- Unit of Innate Immunity and Tissue Remodelling, Division of Regenerative Medicine, Stem Cells and Gene Therapy, Istituto Scientifico San Raffaele, Milano, Italy
Macrophages are present in regenerating skeletal muscles and participate in the repair process. This is due to a unique feature of macrophages, i.e., their ability to perceive signals heralding ongoing tissue injury and to broadcast the news to cells suited at regenerating the tissue such as stem and progenitor cells. Macrophages play a complex role in the skeletal muscle, probably conveying information on the pattern of healing which is appropriate to ensure an effective healing of the tissue, yielding novel functional fibers. Conversely, they are likely to be involved in limiting the efficacy of regeneration, with formation of fibrotic scars and fat replacement of the tissue when the original insult persists. In this review we consider the beneficial versus the detrimental actions of macrophages during the response to muscle injury, with attention to the available information on the molecular code macrophages rely on to guide, throughout the various phases of muscle healing, the function of conventional and unconventional stem cells. Decrypting this code would represent a major step forward toward the establishment of novel targeted therapies for muscle diseases.
Muscle Injury and Inflammation
Resident leukocytes in the healthy skeletal muscle are exceedingly rare. Thereafter, the skeletal muscle represents a microenvironment in which immunologic reactions depend on the characteristics of the noxious event and on the nature and the function of newly recruited immune cells (Wiendl et al., 2005). Muscle inflammation is a common physiologic response to exercise and the hallmark of acute and chronic damages such as strain injury or muscular dystrophies. Muscle inflammation has been felt to run a rather stereotypical course; recent data however indicate that when persistent triggers cause muscle damage, differences exist in the recruitment of the humoral innate immunity at the site of tissue injury. For example, activation of the complement cascade occurs and contributes to the disease in dysferlin-deficient mice, a model for the muscle wasting diseases referred to as dysferlinopathies, but not in mdx mice, a mouse model of Duchenne Muscle Dystrophy (Han et al., 2010).
The inflammation in acutely damaged muscle is characterized by a rapid and sequential invasion of leukocyte populations that persist while muscle repair, regeneration, and growth occur (Paulsen et al., 2010). Neutrophils represent the first leukocyte population in the damaged tissue. They were found in muscle early after exercise completion (Fielding et al., 1993) and infiltrate the tissue for as long as 5 days. Neutrophils release molecules that may contribute to the muscle membrane lysis that follows injury (Nguyen et al., 2005). The actual final effect of neutrophil recruitment in damaged skeletal muscle is however not completely elucidated and recent results suggest that infiltration by neutrophils per se may not be harmful but additional inflammatory stimuli are required to reveal their detrimental potential (Dumont et al., 2008). Some such stimuli may be directly generated as a consequence of myofiber lysis and ensuing release of endogenous inflammatory molecules or may be contributed by other recruited cells, such as platelets (Maugeri et al., 2009; Manfredi et al., 2010).
Interestingly, genetic disruption of the chemokine pathway related to inflammatory leukocyte recruitment reveals an apparent balance between the extent and the duration of tissue infiltration by leukocytes: absence of CXCL16 not only resulted in defective homing of macrophages and severely jeopardized tissue regeneration, but was associated with a persistent and important infiltration of the tissue by neutrophils, with unrestrained inflammation and eventual fibrosis (Zhang et al., 2009b). Conversely, a significant increase in macrophage accumulation and cell proliferation was observed in mice in which a transient neutropenia was induced at early times after injury (Godbout et al., 2010). In general, macrophages represent the dominant leukocyte population in the late phases of the homeostatic response to injury or in conditions in which the original inflammatory noxa persists. Their ability to perceive environmental cues and orchestrate the functional activities of other cells populations, such as immune cells or myogenic precursors (Figure 1), makes them an intriguing field of study in muscle biology.
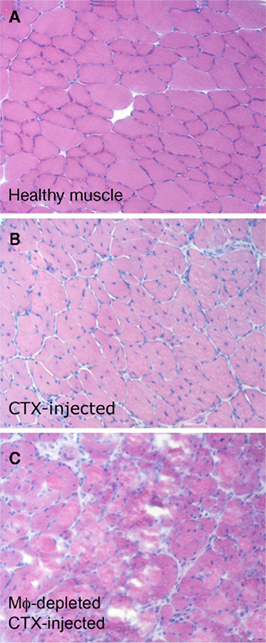
Figure 1. Macrophages, recruited to the skeletal muscle after acute sterile injury, are necessary for effective tissue regeneration. Two months old C57BL/6 mice were treated i.m. with cardiotoxin (CTX). Mice were treated or not with clodronate-containing liposomes to deplete macrophages and sacrificed 15 days after CTX injection. Tibialis anterior and quadriceps muscles were collected. (A) Healthy muscles of untreated control mice. (B) The muscle of macrophage-competent mice undergoes effective and almost complete regeneration in 15 days: regenerating centronucleated fibers are evident throughout the section. (C) In the absence of macrophages, 15 days after acute sterile injury regenerating fibers are hardly evident. Degenerated fibers and cell remnants persist.
Macrophages: What’s in a Name?
Macrophages were originally identified as phagocytic cells responsible for pathogen elimination and housekeeping functions in a wide range of organisms (Metchnikoff, 1905): they were thus included in the Mononuclear Phagocyte System, a population of cells, derived from bone marrow progenitors, that differentiate, enter the blood as monocytes and then the peripheral tissues to become resident macrophages or antigen presenting cells (Van Furth and Cohn, 1968).
Monocyte half-life in the blood is of about 1 day. This observation has fostered the concept that blood monocytes replenish macrophage or dendritic cell (DC) pools in peripheral tissues to maintain homeostasis (Ziegler-Heitbrock, 2000). This loop, by which tissues control the size and distribution of their macrophage populations, becomes evident when acute events, such as injury or infection occur.
Inflammation restricts the growth of invading microbes and guides, when the pathogen has been eliminated, its healing. Macrophages in particular represent an active link between innate and adaptive immunity, by regulating T lymphocyte activation and possibly shaping their polarization and function. The pioneering work on the role of T-cell-dependent protective autoimmunity in the healing of sterile spinal cord injuries makes this contention particularly cogent (Schwartz and Ziv, 2008; Shechter et al., 2009).
The role of macrophages is non-redundant. Depletion in the spleen of marginal zone macrophages, which interact with apoptotic material entering from the circulation, accelerated autoimmunity in mice genetically prone to systemic lupus erythematosus and caused significant mortality in wild-type mice repeatedly exposed to apoptotic cells (Mcgaha et al., 2011). Accumulation of apoptotic cell material per se triggers acceleration of systemic lupus erythematosus (Bondanza et al., 2003, 2004; Munoz et al., 2010). Macrophages recognize tags expressed by apoptotic cells: as a consequence they on one hand dispose of potentially reservoirs of autoantigens; on the other hand secrete regulatory cytokines that contribute to maintain self-tolerance (Manfredi et al., 2009; Elliott and Ravichandran, 2010). The clearance function of macrophages is crucial to limit the actual cross-presentation of apoptotic cell antigens and possibly to modify the cytokine environment in which the productive T-cell activation take place, thus favoring the establishment of protective, or at least not directly damaging, immune responses (Acharya et al., 2010; Elliott and Ravichandran, 2010; Brereton and Blander, 2011; Peng and Elkon, 2011).
Macrophages also support matrix remodeling and neoangiogenesis and have been implicated in conditions in which neoangiogenesis is potentially deleterious, including cancer (Qian and Pollard, 2010) but also non-neoplastic conditions, such as rheumatoid arthritis or endometriosis (Barrera et al., 2000; Bacci et al., 2009; Capobianco et al., 2010, 2011). All-together the data strongly support the ability of macrophages to perceive ongoing injury of various tissues, and to activate homeostatic programs that through the clearance of dying cells, the organization of neovessel generation, the regulation of the extracellular matrix remodeling and the activation of appropriate T lymphocyte responses leads to the effective healing.
Macrophage activation is clearly protective in the case of intense, short lasting injuries. In contrast, macrophage action sustaining regenerative and vascular responses can be deleterious in conditions such as persisting infectious diseases, chronic tissue damage, or event more notably tumors, in which the initial stimulus perceived by macrophages persists. Several excellent reviews have addressed the latter issue (e.g., see Biswas and Mantovani, 2010; Gordon and Martinez, 2010; Mantovani and Sica, 2010; Qian and Pollard, 2010; Squadrito and De Palma, 2011) and we will not discuss the issue further in this essay.
Dedicated pattern-recognition receptors (PRRs) are non-clonally expressed by most innate immune cells (Palm and Medzhitov, 2009). PRRs allow to indentify molecular structures shared by ample classes of microbes, referred to as pathogen-associated molecular patterns (PAMPs; Janeway, 1992). The activation of PRRs results in cascade of tightly coordinated events, including: (i) the production of cytokines and chemokines, which attract and activate leukocytes (Nathan, 2002) (ii) the activation of an acute phase response, with the production of conserved soluble PRRs, such as pentraxins (Manfredi et al., 2008) which tune leukocyte activation and limit their ability to damage the tissue; (iii) the migration of APC to draining lymph nodes, with productive activation of naïve T lymphocytes. The expansion of antigen-specific T-cells is a key event in the establishment of an adaptive immunological response (Bevan, 2011).
