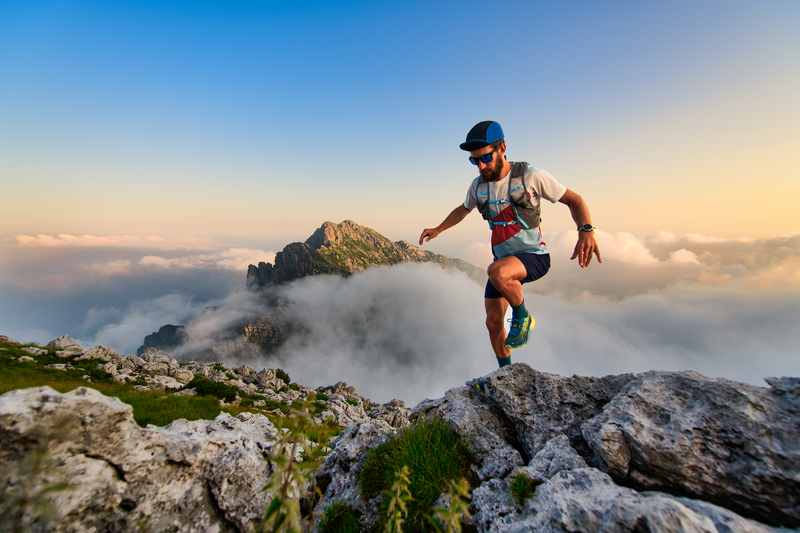
95% of researchers rate our articles as excellent or good
Learn more about the work of our research integrity team to safeguard the quality of each article we publish.
Find out more
REVIEW article
Front. Immunol. , 02 July 2012
Sec. Alloimmunity and Transplantation
Volume 3 - 2012 | https://doi.org/10.3389/fimmu.2012.00182
This article is part of the Research Topic Mesenchymal stem cells in Transplantation and Tissue Regeneration View all 15 articles
Mesenchymal stem cells (MSCs) have differentiation and immunomodulatory properties that make them interesting tools for the treatment of degenerative disorders, allograft rejection, or inflammatory and autoimmune diseases. Biological properties of MSCs can be modulated by the inflammatory microenvironment they face at the sites of injury or inflammation. Indeed, MSCs do not constitutively exert their immunomodulating properties but have to be primed by inflammatory mediators released from immune cells and inflamed tissue. A polarization process, mediated by Toll-like receptors (TLRs), toward either an anti-inflammatory or a pro-inflammatory phenotype has been described for MSCs. TLRs have been linked to allograft rejection and the perpetuation of chronic inflammatory diseases (e.g., Crohn’s disease, rheumatoid arthritis) through the recognition of conserved pathogen-derived components or endogenous ligands (danger signals) produced upon injury. Interest in understanding the effects of TLR activation on MSCs has greatly increased in the last few years since MSCs will likely encounter TLR ligands at sites of injury, and it has been proven that the activation of TLRs in MSCs can modulate their function and therapeutic effect.
Mesenchymal stem cells (MSCs) have emerged in recent years as therapeutic tools based on three important features: (i) differentiation potential, (ii) capacity to modulate immune responses, and (iii) low immunogenicity, which would may allow allogeneic treatments.
Mesenchymal stem cells have been isolated from multiple tissues of mesodermal origin, such as bone marrow (Friedenstein et al., 1976), adipose tissue (Zuk et al., 2002), umbilical cord blood (Romanov et al., 2003), placenta (Fukuchi et al., 2004), synovium (De Bari et al., 2001), or dental pulp (Gronthos et al., 2000), among others. Despite significant efforts, no exclusive surface markers have been identified for MSCs. To date, MSCs are defined according to the three criteria of the International Society for Cellular Therapy (Dominici et al., 2006): (a) Adhesion to plastic: MSCs can be isolated by adhesion to plastic and expanded in vitro in serum containing media with no additional requirements for growth factors or cytokines; (b) Expression of a specific combination of surface markers: MSCs are negative for CD45, CD34, CD14, or CD11b, CD79α, or CD19 and HLA-DR, and positive for a variety of other markers, including CD73, CD90, and CD105; (c) Differentiation potential: MSCs can be identified in vitro by their ability to differentiate into mesenchymal-type cells (trilineage differentiation into adipocytes, osteoblasts, and chondrocytes; Pittenger et al., 1999). Although sharing these main characteristics, differences between MSCs from different sources can be found. The secretome differs between cell types, and bone marrow-derived MSCs (BM-MSCs) and adipose-derived MSCs (AD-MSCs), for instance, show specific RNA and protein expression profiles (De Ugarte et al., 2003; Noël et al., 2008; Skalnikova et al., 2011).
In homeostatic conditions, allogeneic cells are rejected by the immune system upon recognition of their foreign human leukocyte antigen (HLA). Allogeneic cells can also activate T cells through an indirect pathway where their HLA antigens are presented by professional antigen-presenting cells (APC). MSCs express low levels of cell surface HLA class I molecules whereas HLA class II, CD40, CD80, and CD86 are not detectable on the cell surface which theoretically opens the possibility of allogeneic treatments without the requirement of suppression of host immunity. Stimulation with interferon (IFN)γ has been shown to increase both class I and class II molecules. However, MSCs do not express classic co-stimulatory molecules such as CD40, CD80, CD86, even after stimulation in an inflammatory milieu. These features may allow MSCs to avoid or delay immune recognition (Le Blanc et al., 2003a,b; Majumdar et al., 2003; Rasmusson et al., 2003; McIntosh et al., 2006; Chamberlain et al., 2007), although this is a question that needs to be further investigated in both experimental animal models and clinical trials (Griffin et al., 2010).
Mesenchymal stem cells have immunomodulating properties and inhibit function of immune cells (Bartholomew et al., 2002; Krampera et al., 2003; Zhang et al., 2004; Beyth et al., 2005; Glennie et al., 2005; Puissant et al., 2005; Nauta et al., 2006; Yañez et al., 2006; Cui et al., 2007; Chiesa et al., 2011; DelaRosa et al., 2012). The specific molecular and cellular mechanisms involved in the immunoregulatory activity of MSCs are still under investigation and remain poorly understood. There is evidence that the capability to modulate immune responses rely on both cell contact-dependent mechanisms (i.e., through Jagged1–Notch1 interactions; Liotta et al., 2008) and paracrine effects through the release of soluble factors (reviewed by Doorn et al., 2012). A broad panel of soluble factors have been involved including hepatocyte growth factor (HGF), prostanglandin-E2 (PGE2), transforming growth factor (TGF)-β1, indoleamine 2,3-dioxygenase (IDO), nitric oxide (NO), interleukin (IL)-10, heme oxygenase-1 (HO-1), and HLA-G5 (Krampera et al., 2003; Beyth et al., 2005; Puissant et al., 2005; Yañez et al., 2006; Chabannes et al., 2007; Cui et al., 2007; Oh et al., 2007; Selmani et al., 2008; DelaRosa et al., 2009). Differences in the mechanisms of immunomodulation employed by MSCs from different species have been reported. Whereas IDO activity appears to be a key player in human MSC-mediated immunomodulation, mouse MSCs do not express IDO and seem to use NO as the main mediator (DelaRosa et al., 2009; Ren et al., 2009; Meisel et al., 2011). Interestingly, MSCs may also modulate immune responses through the generation of regulatory T cells (Tregs; Krampera et al., 2003; Zhang et al., 2004; Maccario et al., 2005; Nauta et al., 2006; Gonzalez-Rey et al., 2010). Whether this MSC-mediated Treg induction is due to an expansion of pre-existing Tregs, to a de novo induction or to a combination of both needs to be further explored.
