- 1Research Institute of Women's Health, Eberhard Karls University, Tübingen, Germany
- 2Institute of Medical Genetics and Applied Genomics, Eberhard Karls University, Tübingen, Germany
Whilst scientific knowledge about SARS-CoV-2 and COVID-19 is rapidly increasing, much of the effects on pregnant women is still unknown. To accommodate pregnancy, the human endometrium must undergo a physiological transformation called decidualization. These changes encompass the remodeling of endometrial immune cells leading to immunotolerance of the semi-allogenic conceptus as well as defense against pathogens. The angiotensin converting enzyme 2 (ACE2) plays an important regulatory role in the renin-angiotensin-system (RAS) and has been shown to be protective against comorbidities known to worsen COVID-19 outcomes. Furthermore, ACE2 is also crucial for decidualization and thus for early gestation. An astounding gender difference has been found in COVID-19 with male patients presenting with more severe cases and higher mortality rates. This could be attributed to differences in sex chromosomes, hormone levels and behavior patterns. Despite profound changes in the female body during pregnancy, expectant mothers do not face worse outcomes compared with non-pregnant women. Whereas mother-to-child transmission through respiratory droplets during labor or in the postnatal period is known, another question of in utero transmission remains unanswered. Evidence of placental SARS-CoV-2 infection and expression of viral entry receptors at the maternal-fetal interface suggests the possibility of in utero transmission. SARS-CoV-2 can cause further harm through placental damage, maternal systemic inflammation, and hindered access to health care during the pandemic. More research on the effects of COVID-19 during early pregnancy as well as vaccination and treatment options for gravid patients is urgently needed.
Introduction
Since its emergence in December 2019 in Wuhan, China, the Severe Acute Respiratory Syndrome Coronavirus 2 (SARS-CoV-2) has infected over 250 million people and caused more than 4.9 million deaths worldwide (as of October 2021) (1, 2). The disease caused by SARS-CoV-2 is termed Coronavirus Disease 2019 (COVID-19) and was declared a global pandemic in March 2020 (3). Whilst scientific knowledge about this disease is rapidly increasing, much of its effects on pregnant women is still unknown.
Pregnancy is a unique physiological state during which the female body undergoes profound transformations. The immune system is altered during pregnancy, resulting in immunotolerance of the semi-allogenic conceptus as well as protection of both mother and fetus against pathogens (4). Research indicates that during pregnancy, expectant mothers are more susceptible to some infectious diseases, such as influenza or Ebola (5, 6).
The aim of this review is to illustrate what is known about COVID-19 and how it affects pregnancy. First, changes in the human endometrium enabling embryo implantation and pregnancy will be discussed – a process coined decidualization. Special emphasis will be put on the endometrial immune microenvironment, angiotensin-converting enzyme 2 (ACE2) and transmembrane serine protease 2 (TMPRSS2). What follows is a brief overview of SARS-CoV-2 and of COVID-19. The review will further describe the gender differences found in COVID-19 and offer possible explanations. Lastly, what is known about implications of COVID-19 infection during pregnancy will be reviewed, with particular focus on the possibility of vertical transmission of SARS-CoV-2.
The Human Endometrium and Decidualization
The human menstrual cycle is approximately 28 days long and can be divided into two phases: the follicular (proliferative) phase and the luteal (secretory) phase (7, 8). The start of each cycle is marked by the onset of menstruation (9). During the first phase, estrogen is produced by granulosa cells in the ovaries, which leads to thickening of the endometrium (7). This thickening is the result of proliferating epithelial and stromal cells, as well as angiogenesis (7, 10). Ovulation marks the start of the second phase, when the corpus luteum produces progesterone, further preparing the endometrium for the possibility of embryo implantation and pregnancy; a process known as decidualization (11, 12). In the case of no pregnancy, the corpus luteum deteriorates leading to a drop in progesterone levels, vasoconstriction in the endometrium with hypoxia and desquamation of the stratum functionalis (11–13).
The process of decidualization involves the differentiation of endometrial stromal cells, which are of mesenchymal origin and resemble fibroblasts, into decidual cells, similar to epithelial cells (Figure 1) (14–16). During this mesenchymal-epithelial transition, the cells become larger and rounder with an expansion of the rough endoplasmic reticulum and the Golgi apparatus (11, 14). There is an increase in the number of nucleoli and an accumulation of lipid and glycogen droplets within the cytoplasm (11, 16). It was also shown that polyploidy is common among decidual cells, which might limit their lifespan but could benefit the growth of the embryo due to increased protein synthesis (17, 18). Decidual cells produce large quantities of prolactin and insulin-like growth factor binding protein-1, among others, which can also be used as bona fide markers for decidualization (11, 14).
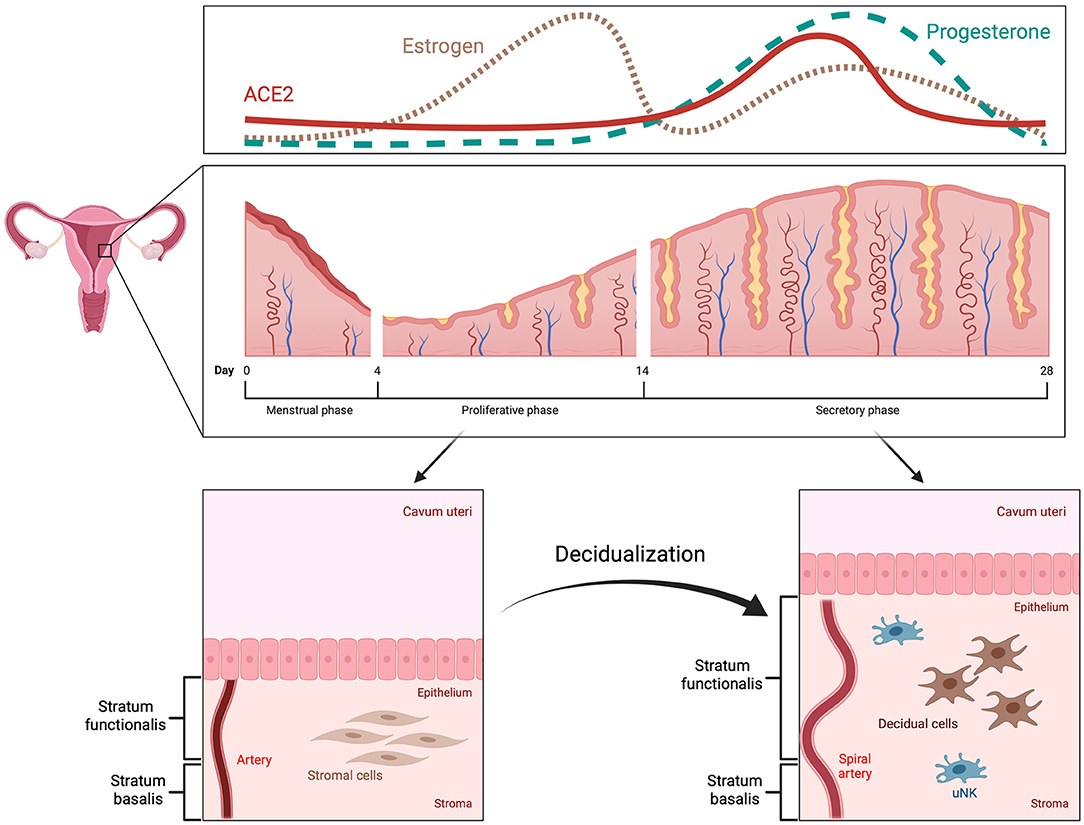
Figure 1. Menstrual cycle and decidualization. The human menstrual cycle repeats itself in 28-day intervals. The start is marked by the onset of menstruation. Subsequently, the endometrium enters the proliferative phase, during which it increases in thickness as a response to high estrogen levels (pink, dotted line). In the secretory phase, decidualization occurs with remodeling of spiral arteries, mesenchymal-epithelial transition of stromal cells and alterations in the endometrial immune system, e.g., increase in uterine natural killer cells. These changes are triggered and regulated by progesterone (green, dashed line) and mainly take place in the upper part of the endometrium, the stratum functionalis, which is also shed during menstruation. ACE2 expression is increased by decidualization in the secretory phase (red, solid line).
The human endometrium is subject to cyclic transformations to provide an optimal environment for embryo implantation, however, the window of implantation is brief (19). The uterus is only receptive to a blastocyst during the limited duration of about 4 days, approximately 6 to 10 days after ovulation (20, 21). Not only does decidualization influence the timing of implantation but it also controls the extent of invasion by the embryo (22). Some studies even suggest that decidual cells are not passively invaded by the trophoblast but actively encapsulate the embryo (23–25). Moreover, the endometrium has the capability to sense the quality of the conceptus and makes a distinction between healthy and impaired embryos (26, 27). Therefore, the decidua promotes implantation of high-quality embryos while rejecting developmentally impaired ones through modulation of gene expression (26–28). Defective decidualization can lead to a plethora of pregnancy complications such as preeclampsia, preterm birth or even recurrent pregnancy loss, highlighting the importance of adequate decidualization in early pregnancy (15, 29).
The Endometrial Immune Microenvironment
Changes in morphology and function are not solely limited to stromal cells. Remodeling of the extracellular matrix as well as cell-cell interactions play a crucial role in decidualization (30, 31). Since pregnancy requires a well-calibrated balance between immunological responsiveness and tolerance, immune cells are another relevant component of the decidua (32, 33). During early pregnancy, up to 40% of all cells within the decidual tissue are leukocytes, such as macrophages, T and B cells and, most prominently, uterine natural killer cells (uNK) (34). The latter sees an increase in number during decidualization and is most abundant in the vicinity of spiral arteries, endometrial glands and at the maternal-fetal interface (7, 35). Although their function is not completely clear, studies suggest that uNK are involved in remodeling of spiral arteries, clearance of senescent decidual cells, regulating maternal immune tolerance and defense against pathogens (15, 35, 36).
The maternal immune system is modulated during pregnancy, which is particularly meaningful when trying to understand the effects of COVID-19 on pregnancy and vice versa. The decidualized cells play an important role in providing immunotolerance toward the allogenic embryo by modulating the spectrum of immune cells at the maternal-fetal interface (32, 37). While there are plenty uNK cells (70% of total leukocytes) and macrophages (20–25%) in the decidual tissue, dendritic cells and B and T lymphocytes are rare (11, 32, 38). It has been shown that dendritic cells, which regularly trigger T cell reaction, are entrapped in the tissue through decidualization and that their density throughout the decidua is reduced (39). Due to this entrapment, the dendritic cells are ineffective in facilitating T cell activation, thus, lowering the chance of immunological attack on the fetus. Furthermore, decidual cells inhibit T cell proliferation, suppress inflammation and prompt apoptosis of activated T cells via the expression of Galectin-1, indoleamine-2,3-dioxygenase and FAS-ligand (40–42).