Damage-associated molecular pattern (DAMP), an array of heterogeneous molecules that are released during cell and tissue necrosis, although non-microbial, share the ability to activate PRRs (Table 1). Ss a consequence DAMPs recognition elicits inflammation and prompts tissue regeneration and acquired T-cell-dependent immune responses even in the context of sterile injuries (Bianchi, 2007; Lotze et al., 2007; Rubartelli and Lotze, 2007; Urbonaviciute et al., 2008; Bianchi and Manfredi, 2009; Manfredi and Rovere-Querini, 2010; Maroso et al., 2010; Castiglioni et al., 2011; Liu et al., 2011b; Zhang et al., 2011). Macrophages undergo an extensive reprogramming of their functional properties in response to PAMPs (Nau et al., 2002; Martinon et al., 2010), but also to signals released directly from damaged tissues (London et al., 2011) and from lymphocytes (Tiemessen et al., 2007; Wong et al., 2010; Liu et al., 2011a).
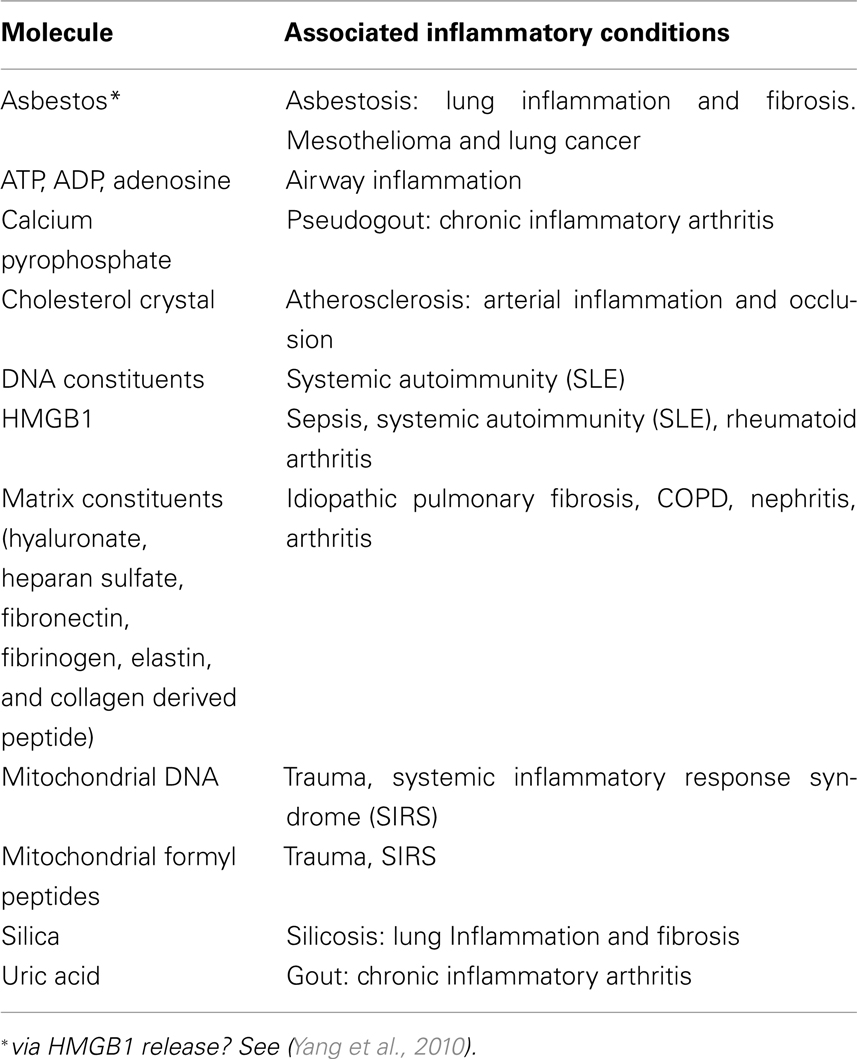
Table 1. Inflammatory sterile stimuli and associated diseases (Manfredi and Rovere-Querini, 2010; Rock et al., 2010, 2011; Zhang et al., 2010a; Castiglioni et al., 2011).
Studies with various probes reveal a phenotype heterogeneity in macrophages that possibly reflects peculiar features and function of macrophages sub-populations within the microenvironment. In response to microenvironmental cues, macrophages in the tissue become potent effector cells integrated in a T helper (Th)-1 response, which kill microorganisms and tumor cells and produce copious amounts of cytokines. Microbial destruction is mediated at least partially by the production of reactive oxygen species (ROS) and nitric oxide (NO). The amount of NO produced is instrumental for the ability of macrophages to control the intracellular parasite L. mexicana (Mylonas et al., 2009). In humans, classically activated macrophages are important for resistance to mycobacteria and Leishmania major infection (Darrah et al., 2007). Macrophages may undergo an alternative activation pathway (referred to as “alternative activation”) that endows them with the ability to tune inflammatory responses and adaptive immunity, scavenge debris, and promote angiogenesis, tissue remodeling, and repair (Mantovani et al., 2004).
Macrophages that infiltrate regenerating tissues in general belong to the second class of healing macrophages (Corna et al., 2010; Daley et al., 2010; O’brien et al., 2010; Schwartz, 2010; Brancato and Albina, 2011; Cairo et al., 2011; David and Kroner, 2011; Harel-Adar et al., 2011; Jaeschke, 2011; London et al., 2011; Wang and Harris, 2011), while unrestrained polarization toward a classically activated phenotype associates with defective healing and persistent inflammation (Sindrilaru et al., 2011). Healing (or alternatively activated) macrophages can be propagated in vitro by exposure to monocyte precursors to low concentrations of M-CSF in the presence of IL4, IL13, or IL10 (Mantovani et al., 2004). In contrast exposure to microbial components such as LPS in the presence of γIFN or to GM-CSF is an effective stimulus to elicit classically activated, inflammatory macrophages (Figure 2).
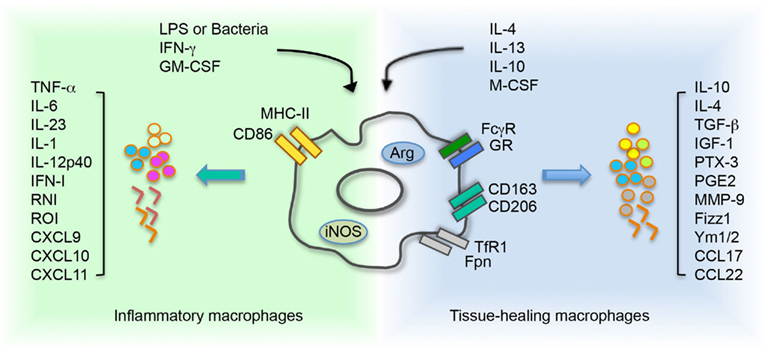
Figure 2. Environment cues control and influence macrophage polarization. Inflammatory macrophage activation is driven by stimulation with LPS or bacteria, and/or IFN-γ or GM-CSF. These molecules induce iNOS activity on macrophages and consequent production of RNI. Treatment with IL4 and/or IL13, IL10 and/or M-CSF supports the activation of tissue healing macrophages. The cytokine network activates an arginase-dependent metabolism of arginine. In macrophages, acquisition of a specific polarization state determines a differential expression of receptors and secretion of molecules. LPS, lipopolysaccharide; IFN-γ, interferon-γ; GM-CSF, granulocyte macrophage-colony stimulating factor; M-CSF, macrophage-colony stimulating factor; MHCII, major histocompatibility complex class II; GR, galactose receptor; CD163, haptoglobin–hemoglobin complex receptor; CD206, mannose receptor; TfR1, transferrin receptor 1; Fpn, ferroportin; TGF-β, transforming growth factor β; IGF1, insulin like growth factor 1; PTX-3, pentraxin 3; PGE2, prostaglandin E2; MMP-9, matrix metalloproteinase 9; Fizz, resistin like molecule; Ym1/2, chitinase like protein 1/2; TNF-α, tumor necrosis factor α; IFN, interferon type I; RNI, reactive nitrogen intermediate; ROI, reactive oxygen intermediate.
The dichotomy between inflammatory and tissue healing macrophages represents a “useful over-simplification” (Mantovani et al., 2009) of a sophisticated array of functions exerted by macrophage populations in injured tissues. The plasticity of macrophages in response to environmental cues has been characterized with particular attention in a model tissue, the skeletal muscle (Brunelli and Rovere-Querini, 2008; Chazaud et al., 2009; Tidball and Villalta, 2010).
A Case for Macrophages in the Skeletal Muscle
Macrophages have been known for a long time to be associated with skeletal muscle injury (Robertson et al., 1993; Mclennan, 1996). Moreover they play a critical role in the pathogenesis and in the natural history of self sustaining muscle diseases, specifically including primary inflammatory myopathies (necrotizing autoimmune myositis, inclusion body myositis and polymyositis) and genetic diseases of the tissue (Duchenne and Becker muscular dystrophies; Dalakas, 2002; Villalta et al., 2009). In vivo studies have unequivocally shown that macrophages actually participate in the tissue repair process (St Pierre and Tidball, 1994; Mclennan, 1996; Chazaud et al., 2003; Warren et al., 2005; Summan et al., 2006; Arnold et al., 2007; Tidball and Wehling-Henricks, 2007; Segawa et al., 2008; Ruffell et al., 2009; Sun et al., 2009; Brigitte et al., 2010; Dumont and Frenette, 2010; Martinez et al., 2010; Vezzoli et al., 2010; Lu et al., 2011b). Macrophages, as discussed above, are professional scavengers of apoptotic cells and debris and produce a vast array of signals involved in matrix remodeling and neovessel formation. Data in various models of skeletal muscle injury, including hindlimb ischemia, freeze-injury, unloading/reloading sequences, and myotoxic agent injection indicate that the recruitment of macrophages in the tissue occurs regardless of the characteristics of the original noxa (Tidball, 2002).