Importantly, MSCs do not constitutively exert their immunomodulating properties but have to be “primed” by inflammatory mediators released from activated immune cells, such as IFNγ, IL1β, and TNFα (Krampera et al., 2006; Prasanna et al., 2010). Also, the functionality of MSCs can be modulated by other inflammatory mediators such as APRIL and BAFF (Zonca et al., 2012). The thinking that MSCs are only anti-proliferative and immune-inhibitory on immune cells has been recently challenged by Waterman et al. (2010) who reported a “licensing” process of MSCs toward either anti-inflammatory or pro-inflammatory phenotypes, depending on the toll-like receptor (TLR) ligand used for activation. For extensive review on the concept of MSC “licensing” see the excellent review by Krampera (2011).
The biological characteristics mentioned above make MSCs an interesting tool for cellular therapy. This is supported by a number of studies in experimental models of inflammatory diseases demonstrating an efficient protection against allograft rejection, graft-versus-host disease, experimental autoimmune encephalomyelitis, collagen-induced arthritis, sepsis, and autoimmune myocarditis (Le Blanc et al., 2004; Zappia et al., 2005; Ohnishi et al., 2007; González et al., 2009a,b; Gonzalez-Rey et al., 2009; Németh et al., 2009). As indicated previously, TLRs have been implicated in the pathology of graft transplantation and inflammatory diseases (Ishihara et al., 2006; Yamamoto-Furusho and Podolsky, 2007) and therefore may modulate MSC function in vivo (DelaRosa and Lombardo, 2010; Krampera, 2011).
Innate immunity relies on the existence of a mechanism of recognition that identifies conserved molecular structures, known as pathogen associated molecular patterns (PAMPs), broadly expressed by different groups of microorganisms. These PAMPs include lipids, lipoproteins, carbohydrates, and nucleic acids (Akira et al., 2006). The recognition of these PAMPs is mediated by a set of germ line-encoded receptors known as pattern recognition receptors (PRRs). This recognition enables eukaryotic hosts to reliably detect a microbial infection, activating a number of signaling pathways that culminate in the induction of pro-inflammatory cytokines, chemokines, and inflammatory mediators. PRRs include TLRs, Retinoic acid-inducible gene I (RIG-I)-like receptors (RLRs) and NOD-like receptors (NLRs). PRRs, through their modulation of innate and adaptive immune responses, are essential players in the battle for tolerance or rejection of transplanted organs (Methe et al., 2004; Penack et al., 2010). The molecular and cellular mechanisms involved remain poorly understood and represent an emerging field of research with potential therapeutic implications.
Toll-like receptors are type I membrane proteins expressed by immune and non-immune cells (i.e., monocytes, macrophages, endothelial cells) either in the plasma membrane or intracellularly (endosomes). To date, 11 human and 13 mouse TLRs have been identified that recognize distinct microbial products from bacteria, viruses, protozoa, and fungi (Moresco et al., 2011). In addition, the recognition of endogenous ligands by TLRs is thought to have an important role in the regulation of inflammation, both in infectious and non-infectious diseases. A number of endogenous ligands have been identified, including heat shock protein (HSP) 60, HSP 70 (Asea et al., 2000; Oashi et al., 2000), heparan sulfate (Johnson et al., 2002), hyaluronan (Termeer et al., 2002), fibronectin extra domain A (Okamura et al., 2001), uric acid (Liu-Bryan et al., 2005), oxidized LDL (Miller et al., 2003), intracellular components of fragmented cells (Boule et al., 2004; Barrat et al., 2005), myeloid-related proteins-8 and 14 (Vogl et al., 2007), eosinophil-derived neurotoxin (Yang et al., 2008), and human defensin-3 (Funderburg et al., 2007). As these ligands are accessible to TLRs in the setting of injury or non-infectious threat, they have been called “danger signals.”
Toll-like receptor activation triggers intracellular signaling pathways that lead to the induction of inflammatory cytokines, type I IFNs, and upregulation of co-stimulatory molecules leading to the activation of the adaptive immune response. Ligand recognition results in the recruitment of intracellular adaptor proteins, including myeloid-differentiation primary-response protein 88 (MyD88), shared by all TLRs except TLR3, and Toll/IL-1R domain-containing adaptor-inducing IFNβ (Trif), employed by TLR3 and TLR4 (O’Neill and Bowie, 2007). Recruitment of MyD88 leads to the activation of the mitogen-activated protein (MAP)-kinases (MAPKs) and nuclear translocation of the transcription factor nuclear factor-κB (NF-κB; MyD88-dependent pathway; Hoebe et al., 2006; Meylan et al., 2006). The activation of these signaling pathways is absent in MyD88-deficient mice in response to all TLRs, except TLR4 and TLR3. This is due to the activation of an alternative pathway triggered by Trif (MyD88-independent pathway) that culminates in the activation of NF-κB, MAPKs, and the transcription factors interferon-responsive factors (IRFs), whose are responsible for induction of type I IFNs, in particular IFNβ (Honda et al., 2006; Stetson and Medzhitov, 2006). Besides MyD88 and Trif, two other adaptor proteins have been described: TIR-domain-containing adaptor protein (TIRAP, required for MyD88-dependent signaling by TLR2 and TLR4), and Trif-related adaptor molecule (TRAM, required for Trif-dependent signaling through TLR4, but not TLR3; Takeda and Akira, 2005; O’Neill and Bowie, 2007). Specific adaptors used by different TLRs combined with cell type-specific signaling pathways determine differential responses: inflammatory response, cell differentiation, proliferation, or apoptosis.
Expression of TLR 1, 2, 3, 4, 5, and 6 has been reported in human and mice AD-MSCs and BM-MSCs, human umbilical cord blood MSCs (UCB-MSCs), human Wharton jelly’s MSCs (WJ-MSCs), human dental pulp (DP), and dental follicle (DF)-MSCs (van den Berk et al., 2009; DelaRosa and Lombardo, 2010; Kim et al., 2010; Raicevic et al., 2011; Tomic et al., 2011). Expression and function of TLRs can be modulated in different ways in MSCs. Hypoxia significantly increased mRNA of TLR1, 2, 5, 9, and 10 (Hwa Cho et al., 2010). Infection of MSCs with baculoviral vectors upregulated expression of TLR3 and activated TLR3 signaling pathway (Chen et al., 2009). Interestingly, the inflammatory environment may also modulate the pattern and function of TLRs expressed by MSCs. When cultured in the presence of an “inflammatory cocktail” (made with IFNα, IFNγ, TNFα, and IL1β) expression of TLR2, 3, and 4 was increased, while TLR6 was downregulated (Raicevic et al., 2010). This modulatory effect seems to depend on the origin of MSCs as differences between BM, AD, and WJ-MSCs was found recently (Raicevic et al., 2011). Fatty acids may also modulate TLR signaling in ob/ob mouse AD-MSCs. Stearidonic and eicosapentainoic acids inhibited LPS-mediated upregulation of TLR2 through a mechanism that involves NF-κB but not ERK signaling pathway (Hsueh et al., 2011).
Adipogenic differentiation of human MSCs does not seem to be affected by TLRs (Hwa Cho et al., 2006; Liotta et al., 2008; Lombardo et al., 2009; Kim et al., 2010; Raicevic et al., 2010). Chondrogenic differentiation of human BM-MSCs has not been reported to be altered by activation through LPS, PolyIC, or R848 (Liotta et al., 2008), but was increased by TLR2 activation on human UCB-MSCs (Kim et al., 2010). The osteogenic differentiation seems to be enhanced in human BM-MSCs, AD-MSCs, and UCB-MSCs after LPS, PGN, or Poly IC activation (Hwa Cho et al., 2006; Mo et al., 2008; Lombardo et al., 2009; Kim et al., 2010), while CpG oligodeoxynucleotides (CpG ODN), have been reported to inhibit it on human AD-MSCs and BM-MSCs (Hwa Cho et al., 2006; Pevsner-Fischer et al., 2007; Liotta et al., 2008; Lombardo et al., 2009; Nørgaard et al., 2010). It has been reported recently that TNFα and TLRs activate osteogenic differentiation of AD-MSC via upregulation of transcriptional coactivator with PDZ-binding motif (TAZ; Hwa Cho et al., 2010).
