptor. J. Exp. Med. 181, 1311–1317.
Pruenster, M., Mudde, L., Bombosi, P., Dimitrova, S., Zsak, M., Middleton, J., Richmond, A., Graham, G. J., Segerer, S., Nibbs, R. J., and Rot, A. (2009). The Duffy antigen receptor for chemokines transports chemokines and supports their promigratory activity. Nat. Immunol. 10, 101–108.
Pruenster, M., and Rot, A. (2006). Throwing light on DARC. Biochem. Soc. Trans. 34(Pt 6), 1005–1008.
Reutershan, J., Harry, B., Chang, D., Bagby, G. J., and Ley, K. (2009). DARC on RBC limits lung injury by balancing compartmental distribution of CXC chemokines. Eur. J. Immunol. 39, 1597–1607.
Rot, A. (1991). Some aspects of NAP-1 pathophysiology: lung damage caused by a blood-borne cytokine. Adv. Exp. Med. Biol. 305, 127–135.
Schnabel, R., Baumert, J., Barbalic, M., Dupuis, J., Ellinor, P. T., Durda, P., Dehghan, A., Bis, J. C., Illig, T., Morrison, A. C., Jenny, N. S., Keaney, J. F. Jr., Gieger, C., Tilley, C., Yamamoto, J. F., Khuseyinova, N., Heiss, G., Doyle, M., Blankenberg, S., Herder, C., Walston, J. D., Zhu, Y., Vasan, R. S., Klopp, N., Boerwinkle, E., Larson, M. G., Psaty, B. M., Peters, A., Ballantyne, C. M., Witteman, J. C., Hoogeveen, R. C., Benjamin, E. J., Koenig, W., and Tracy, R. P. (2010). Duffy antigen receptor for chemokines (Darc) polymorphism regulates circulating concentrations of monocyte chemoattractant protein-1 and other inflammatory mediators. Blood 115, 5289–5299.
Segerer, S., Regele, H., Mack, M., Kain, R., Cartron, J. P., Colin, Y., Kerjaschki, D., and Schlöndorff, D. (2000). The Duffy antigen receptor for chemokines is up-regulated during acute renal transplant rejection and crescentic glomerulonephritis. Kidney Int. 58, 1546–1556.
Shen, H., Schuster, R., Stringer, K. F., Waltz, S. E., and Lentsch, A. B. (2006). The Duffy antigen/receptor for chemokines (DARC) regulates prostate tumor growth. FASEB J. 20, 59–64.
Tournamille, C., Colin, Y., Cartron, J. P., and Le Van Kim, C. (1995). Disruption of a GATA motif in the Duffy gene promoter abolishes erythroid gene expression in Duffy-negative individuals. Nat. Genet. 10, 224–228.
Tournamille, C., Filipe, A., Badaut, C., Riottot, M. M., Longacre, S., Cartron, J. P., Le Van Kim, C., and Colin, Y. (2005). Fine mapping of the Duffy antigen binding site for the Plasmodium vivax Duffy-binding protein. Mol. Biochem. Parasitol. 144, 100–103.
Tournamille, C., Filipe, A., Wasniowska, K., Gane, P., Lisowska, E., Cartron, J. P., Colin, Y., and Le Van Kim, C. (2003). Structure–function analysis of the extracellular domains of the Duffy antigen/receptor for chemokines: characterization of antibody and chemokine binding sites. Br. J. Haematol. 122, 1014–1023.
Tournamille, C., Le Van Kim, C., Gane, P., Blanchard, D., Proudfoot, A. E., Cartron, J. P., and Colin, Y. (1997). Close association of the first and fourth extracellular domains of the duffy antigen/receptor for chemokines by a disulfide bond is required for ligand binding. J. Biol. Chem. 272, 16274–16280.