Arnold et al. (2007) identified a population of circulating monocytes selectively recruited in damaged muscle where they acquire a anti-inflammatory phenotype, correlated to tissue healing. Which are the signals involved in the functional polarization of macrophages in the injured/regenerating muscles? The phagocytosis of muscle cells debris is most likely to favor this transition (Arnold et al., 2007).
A macrophage population associated to the epimysial and perimysial connective tissue plays a crucial non-redundant role in monocyte attraction and activation in acutely injured skeletal muscle, providing signals that control their switch to tissue healing macrophages (Brigitte et al., 2010). Resident macrophages also attract in the injured skeletal muscle cells that express the CD11c integrin, a bona fide marker of myeloid DCs. These cells are endowed with antigen presenting capacity and with the ability to migrate from the muscle into draining lymph nodes (Brigitte et al., 2010). They represent attractive candidates to link the response to injury in the tissue to the local activation and recruitment of T lymphocytes, a hallmark of persistent skeletal muscle inflammation.
Activated myogenic precursors also generate chemoattractive signals for inflammatory cells, and their ability to recruit them at the site of muscle injury is further upregulated by the interaction with macrophages (Chazaud et al., 2003). After injury, myogenic precursors, injured fibers, resident macrophages, and recruited monocytes are a source of CCL2/MCP1 (Chazaud et al., 2003; Brigitte et al., 2010; Lu et al., 2011a). Indeed, severe impairments in skeletal muscle regeneration occur in mice defective of the chemokine-CC-motif receptor 2-deficient (CCR2−/−), which is activated by CCL2/MCP1 (Warren et al., 2005). CCR2 expression on bone marrow derived cells is essential for robust macrophage recruitment after acute sterile injury and muscle regeneration. Surprisingly, injured muscle of lethally irradiated mice transplanted with CCR2-deficient bone marrow cells contain, despite impaired muscle regeneration, increased numbers of myogenic progenitor cells (Sun et al., 2009), suggesting that macrophages are required for precursor cells to fuse and yield effective myofiber formation. Drastic reduction of macrophage recruitment in injured muscle of CCR2−/− mice associates to a dramatically reduced expression of insulin like growth factor 1 (IGF1), a central regulator of muscle regeneration (Lu et al., 2011b). This observation suggests that macrophages regulate muscle healing through IGF1. Indeed, in CCR2−/− mice, local IGF1 injection at least partially makes up for the lack of recruited macrophages (Lu et al., 2011b).
In vitro, IGF1 elicits in muscle cells a biphasic response, first stimulating cell proliferation and subsequently enhancing myogenic differentiation (Rosenthal and Cheng, 1995), a sequence of events that could be teleologically suited to sustain the repair of damaged tissue. In vivo, expression of a muscle specific transgene encoding a locally acting isoform of IGF1 prompts hypertrophy and regeneration in senescent skeletal muscle (Musaro et al., 2001).
The Muscle Microenvironment and the Inflammatory Response
The muscle environment and the mechanisms through which it modulates regeneration have attracted much attention in the recent years (Paylor et al., 2011). Several studies have investigated in particular whether similar events occur during the regeneration of adult skeletal muscle and during embryogenesis (Charge and Rudnicki, 2004). The microenvironment in which the two events occur is strikingly diverse: specifically muscle development occurs without any substantial contribution by infiltrating cells, which are instead present in regenerating muscle at concentrations that exceed 100,000 inflammatory cells/mm3 of tissue (Wehling et al., 2001; Paylor et al., 2011). Recent evidences suggest that a model in which myogenesis occurs independently of the activation of inflammatory pathway may be far too simplistic: for example, myoblasts of mouse embryos and regenerating myocytes in injured adult mouse skeletal muscle, but not mature myocytes, express the receptor for granulocyte colony stimulating factor (G-CSF). Moreover, the C-CSF/G-CSF receptor pathway is crucial for skeletal myocyte development and regeneration (Hara et al., 2011).
Although several molecules have been identified not to be dispensable for muscle regeneration, the overall array of signals macrophages deliver in the tissue and the hierarchy among them is far from being elucidated. At early stages after acute injury macrophages mostly secrete inflammatory molecules, including CCL2/MCP1 and TNFα, which may favor tissue wasting via activation of the FoxO transcription factor (Sandri et al., 2004; Zhao et al., 2007). Simultaneously, they dispose of apoptotic cells and fiber remnants: apoptotic cell clearance has been shown in other systems to trigger the release of cytokines involved in the termination of the inflammatory response and in immune regulation, such as TGFβ and IL10 (Huynh et al., 2002; Zhang et al., 2011; see also below).
At later stages macrophages actively sustain fiber reconstitution (Summan et al., 2006; Arnold et al., 2007; Shireman et al., 2007). At this stage they mainly secrete cytokines that may play a trophic function, such as IGF1 (Musaro et al., 2001; Summan et al., 2006; Pelosi et al., 2007) or IL10 (Strle et al., 2007, 2008; Tidball and Villalta, 2010).
In mdx mice, a well-accepted model for human Duchenne’s muscular dystrophy, the expression of IL10 modulates macrophage activation and reduces the membrane damage: in this system, IL10 has been proposed to deactivate the inflammatory profile of macrophages infiltrating damaged muscle at the early, acute stage of muscle disease, promoting a switch toward an alternative activation profile (Villalta et al., 2010). At later phases however the persistence of alternatively activated macrophages in conditions in which the tissue can not heal may actually play a deleterious role: for example the sustained production in mdx mice of TGFβ may be involved in the fibrotic substitution of the myofibers, a hallmark of the advanced phases of muscular dystrophy: the cytokine indeed is associated to fibroblast activation and proliferation, leading to sustained collagen production and eventually to fibrosis (Vidal et al., 2008).
An exclusive population of progenitors of both fibroblasts and adipocytes has been recently clearly identified in skeletal muscle (Joe et al., 2010; Uezumi et al., 2010), referred to as fibro/adipogenic progenitors (FAP). These cells remain in a quiescent state in normal conditions, undergo a dramatic but transient proliferation in response to injury, contextually delivering trophic signals for proliferating myogenic precursors. Fibrotic scar substitution and accumulation of lipid filled adipocytes is a feature of conditions of the failed regeneration of the skeletal muscle, like it occurs dramatically in muscle dystrophies but at some extent also during physiological aging: when myogenic precursors fail to replace the damaged tissue, FAP would according to recent models (Rodeheffer, 2010; Paylor et al., 2011) take over, differentiating into adipocytes and possibly fibroblasts, thus ensuring the structural continuity of the tissue.
Various stem cell populations, including mesenchymal stem cells (MSC) and neural precursor cells (NPC), regulate the leukocyte fate, through mechanisms involving cell–cell contact and/or various soluble factor. MSC inhibit proliferation of various immune cells, including T, B, and NK lymphocytes (Groh et al., 2005; Krampera et al., 2006; Sotiropoulou et al., 2006; Spaggiari et al., 2006) and specifically influence affect DCs function through the release of IL6, (Djouad et al., 2007), of the Notch ligand Jagged-2, which induces the generation of regulatory DCs (Zhang et al., 2009a) and of prostaglandin E2 (Spaggiari et al., 2009), while NPC restrict the activation of DCs via a BMP-4-dependent-mechanism (Pluchino et al., 2009). MSC also reprogramming macrophages toward an alternatively activated profile (Ohtaki et al., 2008; Kim and Hematti, 2009), which is instrumental in a model of skin wound healing for effective wound repair (Zhang et al., 2010b). The possible cross-talk between FAPs and other precursors of mesenchymal origin and inflammatory cells infiltrating injured skeletal muscle, although demonstrated in other model tissues (Stappenbeck and Miyoshi, 2009; Zhang et al., 2010b; Ehninger and Trumpp, 2011), has not to the best of our knowledge been directly investigated so far.
Macrophages and Myogenic Precursors
In the absence of macrophages injured muscle fail to regenerate (Figure 1; see also above), even if the number and function of the cell populations with myogenic potential in the tissue, the eventual effectors of muscle healing that repair or replace injured or dead fibers (Mauro, 1961), are not directly affected. The results indicate that macrophages actively “license” myogenic precursors to carry out their program, i.e., to proliferate, differentiate, and fuse and thus to regenerate the tissue. This is not an isolated feature of the skeletal muscle: macrophages for example sustain the stem cell survival in various tissues, including the skin and the bone marrow, a limiting step for their regeneration (Tothova and Gilliland, 2007; Blanpain and Fuchs, 2009; Discher et al., 2009; Gurumurthy et al., 2010).