On the other hand in mouse BM-MSCs, TLR2 was found to reduce differentiation into the three mesodermal lineages (Pevsner-Fischer et al., 2007). Interestingly, some reports link TLR signaling pathways with MSC multipotency. MyD88-deficient mouse BM-MSCs, when cultured in the appropriate differentiation media without additional stimulation with TLR ligands, effectively differentiated into adipocytes but failed to differentiate into osteocytes and chondrocytes (Pevsner-Fischer et al., 2007). However, TLR4-deficient mouse BM-MSCs showed higher differentiation rates compared to wild-type BM-MSCs (Wang et al., 2010). Nevertheless, TLR2-deficient mouse BM-MSCs failed to accumulate vacuoles in differentiated adipocytes, suggesting some impairment in the terminal differentiation process (Abarbanell et al., 2010). Therefore, the role of TLR signaling pathways in MSC multipotency needs to be further clarified.
So far, most of the studies have not found effects of TLR activation on human MSC proliferation. Only Hwa Cho et al. (2006) reported that TLR9 activation of AD-MSCs inhibited their proliferation. Interestingly, the use of TLR-deficient mouse BM-MSCs provided some insight on the role of TLRs on proliferation as TLR4-deficient BM-MSCs showed higher proliferation rates and TLR2-deficient showed reduced proliferation compared to wild-type MSCs (Abarbanell et al., 2010; Wang et al., 2010). In addition, TLR2 and TLR4 activation promoted proliferation of mouse BM-MSCs (Pevsner-Fischer et al., 2007; Wang et al., 2009).
Migration to the appropriate site of injury is believed to play a key role in the therapeutic efficacy of MSCs. Tomchuck et al. (2008) demonstrated that TLR3 activation drives the migration of human BM-MSCs in vitro. However, other reports found that TLR activation either impaired or had no effect on mouse BM-MSC migration (Pevsner-Fischer et al., 2007; Lei et al., 2011). In addition, TLR9 activation enhanced human BM-MSC invasion through a mechanism mediated, at least in part, by increased expression of MMP-13 (Nurmenniemi et al., 2010).
Mesenchymal stem cells have been shown to possess the capacity to inhibit proliferation of immune cells upon mitogenic or allogeneic activation. In recent years, inconsistent results have been reported regarding the role of TLR ligands on MSCs capacity to modulate immune responses. We and others found no significant effect of TLR activation on human AD-MSC or mouse BM-MSC-mediated immunosuppression (Pevsner-Fischer et al., 2007; Lombardo et al., 2009). However, other groups have reported that TLR activation may modulate the immunosuppressive properties of human BM-MSCs, although in very different ways. Liotta et al. (2008) found that TLR3 and TLR4 activation reduce the inhibitory activity of human BM-MSCs on T cell proliferation without influencing IDO activity or PGE2 levels, but downregulated expression of Jagged1, suggesting that the Notch signaling pathway mediates cell contact-mediated immunosuppression by MSCs. In contrast, Opitz et al. (2009) reported that TLR3 and TLR4 engagement enhances the immunosuppressive properties of human BM-MSCs through the indirect induction of IDO1. Induction of IDO1 involved an autocrine IFNβ signaling loop, which was dependent on protein kinase R (PKR) and independent of IFNγ. The role of IDO seems to be species dependent as Lanz et al. (2010) reported recently that IDO activity is not required for mouse BM-MSC immunosuppressive capacity both in vitro and in vivo, using IDO-deficient MSCs. Interestingly, TLR2 activation has been reported to impair the capacity of mouse BM-MSCs to induce the generation of regulatory T cells (Lei et al., 2011). Adding more uncertainty, Raicevic et al. (2010) reported that preactivation of human BM-MSCs with TLR3 or TLR4 ligands reduced production of HGF and PGE2 which impaired their capacity to inhibit lymphocyte proliferation. However, these authors found in a later report, that triggering of TLR3 or TLR4 on human MSCs from BM, AD, and Wharton jelly’s did not affect their immunosuppressive capacity (Raicevic et al., 2011). Dental pulp (DP) and Dental follicle (DF)-MSCs can also modulate lymphocyte proliferation in vitro, which is potentiated by TLR3 activation in both cell types, whereas TLR4 activation increased the suppressive role of DF-MSCs and reduced it in DP-MSCs (Tomic et al., 2011). Immunomodulating properties of human umbilical cord blood (UCB-MSCs) were not affected by prestimulation with TLR4 or TLR5 ligands (van den Berk et al., 2009).
Toll-like receptors may polarize MSCs toward pro-inflammatory and antigen-presenting-like phenotypes leading to release of pro-inflammatory cytokines and chemokines capable of enhancing recruitment of inflammatory immune cells (Romieu-Mourez et al., 2009). In line with this, a “licensing” process of MSCs toward either pro-inflammatory (MSC1) or anti-inflammatory (MSC2) phenotypes, which depends on the ligand concentration, timing, and kinetics of activation, has been proposed (Waterman et al., 2010). TLR4 priming results in upregulation of mostly pro-inflammatory cytokines such as IL6 or IL8 (MSC1 phenotype), while TLR3 priming results in production of anti-inflammatory molecules such as IL4, IDO, or PGE2 (MSC2 phenotype). TLR3-activated MSCs maintained the capacity to inhibit lymphocyte proliferation in vitro, while TLR4-primed MSCs activated T lymphocytes. As suggested by the authors, the polarizing effects of TLR priming may also explain the contradictory results obtained so far on the effects of TLRs on immunomodulation by MSCs.
There are other immune functions mediated by MSCs which have been found to be modulated by TLRs. BM-MSCs and parotid-derived MSCs have been shown to support neutrophil survival and chemotaxis in a ratio dependent manner through the release of soluble factors (Raffaghello et al., 2008; Brandau et al., 2010). Recently, Cassatella and colleagues found that TLR3 and TLR4 ligands enhanced the capacity of MSCs to delay neutrophil apoptosis through the induction of IL6, IFNγ, and GM-CSF. Moreover, TLR activation of BM-MSCs strongly increased respiratory burst of neutrophils. This supportive role on neutrophil function was confirmed using MSCs from thymus, spleen, or adipose tissue (Cassatella et al., 2011).
TLR2 and TLR4 mediate the capacity of human BM-MSCs to support short-term expansion of umbilical cord CD34+ cells, promoting myeloid-differentiation through the induction of hematopoietic growth factors (Wang et al., 2012). Moreover, it has been recently reported that resident mouse BM-MSCs, by producing MCP-1 in response to LPS, induce monocyte emigration from bone marrow into circulation to confront potential infections (Shi et al., 2011). These findings suggest an important role for TLRs in the modulation of the immune system by resident MSCs since BM-MSCs could function as sensors of circulating TLR ligands and determine, by expressing MCP-1, the frequency of circulating inflammatory Ly6Chigh, CCR2+ monocytes.
Several studies have reported beneficial effects of MSC treatment in animal models of sepsis or LPS-induced lung injury (in which MSCs were administered within 1 h following LPS challenge; Mei et al., 2007, 2010; Xu et al., 2007; Gonzalez-Rey et al., 2009; Németh et al., 2009, 2010). Based on the therapeutic benefit observed in these experimental models, it can be interpreted that high concentrations of LPS did not polarize MSCs toward a pro-inflammatory phenotype, in apparent contradiction to the reported polarizing process observed in vitro (Waterman et al., 2010). However, Waterman et al. (2010) reported that MSC1 and MSC2 cells were used in mouse models of lung injury and MSC1 aggravated the inflammatory injury, whereas MSC2 improved it, when compared to unstimulated BM-MSCs.