Tournamille, C., Le Van Kim, C., Gane, P., Le Pennec, P. Y., Roubinet, F., Babinet, J., Cartron, J. P., and Colin, Y. (1998). Arg89Cys substitution results in very low membrane expression of the Duffy antigen/receptor for chemokines in Fy(x) individuals. Blood 92, 2147–2156.
Ulvmar, M. H., Hub, E., and Rot, A. (2011). Atypical chemokine receptors. Exp. Cell Res. 317, 556–568.
Vielhauer, V., Allam, R., Lindenmeyer, M. T., Cohen, C. D., Draganovici, D., Mandelbaum, J., Eltrich, N., Nelson, P. J., Anders, H. J., Pruenster, M., Rot, A., Schlöndorff, D., and Segerer, S. (2009). Efficient renal recruitment of macrophages and T cells in mice lacking the duffy antigen/receptor for chemokines. Am. J. Pathol. 175, 119–131.
Wick, N., Haluza, D., Gurnhofer, E., Raab, I., Kasimir, M. T., Prinz, M., Steiner, C. W., Reinisch, C., Howorka, A., Giovanoli, P., Buchsbaum, S., Krieger, S., Tschachler, E., Petzelbauer, P., and Kerjaschki, D. (2008). Lymphatic precollectors contain a novel, specialized subpopulation of podoplanin low, CCL27-expressing lymphatic endothelial cells. Am. J. Pathol. 173, 1202–1209.
Wong, H. R., Cvijanovich, N., Wheeler, D. S., Bigham, M. T., Monaco, M., Odoms, K., Macias, W. L., and Williams, M. D. (2008). Interleukin-8 as a stratification tool for interventional trials involving pediatric septic shock. Am. J. Respir. Crit. Care Med. 178, 276–282.
Xiong, Z., Cavaretta, J., Qu, L., Stolz, D. B., Triulzi, D., and Lee, J. (2011). Red blood cell microparticles show altered inflammatory chemokine binding and release ligand upon interaction with platelets. Transfusion 51, 610–621.
Zarbock, A., Bishop, J., Müller, H., Schmolke, M., Buschmann, K., Van Aken, H., and Singbartl, K. (2010). Chemokine homeostasis vs. chemokine presentation during severe acute lung injury: the other side of the Duffy antigen receptor for chemokines. Am. J. Physiol. Lung Cell. Mol. Physiol. 298, L462–L471.
Keywords: atypical chemokine receptors, chemokines, DARC, duffy antigen, endothelial cells, erythrocytes, inflammation, transcytosis
Citation: Novitzky-Basso I and Rot A (2012) Duffy antigen receptor for chemokines and its involvement in patterning and control of inflammatory chemokines. Front. Immun. 3:266. doi: 10.3389/fimmu.2012.00266
Received: 09 May 2012; Paper pending published: 05 June 2012;
Accepted: 02 August 2012; Published online: 17 August 2012.
Edited by:
Klaus Ley, La Jolla Institute for Allergy and Immunology, USAReviewed by:
Cory Michel Hogaboam, University of Michigan Medical School, USACopyright: © 2012 Novitzky-Basso and Rot. This is an open-access article distributed under the terms of the Creative Commons Attribution License, which permits use, distribution and reproduction in other forums, provided the original authors and source are credited and subject to any copyright notices concerning any third-party graphics etc.
*Correspondence: Antal Rot, MRC Centre for Immune Regulation, Institute of Biomedical Research, School of Infection and Immunity, University of Birmingham, Vincent Drive, Edgbaston, Birmingham B15 2TT, UK. e-mail:YS5yb3RAYmhhbS5hYy51aw==
Disclaimer: All claims expressed in this article are solely those of the authors and do not necessarily represent those of their affiliated organizations, or those of the publisher, the editors and the reviewers. Any product that may be evaluated in this article or claim that may be made by its manufacturer is not guaranteed or endorsed by the publisher.
Research integrity at Frontiers
Learn more about the work of our research integrity team to safeguard the quality of each article we publish.