In summary, decidualization is part of the cyclic morphological alterations in the endometrium. This process is of utmost importance for embryo implantation and early pregnancy. Decidualization mainly encompasses modifications in endometrial stromal cells and is regulated predominantly by progesterone. Further, changes also occur in endometrial immune cells, and uNK cells are of particular interest, as they play an important role in endometrial remodeling during decidualization as well as immune tolerance and defense.
ACE2 – Physiology and Role in Decidualization
The angiotensin-converting enzyme 2 (ACE2) is a typical zinc metallopeptidase that plays an important role in the renin-angiotensin system (RAS) (43). It is an integral membrane glycoprotein, consisting of 805 amino acids and containing a single catalytic domain (44). Major functions of ACE2 include converting angiotensin (Ang) I into Ang 1-9 and Ang II into Ang 1-7 (45, 46). ACE typically converts Ang I into Ang II, causing vasoconstriction, leading to inflammation and fibrosis, ACE2 can be seen as a counterbalance to ACE (44). Thus, ACE2 is a negative regulator of RAS and therefore crucial in regulating blood pressure, fluid and electrolyte balance (Figure 2) (47).
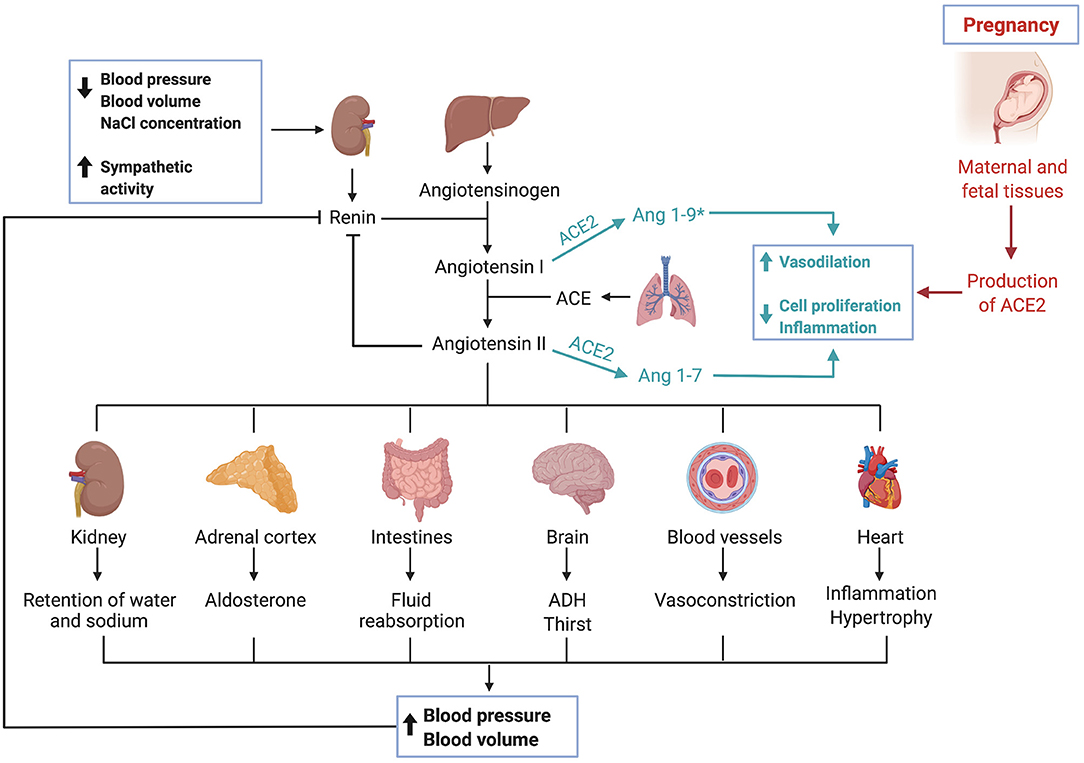
Figure 2. The renin-angiotensin-system and ACE2. Triggered by low blood pressure, low blood volume and low sodium levels as well as sympathetic activity, the kidney secretes renin. Renin, a protease, cleaves angiotensinogen, secreted by the liver, into angiotensin I. Angiotensin I is then converted by the angiotensin-converting enzyme, which can be found in membranes of endothelial cells most abundantly in the lungs and kidneys, into angiotensin II. Various effects are caused by angiotensin II, which ultimately result in an increase of blood pressure and volume. The angiotensin-converting enzyme 2 works as a counterbalance to ACE by cleaving angiotensin I and angiotensin II into Ang 1–9* and Ang 1–7, respectively. (*Ang1–9 is postulated to exert similar effects as Ang1–7, though current data is limited and needs further validation). Activation of this pathway leads to vasodilation, inhibits cell proliferation and has anti-inflammatory effects. During pregnancy, maternal and fetal tissues contribute to the production of ACE2, leading to systemic vasodilation.
Despite being expressed ubiquitously in the human body, some tissues contain remarkably high amounts of ACE2 including the kidneys, heart, lungs, testes and intestines as well as endothelial and vascular smooth muscle cells (43, 48, 49). ACE2-expressing tissues are potential targets for SARS-CoV-2 (50).
Notably, ACE2 has been shown to be protective against heart failure, hypertension, diabetes, renal dysfunction and pulmonary diseases (51–55). These are also comorbidities that have been identified to worsen the outcome of COVID-19 patients, which might be linked to a dysregulation of RAS (43, 56, 57). After facilitating the entry of SARS-CoV-2 into the host cell, ACE2 expression is downregulated (58). Loss of ACE2 is initiated during virus infection since the SARS-CoV-2-ACE2-complex is internalized through endocytosis (59, 60). This depletion of ACE2 leads to a dysregulation of RAS, further aggravating harmful effects of COVID-19, such as acute respiratory distress syndrome (ARDS) and endothelial dysfunction (61–63).
A controversial debate over the continued usage of angiotensin II receptor blockers and ACE inhibitors among COVID-19 patients with preexisting hypertension arose after the ACE2 receptor became known as the entry factor for SARS-CoV-2 (64). Since these drugs were thought to increase the expression of ACE2, it was hypothesized that their application would lead to higher infection rates and more severe COVID-19 cases (65, 66). However, several studies found no significant difference between patients treated with or without ACE inhibitors and/or angiotensin II receptor blockers regarding the infection rate and COVID-19 outcome (67–70). Controversially, others even reported a lowered risk of SARS-CoV-2 infection or critical illness and death, respectively (71, 72). Notably, it was speculated that geographic and ethnic factors may influence the interaction between these drugs and COVID-19 (69, 71). Further studies are required to substantiate these findings.
The influence of gender and age on ACE2 expression is not fully understood yet. While several studies did not prove significant differences of ACE2 expression between young males and females (<55 years), it has been shown that the correlation between ACE2 and immune signatures in the lungs differ between the two sexes (49, 73–75). There have been contrasting results regarding the relation of ACE2 content to increasing age, with some finding an increase, a decrease, or no change at all (49, 73, 74). However, it has been suggested that steroid hormones may affect ACE2 activity with withdrawal of estrogen or testosterone causing an increase or a decrease, respectively (75).
Another important albeit overlooked function of ACE2 was illustrated in a recent study. Chadchan et al. found that the ACE2 protein is not only highly expressed in human endometrial stromal cells (HESCs), in particular during the secretory phase of the menstrual cycle, but it also increases significantly in stromal cells undergoing decidualization in vitro (76). They further observed that loss of ACE2 impeded decidualization (76). Additionally, Chadchan et al. described that ACE2 expression in the endometrium is induced by progesterone. Considering these results, it is plausible that ACE2 plays a vital role in decidualization of the human endometrium.
Furthermore, other studies have found that ACE2 and other components of RAS are expressed both in maternal and fetal tissues during pregnancy, suggesting their crucial role during implantation, vascular remodeling and labor (77–81). During pregnancy, the uterus and placenta contribute substantially to ACE2 production, thus causing a twofold increase in ACE2 activity with subsequent systemic vasodilation (82). The upregulation of RAS in the maternal decidua as well as in the endothelial and perivascular stromal cells during the first trimester of pregnancy coincides with spiral artery remodeling and angiogenesis (83). Dysregulation of uteroplacental RAS is reported to alter the tightly regulated maternal homeostasis causing pregnancy complications such as miscarriage, still birth and preeclampsia (84–86). It was also shown that plasma Ang 1-7, a product of ACE2, is elevated during healthy pregnancies and that preeclamptic mothers had lower levels of Ang 1-7 (87).
Remarkably, ACE2 is most abundant in the decidua in comparison with chorionic or amniotic tissues (77). Another compelling finding is that ACE2 expression is highest during early pregnancy and is negatively correlated with gestational age (88, 89). Moreover, fetal sex might affect maternal RAS and for instance, ACE2 mRNA levels were higher in decidual explants after 24h from women carrying a female fetus compared with those carrying a male fetus (90).
Briefly, ACE2 has essential functions for the RAS and contributes to the control of blood pressure as well as fluid and electrolyte homeostasis. ACE2 is expressed in various tissues throughout the human body and is protective against cardiovascular and respiratory diseases, among others. ACE2 is essential for decidualization and its production increases during pregnancy. Since ACE2 is also the entry receptor for SARS-CoV-2, its high expression in placental tissues has implications for pregnancies during COVID-19 infection, which will be covered in detail below.
SARS-CoV-2 and COVID-19
SARS-CoV-2 belongs to the same genus betacoronavirus as SARS-CoV and MERS-CoV, which are all enveloped viruses with a single-stranded positive-sense RNA (91, 92). Although the origin of SARS-CoV-2 has not been fully clarified yet, it is most likely that it originated from bats, which are a natural reservoir for coronaviruses, and was passed on to humans via an intermediate host such as pangolins (93, 94).
SARS-CoV-2 consists of four structural proteins: spike (S), nucleocapsid (N), membrane (M) and envelope (E) protein (95). The S-protein is of utmost interest, as it facilitates virus entry into host cells (96, 97). Due to the similarity of their S-proteins, SARS-CoV and SARS-CoV-2 both utilize the cell surface receptor ACE2 for attachment and penetration of host cells (97). However, the receptor-binding domain (RBD) of the S-protein differs among SARS-CoV and SARS-CoV-2, resulting in higher binding affinity to ACE2 of the latter (98). A precondition for the interaction of SARS-CoV-2 with ACE2 is S-protein priming by host proteases, among which the most relevant seems to be transmembrane protease serine 2 (TMPRSS2) (Figure 3) (99).