Conflicting results have been reported regarding the modulation of MSC-mediated cardiac protection by TLRs. LPS preconditioning of mouse BM-MSCs can, when compared to unconditioned MSCs, improve their survival and engraftment and increases the release of vascular endothelial growth factor (VEGF) in a model of rat acute myocardial infarction leading to enhanced therapeutic effects (Yao et al., 2009). These effects can be mediated through a TLR4-mediated protection of MSCs from apoptosis induced by oxidative stress (Wang et al., 2009). In contrast, TLR4-deficient mouse BM-MSCs had increased cardiac protection which was mediated by activated STAT3 signaling, leading to expression of higher levels of angiogenic factors such as VEGF and HGF (Wang et al., 2010). TLR2 activity also seems to be involved in cardioprotective effects by mouse BM-MSCs after ischemia/reperfusion injury. TLR2-deficient mouse BM-MSC showed impaired capacity to recover heart function, which correlates with reduced production of VEGF in hearts treated with TLR2-deficient MSCs compared to wild-type controls (Abarbanell et al., 2010). Therefore, further investigation in experimental animal models is required to clarify the role of TLRs in the licensing process as well as in the therapeutic potential of MSCs in vivo.
Despite discrepancies and inconsistencies reported by authors, some general conclusions can be made: (a) TLR expression: MSCs from different sources express TLRs at the mRNA level, although expression at a protein level seems to be low (i.e., compared to monocytes), and often makes difficult detection by flow cytometry, (b) MSC differentiation: in human MSCs, adipogenic differentiation does not seem to be affected by TLRs but osteogenic differentiation seems to be enhanced by TLR2, TLR3, or TLR4, while inhibited by TLR9. In mouse MSCs, TLR signaling might be linked to multipotency of MSCs as MyD88-deficient BM-MSCs failed to efficiently differentiate into chodrogenic and osteogenic lineage, (c) MSC proliferation: in human MSCs, only TLR9 activation has been reported to affect AD-MSC proliferation, (d) immunomodulatory capacity of MSCs: contradictory results have been reported that can be explained, at least in part, by the experimental conditions and the source of MSCs. The fact that differences in the experimental settings may lead MSCs to behave differently, suggests that MSCs can adjust their response in a dynamic way to the specific environmental conditions they face. In this regard, Waterman et al. (2010) challenged the concept of MSCs being always immunosuppressive and suggested that a polarizing process toward a pro-inflammatory or anti-inflammatory phenotype may occur depending on the TLR activated. However, the anti-inflammatory and therapeutic effects reported in mouse models of sepsis and lung injury, where MSCs were exposed to high levels of LPS, seems to be in apparent contradiction to the polarizing process described in vitro. Therefore, the in vivo modulation of MSC biology by TLR ligands deserves to be further investigated and clarified.
The inflammatory conditions MSCs face when administered in vivo is now believed to play a fundamental role in their successful therapeutic use. Research on modulation of MSCs by TLRs can strongly contribute to better understand the immunomodulating properties of MSCs under different inflammatory environments and to characterize the features an inflammatory milieu should have for MSCs to best modulate immune reactions (i.e., composition, ratio or activity of immune cells, cytokines or other inflammatory mediators such as TLR ligands).
Authors are full time employees of TiGenix.
We thank Debjani Roy for critical reading of the manuscript. This work was supported by funding from the Ministry of Science and Innovation of Spain (INNPACTO IPT-010000-2010-40) and a grant from the European Union Seventh Framework Programme: REGENER-AR, grant agreement no. 279174.
Abarbanell, A. M., Wang, Y., Herrmann, J. L., Weil, B. R., Poynter, J. A., Manukyan, M. C., and Meldrum, D. R. (2010). Toll-like receptor 2 mediates mesenchymal stem cell-associated myocardial recovery and VEGF production following acute ischemia-reperfusion injury. Am. J. Physiol. Heart Circ. Physiol. 298, 1529–1536.
Akira, S., Uematsu, S., and Takeuchi, O. (2006). Pathogen recognition and innate immunity. Cell 124, 783–801.
Asea, A., Kraeft, S. K., Kurt-Jones, E. A., Stevenson, M. A., Chen, L. B., Finberg, R. W., Koo, G. C., and Calderwood, S. K. (2000). HSP70 stimulates cytokine production through a CD14-dependant pathway, demonstrating its dual role as a chaperone and cytokine. Nat. Med. 6, 435–442.
Barrat, F. J., Meeker, T., Gregorio, J., Chan, J. H., Uematsu, S., Akira, S., Chang, B., Duramad, O., and Coffman, R. L. (2005). Nucleic acids of mammalian origin can act as endogenous ligands for toll-like receptors and may promote systemic lupus erythematosus. J. Exp. Med. 202, 1131–1139.
Bartholomew, A., Sturgeon, C., Siatskas, M., Ferrer, K., McIntosh, K., Patil, S., Hardy, W., Devine, S., Ucker, D., Deans, R., Moseley, A., and Hoffman, R. (2002). Mesenchymal stem cells suppress lymphocyte proliferation in vitro and prolong skin graft survival in vivo. Exp. Hematol. 30, 42–48.
Beyth, S., Borovsky, Z., Mevorach, D., Liebergall, M., Gazit, Z., Aslan, H., Galun, E., and Rachmilewitz, J. (2005). Human mesenchymal stem cells alter antigen-presenting cell maturation and induce T-cell unresponsiveness. Blood 105, 2214–2219.
Boule, M. W., Broughton, C., Mackay, F., Akira, S., Marshak-Rothstein, A., and Rifkin, I. (2004). Toll-like Receptor 9-dependent and -independent dendritic cell activation by chromatin-immunoglobulin G complexes. J. Exp. Med. 199, 1631–1640.
Brandau, S., Jakob, M., Hemeda, H., Bruderek, K., Janeschik, S., Bootz, F., and Lang, S. (2010). Tissue-resident mesenchymal stem cells attract peripheral blood neutrophils and enhance their inflammatory activity in response to microbial challenge. J. Leukoc. Biol. 88, 1005–1015.
Cassatella, M. A., Mosna, F., Micheletti, A., Lisi, V., Tamassia, N., Cont, C., Calzetti, F., Pelletier, M., Pizzolo, G., and Krampera, M. (2011). Toll-like receptor-3-activated human mesenchymal stromal cells significantly prolong the survival and function of neutrophils. Stem Cells 29, 1001–1011.
Chabannes, D., Hill, M., Merieau, E., Rossignol, J., Brion, R., Soulillou, J. P., Anegon, I., and Cuturi, M. C. (2007). A role for heme oxygenase-1 in the immunosuppressive effect of adult rat and human mesenchymal stem cells. Blood 110, 3691–3694.
Chamberlain, G., Fox, J., Ashton, B., and Middleton, J. (2007). Concise review: mesenchymal stem cells: their phenotype, differentiation capacity, immunological features, and potential for homing. Stem Cells 25, 2739.
Chen, G. Y., Shiah, H. C., Su, H. J., Chen, C. Y., Chuang, Y. J., Lo, W. H., Huang, J. L., Chuang, C. K., Hwang, S. M., and Hu, Y. C. (2009). Baculovirus transduction of mesenchymal stem cells triggers the toll-like receptor 3 pathway. J. Virol. 83, 10548–10556.