It is still not clear at which level(s) macrophages actually specifically act. For example, myogenic progenitor cells were significantly increased in injured muscle of mice with CCR2-defective bone marrow cells even if muscle regeneration is severely affected (Sun et al., 2009). Satellite cells are considered the resident “stem-like” cells in skeletal muscle and are responsible for muscle growth and regeneration in postnatal life (Holterman and Rudnicki, 2005). In response to muscle injuries, quiescent satellite cells undergo activation, proliferate, and fuse with each other or with damaged fibers (Kuang et al., 2008); conversely, some precursors undergo self-renewal, and thus maintain the integrity of the quiescent satellite cell pool (Zammit, 2008; Kang and Krauss, 2010). A pathway strictly associated to the control of neoangiogenesis, which comprises the interaction between angiopoietin 1 (Ang1) and its receptor Tie-2 and the downstream activation of the ERK1/2 kinase, has been in elegant studies implicated in the ability of satellite cells to re-enter the stem cell niche (Abou-Khalil et al., 2009; Mounier et al., 2011), thus suggesting that endothelial cells and possibly other non-muscle cells, are involved in the maintenance of the satellite cell niche. The observation that even single transplanted satellite cells both differentiate and self-renew after transplantation in vivo (Sacco et al., 2008) provides a formal demonstration that satellite cells are indeed endowed with stem cell properties. Telomeres length in muscle cells and the control of muscle stem cell regenerative capacity represents, in particular in the setting of muscular dystrophies, a particular attractive target for the action of inflammatory molecules (Sacco et al., 2010).
Despite the concentrated effort of several groups, our actual insight on the role of the microenvironment in determining the overall outcome of the tissue response to injury is still fragmentary. Recent studies specifically highlight the importance of mechanical factors, such as tissue rigidity/elasticity in regulating the fate of muscle stem cells (Gilbert et al., 2010), revealing important caveats that apply to the in vitro systems that are commonly used. Other environmental influences are possibly as relevant: for example, we have recently observed that regeneration after an acute sterile injury of the skeletal muscle is accompanied by the substantial generation of ROS production, which is counterbalanced and rapidly overcome by the generation of antioxidant moieties. Mitochondria are initially responsible for ROS formation while at later time points, non-mitochondrial sources are involved. Both regenerating fibers and macrophages express high levels of free thiols and antioxidant enzymes, such as superoxide dismutase 1 (SOD1) and thioredoxin (Vezzoli et al., 2011). The well-characterized role of a reduced environment in maintaining the extracellular function of DAMP molecules (Lotze et al., 2007; Rubartelli and Lotze, 2007; Carta et al., 2009; Rubartelli and Sitia, 2009), either directly released by damaged fibers of actively secreted by infiltrating macrophages suggests that the antioxidant response directly contributes in the acutely injured tissue to homeostasis.
Preliminary data from our laboratory indicate that macrophages also influence other cells with myogenic potential, such as mesoangioblasts. Mesoangioblasts are vessel-associated progenitors that ameliorate defective muscle structure and function in dystrophic mice and dogs (Sampaolesi et al., 2003, 2006; Tedesco et al., 2010). A clonal analysis of embryonic explanted organ rudiments led to the positive identification of a “mesoangioblast” cell population in the embryonic dorsal aorta (De Angelis et al., 1999) and later studies implicated pericytes associated with microvascular walls in the human skeletal muscle as their human counterpart (Dellavalle et al., 2007). Mesoangioblasts are endowed with the ability to cross the vessel wall, a feature missing in satellite cell-derived myogenic precursors (Dellavalle et al., 2007): when injected into the blood, mesoangioblasts are indeed able to migrate outside the vessel toward injured and inflamed tissues, including the dystrophic muscle (Sampaolesi et al., 2003), possibly following chemotactic signals generated by activated innate cells, macrophages in particular (Lolmede et al., 2009). These features allow a systemic delivery of mesoangioblasts, thus overcoming migration-related problems described for other stem cell populations. In the recent years various tools were investigated to improve mesoangioblast migration toward damaged muscle and their local differentiation to optimize future cell therapy protocols for muscular dystrophies (Tedesco et al., 2010).
Macrophages are important to recruit and locally activate mesoangioblasts, committing them to myogenic differentiation. Conversely, we have observed that mesoangioblasts regulate gene expression of in vitro bone marrow derived macrophages (Bosurgi et al., unpublished results). Genes associated to macrophage scavenger function, phagocytic activity, chemokines release, and response to cytokines, which are regulated at the expression level, are all targets of mesoangioblast action. Their regulation is selective, since several other genes associated to macrophage housekeeping functions, to the iron metabolism and to the redox control are unaffected. We are actively verifying the possibility that mesoangioblasts prime the macrophages function toward a regulatory activity and this finely tuned cross-talk regulates the outcome of tissue remodeling.
Conclusion
In summary, myogenic precursors derived from satellite cells and other muscle stem cells are the final effectors of muscle regeneration. Substantial evidence indicates that they need licensing by accessory cells, in particular by inflammatory cells such as macrophages. Other mesenchymal precursors expand when muscle regeneration fails, leading to the eventual fibrotic scar and fat replacement of the tissue. The latter event is again possibly dependent on the inflammatory environment of the tissue. The efforts of the next years are likely to break the code by which the immune response controls the regeneration of the skeletal muscle, thus leading to the development of effective targeted therapies for genetic defects of the tissue and for the most common causes of physiological muscle wasting and sarcopenia.
Conflict of Interest Statement
The authors declare that the research was conducted in the absence of any commercial or financial relationships that could be construed as a potential conflict of interest.
References
Abou-Khalil, R., Le Grand, F., Pallafacchina, G., Valable, S., Authier, F. J., Rudnicki, M. A., Gherardi, R. K., Germain, S., Chretien, F., Sotiropoulos, A., Lafuste, P., Montarras, D., and Chazaud, B. (2009). Autocrine and paracrine angiopoietin 1/Tie-2 signaling promotes muscle satellite cell self-renewal. Cell Stem Cell 5, 298–309.
Acharya, M., Mukhopadhyay, S., Paidassi, H., Jamil, T., Chow, C., Kissler, S., Stuart, L. M., Hynes, R. O., and Lacy-Hulbert, A. (2010). αv Integrin expression by DCs is required for Th17 cell differentiation and development of experimental autoimmune encephalomyelitis in mice. J. Clin. Invest. 120, 4445–4452.
Arnold, L., Henry, A., Poron, F., Baba-Amer, Y., Van Rooijen, N., Plonquet, A., Gherardi, R. K., and Chazaud, B. (2007). Inflammatory monocytes recruited after skeletal muscle injury switch into antiinflammatory macrophages to support myogenesis. J. Exp. Med. 204, 1057–1069.
Bacci, M., Capobianco, A., Monno, A., Cottone, L., Di Puppo, F., Camisa, B., Mariani, M., Brignole, C., Ponzoni, M., Ferrari, S., Panina-Bordignon, P., Manfredi, A. A., and Rovere-Querini, P. (2009). Macrophages are alternatively activated in patients with endometriosis and required for growth and vascularization of lesions in a mouse model of disease. Am. J. Pathol. 175, 547–556.
Barrera, P., Blom, A., Van Lent, P. L., Van Bloois, L., Beijnen, J. H., Van Rooijen, N., De Waal Malefijt, M. C., Van De Putte, L. B., Storm, G., and Van Den Berg, W. B. (2000). Synovial macrophage depletion with clodronate-containing liposomes in rheumatoid arthritis. Arthritis Rheum. 43, 1951–1959.
Bianchi, M. E. (2007). DAMPs, PAMPs and alarmins: all we need to know about danger. J. Leukoc. Biol. 81, 1–5.
Biswas, S. K., and Mantovani, A. (2010). Macrophage plasticity and interaction with lymphocyte subsets: cancer as a paradigm. Nat. Immunol. 11, 889–896.
Blanpain, C., and Fuchs, E. (2009). Epidermal homeostasis: a balancing act of stem cells in the skin. Nat. Rev. Mol. Cell Biol. 10, 207–217.
Bondanza, A., Zimmermann, V. S., Dell’antonio, G., Cin, E. D., Balestrieri, G., Tincani, A., Amoura, Z., Piette, J. C., Sabbadini, M. G., Rovere-Querini, P., and Manfredi, A. A. (2004). Requirement of dying cells and environmental adjuvants for the induction of autoimmunity. Arthritis Rheum. 50, 1549–1560.
Bondanza, A., Zimmermann, V. S., Dell’antonio, G., Dal Cin, E., Capobianco, A., Sabbadini, M. G., Manfredi, A. A., and Rovere-Querini, P. (2003). Cutting edge: dissociation between autoimmune response and clinical disease after vaccination with dendritic cells. J. Immunol. 170, 24–27.
Brancato, S. K., and Albina, J. E. (2011). Wound macrophages as key regulators of repair: origin, phenotype, and function. Am. J. Pathol. 178, 19–25.
Brereton, C. F., and Blander, J. M. (2011). The unexpected link between infection-induced apoptosis and a TH17 immune response. J. Leukoc. Biol. 89, 565–576.
Brigitte, M., Schilte, C., Plonquet, A., Baba-Amer, Y., Henri, A., Charlier, C., Tajbakhsh, S., Albert, M., Gherardi, R. K., and Chretien, F. (2010). Muscle resident macrophages control the immune cell reaction in a mouse model of notexin-induced myoinjury. Arthritis Rheum. 62, 268–279.