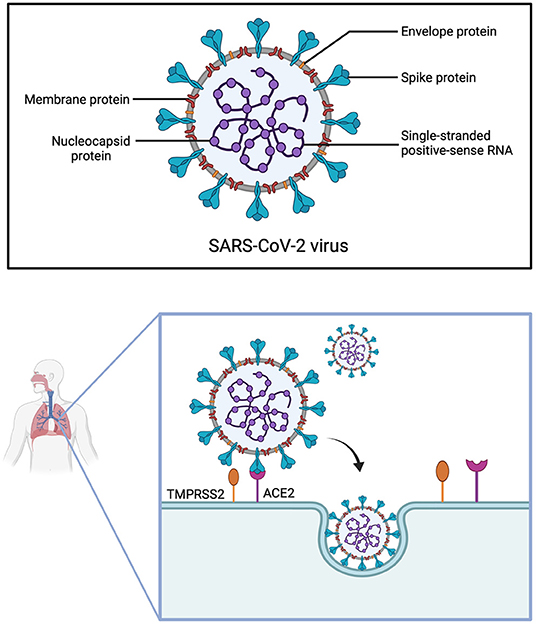
Figure 3. Structure of SARS-CoV-2 and cell entry. The Severe Acute Respiratory Syndrome Coronavirus 2 is made up of four structural proteins (envelope, membrane, spike and nucleocapsid protein) and has a single-stranded positive-sense RNA. For SARS-CoV-2 to infect cells of the respiratory tract, the spike protein first has to be cleaved by the transmembrane protease serine 2 (TMPRSS2), before it can interact with the angiotensin-converting enzyme 2 (ACE2) receptor. The virus then enters the cell through endocytosis.
The main route of transmission is through respiratory droplets, nevertheless, SARS-CoV-2 can be further spread via aerosols, direct and indirect contact, and feces (100–103). While it has been suggested that SARS-CoV-2 could be passed on via sexual contact and breastfeeding, more evidence is necessary (104, 105).
For COVID-19, the incubation period, defined as the time between infection and onset of symptoms, is approximately 5 days (106, 107). The basic reproduction number R0 of SARS-CoV-2 is estimated to lie between 2 and 3 with a peak viral load in the upper respiratory tract around symptom onset or shortly after (108–110). Not only can patients be infectious 1 to 3 days before any symptoms occur, but there have also been reports of asymptomatic transmission (110, 111).
The clinical presentation of COVID-19 varies greatly from asymptomatic and mild to critical and even fatal cases (112). Diagnosis is further impeded by unspecific symptoms, which resemble the clinical picture of the common cold, influenza or other respiratory diseases (113). The most common manifestations are fever and cough, which are present in the majority of the patients, followed by fatigue and shortness of breath (50, 92, 114). Anosmia, ageusia, myalgia and diarrhea are less frequent among COVID-19 patients (115, 116). Some symptoms, for instance fatigue or dyspnea, can persist despite microbiological recovery – a condition termed Long COVID (117, 118).
COVID-19 originally revealed itself through an outbreak of pneumonia (97). In severe cases, patients develop ARDS with hypoxia or even respiratory failure (119). Histopathological findings are diffuse alveolar damage with desquamation of pneumocytes, formation of hyaline membranes, edema and inflammatory infiltration by lymphocytes, as well as microvascular injury (120, 121). Correspondingly, chest computed tomography (CT) scans of COVID-19 patients commonly show bilateral distribution of ground-glass opacities with or without consolidations, “crazy paving” patterns and air bronchogram signs (122, 123).
COVID-19 not only involves the lungs and the respiratory tract, but also multiple organ systems (124). This includes cardiovascular (e.g. acute cardiac injury, myocarditis), gastrointestinal (e.g. nausea and vomiting, diarrhea), neurological (e.g. dizziness, stroke) and hematological manifestations (e.g. lymphocytopenia, thrombotic events, disseminated intravascular coagulation (DIC)) (124–128). A case point feature of COVID-19 is, that it triggers an extensive inflammatory response, the “cytokine storm”, which further aggravates damage done by the virus (129). A delay in immune response due to immune evasion of SARS-CoV-2 with consequentially unhindered virus replication is found in severe cases of COVID-19 (130–132). Virus-induced cell death prompts the recruitment of macrophages and neutrophils, followed by hyperinflammation (130). Subsequent tissue damage and multi-organ failure are the main cause of death in COVID-19 (133, 134).
The vast majority (80–90%) of COVID-19 cases are asymptomatic or mildly symptomatic, whereas among the critically ill the mortality rate is as high as 49% (112, 119, 135). The most important prognostic factor is age, with children mainly being asymptomatic or only exhibiting mild symptoms whereas elderly patients are at high risk for mortality (136–138). Other factors contributing to poor outcome are comorbidities such as hypertension, heart disease, diabetes mellitus, Chronic Obstructive Pulmonary Disease (COPD) and chronic renal disease (139–141). Furthermore, obesity and the male sex have been linked to increased severity of COVID-19 (141–143). Genetic factors also have an impact on COVID-19 infection and outcome with some studies suggesting links to HLA or ABO blood type (Table 1) (164–167).
COVID-19 and Women
Historically, women were overlooked in biomedical research and the male model was seen as the “norm”. Women were and are still underrepresented in clinical trials due to the notion that studies could be complicated by the menstrual cycle and that a potential fetus could be harmed (173). This practice of exclusion from clinical trials is even more common for pregnant women (174). Indeed, sex discrepancy also applies to animal models with a male bias in the majority of research fields (175). The belief that male data can be simply extrapolated to women leads to inadequate treatment of female patients, such as wrong dosage of drugs or more severe side effects (173, 176).
An astounding gender difference has been found in COVID-19; in that whilst infection rates are similar in both sexes, men are prone to having more severe infection and higher mortality (two- to threefold) (138, 144, 145, 177). Likewise, this bias toward males was also present in the MERS outbreak in 2014 (178). However, this pattern was not as consistent in the previous SARS epidemic in 2002, with only one study showing a significant difference in case fatality ratio between men and women (179, 180). Controversially, another review even reported that mainly females were affected by SARS (181).
One possible rationale for the female advantage lies in the sex chromosomes (182, 183). Since women possess two X-chromosomes, one of the X-chromosomes is silenced to compensate gene dosage (184). Some genes escape inactivation resulting in differential expression between sexes (185). The gene of the SARS-CoV-2 receptor ACE2 is located on the X-chromosome and is further recognized as an escape gene (185, 186). This implies that women might be in a more favorable position of elevated ACE2 expression which counterbalances the downregulation of ACE2 upon SARS-CoV-2 infection and therefore protects from an overactive RAS. However, expression does not equal enzyme activity and as described above, sex differential expression of ACE2 is still controversial (187, 188). Notably, soluble ACE2 (sACE2), which is generated through shedding of membrane-bound ACE2, was found to be higher in men compared with women as well as postmenopausal compared with premenopausal women (189–191). As higher levels of sACE2 are correlated with cardiovascular disease and diabetes, known risk factors for more severe cases of COVID-19, this may contribute to the male disadvantage (192).
Another potential link between genetics and the purported reduced risk in females is the fact that the X-chromosome contains a great repertoire of immune-related genes (193). It is noteworthy that women generally mount a faster and stronger innate and adaptive immune response whereas men are subject to reduced immune response and higher pathogen load (194). X-linked genes are suggested to play a pivotal role in autoimmune diseases, which are characterized by a heightened immune response against the patient's own cells and primarily affect women (195, 196). Genes encoding for pattern recognition receptors (PRRs) have a vital function in the innate immune system and consequently are of special interest regarding the delayed immune response in COVID-19 (197). Among them, Toll-like receptor 7 (TLR7) is not only responsible for the recognition of single-stranded viral RNA in endosomes, but its gene is also located on the X-chromosome and known to escape silencing in immune cells (198–200). Furthermore, reaction to TLR7 stimulation also differs depending on sex as peripheral blood mononuclear cells from females produce more Interferon-α (IFN-α), eliciting an anti-viral response, whereas in males higher production of Interleukin-10 (IL-10), an immunosuppressive cytokine, is induced (201, 202). Considering that differences in the immune response between the sexes occurs across all age groups, it is perhaps plausible that sex chromosomes are part of the reason why females seem to clear pathogens faster and have less severe COVID-19 cases (203).
Endocrine factors are another conceivable explanation for sex disparities in COVID-19 outcomes as the mortality rate in postmenopausal women is higher than in premenopausal women (204, 205). Estrogen has potent immunomodulatory effects and estrogen receptors are expressed by several immune cells (206). At high concentrations, as found periovulatory or during pregnancy, estrogen has mainly anti-inflammatory effects, for instance decreasing levels of C-reactive protein (CRP), IL-6 and TNF-α (207–210). In contrast, estrogen triggers pro-inflammatory pathways at lower doses (211). Furthermore, estrogen has proven to reduce morbidity of influenza infection through modifying immune cell recruitment and cytokine production, as well as scaling down virus replication in females (212, 213). Despite less elderly women succumbing to COVID-19 in comparison to elderly men, climacteric women are still at higher risk of worse outcomes than their premenopausal counterparts, and futher, estradiol treatment was shown to improve survival (214, 215).
Certain comorbidities are known to negatively impact COVID-19 outcomes; it is noteworthy that the prevalence of hypertension, diabetes and COPD is lower in the female population (216–218). This can be attributed to women leading healthier lifestyles, such as less smoking, lower alcohol consumption and more physical activity (219–221). Nonetheless, biological factors might also have an impact on the development of the said comorbidities. Aside from ACE2 activity positively correlating with estrogen levels, protective effects of estrogen on atrial tissue through modulation of RAS and upregulation of ACE2 were observed (222, 223). Moreover, in a study on mice, obese females had higher adipose ACE2 activity and, unlike obese males, did not develop hypertension (224). When ovariectomized, female mice also showed reduced ACE2 activity and obesity-hypertension, which could be reversed through treatment with estrogen (224, 225). Akin to estrogen's shielding properties in hypertension, estrogen also guards premenopausal women from diabetes mellitus and estrogen replacement therapy in postmenopausal patients is beneficial for metabolic health (226, 227). In respect to COVID-19, it is noteworthy to point out that estrogen was shown to decrease ACE2 expression of differentiated airway epithelial cells, providing another clue for sex-difference in infection (228).
In summary, COVID-19 affects men with disproportionately higher infection rates, more severe cases, and higher mortality than women. This can be attributed to sex chromosomes, as the ACE2 gene lies on the X-chromosome and is known to escape silencing. Several genes related to immune response are also located on the X-chromosome, resulting in a faster and stronger immune defense in females. Additionally, hormones might cause sex differences in COVID-19 outcomes. High concentrations of estrogen have anti-inflammatory properties, thereby alleviating the detrimental effects of the cytokine storm in COVID-19. Lastly, women tend to have less comorbidities than men, due to healthier lifestyles and the protective effects of estrogen.