Chiesa, S., Morbelli, S., Morando, S., Massollo, M., Marini, C., Bertoni, A., Frassoni, F., Bartolomé, S. T., Sambuceti, G., Traggiai, E., and Uccelli, A. (2011). Mesenchymal stem cells impair in vivo T-cell priming by dendritic cells. Proc. Natl. Acad. Sci. U.S.A. 108, 17384–17389.
Cui, L., Yin, S., Liu, W., Li, N., Zhang, W., and Cao, Y. (2007). Expanded adipose-derived stem cells suppress mixed lymphocyte reaction by secretion of prostaglandin E2. Tissue Eng. 13, 1185–1195.
De Bari, C., Dell’Accio, F., Tylzanowski, P., and Luyten, F. P. (2001). Multipotent mesenchymal stem cells from adult human synovial membrane. Arthritis Rheum. 44, 1928–1942.
De Ugarte, D. A., Morizono, K., Elbarbary, A., Alfonso, Z., Zuk, P. A., Zhu, M., Dragoo, J. L., Ashjian, P., Thomas, B., Benhaim, P., Chen, I., Fraser, J., and Hedrick, M. H. (2003). Comparison of multi-lineage cells from human adipose tissue and bone marrow. Cells Tissues Organs (Print) 174, 101–109.
DelaRosa, O., and Lombardo, E. (2010). Modulation of adult mesenchymal stem cells activity by toll-like receptors: implications on therapeutic potential. Mediators Inflamm. 2010, 865601.
DelaRosa, O., Lombardo, E., Beraza, A., Mancheño-Corvo, P., Ramirez, C., Menta, R., Rico, L., Camarillo, E., García, L., Abad, J. L., Trigueros, C., Delgado, M., and Büscher, D. (2009). Requirement of IFN-gamma-mediated indoleamine 2,3-dioxygenase expression in the modulation of lymphocyte proliferation by human adipose-derived stem cells. Tissue. Eng. Part. A. 15, 2795–2806.
DelaRosa, O., Sánchez-Correa, B., Morgado, S., Ramírez, C., Del Río, B., Menta, R., Lombardo, E., Tarazona, R., and Casado, J. G. (2012). Human adipose-derived stem cells impair natural killer cell function and exhibit low susceptibility to natural killer-mediated lysis. Stem Cells Dev. 21, 1333–1343.
Dominici, M., Le Blanc, K., Mueller, I., Slaper-Cortenbach, I., Marini, F., Krause, D., Deans, R., Keating, A., Prockop, D. J., and Horwitz, E. (2006). Minimal criteria for defining multipotent mesenchymal stromal cells. The International Society for Cellular Therapy position statement. Cytotherapy 8, 315–317.
Doorn, J., Moll, G., Le Blanc, K., van Blitterswijk, C., and de Boer, J. (2012). Therapeutic applications of mesenchymal stromal cells: paracrine effects and potential improvements. Tissue Eng. Part B Rev. 2012 18, 101–115.
Friedenstein, A. J., Gorskaja, J. F., and Kulagina, N. N. (1976). Fibroblast precursors in normal and irradiated mouse hematopoietic organs. Exp. Hematol. 4, 267–274.
Fukuchi, Y., Nakajima, H., Sugiyama, D., Hirose, I., Kitamura, T., and Tsuji, K. (2004). Human placenta-derived cells have mesenchymal stem/progenitor cell potential. Stem Cells 22, 649–658.
Funderburg, N., Lederman, M. M., Feng, Z., Drage, M. G., Jadlowsky, J., Harding, C. V., Weinberg, A., and Sieg, S. F. (2007). Human-defensin-3 activates professional antigen-presenting cells via toll-like receptors 1 and 2. Proc. Natl. Acad. Sci. U.S.A. 104, 18631–18635.
Glennie, S., Soeiro, I., Dyson, P. J., Lam, E. W., and Dazzi, F. (2005). Bone marrow mesenchymal stem cells induce division arrest anergy of activated T cells. Blood 105, 2821–2827.
González, M. A., Gonzalez-Rey, E., Rico, L., Büscher, D., and Delgado, M. (2009a). Treatment of experimental arthritis by inducing immune tolerance with human adipose-derived mesenchymal stem cells. Arthritis Rheum. 60, 1006–1019.
González, M. A., Gonzalez-Rey, E., Rico, L., Büscher, D., and Delgado, M. (2009b). Adipose-derived mesenchymal stem cells alleviate experimental colitis by inhibiting inflammatory and autoimmune responses. Gastroenterology 136, 978–989.
Gonzalez-Rey, E., Anderson, P., González, M. A., Rico, L., Büscher, D., and Delgado, M. (2009). Human adult stem cells derived from adipose tissue protect against experimental colitis and sepsis. Gut 58, 929–939.
Gonzalez-Rey, E., Gonzalez, M. A., Varela, N., O’Valle, F., Hernandez-Cortes, P., Rico, L., Büscher, D., and Delgado, M. (2010). Human adipose-derived mesenchymal stem cells reduce inflammatory and T cell responses and induce regulatory T cells in vitro in rheumatoid arthritis. Ann. Rheum. Dis. 69, 241–248.
Griffin, M. D., Ritter, T., and Mahon, B. P. (2010). Immunological aspects of allogeneic mesenchymal stem cell therapies. Hum. Gene Ther. 21, 1641–1655.
Gronthos, S., Mankani, M., Brahim, J., Robey, P. G., and Shi, S. (2000). Postnatal human dental pulp stem cells (DPSCs) in vitro and in vivo. Proc. Natl. Acad. Sci. U.S.A. 97, 13625–13630.
Hoebe, K., Jiang, Z., Georgel, P., Tabeta, K., Janssen, E., Du, X., and Beutler, B. (2006). TLR signaling pathways: opportunities for activation and blockade in pursuit of therapy. Curr. Pharm. Des. 12, 4123–4134.
Honda, K., Takaoka, A., and Taniguchi, T. (2006). Type I interferon [corrected] gene induction by the interferon regulatory factor family of transcription factors. Immunity 25, 349–360.
Hsueh, H. W., Zhou, Z., Whelan, J., Allen, K. G., Moustaid-Moussa, N., Kim, H., and Claycombe, K. J. (2011). Stearidonic and eicosapentaenoic acids inhibit interleukin-6 expression in ob/ob mouse adipose stem cells via Toll-like receptor-2-mediated pathways. J. Nutr. 141, 1260–1266.
Hwa Cho, H., Bae, Y. C., and Jung, J. S. (2006). Role of toll-like receptors on human adipose-derived stromal cells. Stem Cells 24, 2744–2752.
Hwa Cho, H., Shin, K. K., Kim, Y. J., Song, J. S., Kim, J. M., Bae, Y. C., Kim, C. D., and Jung, J. S. (2010). NF-kappaB activation stimulates osteogenic differentiation of mesenchymal stem cells derived from human adipose tissue by increasing TAZ expression. J. Cell. Physiol. 223, 168–177.
Ishihara, S., Rumi, M. A., Ortega-Cava, C. F., Kazumori, H., Kadowaki, Y., Ishimura, N., and Kinoshita, Y. (2006). Therapeutic targeting of toll-like receptors in gastrointestinal inflammation. Curr. Pharm. Des. 12, 4215–4228.
Johnson, G., Brunn, G. J., Kodaira, Y., and Platt, J. L. (2002). Receptor-mediated monitoring of tissue well-being via detection of soluble heparan sulfate by toll-like receptor 4. J. Immunol. 168, 5233–5239.
Kim, H. S., Shin, T. H., Yang, S. R., Seo, M. S., Kim, D. J., Kang, S. K., Park, J. H., and Kang, K. S. (2010). Implication of NOD1 and NOD2 for the differentiation of multipotent mesenchymal stem cells derived from human umbilical cord blood. PLoS ONE 5, e15369. doi:10.1371/journal.pone.0015369
Krampera, M. (2011). Mesenchymal stromal cell ‘licensing’: a multistep process. Leukemia 25, 1408–1414.