Brunelli, S., and Rovere-Querini, P. (2008). The immune system and the repair of skeletal muscle. Pharmacol. Res. 58, 117–121.
Cairo, G., Recalcati, S., Mantovani, A., and Locati, M. (2011). Iron trafficking and metabolism in macrophages: contribution to the polarized phenotype. Trends Immunol. 32, 241–247.
Capobianco, A., Cottone, L., Monno, A., Ferrari, S., Panina-Bordignon, P., Manfredi, A. A., and Rovere-Querini, P. (2010). Innate immune cells: gatekeepers of endometriotic lesions growth and vascularization. J. Endometriosis 2, 55–62.
Capobianco, A., Monno, A., Cottone, L., Venneri, M., Biziato, D., Di Puppo, F., Ferrari, S., De Palma, M., Manfredi, A. A., and Rovere-Querini, P. (2011). Pro-angiogenic Tie2+ macrophages infiltrate human and murine endometriotic lesions and dictate their growth in a mouse model of the disease. Am. J. Pathol. 179, 2651–2659.
Carta, S., Castellani, P., Delfino, L., Tassi, S., Vene, R., and Rubartelli, A. (2009). DAMPs and inflammatory processes: the role of redox in the different outcomes. J. Leukoc. Biol. 86, 549–555.
Castiglioni, A., Canti, V., Rovere-Querini, P., and Manfredi, A. A. (2011). High-mobility group box 1 (HMGB1) as a master regulator of innate immunity. Cell Tissue Res. 343, 189–199.
Charge, S. B., and Rudnicki, M. A. (2004). Cellular and molecular regulation of muscle regeneration. Physiol. Rev. 84, 209–238.
Chazaud, B., Brigitte, M., Yacoub-Youssef, H., Arnold, L., Gherardi, R., Sonnet, C., Lafuste, P., and Chretien, F. (2009). Dual and beneficial roles of macrophages during skeletal muscle regeneration. Exerc. Sport Sci. Rev. 37, 18–22.
Chazaud, B., Sonnet, C., Lafuste, P., Bassez, G., Rimaniol, A. C., Poron, F., Authier, F. J., Dreyfus, P. A., and Gherardi, R. K. (2003). Satellite cells attract monocytes and use macrophages as a support to escape apoptosis and enhance muscle growth. J. Cell Biol. 163, 1133–1143.
Corna, G., Campana, L., Pignatti, E., Castiglioni, A., Tagliafico, E., Bosurgi, L., Campanella, A., Brunelli, S., Manfredi, A., Apostoli, P., Silvestri, L., Camaschella, C., and Rovere-Querini, P. (2010). Polarization dictates iron handling by inflammatory and alternatively activated macrophages. Haematologica 95, 1814–1822.
Dalakas, M. C. (2002). Muscle biopsy findings in inflammatory myopathies. Rheum. Dis. Clin. North Am. 28, 779–798.
Daley, J. M., Brancato, S. K., Thomay, A. A., Reichner, J. S., and Albina, J. E. (2010). The phenotype of murine wound macrophages. J. Leukoc. Biol. 87, 59–67.
Darrah, P. A., Patel, D. T., De Luca, P. M., Lindsay, R. W., Davey, D. F., Flynn, B. J., Hoff, S. T., Andersen, P., Reed, S. G., Morris, S. L., Roederer, M., and Seder, R. A. (2007). Multifunctional TH1 cells define a correlate of vaccine-mediated protection against Leishmania major. Nat. Med. 13, 843–850.
David, S., and Kroner, A. (2011). Repertoire of microglial and macrophage responses after spinal cord injury. Nat. Rev. Neurosci. 12, 388–399.
De Angelis, L., Berghella, L., Coletta, M., Lattanzi, L., Zanchi, M., Cusella-De Angelis, M. G., Ponzetto, C., and Cossu, G. (1999). Skeletal myogenic progenitors originating from embryonic dorsal aorta coexpress endothelial and myogenic markers and contribute to postnatal muscle growth and regeneration. J. Cell Biol. 147, 869–878.
Dellavalle, A., Sampaolesi, M., Tonlorenzi, R., Tagliafico, E., Sacchetti, B., Perani, L., Innocenzi, A., Galvez, B. G., Messina, G., Morosetti, R., Li, S., Belicchi, M., Peretti, G., Chamberlain, J. S., Wright, W. E., Torrente, Y., Ferrari, S., Bianco, P., and Cossu, G. (2007). Pericytes of human skeletal muscle are myogenic precursors distinct from satellite cells. Nat. Cell Biol. 9, 255–267.
Discher, D. E., Mooney, D. J., and Zandstra, P. W. (2009). Growth factors, matrices, and forces combine and control stem cells. Science 324, 1673–1677.
Djouad, F., Charbonnier, L. M., Bouffi, C., Louis-Plence, P., Bony, C., Apparailly, F., Cantos, C., Jorgensen, C., and Noel, D. (2007). Mesenchymal stem cells inhibit the differentiation of dendritic cells through an interleukin-6-dependent mechanism. Stem Cells 25, 2025–2032.
Dumont, N., Bouchard, P., and Frenette, J. (2008). Neutrophil-induced skeletal muscle damage: a calculated and controlled response following hindlimb unloading and reloading. Am. J. Physiol. Regul. Integr. Comp. Physiol. 295, R1831–R1838.
Dumont, N., and Frenette, J. (2010). Macrophages protect against muscle atrophy and promote muscle recovery in vivo and in vitro: a mechanism partly dependent on the insulin-like growth factor-1 signaling molecule. Am. J. Pathol. 176, 2228–2235.
Ehninger, A., and Trumpp, A. (2011). The bone marrow stem cell niche grows up: mesenchymal stem cells and macrophages move in. J. Exp. Med. 208, 421–428.
Elliott, M. R., and Ravichandran, K. S. (2010). Clearance of apoptotic cells: implications in health and disease. J. Cell Biol. 189, 1059–1070.
Fielding, R. A., Manfredi, T. J., Ding, W., Fiatarone, M. A., Evans, W. J., and Cannon, J. G. (1993). Acute phase response in exercise. III. Neutrophil and IL-1 beta accumulation in skeletal muscle. Am. J. Physiol. 265, R166–R172.
Gilbert, P. M., Havenstrite, K. L., Magnusson, K. E., Sacco, A., Leonardi, N. A., Kraft, P., Nguyen, N. K., Thrun, S., Lutolf, M. P., and Blau, H. M. (2010). Substrate elasticity regulates skeletal muscle stem cell self-renewal in culture. Science 329, 1078–1081.
Godbout, C., Bilodeau, R., Van Rooijen, N., Bouchard, P., and Frenette, J. (2010). Transient neutropenia increases macrophage accumulation and cell proliferation but does not improve repair following intratendinous rupture of Achilles tendon. J. Orthop. Res. 28, 1084–1091.
Gordon, S., and Martinez, F. O. (2010). Alternative activation of macrophages: mechanism and functions. Immunity 32, 593–604.
Groh, M. E., Maitra, B., Szekely, E., and Koc, O. N. (2005). Human mesenchymal stem cells require monocyte-mediated activation to suppress alloreactive T cells. Exp. Hematol. 33, 928–934.
Gurumurthy, S., Xie, S. Z., Alagesan, B., Kim, J., Yusuf, R. Z., Saez, B., Tzatsos, A., Ozsolak, F., Milos, P., Ferrari, F., Park, P. J., Shirihai, O. S., Scadden, D. T., and Bardeesy, N. (2010). The Lkb1 metabolic sensor maintains haematopoietic stem cell survival. Nature 468, 659–663.
Han, R., Frett, E. M., Levy, J. R., Rader, E. P., Lueck, J. D., Bansal, D., Moore, S. A., Ng, R., Beltran-Valero De Bernabe, D., Faulkner, J. A., and Campbell, K. P. (2010). Genetic ablation of complement C3 attenuates muscle pathology in dysferlin-deficient mice. J. Clin. Invest. 120, 4366–4374.
Hara, M., Yuasa, S., Shimoji, K., Onizuka, T., Hayashiji, N., Ohno, Y., Arai, T., Hattori, F., Kaneda, R., Kimura, K., Makino, S., Sano, M., and Fukuda, K. (2011). G-CSF influences mouse skeletal muscle development and regeneration by stimulating myoblast proliferation. J. Exp. Med. 208, 715–727.
Harel-Adar, T., Ben Mordechai, T., Amsalem, Y., Feinberg, M. S., Leor, J., and Cohen, S. (2011). Modulation of cardiac macrophages by phosphatidylserine-presenting liposomes improves infarct repair. Proc. Natl. Acad. Sci. U.S.A. 108, 1827–1832.
Holterman, C. E., and Rudnicki, M. A. (2005). Molecular regulation of satellite cell function. Semin. Cell Dev. Biol. 16, 575–584.
Huynh, M. L., Fadok, V. A., and Henson, P. M. (2002). Phosphatidylserine-dependent ingestion of apoptotic cells promotes TGF-beta1 secretion and the resolution of inflammation. J. Clin. Invest. 109, 41–50.
Jaeschke, H. (2011). Reactive oxygen and mechanisms of inflammatory liver injury: present concepts. J. Gastroenterol. Hepatol. 26(Suppl. 1), 173–179.