COVID-19 and Pregnancy
Pregnancy is a unique physiological condition with changes in the endocrine system (e.g., high levels of cortisol, progesterone and estrogen), cardiovascular system (e.g., increased cardiac output, decreased systemic vascular resistance, higher blood volume) and respiratory system (e.g., swelling of upper respiratory tract, elevated diaphragm, lower total lung capacity, hyperventilation) (229, 230). Considering COVID-19, physiologically elevated basal oxygen consumption levels, a predisposition to developing lung edema and dyspnea, as well as hypercoagulability are all relevant during pregnancy (231–234).
According to a meta-analysis from Di Toro et al. which included 1,100 pregnancies, most pregnant women infected with SARS-CoV-2 had uncomplicated clinical courses with frequent symptoms being fever and cough, pneumonia was prevalent in 89% of the cases (235). They further found an ICU admission rate of 8% and 5 maternal deaths, implying that pregnant women do not face worse outcomes than non-pregnant women. Other studies have also found pregnant COVID-19 patients to have similar clinical characteristics and disease outcomes as non-pregnant women (236, 237). Conversely, higher admission rates to ICU and a higher risk for more severe COVID-19 cases was reported among pregnant compared with non-pregnant patients (238–240). Advanced maternal age, comorbidities such as diabetes mellitus, hypertension, low socioeconomic status as well as obesity have all been identified as possible risk factors for maternal death from COVID-19 (241).
The current pandemic has resulted in the requirement to transform and adapt healthcare services for pregnant women in high-risk groups including women with gestational diabetes mellitus (GDM) or diabetes mellitus. GDM is the most common medical complication of pregnancy and affects 10% of pregnancies globally. Those diagnosed with this condition are at higher risk for a severe COVID-19 infection due to predisposing factors such as hyperglycemia, obesity and hypertension (242). Critically, the most common underlying conditions of pregnant women with SARS-CoV-2 that were hospitalized for severe disease were pre-pregnancy BMI ≥30 kg/m2 (41.7%) and diabetes mellitus (Type 2) (12.5%) (243). An interplay of several pathophysiological mechanisms is thought to increase the risk of an unfavorable course and a worse prognosis for patients with GDM. In general, diabetes or insulin resistance reduces T cell function leading to an impaired immune response. This results in the global cellular dysfunction underlying a variety of symptoms associated with diabetes, including a higher risk of respiratory infections (244). Moreover, it has been indicated that some COVID-19 patients develop a diabetes-like syndrome (245). Taken together, these factors could lead to poor pregnancy outcomes including pre-term labor, neonatal admissions to ICU or still birth. Therefore, several healthcare guidelines from the NIH (USA), STIKO/Robert Koch Institute (Germany) and the RCOG (UK) have emphatically expressed the urgency for pregnant women (diabetic and/or obese) to be vaccinated.
Commonly reported neonatal complications are preterm birth, premature rupture of membranes and fetal distress, while intrauterine growth restriction, miscarriage and death are rare events (246, 247). The high cesarean section rate (85%) among COVID-19 positive women is noteworthy, despite COVID-19 not posing a contraindication for vaginal delivery (235). Possibly, improved infection control during labor explains the preference for C-section, however, vaginal fluids were repeatedly tested were negative for SARS-CoV-2, indicating low risk for intrapartum transmission (246, 247).
Vertical transmission describes the process of ante-, peri- or postnatal mother-to-child transmission of infectious agents (248). The focus of this review will be on vertical transmission before birth, meaning in utero infection of the fetus. Several bacteria, viruses and parasites are known to cause congenital infection, the most common one being the cytomegalovirus (CMV) (249). The consequences of these infections depend on the pathogens, with some causing fetal death (e.g., parvovirus B19, mumps virus, rubella virus) and others leading to malformations or organ defects (e.g., Chlamydia trachomatis, Treponema pallidum, CMV, Toxoplasma gondii) (250). Another determinant of teratogenicity is the time of infection: the rubella virus causes cerebral, cardiac, ophthalmic and auditory defects when infection occurs in the first trimester of pregnancy (during organogenesis), whereas the fetus is most vulnerable to the hemolytic effect of parvovirus B19 and subsequent hydrops fetalis during the second trimester, due to heightened hematopoiesis in the fetal liver (Figure 4) (250, 251).
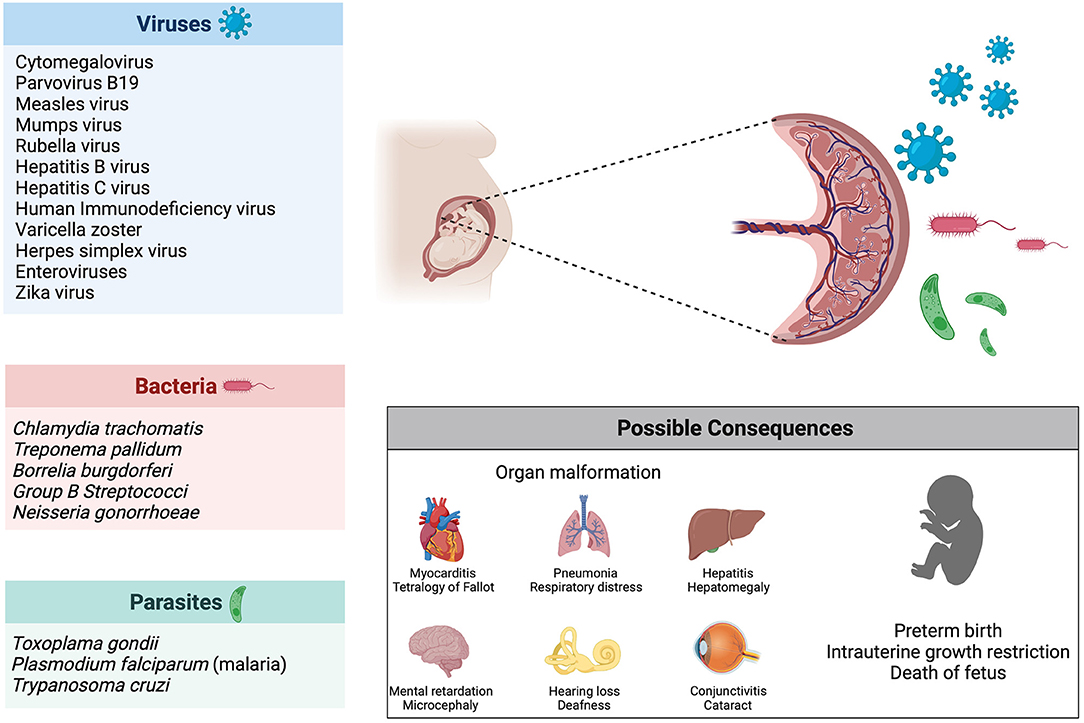
Figure 4. Infection during pregnancy. Several pathogens (viruses, bacteria and parasites) are known to be vertically transmitted during pregnancy. Possible consequences of infection during pregnancy include organ malformations, preterm birth and death of the fetus.
While SARS-CoV-2 can be passed from mother to infant through respiratory droplets during labor or in the postnatal period, the question of in utero transmission remains unresolved (252). Infection rates among neonates born to COVID-19 positive mothers are low (6%), however, cases of early-onset COVID-19 exist with infants testing positive via nasopharyngeal swabs within 12 h postpartum (235, 253). Further, antibodies against SARS-CoV-2 identified in newborns shed additional light on the possibility of prenatal vertical transmission (254, 255). In contrast to IgG, which is subject to physiological transplacental transfer and therefore could originate from maternal blood, elevated levels of IgM indicate infection of the fetus in utero, as IgM usually does not cross the placental barrier (256). Nevertheless, inflammatory processes can affect the placental barrier and result in altered transfer of immunoglobulin (257). Thus, elevated IgM levels in neonates are no definite proof for in utero transmission of SARS-CoV-2.
Furthermore, in a study from Hecht et al. SARS-CoV-2 RNA was detected in the syncytiotrophoblast and cytotrophoblast of placentas from COVID-19 positive mothers (258). This demonstrates that SARS-CoV-2 can infect the placenta, however, it does not definitely confirm vertical transmission. Further, these women were tested (positive for COVID-19) peripartum, limiting the insights into late pregnancy infection.
A potential way for vertical transmission of SARS-CoV-2 during pregnancy is via ACE2. As reviewed above, ACE2 is expressed in fetal and maternal tissues during pregnancy and most amply so in the early stages (77–81, 88, 89). Strong co-expression of ACE2 and TMPRSS2, necessary for cleavage of the spike protein, at the maternal-fetal-interface was reported by some studies (78, 259). In contrast, when examining expression patterns of ACE2 and TMPRSS2 in placentas, Pique-Regi et al. only found negligible co-transcription, especially compared to receptors for Zika virus and CMV, both known to cause congenital infections (260). While ACE2 was repeatedly shown to be present in endometrial and placental tissues, research on TMPRSS2 is inconclusive (258, 260, 261). It should be noted that low expression of TMPRSS2 does not necessarily equal low risk of SARS-CoV-2 infection. Other proteases have been suggested to provide an alternate pathway for viral cell entry, such as TMPRSS4, furin or cathepsin L (94). Notably, TMPRSS4 is expressed alongside ACE2 in the endometrium and, akin to ACE2, increases toward the window of implantation (261). Furthermore, TMPRSS4 has the capacity of facilitating cell entry of SARS-CoV-2, thus, making it a candidate for promoting vertical transmission (262).
Another option for in utero transmission of SARS-CoV-2 while omitting ACE2 is through infected blood cells. While viremia in COVID-19 exists, only low levels of SARS-CoV-2 RNA are detectable in blood of infected patients (263, 264). When studying the full-term placenta from a COVID-19 positive mother whose newborn was also tested positive, Facchetti et al. showed that viral proteins and RNA were present in numerous fetal and maternal cells (265). Of particular interest is the finding that fetal monocytes were infected with SARS-CoV-2, thus, providing a potential vehicle for vertical transmission.
When interpreting results regarding vertical transmission, it must be considered that most studies solely include mothers tested positive for COVID-19 during the third trimester or peripartum and little attention has been devoted to early pregnancy. Recently, Valdespino-Vázquez et al. examined placental and fetal tissues from a miscarriage during the first trimester of a COVID-19 positive patient (266). They found viral proteins and RNA as well as hyper-inflammation present in both the placenta and the fetal organs (266). As organogenesis occurs in early pregnancy, infection during this crucial time would have detrimental effects on the fetal outcome. Additionally, a case report on a first trimester COVID-19 infection indicated, not only that SARS-CoV-2 persists in the placenta, but it also infected the amniotic fluid and fetal membranes (267). Strikingly, while the mother remained asymptomatic, her unborn succumbed to hydrops fetalis and death (267). Therefore, more research on COVID-19 and its effects on early pregnancy is urgently needed.