Krampera, M., Cosmi, L., Angeli, R., Pasini, A., Liotta, F., Andreini, A., Santarlasci, V., Mazzinghi, B., Pizzolo, G., Vinante, F., Romagnani, P., Maggi, E., Romagnani, S., and Annunziato, F. (2006). Role for interferon-gamma in the immunomodulatory activity of human bone marrow mesenchymal stem cells. Stem Cells 24, 386–398.
Krampera, M., Glennie, S., Dyson, J., Scott, D., Laylor, R., Simpson, E., and Dazzi, F. (2003). Bone marrow mesenchymal stem cells inhibit the response of naive and memory antigen-specific T cells to their cognate peptide. Blood 101, 3722–3729.
Lanz, T. V., Opitz, C. A., Ho, P. P., Agrawal, A., Lutz, C., Weller, M., Mellor, A. L., Steinman, L., Wick, W., and Platten, M. (2010). Mouse mesenchymal stem cells suppress antigen-specific TH cell immunity independent of indoleamine 2,3-dioxygenase 1 (IDO1). Stem Cells Dev. 19, 657–668.
Le Blanc, K., Tammik, C., Rosendahl, K., Zetterberg, E., and Ringdén, O. (2003a). HLA expression and immunologic properties of differentiated and undifferentiated mesenchymal stem cells. Exp. Hematol. 31, 890–896.
Le Blanc, K., Tammik, L., Sundberg, B., Haynesworth, S. E., and Ringdén, O. (2003b). Mesenchymal stem cells inhibit and stimulate mixed lymphocyte cultures and mitogenic responses independently of the major histocompatibility complex. Scand. J. Immunol. 57, 1.
Le Blanc, K., Rasmusson, I., Sundberg, B., Götherström, C., Hassan, M., Uzunel, M., and Ringdén, O. (2004). Treatment of severe acute graft-versus-host disease with third party haploidentical mesenchymal stem cells. Lancet 363, 1439–1441.
Lei, J., Wang, Z., Hui, D., Yu, W., Zhou, D., Xia, W., Chen, C., Zhang, Q., Wang, Z., Zhang, Q., and Xiang, A. P. (2011). Ligation of TLR2 and TLR4 on murine bone marrow-derived mesenchymal stem cells triggers differential effects on their immunosuppressive activity. Cell. Immunol. 271, 147–156.
Liotta, F., Angeli, R., Cosmi, L., Filí, L., Manuelli, C., Frosali, F., Mazzinghi, B., Maggi, L., Pasini, A., Lisi, V., Santarlasci, V., Consoloni, L., Angelotti, M. L., Romagnani, P., Parronchi, P., Krampera, M., Maggi, E., Romagnani, S., and Annunziato, F. (2008). Toll-like receptors 3 and 4 are expressed by human bone marrow-derived mesenchymal stem cells and can inhibit their T-cell modulatory activity by impairing Notch signaling. Stem Cells 26, 279–289.
Liu-Bryan, I., Sott, P., Sydlaske, A., Rose, D. M., and Terkeltaub, R. (2005). Innate immunity conferred by toll-like receptors 2 and 4 and myeloid differentiation factor 88 expression is pivotal to monosodium urate monohydrate crystal-induced inflammation. Arthritis Rheum. 52, 2936–2946.
Lombardo, E., DelaRosa, O., Mancheño-Corvo, P., Menta, R., Ramírez, C., and Büscher, D. (2009). Toll-like receptor-mediated signaling in human adipose-derived stem cells: implications for immunogenicity and immunosuppressive potential. Tissue. Eng. Part A. 15, 1579–1589.
Maccario, R., Podestà, M., Moretta, A., Cometa, A., Comoli, P., Montagna, D., Daudt, L., Ibatici, A., Piaggio, G., Pozzi, S., Frassoni, F., and Locatelli, F. (2005). Interaction of human mesenchymal stem cells with cells involved in alloantigen-specific immune response favors the differentiation of CD4+ T-cell subsets expressing a regulatory/suppressive phenotype. Haematologica 90, 516–255.
Majumdar, M. K., Keane-Moore, M., Buyaner, D., Hardy, W. B., Moorman, M. A., McIntosh, K. R., and Mosca, J. D. (2003). Characterization and functionality of cell surface molecules on human mesenchymal stem cells. J. Biomed. Sci. 10, 2.
McIntosh, K., Zvonic, S., Garrett, S., Mitchell, J. B., Floyd, Z. E., Hammill, L., Kloster, A., Di Halvorsen, Y., Ting, J. P., Storms, R. W., Goh, B., Kilroy, G., Wu, X., and Gimble, J. M. (2006). The immunogenicity of human adipose-derived cells: temporal changes in vitro. Stem Cells 24, 1246–1253.
Mei, S. H., Haitsma, J. J., Dos Santos, C. C., Deng, Y., Lai, P. F., Slutsky, A. S., Liles, W. C., and Stewart, D. J. (2010). Mesenchymal stem cells reduce inflammation while enhancing bacterial clearance and improving survival in sepsis. Am. J. Respir. Crit. Care Med. 182, 1047–1057.
Mei, S. H., McCarter, S. D., Deng, Y., Parker, C. H., Liles, W. C., and Stewart, D. J. (2007). Prevention of LPS-induced acute lung injury in mice by mesenchymal stem cells overexpressing angiopoietin 1. PLoS Med. 4, 1525–1537. doi:10.1371/journal.pmed.0040269
Meisel, R., Brockers, S., Heseler, K., Degistirici, O., Bülle, H., Woite, C., Stuhlsatz, S., Schwippert, W., Jäger, M., Sorg, R., Henschler, R., Seissler, J., Dilloo, D., and Däubener, W. (2011). Human but not murine multipotent mesenchymal stromal cells exhibit broad-spectrum antimicrobial effector function mediated by indoleamine 2,3-dioxygenase. Leukemia 25, 648–654.
Methe, H., Zimmer, E., Grimm, C., Nabauer, M., and Koglin, J. (2004). Evidence for a role of toll-like receptor 4 in development of chronic allograft rejection after cardiac transplantation. Transplantation 78, 1324–1331.
Meylan, E., Tschopp, J., and Karin, M. (2006). Intracellular pattern recognition receptors in the host response. Nature 442, 39–44.
Miller, Y. L., Variyakosol, S., Binder, C. J., Feramisco, J. R., Kirkland, T. N., and Witztum, J. (2003). Minimally modified LDL binds to CD14, induces macrophage spreading via TLR4/MD2, and inhibit phagocytosis of apoptotic cells. J. Biol. Chem. 278, 1561–1568.
Mo, I. F., Yip, K. H., Chan, W. K., Law, H. K., Lau, Y. L., and Chan, G. C. (2008). Prolonged exposure to bacterial toxins downregulated expression of toll-like receptors in mesenchymal stromal cell-derived osteoprogenitors. BMC Cell Biol. 9, 52. doi:10.1186/1471-2121-9-52
Nauta, A. J., Kruisselbrink, A. B., Lurvink, E., Willemze, R., and Fibbe, W. E. (2006). Mesenchymal stem cells inhibit generation and function of both CD34+-derived and monocyte-derived dendritic cells. J. Immunol. 177, 2080–2087.
Németh, K., Leelahavanichkul, A., Yuen, P. S., Mayer, B., Parmelee, A., Doi, K., Robey, P. G., Leelahavanichkul, K., Koller, B. H., Brown, J. M., Hu, X., Jelinek, I., Star, R. A., and Mezey, E. (2009). Bone marrow stromal cells attenuate sepsis via prostaglandin E(2)-dependent reprogramming of host macrophages to increase their interleukin-10 production. Nat. Med. 15, 42–49. [Erratum in: Nat Med. 2009 15, 462.]