Janeway, C. A. Jr. (1992). The immune system evolved to discriminate infectious nonself from noninfectious self. Immunol. Today 13, 11–16.
Joe, A. W., Yi, L., Natarajan, A., Le Grand, F., So, L., Wang, J., Rudnicki, M. A., and Rossi, F. M. (2010). Muscle injury activates resident fibro/adipogenic progenitors that facilitate myogenesis. Nat. Cell Biol. 12, 153–163.
Kang, J. S., and Krauss, R. S. (2010). Muscle stem cells in developmental and regenerative myogenesis. Curr. Opin. Clin. Nutr. Metab. Care 13, 243–248.
Kim, J., and Hematti, P. (2009). Mesenchymal stem cell-educated macrophages: a novel type of alternatively activated macrophages. Exp. Hematol. 37, 1445–1453.
Krampera, M., Cosmi, L., Angeli, R., Pasini, A., Liotta, F., Andreini, A., Santarlasci, V., Mazzinghi, B., Pizzolo, G., Vinante, F., Romagnani, P., Maggi, E., Romagnani, S., and Annunziato, F. (2006). Role for interferon-gamma in the immunomodulatory activity of human bone marrow mesenchymal stem cells. Stem Cells 24, 386–398.
Kuang, S., Gillespie, M. A., and Rudnicki, M. A. (2008). Niche regulation of muscle satellite cell self-renewal and differentiation. Cell Stem Cell 2, 22–31.
Liu, G., Ma, H., Qiu, L., Li, L., Cao, Y., Ma, J., and Zhao, Y. (2011a). Phenotypic and functional switch of macrophages induced by regulatory CD4+CD25+ T cells in mice. Immunol. Cell Biol. 89, 130–142.
Liu, Y., Chen, G. Y., and Zheng, P. (2011b). Sialoside-based pattern recognitions discriminating infections from tissue injuries. Curr. Opin. Immunol. 23, 41–45.
Lolmede, K., Campana, L., Vezzoli, M., Bosurgi, L., Tonlorenzi, R., Clementi, E., Bianchi, M. E., Cossu, G., Manfredi, A. A., Brunelli, S., and Rovere-Querini, P. (2009). Inflammatory and alternatively activated human macrophages attract vessel-associated stem cells, relying on separate HMGB1- and MMP-9-dependent pathways. J. Leukoc. Biol. 85, 779–787.
London, A., Itskovich, E., Benhar, I., Kalchenko, V., Mack, M., Jung, S., and Schwartz, M. (2011). Neuroprotection and progenitor cell renewal in the injured adult murine retina requires healing monocyte-derived macrophages. J. Exp. Med. 208, 23–39.
Lotze, M. T., Zeh, H. J., Rubartelli, A., Sparvero, L. J., Amoscato, A. A., Washburn, N. R., Devera, M. E., Liang, X., Tor, M., and Billiar, T. (2007). The grateful dead: damage-associated molecular pattern molecules and reduction/oxidation regulate immunity. Immunol. Rev. 220, 60–81.
Lu, H., Huang, D., Ransohoff, R. M., and Zhou, L. (2011a). Acute skeletal muscle injury: CCL2 expression by both monocytes and injured muscle is required for repair. FASEB J. 25, 3344–3355.
Lu, H., Huang, D., Saederup, N., Charo, I. F., Ransohoff, R. M., and Zhou, L. (2011b). Macrophages recruited via CCR2 produce insulin-like growth factor-1 to repair acute skeletal muscle injury. FASEB J. 25, 358–369.
Manfredi, A. A., Capobianco, A., Bianchi, M. E., and Rovere-Querini, P. (2009). Regulation of dendritic- and T-cell fate by injury-associated endogenous signals. Crit. Rev. Immunol. 29, 69–86.
Manfredi, A. A., and Rovere-Querini, P. (2010). The mitochondrion –a Trojan horse that kicks off inflammation? N. Engl. J. Med. 362, 2132–2134.
Manfredi, A. A., Rovere-Querini, P., Bottazzi, B., Garlanda, C., and Mantovani, A. (2008). Pentraxins, humoral innate immunity and tissue injury. Curr. Opin. Immunol. 20, 538–544.
Manfredi, A. A., Rovere-Querini, P., and Maugeri, N. (2010). Dangerous connections: neutrophils and the phagocytic clearance of activated platelets. Curr. Opin. Hematol. 17, 3–8.
Mantovani, A., and Sica, A. (2010). Macrophages, innate immunity and cancer: balance, tolerance, and diversity. Curr. Opin. Immunol. 22, 231–237.
Mantovani, A., Sica, A., Allavena, P., Garlanda, C., and Locati, M. (2009). Tumor-associated macrophages and the related myeloid-derived suppressor cells as a paradigm of the diversity of macrophage activation. Hum. Immunol. 70, 325–330.
Mantovani, A., Sica, A., Sozzani, S., Allavena, P., Vecchi, A., and Locati, M. (2004). The chemokine system in diverse forms of macrophage activation and polarization. Trends Immunol. 25, 677–686.
Maroso, M., Balosso, S., Ravizza, T., Liu, J., Aronica, E., Iyer, A. M., Rossetti, C., Molteni, M., Casalgrandi, M., Manfredi, A. A., Bianchi, M. E., and Vezzani, A. (2010). Toll-like receptor 4 and high-mobility group box-1 are involved in ictogenesis and can be targeted to reduce seizures. Nat. Med. 16, 413–419.
Martinez, C. O., Mchale, M. J., Wells, J. T., Ochoa, O., Michalek, J. E., Mcmanus, L. M., and Shireman, P. K. (2010). Regulation of skeletal muscle regeneration by CCR2-activating chemokines is directly related to macrophage recruitment. Am. J. Physiol. Regul. Integr. Comp. Physiol. 299, R832–R842.
Martinon, F., Chen, X., Lee, A. H., and Glimcher, L. H. (2010). TLR activation of the transcription factor XBP1 regulates innate immune responses in macrophages. Nat. Immunol. 11, 411–418.
Maugeri, N., Rovere-Querini, P., Baldini, M., Sabbadini, M. G., and Manfredi, A. A. (2009). Translational mini-review series on immunology of vascular disease: mechanisms of vascular inflammation and remodelling in systemic vasculitis. Clin. Exp. Immunol. 156, 395–404.
Mcgaha, T. L., Chen, Y., Ravishankar, B., Van Rooijen, N., and Karlsson, M. C. (2011). Marginal zone macrophages suppress innate and adaptive immunity to apoptotic cells in the spleen. Blood 117, 5403–5412.
Mclennan, I. S. (1996). Degenerating and regenerating skeletal muscles contain several subpopulations of macrophages with distinct spatial and temporal distributions. J. Anat. 188, 17–28.
Mounier, R., Chretien, F., and Chazaud, B. (2011). Blood vessels and the satellite cell niche. Curr. Top. Dev. Biol. 96, 121–138.
Munoz, L. E., Lauber, K., Schiller, M., Manfredi, A. A., and Herrmann, M. (2010). The role of defective clearance of apoptotic cells in systemic autoimmunity. Nat. Rev. Rheumatol. 6, 280–289.
Musaro, A., Mccullagh, K., Paul, A., Houghton, L., Dobrowolny, G., Molinaro, M., Barton, E. R., Sweeney, H. L., and Rosenthal, N. (2001). Localized Igf-1 transgene expression sustains hypertrophy and regeneration in senescent skeletal muscle. Nat. Genet. 27, 195–200.
Mylonas, K. J., Nair, M. G., Prieto-Lafuente, L., Paape, D., and Allen, J. E. (2009). Alternatively activated macrophages elicited by helminth infection can be reprogrammed to enable microbial killing. J. Immunol. 182, 3084–3094.
Nau, G. J., Richmond, J. F., Schlesinger, A., Jennings, E. G., Lander, E. S., and Young, R. A. (2002). Human macrophage activation programs induced by bacterial pathogens. Proc. Natl. Acad. Sci. U.S.A. 99, 1503–1508.
Nguyen, H. X., Lusis, A. J., and Tidball, J. G. (2005). Null mutation of myeloperoxidase in mice prevents mechanical activation of neutrophil lysis of muscle cell membranes in vitro and in vivo. J. Physiol. (Lond.) 565, 403–413.
O’brien, J., Lyons, T., Monks, J., Lucia, M. S., Wilson, R. S., Hines, L., Man, Y. G., Borges, V., and Schedin, P. (2010). Alternatively activated macrophages and collagen remodeling characterize the postpartum involuting mammary gland across species. Am. J. Pathol. 176, 1241–1255.
Ohtaki, H., Ylostalo, J. H., Foraker, J. E., Robinson, A. P., Reger, R. L., Shioda, S., and Prockop, D. J. (2008). Stem/progenitor cells from bone marrow decrease neuronal death in global ischemia by modulation of inflammatory/immune responses. Proc. Natl. Acad. Sci. U.S.A. 105, 14638–14643.
Palm, N. W., and Medzhitov, R. (2009). Pattern recognition receptors and control of adaptive immunity. Immunol. Rev. 227, 221–233.
Paulsen, G., Crameri, R., Benestad, H. B., Fjeld, J. G., Morkrid, L., Hallen, J., and Raastad, T. (2010). Time course of leukocyte accumulation in human muscle after eccentric exercise. Med. Sci. Sports Exerc. 42, 75–85.