Indeed, vertical transmission is not the only way a pathogen can harm an unborn infant. Firstly, SARS-CoV-2 is known to cause placental damage, including maternal and fetal vascular malperfusion, decidual arteriopathy, intervillous thrombi as well as inflammation (e.g., villitis, intervillositis, chorioamnitis) (268–270). Abnormal ACE2 expression caused by SARS-CoV-2 infection in both decidua and placenta could potentially impair key physiological processes, such as placentation and vascularization during pregnancy (271). Hence, dysregulation of RAS could play a critical role in developing preeclampsia-like placental pathology, COVID-19 associated miscarriages and still births. Placental impairment leads to compromised fetal supply of oxygen and nutrients with subsequent complications, such as intrauterine growth restriction or miscarriage, independent of vertical transmission (272). Remarkably, placental pathology exists even in mild or asymptomatic cases of COVID-19 (273, 274).
Aside from locally impacting the placenta, COVID-19 is a systemic disease and the maternal immune response can result in fetal injury (250). Being subjected to an inflammatory milieu, can damage the lungs and brain of the developing fetus (250). Likewise, maternal fever and upper respiratory infection, both characteristics of COVID-19, are linked to congenital heart disease (275). Deleterious effects of the cytokine storm induced by SARS-CoV-2 are not confined to the pregnant woman but could also affect the fetus, possibly resulting in multi-organ failure and ultimately fetal demise (276).
Of equal importance are psychological implications of the ongoing pandemic, such as higher rates of depression and anxiety among pregnant women, which might impact health and well-being of both mother and child (277, 278). Psychological stress is a known risk factor for miscarriage, especially during early pregnancy, which might be linked to elevated cortisol levels (279, 280). Furthermore, the pandemic lead to hampered access to pre- and postnatal care services, possibly contributing to underdiagnosis of complications (281). Interestingly, pregnant women were found to be at lower risk for depressive symptoms in comparison to non-pregnant women and mothers delivering during the COVID-19 pandemic had reduced risk of postpartum-depression than before the pandemic (282, 283). This points to substantial psychological resilience among expectant mothers.
In general, gravid patients present certain challenges to medical treatment. As mentioned above, pregnant women exhibit edema and swelling in the upper respiratory tract, thereby complicating endotracheal intubation (229). Moreover, pregnancy has implications on medication used to treat COVID-19 and vice versa; for example, Favipiravir, an antiviral drug used against COVID-19, should not be administered during pregnancy, and for magnesium sulfate, used for prophylaxis and treatment of preeclampsia, dosage adjustment is required in COVID-19 patients (284, 285).
While vaccination is seen as a promising way to resolve the COVID-19 pandemic, pregnant women were not included in clinical trials and, accordingly, a lack of evidence exists regarding safety and efficacy of COVID-19 vaccines in pregnancy (286). This not only resulted in differing recommendations from national and international organizations regarding vaccination against SARS-CoV-2 during pregnancy, but also in confusion and low acceptance among pregnant women (287, 288). Very recently, evidence about the safety and effectiveness of COVID-19 vaccines for pregnant women is emerging. Preliminary data indicates that vaccination against COVID-19 during pregnancy and lactation is safe regarding maternal side effects, female fertility as well as pregnancy and neonatal outcomes (289, 290). Based on 35,691 volunteers during or shortly before pregnancy, participants did not report any adverse side effects among pregnant women who received mRNA COVID-19 vaccines either from Pfizer/BioNTech or Moderna (290). It was demonstrated for COVID-19 mRNA vaccines that not only an immune response could be elicited in pregnant and lactating women but also that the antibodies could be passed onto their infants through the umbilical cord blood and breast milk (291–294). Given the ongoing pandemic, further research is urgently needed to provide reliable recommendations for the vaccination of pregnant and lactating women.
Concluding Remarks and Future Perspectives
This review describes the impact of COVID-19 on non-pregnant and pregnant women. Its aim is to explore why the SARS-CoV-2 infection affects men more severely than women and bringing knowledge about genetic, endocrine and exogenous factors together. Different ways of how COVID-19 can harm pregnant mothers and neonates are discussed and currently available data on the possibility of vertical transmission is summarized. This review intends to connect the pathophysiology of COVID-19 to the physiology of pregnancy, decidualization and RAS.
While knowledge on COVID-19 is increasing rapidly, a lack of evidence for the impact on pregnancy remains. Although the currently available data shows that non-pregnant and pregnant women seem to be less affected in terms of severity and mortality, little is known about the long-term effects of viral infection on the fetus. Vertical transmission seems to be rare, but neither is the possibility of in utero transmission excluded, nor are the effects of the maternal immune response on the unborn fully understood. Considering the therapeutic obstacles that the pandemic poses on pregnant COVID-19 patients, further research is needed to improve maternal and fetal management.
Notably, a major part of research on COVID-19 and pregnancy focuses explicitly on the third trimester, resulting in a lack of knowledge concerning adverse effects and vertical transmission during early pregnancy. With new SARS-CoV-2 variants emerging, continuous effort is required to shed light on their consequences for pregnancy as well as lactation. Furthermore, pregnant women should not be excluded in future clinical trials for COVID-19 vaccines and therapies. Scientific progress will enable doctors and health workers to provide evidence-based treatments for pregnant women.
Author Contributions
AL, MS, and JR performed information retrieval and/or analyzed data. MS, YS, and SB provided intellectual input, resources, and funding. AL wrote the original draft of the manuscript and drew the figures. The presented work here is used in MD thesis of AL. All authors reviewed the manuscript and approved of submission.
Funding
This work was supported by funding to MS intramural funds of Tübingen University the IZKF (2510-0-0) and by the Margarete von Wrangell (MvW 31-7635.41/118/3) habilitation scholarship co-funded by the Ministry of Science, Research and the arts (MWK) of the state of Baden-Württemberg and by the European Social Funds. AL is supported by the Tübingen Medical faculty Interdisziplinäres Promotionskolleg Medizin program (2021-1-02). To YS and MS the Ferring COVID-19 Investigational Grants in Reproductive Medicine and Maternal Health (RMMH) and we also thank the Open Access Publishing Fund of Tuebingen University. The funders played no role in the study design, in the collection, analysis and interpretation of data, in the writing of the report or in the decision to submit the article for publication.
Conflict of Interest
The authors declare that the research was conducted in the absence of any commercial or financial relationships that could be construed as a potential conflict of interest.
Publisher's Note
All claims expressed in this article are solely those of the authors and do not necessarily represent those of their affiliated organizations, or those of the publisher, the editors and the reviewers. Any product that may be evaluated in this article, or claim that may be made by its manufacturer, is not guaranteed or endorsed by the publisher.
Acknowledgments
All figures were created with BioRender.com. Figure 2 was adapted from Renin-Angiotensin System by BioRender.com.
References
1. Wang C, Horby PW, Hayden FG, Gao GF, A. novel coronavirus outbreak of global health concern. Lancet. (2020) 395:470–3. doi: 10.1016/S0140-6736(20)30185-9
2. WHO. WHO COVID-19 Dashboard Genova (2020). Available online at: https://covid19.who.int/ (accessed October 30, 2021).
3. Ghebreyesus T. WHO Director-General's opening remarks at the media briefing on COVID-19. (2020). Available from: https://www.who.int/director-general/speeches/detail/who-director-general-s-opening-remarks-at-the-media-briefing-on-covid-19---11-march-2020 (accessed March 11, 2020)
4. Mor G, Cardenas I. The immune system in pregnancy: a unique complexity. Am J Reprod Immunol. (2010) 63:425–33. doi: 10.1111/j.1600-0897.2010.00836.x
5. Somerville LK, Basile K, Dwyer DE, Kok J. The impact of influenza virus infection in pregnancy. Future Microbiol. (2018) 13:263–74. doi: 10.2217/fmb-2017-0096
6. Silasi M, Cardenas I, Kwon JY, Racicot K, Aldo P, Mor G. Viral infections during pregnancy. Am J Reprod Immunol. (2015) 73:199–213. doi: 10.1111/aji.12355