Németh, K., Mayer, B., and Mezey, E. (2010). Modulation of bone marrow stromal cell functions in infectious diseases by toll-like receptor ligands. J. Mol. Med. (Berl.) 88, 5–10.
Noël, D., Caton, D., Roche, S., Bony, C., Lehmann, S., Casteilla, L., Jorgensen, C., and Cousin, B. (2008). Cell specific differences between human adipose-derived and mesenchymal-stromal cells despite similar differentiation potentials. Exp. Cell Res. 314, 1575–1584.
Nørgaard, N. N., Holien, T., Jönsson, S., Hella, H., Espevik, T., Sundan, A., and Standal, T. (2010). CpG-oligodeoxynucleotide inhibits Smad-dependent bone morphogenetic protein signaling: effects on myeloma cell apoptosis and in vitro osteoblastogenesis. J. Immunol. 185, 3131–3139.
Nurmenniemi, S., Kuvaja, P., Lehtonen, S., Tiuraniemi, S., Alahuhta, I., Mattila, R. K., Risteli, J., Salo, T., Selander, K. S., Nyberg, P., and Lehenkari, P. (2010). Toll-like receptor 9 ligands enhance mesenchymal stem cell invasion and expression of matrix metalloprotease-13. Exp. Cell Res. 316, 2676–2682.
Oashi, K., Burkart, V., Flohe, S., and Kolb, H. (2000). Heat shock protein 60 is a putative endogenous ligand of toll-like receptor-4 complex. J. Immunol. 164, 558–561.
Oh, I., Ozaki, K., Sato, K., Meguro, A., Tatara, R., Hatanaka, K., Nagai, T., Muroi, K., and Ozawa, K. (2007). Interferon-gamma and NF-kappaB mediate nitric oxide production by mesenchymal stromal cells. Biochem. Biophys. Res. Commun. 355, 956–962.
Ohnishi, S., Yanagawa, B., Tanaka, K., Miyahara, Y., Obata, H., Kataoka, M., Kodama, M., Ishibashi-Ueda, H., Kangawa, K., Kitamura, S., and Nagaya, N. (2007). Transplantation of mesenchymal stem cells attenuates myocardial injury and dysfunction in a rat model of acute myocarditis. J. Mol. Cell. Cardiol. 42, 88–97.
Okamura, Y., Watari, M., Jerud, E. S., Young, D. W., Ishizaka, S. T., Rose, J., Chow, J. C., and Strauss, J. F. III. (2001). The extra domain A of fibronectin activates Toll-like receptor 4. J. Biol. Chem. 276, 10229–10233.
O’Neill, L. A., and Bowie, A. G. (2007). The family of five: TIR-domain-containing adaptors in Toll-like receptor signalling. Nat. Rev. Immunol. 7, 353–364.
Opitz, C. A., Litzenburger, U. M., Lutz, C., Lanz, T. V., Tritschler, I., Köppel, A., Tolosa, E., Hoberg, M., Anderl, J., Aicher, W. K., Weller, M., Wick, W., and Platten, M. (2009). Toll-like receptor engagement enhances the immunosuppressive properties of human bone marrow-derived mesenchymal stem cells by inducing indoleamine-2,3-dioxygenase-1 via interferon-beta and protein kinase R. Stem Cells 27, 909–919.
Penack, O., Holler, E., and van den Brink, M. R. (2010). Graft-versus-host disease: regulation by microbe-associated molecules and innate immune receptors. Blood 115, 1865–7182.
Pevsner-Fischer, M., Morad, V., Cohen-Sfady, M., Rousso-Noori, L., Zanin-Zhorov, A., Cohen, S., Cohen, I. R., and Zipori, D. (2007). Toll-like receptors and their ligands control mesenchymal stem cell functions. Blood 109, 1422–1432.
Pittenger, M. F., Mackay, A. M., Beck, S. C., Jaiswal, R. K., Douglas, R., Mosca, J. D., Moorman, M. A., Simonetti, D. W., Craig, S., and Marshak, D. R. (1999). Multilineage potential of adult human mesenchymal stem cells. Science 284, 143–147.
Prasanna, S. J., Gopalakrishnan, D., Shankar, S. R., and Vasandan, A. B. (2010). Pro-inflammatory cytokines, IFNgamma and TNFalpha, influence immune properties of human bone marrow and Wharton jelly mesenchymal stem cells differentially. PLoS ONE 5, e9016. doi:10.1371.journal.pone.0009016
Puissant, B., Barreau, C., Bourin, P., Clavel, C., Corre, J., Bousquet, C., Taureau, C., Cousin, B., Abbal, M., Laharrague, P., Penicaud, L., Casteilla, L., and Blancher, A. (2005). Immunomodulatory effect of human adipose tissue-derived adult stem cells: comparison with bone marrow mesenchymal stem cells. Br. J. Haematol. 129, 118–129.
Raffaghello, L., Bianchi, G., Bertolotto, M., Montecucco, F., Busca, A., Dallegri, F., Ottonello, L., and Pistoia, V. (2008). Human mesenchymal stem cells inhibit neutrophil apoptosis: a model for neutrophil preservation in the bone marrow niche. Stem Cells 26, 151–162.
Raicevic, G., Najar, M., Stamatopoulos, B., De Bruyn, C., Meuleman, N., Bron, D., Toungouz, M., and Lagneaux, L. (2011). The source of human mesenchymal stromal cells influences their TLR profile as well as their functional properties. Cell. Immunol. 270, 207–216.
Raicevic, G., Rouas, R., Najar, M., Stordeur, P., Boufker, H. I., Bron, D., Martiat, P., Goldman, M., Nevessignsky, M. T., and Lagneaux, L. (2010). Inflammation modifies the pattern and the function of Toll-like receptors expressed by human mesenchymal stromal cells. Hum. Immunol. 71, 235–244.
Rasmusson, I., Ringdén, O., Sundberg, B., and Le Blanc, K. (2003). Mesenchymal stem cells inhibit the formation of cytotoxic T lymphocytes, but not activated cytotoxic T lymphocytes or natural killer cells. Transplantation 76, 1208–1213
Ren, G., Su, J., Zhang, L., Zhao, X., Ling, W., L’Huillie, A., Zhang, J., Lu, Y., Roberts, A. I., Ji, W., Zhang, H., Rabson, A. B., and Shi, Y. (2009). Species variation in the mechanisms of mesenchymal stem cell-mediated immunosuppression. Stem Cells 27, 1954–1962.
Romanov, Y. A., Svintsitskaya, V. A., and Smirnov, V. N. (2003). Searching for alternative sources of postnatal human mesenchymal stem cells: candidate MSC-like cells from umbilical cord. Stem Cells 21, 105–110.
Romieu-Mourez, R., François, M., Boivin, M. N., Bouchentouf, M., Spaner, D. E., and Galipeau, J. (2009). Cytokine modulation of TLR expression and activation in mesenchymal stromal cells leads to a proinflammatory phenotype. J. Immunol. 182, 7963–7973.
Selmani, Z., Naji, A., Zidi, I., Favier, B., Gaiffe, E., Obert, L., Borg, C., Saas, P., Tiberghien, P., Rouas-Freiss, N., Carosella, E. D., and Deschaseaux, F. (2008). Human leukocyte antigen-G5 secretion by human mesenchymal stem cells is required to suppress T lymphocyte and natural killer function and to induce CD4+CD25highFOXP3+ regulatory T cells. Stem Cells 26, 212–222.