Paylor, B., Natarajan, A., Zhang, R. H., and Rossi, F. (2011). Nonmyogenic cells in skeletal muscle regeneration. Curr. Top. Dev. Biol. 96, 139–165.
Pelosi, L., Giacinti, C., Nardis, C., Borsellino, G., Rizzuto, E., Nicoletti, C., Wannenes, F., Battistini, L., Rosenthal, N., Molinaro, M., and Musaro, A. (2007). Local expression of IGF-1 accelerates muscle regeneration by rapidly modulating inflammatory cytokines and chemokines. FASEB J. 21, 1393–1402.
Peng, Y., and Elkon, K. B. (2011). Autoimmunity in MFG-E8-deficient mice is associated with altered trafficking and enhanced cross-presentation of apoptotic cell antigens. J. Clin. Invest. 121, 2221–2241.
Pluchino, S., Zanotti, L., Brambilla, E., Rovere-Querini, P., Capobianco, A., Alfaro-Cervello, C., Salani, G., Cossetti, C., Borsellino, G., Battistini, L., Ponzoni, M., Doglioni, C., Garcia-Verdugo, J. M., Comi, G., Manfredi, A. A., and Martino, G. (2009). Immune regulatory neural stem/precursor cells protect from central nervous system autoimmunity by restraining dendritic cell function. PLoS ONE 4, e5959. doi:10.1371/journal.pone.0005959
Qian, B. Z., and Pollard, J. W. (2010). Macrophage diversity enhances tumor progression and metastasis. Cell 141, 39–51.
Robertson, T. A., Maley, M. A., Grounds, M. D., and Papadimitriou, J. M. (1993). The role of macrophages in skeletal muscle regeneration with particular reference to chemotaxis. Exp. Cell Res. 207, 321–331.
Rock, K. L., Lai, J. J., and Kono, H. (2011). Innate and adaptive immune responses to cell death. Immunol. Rev. 243, 191–205.
Rock, K. L., Latz, E., Ontiveros, F., and Kono, H. (2010). The sterile inflammatory response. Annu. Rev. Immunol. 28, 321–342.
Rosenthal, S. M., and Cheng, Z. Q. (1995). Opposing early and late effects of insulin-like growth factor I on differentiation and the cell cycle regulatory retinoblastoma protein in skeletal myoblasts. Proc. Natl. Acad. Sci. U.S.A. 92, 10307–10311.
Rubartelli, A., and Lotze, M. T. (2007). Inside, outside, upside down: damage-associated molecular-pattern molecules (DAMPs) and redox. Trends Immunol. 28, 429–436.
Rubartelli, A., and Sitia, R. (2009). Stress as an intercellular signal: the emergence of stress-associated molecular patterns (SAMP). Antioxid. Redox Signal. 11, 2621–2629.
Ruffell, D., Mourkioti, F., Gambardella, A., Kirstetter, P., Lopez, R. G., Rosenthal, N., and Nerlov, C. (2009). A CREB-C/EBP beta cascade induces M2 macrophage-specific gene expression and promotes muscle injury repair. Proc. Natl. Acad. Sci. U.S.A. 106, 17475–17480.
Sacco, A., Doyonnas, R., Kraft, P., Vitorovic, S., and Blau, H. M. (2008). Self-renewal and expansion of single transplanted muscle stem cells. Nature 456, 502–506.
Sacco, A., Mourkioti, F., Tran, R., Choi, J., Llewellyn, M., Kraft, P., Shkreli, M., Delp, S., Pomerantz, J. H., Artandi, S. E., and Blau, H. M. (2010). Short telomeres and stem cell exhaustion model Duchenne muscular dystrophy in mdx/mTR mice. Cell 143, 1059–1071.
Sampaolesi, M., Blot, S., D’antona, G., Granger, N., Tonlorenzi, R., Innocenzi, A., Mognol, P., Thibaud, J. L., Galvez, B. G., Barthelemy, I., Perani, L., Mantero, S., Guttinger, M., Pansarasa, O., Rinaldi, C., Cusella De Angelis, M. G., Torrente, Y., Bordignon, C., Bottinelli, R., and Cossu, G. (2006). Mesoangioblast stem cells ameliorate muscle function in dystrophic dogs. Nature 444, 574–579.
Sampaolesi, M., Torrente, Y., Innocenzi, A., Tonlorenzi, R., D’antona, G., Pellegrino, M. A., Barresi, R., Bresolin, N., De Angelis, M. G., Campbell, K. P., Bottinelli, R., and Cossu, G. (2003). Cell therapy of alpha-sarcoglycan null dystrophic mice through intra-arterial delivery of mesoangioblasts. Science 301, 487–492.
Sandri, M., Sandri, C., Gilbert, A., Skurk, C., Calabria, E., Picard, A., Walsh, K., Schiaffino, S., Lecker, S. H., and Goldberg, A. L. (2004). Foxo transcription factors induce the atrophy-related ubiquitin ligase atrogin-1 and cause skeletal muscle atrophy. Cell 117, 399–412.
Schwartz, M. (2010). “Tissue-repairing” blood-derived macrophages are essential for healing of the injured spinal cord: from skin-activated macrophages to infiltrating blood-derived cells? Brain Behav. Immun. 24, 1054–1057.
Schwartz, M., and Ziv, Y. (2008). Immunity to self and self-maintenance: a unified theory of brain pathologies. Trends Immunol. 29, 211–219.
Segawa, M., Fukada, S., Yamamoto, Y., Yahagi, H., Kanematsu, M., Sato, M., Ito, T., Uezumi, A., Hayashi, S., Miyagoe-Suzuki, Y., Takeda, S., Tsujikawa, K., and Yamamoto, H. (2008). Suppression of macrophage functions impairs skeletal muscle regeneration with severe fibrosis. Exp. Cell Res. 314, 3232–3244.
Shechter, R., London, A., Varol, C., Raposo, C., Cusimano, M., Yovel, G., Rolls, A., Mack, M., Pluchino, S., Martino, G., Jung, S., and Schwartz, M. (2009). Infiltrating blood-derived macrophages are vital cells playing an anti-inflammatory role in recovery from spinal cord injury in mice. PLoS Med. 6, e1000113. doi:10.1371/journal.pmed.1000113
Shireman, P. K., Contreras-Shannon, V., Ochoa, O., Karia, B. P., Michalek, J. E., and Mcmanus, L. M. (2007). MCP-1 deficiency causes altered inflammation with impaired skeletal muscle regeneration. J. Leukoc. Biol. 81, 775–785.
Sindrilaru, A., Peters, T., Wieschalka, S., Baican, C., Baican, A., Peter, H., Hainzl, A., Schatz, S., Qi, Y., Schlecht, A., Weiss, J. M., Wlaschek, M., Sunderkotter, C., and Scharffetter-Kochanek, K. (2011). An unrestrained proinflammatory M1 macrophage population induced by iron impairs wound healing in humans and mice. J. Clin. Invest. 121, 985–997.
Sotiropoulou, P. A., Perez, S. A., Gritzapis, A. D., Baxevanis, C. N., and Papamichail, M. (2006). Interactions between human mesenchymal stem cells and natural killer cells. Stem Cells 24, 74–85.
Spaggiari, G. M., Abdelrazik, H., Becchetti, F., and Moretta, L. (2009). MSCs inhibit monocyte-derived DC maturation and function by selectively interfering with the generation of immature DCs: central role of MSC-derived prostaglandin E2. Blood 113, 6576–6583.
Spaggiari, G. M., Capobianco, A., Becchetti, S., Mingari, M. C., and Moretta, L. (2006). Mesenchymal stem cell-natural killer cell interactions: evidence that activated NK cells are capable of killing MSCs, whereas MSCs can inhibit IL-2-induced NK-cell proliferation. Blood 107, 1484–1490.
Squadrito, M. L., and De Palma, M. (2011). Macrophage regulation of tumor angiogenesis: implications for cancer therapy. Mol. Aspects Med. 32, 123–145.
St Pierre, B. A., and Tidball, J. G. (1994). Differential response of macrophage subpopulations to soleus muscle reloading after rat hindlimb suspension. J. Appl. Physiol. 77, 290–297.
Stappenbeck, T. S., and Miyoshi, H. (2009). The role of stromal stem cells in tissue regeneration and wound repair. Science 324, 1666–1669.
Strle, K., Mccusker, R. H., Johnson, R. W., Zunich, S. M., Dantzer, R., and Kelley, K. W. (2008). Prototypical anti-inflammatory cytokine IL-10 prevents loss of IGF-I-induced myogenin protein expression caused by IL-1beta. Am. J. Physiol. Endocrinol. Metab. 294, E709–E718.
Strle, K., Mccusker, R. H., Tran, L., King, A., Johnson, R. W., Freund, G. G., Dantzer, R., and Kelley, K. W. (2007). Novel activity of an anti-inflammatory cytokine: IL-10 prevents TNFalpha-induced resistance to IGF-I in myoblasts. J. Neuroimmunol. 188, 48–55.
Summan, M., Warren, G. L., Mercer, R. R., Chapman, R., Hulderman, T., Van Rooijen, N., and Simeonova, P. P. (2006). Macrophages and skeletal muscle regeneration: a clodronate-containing liposome depletion study. Am. J. Physiol. Regul. Integr. Comp. Physiol. 290, R1488–R1495.