7. Ochoa-Bernal MA, Fazleabas AT. Physiologic events of embryo implantation and decidualization in human and non-human primates. Int J Mol Sci. (2020) 21. doi: 10.3390/ijms21061973
8. Critchley HOD, Maybin JA, Armstrong GM, Williams ARW. Physiology of the Endometrium and Regulation of Menstruation. Physiol Rev. (2020) 100:1149–79. doi: 10.1152/physrev.00031.2019
9. Brosens JJ, Parker MG, McIndoe A, Pijnenborg R, Brosens IA. A role for menstruation in preconditioning the uterus for successful pregnancy. Am J Obstet Gynecol. (2009) 200:615.e1–6. doi: 10.1016/j.ajog.2008.11.037
10. Clancy KB. Reproductive ecology and the endometrium: physiology, variation, and new directions. Am J Phys Anthropol. (2009) 140 Suppl 49:137–54. doi: 10.1002/ajpa.21188
11. Gellersen B, Brosens JJ. Cyclic decidualization of the human endometrium in reproductive health and failure. Endocr Rev. (2014) 35:851–905. doi: 10.1210/er.2014-1045
12. Suthaporn S, Jayaprakasan K, Thornton JG, Walker KF, Castellanos M, May S, et al. Evaluating the influence of progesterone concentration and time of exposure on in vitro endometrial decidualisation. Mol Cell Endocrinol. (2021) 528:111242. doi: 10.1016/j.mce.2021.111242
13. Roberts DK, Parmley TH, Walker NJ, Horbelt DV. Ultrastructure of the microvasculature in the human endometrium throughout the normal menstrual cycle. Am J Obstet Gynecol. (1992) 166:1393–406. doi: 10.1016/0002-9378(92)91611-D
14. Gellersen B, Brosens IA, Brosens JJ. Decidualization of the human endometrium: mechanisms, functions, and clinical perspectives. Semin Reprod Med. (2007) 25:445–53. doi: 10.1055/s-2007-991042
15. Ng SW, Norwitz GA, Pavlicev M, Tilburgs T, Simón C, Norwitz ER. Endometrial decidualization: the primary driver of pregnancy health. Int J Mol Sci. (2020) 21. doi: 10.3390/ijms21114092
16. Okada H, Tsuzuki T, Murata H. Decidualization of the human endometrium. Reprod Med Biol. (2018) 17:220–7. doi: 10.1002/rmb2.12088
17. Sachs L, Shelesnyak MC. The development and suppression of polyploidy in the developing and suppressed deciduoma in the rat. J Endocrinol. (1955) 12:146–51. doi: 10.1677/joe.0.0120146
18. Sroga JM, Ma X, Das SK. Developmental regulation of decidual cell polyploidy at the site of implantation. Front Biosci. (2012) 4:1475–86. doi: 10.2741/s347
19. Tan J, Kan A, Hitkari J, Taylor B, Tallon N, Warraich G, et al. The role of the endometrial receptivity array (ERA) in patients who have failed euploid embryo transfers. J Assist Reprod Genet. (2018) 35:683–92. doi: 10.1007/s10815-017-1112-2
20. Kimber SJ. Molecular interactions at the maternal-embryonic interface during the early phase of implantation. Semin Reprod Med. (2000) 18:237–53. doi: 10.1055/s-2000-12562
21. Achache H, Revel A. Endometrial receptivity markers, the journey to successful embryo implantation. Hum Reprod Update. (2006) 12:731–46. doi: 10.1093/humupd/dml004
22. Sharma S, Godbole G, Modi D. Decidual control of trophoblast invasion. Am J Reprod Immunol. (2016) 75:341–50. doi: 10.1111/aji.12466
23. Weimar CH, Macklon NS, Post Uiterweer ED, Brosens JJ, Gellersen B. The motile and invasive capacity of human endometrial stromal cells: implications for normal and impaired reproductive function. Hum Reprod Update. (2013) 19:542–57. doi: 10.1093/humupd/dmt025
24. Grewal S, Carver J, Ridley AJ, Mardon HJ. Human endometrial stromal cell rho GTPases have opposing roles in regulating focal adhesion turnover and embryo invasion in vitro. Biol Reprod. (2010) 83:75–82. doi: 10.1095/biolreprod.109.080630
25. Grewal S, Carver JG, Ridley AJ, Mardon HJ. Implantation of the human embryo requires Rac1-dependent endometrial stromal cell migration. Proc Natl Acad Sci U S A. (2008) 105:16189–94. doi: 10.1073/pnas.0806219105
26. Teklenburg G, Salker M, Molokhia M, Lavery S, Trew G, Aojanepong T, et al. Natural selection of human embryos: decidualizing endometrial stromal cells serve as sensors of embryo quality upon implantation. PLoS ONE. (2010) 5:e10258. doi: 10.1371/journal.pone.0010258
27. Brosens JJ, Salker MS, Teklenburg G, Nautiyal J, Salter S, Lucas ES, et al. Uterine selection of human embryos at implantation. Sci Rep. (2014) 4:3894. doi: 10.1038/srep03894
28. Koot YE, Teklenburg G, Salker MS, Brosens JJ, Macklon NS. Molecular aspects of implantation failure. Biochim Biophys Acta. (2012) 1822:1943–50. doi: 10.1016/j.bbadis.2012.05.017
29. Cha J, Sun X, Dey SK. Mechanisms of implantation: strategies for successful pregnancy. Nat Med. (2012) 18:1754–67. doi: 10.1038/nm.3012
30. Damsky C, Sutherland A, Fisher S. Extracellular matrix 5: adhesive interactions in early mammalian embryogenesis, implantation, and placentation. FASEB J. (1993) 7:1320–9. doi: 10.1096/fasebj.7.14.8224605
31. Iwahashi M, Muragaki Y, Ooshima A, Yamoto M, Nakano R. Alterations in distribution and composition of the extracellular matrix during decidualization of the human endometrium. J Reprod Fertil. (1996) 108:147–55. doi: 10.1530/jrf.0.1080147
32. Erlebacher A. Immunology of the maternal-fetal interface. Annu Rev Immunol. (2013) 31:387–411. doi: 10.1146/annurev-immunol-032712-100003
33. Yang F, Zheng Q, Jin L. Dynamic function and composition changes of immune cells during normal and pathological pregnancy at the maternal-fetal interface. Front Immunol. (2019) 10:2317. doi: 10.3389/fimmu.2019.02317
34. Bulmer JN, Williams PJ, Lash GE. Immune cells in the placental bed. Int J Dev Biol. (2010) 54:281–94. doi: 10.1387/ijdb.082763jb
35. Sojka DK, Yang L, Yokoyama WM. Uterine natural killer cells. Front Immunol. (2019) 10:960. doi: 10.3389/fimmu.2019.00960
36. Brighton PJ, Maruyama Y, Fishwick K, Vrljicak P, Tewary S, Fujihara R, et al. Clearance of senescent decidual cells by uterine natural killer cells in cycling human endometrium. Elife. (2017) 6. doi: 10.7554/eLife.31274
37. Bonney EA. Alternative theories: Pregnancy and immune tolerance. J Reprod Immunol. (2017) 123:65–71. doi: 10.1016/j.jri.2017.09.005
38. Ticconi C, Pietropolli A, Di Simone N, Piccione E, Fazleabas A. Endometrial immune dysfunction in recurrent pregnancy loss. Int J Mol Sci. (2019) 20. doi: 10.3390/ijms20215332
39. Collins MK, Tay CS, Erlebacher A. Dendritic cell entrapment within the pregnant uterus inhibits immune surveillance of the maternal/fetal interface in mice. J Clin Invest. (2009) 119:2062–73. doi: 10.1172/JCI38714
40. Tirado-González I, Freitag N, Barrientos G, Shaikly V, Nagaeva O, Strand M, et al. Galectin-1 influences trophoblast immune evasion and emerges as a predictive factor for the outcome of pregnancy. Mol Hum Reprod. (2013) 19:43–53. doi: 10.1093/molehr/gas043
41. Chang RQ, Li DJ, Li MQ. The role of indoleamine-2,3-dioxygenase in normal and pathological pregnancies. Am J Reprod Immunol. (2018) 79:e12786. doi: 10.1111/aji.12786
42. Sagrillo-Fagundes L, Bienvenue-Pariseault J, Legembre P, Vaillancourt C. An insight into the role of the death receptor CD95 throughout pregnancy: guardian, facilitator, or foe. Birth Defects Res. (2019) 111:197–211. doi: 10.1002/bdr2.1470
43. Gheblawi M, Wang K, Viveiros A, Nguyen Q, Zhong JC, Turner AJ, et al. Angiotensin-converting enzyme 2: SARS-CoV-2 receptor and regulator of the renin-angiotensin system: celebrating the 20th anniversary of the discovery of ACE2. Circ Res. (2020) 126:1456–74. doi: 10.1161/CIRCRESAHA.120.317015
44. Hamming I, Cooper ME, Haagmans BL, Hooper NM, Korstanje R, Osterhaus AD, et al. The emerging role of ACE2 in physiology and disease. J Pathol. (2007) 212:1–11. doi: 10.1002/path.2162
45. Donoghue M, Hsieh F, Baronas E, Godbout K, Gosselin M, Stagliano N, et al. A novel angiotensin-converting enzyme-related carboxypeptidase (ACE2) converts angiotensin I to angiotensin 1-9. Circ Res. (2000) 87:E1–9. doi: 10.1161/01.RES.87.5.e1
46. Wang K, Gheblawi M, Oudit GY. Angiotensin converting enzyme 2: a double-edged sword. Circulation. (2020) 142:426–8. doi: 10.1161/CIRCULATIONAHA.120.047049
47. Li Y, Zhou W, Yang L, You R. Physiological and pathological regulation of ACE2, the SARS-CoV-2 receptor. Pharmacol Res. (2020) 157:104833. doi: 10.1016/j.phrs.2020.104833
48. Hamming I, Timens W, Bulthuis ML, Lely AT, Navis G, van Goor H. Tissue distribution of ACE2 protein, the functional receptor for SARS coronavirus. A first step in understanding SARS pathogenesis. J Pathol. (2004) 203:631–7. doi: 10.1002/path.1570
49. Li MY, Li L, Zhang Y, Wang XS. Expression of the SARS-CoV-2 cell receptor gene ACE2 in a wide variety of human tissues. Infect Dis Poverty. (2020) 9:45. doi: 10.1186/s40249-020-00662-x
50. Wiersinga WJ, Rhodes A, Cheng AC, Peacock SJ, Prescott HC. Pathophysiology, transmission, diagnosis, and treatment of coronavirus disease 2019 (COVID-19): a review. Jama. (2020) 324:782–93. doi: 10.1001/jama.2020.12839
51. Patel VB, Zhong JC, Grant MB, Oudit GY. Role of the ACE2/Angiotensin 1-7 axis of the renin-angiotensin system in heart failure. Circ Res. (2016) 118:1313–26. doi: 10.1161/CIRCRESAHA.116.307708
52. Crackower MA, Sarao R, Oudit GY, Yagil C, Kozieradzki I, Scanga SE, et al. Angiotensin-converting enzyme 2 is an essential regulator of heart function. Nature. (2002) 417:822–8. doi: 10.1038/nature00786