Shi, C., Jia, T., Mendez-Ferrer, S., Hohl, T. M., Serbina, N. V., Lipuma, L., Leiner, I., Li, M. O., Frenette, P. S., and Pamer, E. G. (2011). Bone marrow mesenchymal stem and progenitor cells induce monocyte emigration in response to circulating toll-like receptor ligands. Immunity 34, 590–601.
Skalnikova, H., Motlik, J., Gadher, S. J., and Kovarova, H. (2011). Mapping of the secretome of primary isolates of mammalian cells, stem cells and derived cell lines. Proteomics 11, 691–708.
Termeer, C., Benedix, F., Sleeman, J., Fieber, C., Voith, U., Ahrens, T., Miyake, K., Freudenberg, M., Galanos, C., and Simon, J. C. (2002). Oligosaccharides of Hyaluronan activate dendritic cells via toll-like receptor 4. J. Exp. Med. 195, 99–111.
Tomchuck, S. L., Zwezdaryk, K. J., Coffelt, S. B., Waterman, R. S., Danka, E. S., and Scandurro, A. B. (2008). Toll-like receptors on human mesenchymal stem cells drive their migration and immunomodulating responses. Stem Cells 26, 99–107.
Tomic, S., Djokic, J., Vasilijic, S., Vucevic, D., Todorovic, V., Supic, G., and Colic, M. (2011). Immunomodulatory properties of mesenchymal stem cells derived from dental pulp and dental follicle are susceptible to activation by toll-like receptor agonists. Stem Cells Dev. 20, 695–708.
van den Berk, L. C., Jansen, B. J., Siebers-Vermeulen, K. G., Netea, M. G., Latuhihin, T., Bergevoet, S., Raymakers, R. A., Kögler, G., Figdor, C. C., Adema, G. J., and Torensma, R. (2009). Toll-like receptor triggering in cord blood mesenchymal stem cells. J. Cell. Mol. Med. 13, 3415–3426.
Vogl, T., Tenbrock, K., Ludwig, S., Leukert, N., Ehrhardt, C., van Zoelen, M. A., Nacken, W., Foell, D., van der Poll, T., Sorg, C., and Roth, J. (2007). Mrp8 and Mrp14 are endogenous activators of Toll-like receptor 4, promoting lethal, endotoxin-induced shock. Nat. Med. 13, 1042–1049.
Wang, Y., Abarbanell, A. M., Herrmann, J. L., Weil, B. R., Manukyan, M. C., Poynter, J. A., and Meldrum, D. R. (2010). TLR4 inhibits mesenchymal stem cell (MSC) STAT3 activation and thereby exerts deleterious effects on MSC-mediated cardioprotection. PLoS ONE 5, e14206. doi:10.1371.journal.pone.0014206
Wang, X., Cheng, Q., Li, L., Wang, J., Xia, L., Xu, X., and Sun, Z. (2012). Toll-like receptors 2 and 4 mediate the capacity of mesenchymal stromal cells to support the proliferation and differentiation of CD34? cells. Exp. Cell Res. 318, 196–206.
Wang, Z. J., Zhang, F. M., Wang, L. S., Yao, Y. W., Zhao, Q., and Gao, X. (2009). Lipopolysaccharides can protect mesenchymal stem cells (MSCs) from oxidative stress-induced apoptosis and enhance proliferation of MSCs via Toll-like receptor(TLR)-4 and PI3K/Akt. Cell Biol. Int. 33, 665–764.
Waterman, R. S., Tomchuck, S. L., Henkle, S. L., and Betancourt, A. M. (2010). A new mesenchymal stem cell (MSC) paradigm: polarization into a pro-inflammatory MSC1 or an Immunosuppressive MSC2 phenotype. PLoS ONE 5, e10088. doi:10.1371.journal.pone.0010088
Xu, J., Woods, C. R., Mora, A. L., Joodi, R., Brigham, K. L., Iyer, S., and Rojas, M. (2007). Prevention of endotoxin-induced systemic response by bone marrow-derived mesenchymal stem cells in mice. Am. J. Physiol. Lung Cell Mol. Physiol. 293, 131–141.
Yamamoto-Furusho, J. K., and Podolsky, D. K. (2007). Innate immunity in inflammatory bowel disease. World J. Gastroenterol. 13, 5577–5580.
Yang, D., Chen, Q., Su, S. B., Zhang, P., Kurosaka, K., Caspi, R. R., Michalek, S. M., Rosenberg, H. F., Zhang, N., and Oppenheim, J. J. (2008). Eosinophil-derived neurotoxin acts as an alarmin to activate the TLR2-MyD88 signal pathway in dendritic cells and enhances Th2 immune responses. J. Exp. Med. 205, 79–90.
Yañez, R., Lamana, M. L., García-Castro, J., Colmenero, I., Ramírez, M., and Bueren, J. A. (2006). Adipose tissue-derived mesenchymal stem cells have in vivo immunosuppressive properties applicable for the control of the graft-versus-host disease. Stem Cells 24, 2582–2591.
Yao, Y., Zhang, F., Wang, L., Zhang, G., Wang, Z., Chen, J., and Gao, X. (2009). Lipopolysaccharide preconditioning enhances the efficacy of mesenchymal stem cells transplantation in a rat model of acute myocardial infarction. J. Biomed. Sci. 16, 74.
Zappia, E., Casazza, S., Pedemonte, E., Benvenuto, F., Bonanni, I., Gerdoni, E., Giunti, D., Ceravolo, A., Cazzanti, F., Frassoni, F., Mancardi, G., and Uccelli, A. (2005). Mesenchymal stem cells ameliorate experimental autoimmune encephalomyelitis inducing T-cell anergy. Blood 106, 1755–1761.
Zhang, W., Ge, W., Li, C., You, S., Liao, L., Han, Q., Deng, W., and Zhao, R. C. (2004). Effects of mesenchymal stem cells on differentiation, maturation, and function of human monocyte-derived dendritic cells. Stem Cells Dev. 13, 263–271.
Zonca, M., Mancheño-Corvo, P., Delarosa, O., Mañes, S., Büscher, D., Lombardo, E., and Planelles, L. (2012). APRIL and BAFF proteins increase proliferation of human adipose-derived stem cells through activation of Erk1/2 MAP kinase. Tissue Eng. Part A 18, 852–859.
Keywords: toll-like receptor, mesenchymal stem cells, cell therapy
Citation: DelaRosa O, Dalemans W and Lombardo E (2012) Toll-like receptors as modulators of mesenchymal stem cells. Front. Immun. 3:182. doi: 10.3389/fimmu.2012.00182
Received: 14 March 2012; Accepted: 13 June 2012;
Published online: 02 July 2012.
Edited by:
Martin Johannes Hoogduijn, Erasmus Medical Center, NetherlandsReviewed by:
Richard Verbeek, DeltaLife, NetherlandsCopyright: © 2012 DelaRosa, Dalemans and Lombardo. This is an open-access article distributed under the terms of the Creative Commons Attribution Non Commercial License, which permits non-commercial use, distribution, and reproduction in other forums, provided the original authors and source are credited.
*Correspondence: Eleuterio Lombardo, Research and Development Department, TiGenix SA, Parque Tecnológico de Madrid, C/Marconi 1, 28760 Tres Cantos, Madrid, Spain. e-mail:ZWxldXRlcmlvLmxvbWJhcmRvQHRpZ2VuaXguY29t
Disclaimer: All claims expressed in this article are solely those of the authors and do not necessarily represent those of their affiliated organizations, or those of the publisher, the editors and the reviewers. Any product that may be evaluated in this article or claim that may be made by its manufacturer is not guaranteed or endorsed by the publisher.
Research integrity at Frontiers
Learn more about the work of our research integrity team to safeguard the quality of each article we publish.