Sun, D., Martinez, C. O., Ochoa, O., Ruiz-Willhite, L., Bonilla, J. R., Centonze, V. E., Waite, L. L., Michalek, J. E., Mcmanus, L. M., and Shireman, P. K. (2009). Bone marrow-derived cell regulation of skeletal muscle regeneration. FASEB J. 23, 382–395.
Tedesco, F. S., Dellavalle, A., Diaz-Manera, J., Messina, G., and Cossu, G. (2010). Repairing skeletal muscle: regenerative potential of skeletal muscle stem cells. J. Clin. Invest. 120, 11–19.
Tidball, J. G. (2002). Interactions between muscle and the immune system during modified musculoskeletal loading. Clin. Orthop. Relat. Res. (Suppl. 403), S100–S109.
Tidball, J. G., and Villalta, S. A. (2010). Regulatory interactions between muscle and the immune system during muscle regeneration. Am. J. Physiol. Regul. Integr. Comp. Physiol. 298, R1173–R1187.
Tidball, J. G., and Wehling-Henricks, M. (2007). Macrophages promote muscle membrane repair and muscle fibre growth and regeneration during modified muscle loading in mice in vivo. J. Physiol. (Lond.) 578, 327–336.
Tiemessen, M. M., Jagger, A. L., Evans, H. G., Van Herwijnen, M. J., John, S., and Taams, L. S. (2007). CD4+CD25+Foxp3+ regulatory T cells induce alternative activation of human monocytes/macrophages. Proc. Natl. Acad. Sci. U.S.A. 104, 19446–19451.
Tothova, Z., and Gilliland, D. G. (2007). FoxO transcription factors and stem cell homeostasis: insights from the hematopoietic system. Cell Stem Cell 1, 140–152.
Uezumi, A., Fukada, S., Yamamoto, N., Takeda, S., and Tsuchida, K. (2010). Mesenchymal progenitors distinct from satellite cells contribute to ectopic fat cell formation in skeletal muscle. Nat. Cell Biol. 12, 143–152.
Urbonaviciute, V., Furnrohr, B. G., Meister, S., Munoz, L., Heyder, P., De Marchis, F., Bianchi, M. E., Kirschning, C., Wagner, H., Manfredi, A. A., Kalden, J. R., Schett, G., Rovere-Querini, P., Herrmann, M., and Voll, R. E. (2008). Induction of inflammatory and immune responses by HMGB1-nucleosome complexes: implications for the pathogenesis of SLE. J. Exp. Med. 205, 3007–3018.
Van Furth, R., and Cohn, Z. A. (1968). The origin and kinetics of mononuclear phagocytes. J. Exp. Med. 128, 415–435.
Vezzoli, M., Castellani, P., Campana, L., Corna, G., Bosurgi, L., Manfredi, A. A., Bianchi, M. E., Rubartelli, A., and Rovere-Querini, P. (2010). Redox remodeling: a candidate regulator of HMGB1 function in injured skeletal muscle. Ann. N. Y. Acad. Sci. 1209, 83–90.
Vezzoli, M., Castellani, P., Corna, G., Castiglioni, A., Bosurgi, L., Monno, A., Brunelli, S., Manfredi, A. A., Rubartelli, A., and Rovere-Querini, P. (2011). High-mobility group box 1 release and redox regulation accompany regeneration and remodeling of skeletal muscle. Antioxid. Redox Signal. 15, 2161–2174.
Vidal, B., Serrano, A. L., Tjwa, M., Suelves, M., Ardite, E., De Mori, R., Baeza-Raja, B., Martinez De Lagran, M., Lafuste, P., Ruiz-Bonilla, V., Jardi, M., Gherardi, R., Christov, C., Dierssen, M., Carmeliet, P., Degen, J. L., Dewerchin, M., and Munoz-Canoves, P. (2008). Fibrinogen drives dystrophic muscle fibrosis via a TGF beta/alternative macrophage activation pathway. Genes Dev. 22, 1747–1752.
Villalta, S. A., Nguyen, H. X., Deng, B., Gotoh, T., and Tidball, J. G. (2009). Shifts in macrophage phenotypes and macrophage competition for arginine metabolism affect the severity of muscle pathology in muscular dystrophy. Hum. Mol. Genet. 18, 482–496.
Villalta, S. A., Rinaldi, C., Deng, B., Liu, G., Fedor, B., and Tidball, J. G. (2010). Interleukin-10 reduces the pathology of mdx muscular dystrophy by deactivating M1 macrophages and modulating macrophage phenotype. Hum. Mol. Genet. 20, 790–805.
Warren, G. L., Hulderman, T., Mishra, D., Gao, X., Millecchia, L., O’farrell, L., Kuziel, W. A., and Simeonova, P. P. (2005). Chemokine receptor CCR2 involvement in skeletal muscle regeneration. FASEB J. 19, 413–415.
Wehling, M., Spencer, M. J., and Tidball, J. G. (2001). A nitric oxide synthase transgene ameliorates muscular dystrophy in mdx mice. J. Cell Biol. 155, 123–131.
Wiendl, H., Hohlfeld, R., and Kieseier, B. C. (2005). Immunobiology of muscle: advances in understanding an immunological microenvironment. Trends Immunol. 26, 373–380.
Wong, S. C., Puaux, A. L., Chittezhath, M., Shalova, I., Kajiji, T. S., Wang, X., Abastado, J. P., Lam, K. P., and Biswas, S. K. (2010). Macrophage polarization to a unique phenotype driven by B cells. Eur. J. Immunol. 40, 2296–2307.
Yang, H., Rivera, Z., Jube, S., Nasu, M., Bertino, P., Goparaju, C., Franzoso, G., Lotze, M. T., Krausz, T., Pass, H. I., Bianchi, M. E., and Carbone, M. (2010). Programmed necrosis induced by asbestos in human mesothelial cells causes high-mobility group box 1 protein release and resultant inflammation. Proc. Natl. Acad. Sci. U.S.A. 107, 12611–12616.
Zammit, P. S. (2008). All muscle satellite cells are equal, but are some more equal than others? J. Cell Sci. 121, 2975–2982.
Zhang, B., Liu, R., Shi, D., Liu, X., Chen, Y., Dou, X., Zhu, X., Lu, C., Liang, W., Liao, L., Zenke, M., and Zhao, R. C. (2009a). Mesenchymal stem cells induce mature dendritic cells into a novel Jagged-2-dependent regulatory dendritic cell population. Blood 113, 46–57.
Zhang, L., Ran, L., Garcia, G. E., Wang, X. H., Han, S., Du, J., and Mitch, W. E. (2009b). Chemokine CXCL16 regulates neutrophil and macrophage infiltration into injured muscle, promoting muscle regeneration. Am. J. Pathol. 175, 2518–2527.
Zhang, M., Xu, S., Han, Y., and Cao, X. (2011). Apoptotic cells attenuate fulminant hepatitis by priming Kupffer cells to produce interleukin-10 through membrane-bound TGF-beta. Hepatology 53, 306–316.
Zhang, Q., Raoof, M., Chen, Y., Sumi, Y., Sursal, T., Junger, W., Brohi, K., Itagaki, K., and Hauser, C. J. (2010a). Circulating mitochondrial DAMPs cause inflammatory responses to injury. Nature 464, 104–107.
Zhang, Q. Z., Su, W. R., Shi, S. H., Wilder-Smith, P., Xiang, A. P., Wong, A., Nguyen, A. L., Kwon, C. W., and Le, A. D. (2010b). Human gingiva-derived mesenchymal stem cells elicit polarization of m2 macrophages and enhance cutaneous wound healing. Stem Cells 28, 1856–1868.
Zhao, J., Brault, J. J., Schild, A., Cao, P., Sandri, M., Schiaffino, S., Lecker, S. H., and Goldberg, A. L. (2007). FoxO3 coordinately activates protein degradation by the autophagic/lysosomal and proteasomal pathways in atrophying muscle cells. Cell Metab. 6, 472–483.
Keywords: macrophages, skeletal muscle, innate immunity, wound healing, alternative activation
Citation: Bosurgi L, Manfredi AA and Rovere-Querini P (2011) Macrophages in injured skeletal muscle: a perpetuum mobile causing and limiting fibrosis, prompting or restricting resolution and regeneration. Front. Immun. 2:62. doi: 10.3389/fimmu.2011.00062
Received: 05 August 2011; Accepted: 28 October 2011;
Published online: 16 November 2011.
Edited by:
Heiko Mühl, University Hospital Goethe University, GermanyReviewed by:
Ralf J. Ludwig, University of Lübeck, GermanyAndreas Ludwig, RWTH Aachen University, Germany
Itamar Goren, J. W. Goethe University, Germany
Copyright: © 2011 Bosurgi, Manfredi and Rovere-Querini. This is an open-access article subject to a non-exclusive license between the authors and Frontiers Media SA, which permits use, distribution and reproduction in other forums, provided the original authors and source are credited and other Frontiers conditions are complied with.
*Correspondence: Patrizia Rovere-Querini, Unit of Innate Immunity and Tissue Remodelling, Division of Regenerative Medicine, Stem Cells and Gene Therapy, Istituto Scientifico San Raffaele, 20132 Milano, Italy. e-mail:cm92ZXJlLnBhdHJpemlhQGhzci5pdA==