53. Bindom SM, Lazartigues E. The sweeter side of ACE2: physiological evidence for a role in diabetes. Mol Cell Endocrinol. (2009) 302:193–202. doi: 10.1016/j.mce.2008.09.020
54. Shiota A, Yamamoto K, Ohishi M, Tatara Y, Ohnishi M, Maekawa Y, et al. Loss of ACE2 accelerates time-dependent glomerular and tubulointerstitial damage in streptozotocin-induced diabetic mice. Hypertens Res. (2010) 33:298–307. doi: 10.1038/hr.2009.231
55. Imai Y, Kuba K, Rao S, Huan Y, Guo F, Guan B, et al. Angiotensin-converting enzyme 2 protects from severe acute lung failure. Nature. (2005) 436:112–6. doi: 10.1038/nature03712
56. Ejaz H, Alsrhani A, Zafar A, Javed H, Junaid K, Abdalla AE, et al. COVID-19 and comorbidities: deleterious impact on infected patients. J Infect Public Health. (2020) 13:1833–9. doi: 10.1016/j.jiph.2020.07.014
57. Cheng H, Wang Y, Wang GQ. Organ-protective effect of angiotensin-converting enzyme 2 and its effect on the prognosis of COVID-19. J Med Virol. (2020) 92:726–30. doi: 10.1002/jmv.25785
58. Verdecchia P, Cavallini C, Spanevello A, Angeli F. The pivotal link between ACE2 deficiency and SARS-CoV-2 infection. Eur J Intern Med. (2020) 76:14–20. doi: 10.1016/j.ejim.2020.04.037
59. Wang H, Yang P, Liu K, Guo F, Zhang Y, Zhang G, et al. SARS coronavirus entry into host cells through a novel clathrin- and caveolae-independent endocytic pathway. Cell Res. (2008) 18:290–301. doi: 10.1038/cr.2008.15
60. Ou X, Liu Y, Lei X, Li P, Mi D, Ren L, et al. Characterization of spike glycoprotein of SARS-CoV-2 on virus entry and its immune cross-reactivity with SARS-CoV. Nat Commun. (2020) 11:1620. doi: 10.1038/s41467-020-15562-9
61. Sarzani R, Giulietti F, Di Pentima C, Giordano P, Spannella F. Disequilibrium between the classic renin-angiotensin system and its opposing arm in SARS-CoV-2-related lung injury. Am J Physiol Lung Cell Mol Physiol. (2020) 319:L325–l36. doi: 10.1152/ajplung.00189.2020
62. Amraei R, Rahimi N. COVID-19, Renin-Angiotensin System and Endothelial Dysfunction. Cells. (2020) 9. doi: 10.3390/cells9071652
63. D'Ardes D, Boccatonda A, Rossi I, Guagnano MT, Santilli F, Cipollone F, et al. COVID-19 and RAS: unravelling an unclear relationship. Int J Mol Sci. (2020) 21. doi: 10.3390/ijms21083003
64. Rossi GP, Sanga V, Barton M. Potential harmful effects of discontinuing ACE-inhibitors and ARBs in COVID-19 patients. eLife. (2020) 9:e57278. doi: 10.7554/eLife.57278
65. Fang L, Karakiulakis G, Roth M. Are patients with hypertension and diabetes mellitus at increased risk for COVID-19 infection? Lancet Respir Med. (2020) 8:e21. doi: 10.1016/S2213-2600(20)30116-8
66. Sriram K, Insel PA. Risks of ACE inhibitor and ARB usage in COVID-19: evaluating the evidence. Clin Pharmacol Ther. (2020) 108:236–41. doi: 10.1002/cpt.1863
67. Fosbøl EL, Butt JH, Østergaard L, Andersson C, Selmer C, Kragholm K, et al. Association of angiotensin-converting enzyme inhibitor or angiotensin receptor blocker use with COVID-19 diagnosis and mortality. Jama. (2020) 324:168–77. doi: 10.1001/jama.2020.11301
68. Flacco ME, Acuti Martellucci C, Bravi F, Parruti G, Cappadona R, Mascitelli A, et al. Treatment with ACE inhibitors or ARBs and risk of severe/lethal COVID-19: a meta-analysis. Heart. (2020) 106:1519–24. doi: 10.1136/heartjnl-2020-317336
69. Patoulias D, Katsimardou A, Stavropoulos K, Imprialos K, Kalogirou MS, Doumas M. Renin-angiotensin system inhibitors and COVID-19: a systematic review and meta-analysis. Evidence for significant geographical disparities. Curr Hypertens Rep. (2020) 22:90. doi: 10.1007/s11906-020-01101-w
70. Reynolds HR, Adhikari S, Pulgarin C, Troxel AB, Iturrate E, Johnson SB, et al. Renin-angiotensin-aldosterone system inhibitors and risk of Covid-19. N Engl J Med. (2020) 382:2441–8. doi: 10.1056/NEJMoa2008975
71. Hippisley-Cox J, Young D, Coupland C, Channon KM, Tan PS, Harrison DA, et al. Risk of severe COVID-19 disease with ACE inhibitors and angiotensin receptor blockers: cohort study including 83 million people. Heart. (2020) 106:1503–11. doi: 10.1136/heartjnl-2020-317393
72. Yang G, Tan Z, Zhou L, Yang M, Peng L, Liu J, et al. Effects of angiotensin II receptor blockers and ACE (angiotensin-converting enzyme) inhibitors on virus infection, inflammatory status, and clinical outcomes in patients with COVID-19 and hypertension: a single-center retrospective study. Hypertension. (2020) 76:51–8. doi: 10.1161/HYPERTENSIONAHA.120.15143
73. Fernández-Atucha A, Izagirre A, Fraile-Bermúdez AB, Kortajarena M, Larrinaga G, Martinez-Lage P, et al. Sex differences in the aging pattern of renin-angiotensin system serum peptidases. Biol Sex Differ. (2017) 8:5. doi: 10.1186/s13293-017-0128-8
74. Xie X, Chen J, Wang X, Zhang F, Liu Y. Age- and gender-related difference of ACE2 expression in rat lung. Life Sci. (2006) 78:2166–71. doi: 10.1016/j.lfs.2005.09.038
75. Dalpiaz PL, Lamas AZ, Caliman IF, Ribeiro RF, Abreu GR, Moyses MR, et al. Sex hormones promote opposite effects on ACE and ACE2 activity, hypertrophy and cardiac contractility in spontaneously hypertensive rats. PLoS ONE. (2015) 10:e0127515. doi: 10.1371/journal.pone.0127515
76. Chadchan SB, Popli P, Maurya VK, Kommagani R. The SARS-CoV-2 receptor, angiotensin-converting enzyme 2, is required for human endometrial stromal cell decidualization†. Biol Reprod. (2021) 104:336–43. doi: 10.1093/biolre/ioaa211
77. Marques FZ, Pringle KG, Conquest A, Hirst JJ, Markus MA, Sarris M, et al. Molecular characterization of renin-angiotensin system components in human intrauterine tissues and fetal membranes from vaginal delivery and cesarean section. Placenta. (2011) 32:214–21. doi: 10.1016/j.placenta.2010.12.006
78. Weatherbee BAT, Glover DM, Zernicka-Goetz M. Expression of SARS-CoV-2 receptor ACE2 and the protease TMPRSS2 suggests susceptibility of the human embryo in the first trimester. Open Biol. (2020) 10:200162. doi: 10.1098/rsob.200162
79. Valdés G, Neves LA, Anton L, Corthorn J, Chacón C, Germain AM, et al. Distribution of angiotensin-(1-7) and ACE2 in human placentas of normal and pathological pregnancies. Placenta. (2006) 27:200–7. doi: 10.1016/j.placenta.2005.02.015
80. Valdés G, Corthorn J, Bharadwaj MS, Joyner J, Schneider D, Brosnihan KB. Utero-placental expression of angiotensin-(1-7) and ACE2 in the pregnant guinea-pig. Reprod Biol Endocrinol. (2013) 11:5. doi: 10.1186/1477-7827-11-5
81. Li M, Chen L, Zhang J, Xiong C, Li X. The SARS-CoV-2 receptor ACE2 expression of maternal-fetal interface and fetal organs by single-cell transcriptome study. PLoS ONE. (2020) 15:e0230295. doi: 10.1371/journal.pone.0230295
82. Levy A, Yagil Y, Bursztyn M, Barkalifa R, Scharf S, Yagil C. ACE2 expression and activity are enhanced during pregnancy. Am J Physiol Regul Integr Comp Physiol. (2008) 295:R1953–61. doi: 10.1152/ajpregu.90592.2008
83. Morgan T, Craven C, Ward K. Human spiral artery renin-angiotensin system. Hypertension. (1998) 32:683–7. doi: 10.1161/01.HYP.32.4.683
84. Irani RA, Xia Y. Renin angiotensin signaling in normal pregnancy and preeclampsia. Semin Nephrol. (2011) 31:47–58. doi: 10.1016/j.semnephrol.2010.10.005
85. Irani RA, Xia Y. The functional role of the renin-angiotensin system in pregnancy and preeclampsia. Placenta. (2008) 29:763–71. doi: 10.1016/j.placenta.2008.06.011
86. Verdonk K, Visser W, Van Den Meiracker AH, Danser AH. The renin-angiotensin-aldosterone system in pre-eclampsia: the delicate balance between good and bad. Clin Sci (Lond). (2014) 126:537–44. doi: 10.1042/CS20130455
87. Merrill DC, Karoly M, Chen K, Ferrario CM, Brosnihan KB. Angiotensin-(1-7) in normal and preeclamptic pregnancy. Endocrine. (2002) 18:239–45. doi: 10.1385/ENDO:18:3:239
88. Bloise E, Zhang J, Nakpu J, Hamada H, Dunk CE, Li S, et al. Expression of severe acute respiratory syndrome coronavirus 2 cell entry genes, angiotensin-converting enzyme 2 and transmembrane protease serine 2, in the placenta across gestation and at the maternal-fetal interface in pregnancies complicated by preterm birth or preeclampsia. Am J Obstet Gynecol. (2021) 224:298.e1–e8. doi: 10.1016/j.ajog.2020.08.055
89. Neves LA, Stovall K, Joyner J, Valdés G, Gallagher PE, Ferrario CM, et al. ACE2 and ANG-(1-7) in the rat uterus during early and late gestation. Am J Physiol Regul Integr Comp Physiol. (2008) 294:R151–61. doi: 10.1152/ajpregu.00514.2007
90. Wang Y, Pringle KG, Sykes SD, Marques FZ, Morris BJ, Zakar T, et al. Fetal sex affects expression of renin-angiotensin system components in term human decidua. Endocrinology. (2012) 153:462–8. doi: 10.1210/en.2011-1316
91. Lu R, Zhao X, Li J, Niu P, Yang B, Wu H, et al. Genomic characterisation and epidemiology of 2019 novel coronavirus: implications for virus origins and receptor binding. Lancet. (2020) 395:565–74. doi: 10.1016/S0140-6736(20)30251-8
92. Samudrala PK, Kumar P, Choudhary K, Thakur N, Wadekar GS, Dayaramani R, et al. Virology, pathogenesis, diagnosis and in-line treatment of COVID-19. Eur J Pharmacol. (2020) 883:173375. doi: 10.1016/j.ejphar.2020.173375
93. Zhang YZ, Holmes EC, A. Genomic Perspective on the Origin and Emergence of SARS-CoV-2. Cell. (2020) 181:223–7. doi: 10.1016/j.cell.2020.03.035
94. Hu B, Guo H, Zhou P, Shi ZL. Characteristics of SARS-CoV-2 and COVID-19. Nat Rev Microbiol. (2021) 19:141–54. doi: 10.1038/s41579-020-00459-7
95. Wang MY, Zhao R, Gao LJ, Gao XF, Wang DP, Cao JM. SARS-CoV-2: structure, biology, and structure-based therapeutics development. Front Cell Infect Microbiol. (2020) 10:587269. doi: 10.3389/fcimb.2020.587269
96. Li W, Moore MJ, Vasilieva N, Sui J, Wong SK, Berne MA, et al. Angiotensin-converting enzyme 2 is a functional receptor for the SARS coronavirus. Nature. (2003) 426:450–4. doi: 10.1038/nature02145
97. Zhou P, Yang XL, Wang XG, Hu B, Zhang L, Zhang W, et al. A pneumonia outbreak associated with a new coronavirus of probable bat origin. Nature. (2020) 579:270–3. doi: 10.1038/s41586-020-2012-7
98. Shang J, Ye G, Shi K, Wan Y, Luo C, Aihara H, et al. Structural basis of receptor recognition by SARS-CoV-2. Nature. (2020) 581:221–4. doi: 10.1038/s41586-020-2179-y
99. Hoffmann M, Kleine-Weber H, Schroeder S, Krüger N, Herrler T, Erichsen S, et al. SARS-CoV-2 cell entry depends on ACE2 and TMPRSS2 and is blocked by a clinically proven protease inhibitor. Cell. (2020) 181:271–80.e8. doi: 10.1016/j.cell.2020.02.052
100. Stadnytskyi V, Bax CE, Bax A, Anfinrud P. The airborne lifetime of small speech droplets and their potential importance in SARS-CoV-2 transmission. Proc Natl Acad Sci U S A. (2020) 117:11875–7. doi: 10.1073/pnas.2006874117
101. Tang S, Mao Y, Jones RM, Tan Q, Ji JS, Li N, et al. Aerosol transmission of SARS-CoV-2? Evidence, prevention and control. Environ Int. (2020) 144:106039. doi: 10.1016/j.envint.2020.106039
102. Abd EW, Eassa SM, Metwally M, Al-Hraishawi H, Omar SR. SARS-CoV-2 transmission channels: a review of the literature. MEDICC Rev. (2020) 22:51–69. doi: 10.37757/MR2020.V22.N4.3
103. Chen Y, Chen L, Deng Q, Zhang G, Wu K, Ni L, et al. The presence of SARS-CoV-2 RNA in the feces of COVID-19 patients. J Med Virol. (2020) 92:833–40. doi: 10.1002/jmv.25825
104. Li D, Jin M, Bao P, Zhao W, Zhang S. Clinical characteristics and results of semen tests among men with coronavirus disease 2019. JAMA Netw Open. (2020) 3:e208292. doi: 10.1001/jamanetworkopen.2020.8292
105. Groß R, Conzelmann C, Müller JA, Stenger S, Steinhart K, Kirchhoff F, et al. Detection of SARS-CoV-2 in human breastmilk. Lancet. (2020) 395:1757–8. doi: 10.1016/S0140-6736(20)31181-8
106. Lauer SA, Grantz KH, Bi Q, Jones FK, Zheng Q, Meredith HR, et al. The incubation period of coronavirus disease 2019 (COVID-19) from publicly reported confirmed cases: estimation and application. Ann Intern Med. (2020) 172:577–82. doi: 10.7326/M20-0504
107. McAloon C, Collins Á, Hunt K, Barber A, Byrne AW, Butler F, et al. Incubation period of COVID-19: a rapid systematic review and meta-analysis of observational research. BMJ Open. (2020) 10:e039652. doi: 10.1136/bmjopen-2020-039652
108. Salzberger B, Buder F, Lampl B, Ehrenstein B, Hitzenbichler F, Holzmann T, et al. Epidemiology of SARS-CoV-2. Infection. (2021) 49:233–9. doi: 10.1007/s15010-020-01531-3
109. Singanayagam A, Patel M, Charlett A, Lopez Bernal J, Saliba V, Ellis J, et al. Duration of infectiousness and correlation with RT-PCR cycle threshold values in cases of COVID-19, England, January to May 2020. Euro Surveill. (2020) 25. doi: 10.2807/1560-7917.ES.2020.25.32.2001483
110. Walsh KA, Jordan K, Clyne B, Rohde D, Drummond L, Byrne P, et al. SARS-CoV-2 detection, viral load and infectivity over the course of an infection. J Infect. (2020) 81:357–71. doi: 10.1016/j.jinf.2020.06.067
111. Widders A, Broom A, Broom J. SARS-CoV-2: the viral shedding vs infectivity dilemma. Infect Dis Health. (2020) 25:210–5. doi: 10.1016/j.idh.2020.05.002
112. Cevik M, Bamford CGG, Ho A. COVID-19 pandemic-a focused review for clinicians. Clin Microbiol Infect. (2020) 26:842–7. doi: 10.1016/j.cmi.2020.04.023
113. Liu X, Liu C, Liu G, Luo W, Xia N. COVID-19: Progress in diagnostics, therapy and vaccination. Theranostics. (2020) 10:7821–35. doi: 10.7150/thno.47987
114. Guan WJ, Ni ZY, Hu Y, Liang WH, Ou CQ, He JX, et al. Clinical characteristics of coronavirus disease 2019 in China. N Engl J Med. (2020) 382:1708–20. doi: 10.1056/NEJMoa2002032
115. Adil MT, Rahman R, Whitelaw D, Jain V, Al-Taan O, Rashid F, et al. SARS-CoV-2 and the pandemic of COVID-19. Postgrad Med J. (2021) 97:110–6. doi: 10.1136/postgradmedj-2020-138386
116. Spinato G, Fabbris C, Polesel J, Cazzador D, Borsetto D, Hopkins C, et al. Alterations in smell or taste in mildly symptomatic outpatients with SARS-CoV-2 infection. Jama. (2020) 323:2089–90. doi: 10.1001/jama.2020.6771
117. Carfì A, Bernabei R, Landi F. Persistent symptoms in patients after acute COVID-19. Jama. (2020) 324:603–5. doi: 10.1001/jama.2020.12603
118. Halpin SJ, McIvor C, Whyatt G, Adams A, Harvey O, McLean L, et al. Postdischarge symptoms and rehabilitation needs in survivors of COVID-19 infection: a cross-sectional evaluation. J Med Virol. (2021) 93:1013–22. doi: 10.1002/jmv.26368
119. Berlin DA, Gulick RM, Martinez FJ. Severe COVID-19. N Engl J Med. (2020) 383:2451–60. doi: 10.1056/NEJMcp2009575
120. Xu Z, Shi L, Wang Y, Zhang J, Huang L, Zhang C, et al. Pathological findings of COVID-19 associated with acute respiratory distress syndrome. Lancet Respir Med. (2020) 8:420–2. doi: 10.1016/S2213-2600(20)30076-X
121. Calabrese F, Pezzuto F, Fortarezza F, Hofman P, Kern I, Panizo A, et al. Pulmonary pathology and COVID-19: lessons from autopsy. The experience of European Pulmonary Pathologists. Virchows Arch. (2020) 477:359–72. doi: 10.1007/s00428-020-02886-6
122. Ye Z, Zhang Y, Wang Y, Huang Z, Song B. Chest CT manifestations of new coronavirus disease 2019 (COVID-19): a pictorial review. Eur Radiol. (2020) 30:4381–9. doi: 10.1007/s00330-020-06801-0
123. Wan S, Li M, Ye Z, Yang C, Cai Q, Duan S, et al. CT manifestations and clinical characteristics of 1115 patients with coronavirus disease 2019 (COVID-19): a systematic review and meta-analysis. Acad Radiol. (2020) 27:910–21. doi: 10.1016/j.acra.2020.04.033
124. Lai CC, Ko WC, Lee PI, Jean SS, Hsueh PR. Extra-respiratory manifestations of COVID-19. Int J Antimicrob Agents. (2020) 56:106024. doi: 10.1016/j.ijantimicag.2020.106024
125. Magadum A, Kishore R. Cardiovascular Manifestations of COVID-19 Infection. Cells. (2020) 9. doi: 10.3390/cells9112508
126. Mao R, Qiu Y, He JS, Tan JY, Li XH, Liang J, et al. Manifestations and prognosis of gastrointestinal and liver involvement in patients with COVID-19: a systematic review and meta-analysis. Lancet Gastroenterol Hepatol. (2020) 5:667–78. doi: 10.1016/S2468-1253(20)30126-6
127. Orsini A, Corsi M, Santangelo A, Riva A, Peroni D, Foiadelli T, et al. Challenges and management of neurological and psychiatric manifestations in SARS-CoV-2 (COVID-19) patients. Neurol Sci. (2020) 41:2353–66. doi: 10.1007/s10072-020-04544-w
128. Rahi MS, Jindal V, Reyes SP, Gunasekaran K, Gupta R, Jaiyesimi I. Hematologic disorders associated with COVID-19: a review. Ann Hematol. (2021) 100:309–20. doi: 10.1007/s00277-020-04366-y
129. Ragab D, Salah Eldin H, Taeimah M, Khattab R, Salem R. The COVID-19 cytokine storm; what we know so far. Front Immunol. (2020) 11:1446. doi: 10.3389/fimmu.2020.01446
130. Felsenstein S, Herbert JA, McNamara PS, Hedrich CM. COVID-19: Immunology and treatment options. Clin Immunol. (2020) 215:108448. doi: 10.1016/j.clim.2020.108448
131. Blanco-Melo D, Nilsson-Payant BE, Liu WC, Uhl S, Hoagland D, Møller R, et al. Imbalanced Host Response to SARS-CoV-2 Drives Development of COVID-19. Cell. (2020) 181:1036–45.e9. doi: 10.1016/j.cell.2020.04.026
132. Keam S, Megawati D, Patel SK, Tiwari R, Dhama K, Harapan H. Immunopathology and immunotherapeutic strategies in severe acute respiratory syndrome coronavirus 2 infection. Rev Med Virol. (2020) 30:e2123. doi: 10.1002/rmv.2123
133. Melenotte C, Silvin A, Goubet AG, Lahmar I, Dubuisson A, Zumla A, et al. Immune responses during COVID-19 infection. Oncoimmunology. (2020) 9:1807836. doi: 10.1080/2162402X.2020.1807836
134. Wang J, Jiang M, Chen X, Montaner LJ. Cytokine storm and leukocyte changes in mild versus severe SARS-CoV-2 infection: Review of 3939 COVID-19 patients in China and emerging pathogenesis and therapy concepts. J Leukoc Biol. (2020) 108:17–41. doi: 10.1002/JLB.3COVR0520-272R
135. Wu Z, McGoogan JM. Characteristics of and important lessons from the coronavirus disease 2019 (COVID-19) Outbreak in China: summary of a report of 72 314 cases from the chinese center for disease control and prevention. Jama. (2020) 323:1239–42. doi: 10.1001/jama.2020.2648
136. Pollard CA, Morran MP, Nestor-Kalinoski AL. The COVID-19 pandemic: a global health crisis. Physiol Genomics. (2020) 52:549–57. doi: 10.1152/physiolgenomics.00089.2020
137. Yasuhara J, Kuno T, Takagi H, Sumitomo N. Clinical characteristics of COVID-19 in children: a systematic review. Pediatr Pulmonol. (2020) 55:2565–75. doi: 10.1002/ppul.24991
138. Zhou F, Yu T, Du R, Fan G, Liu Y, Liu Z, et al. Clinical course and risk factors for mortality of adult inpatients with COVID-19 in Wuhan, China: a retrospective cohort study. Lancet. (2020) 395:1054–62. doi: 10.1016/S0140-6736(20)30566-3
139. Zhang B, Zhou X, Qiu Y, Song Y, Feng F, Feng J, et al. Clinical characteristics of 82 cases of death from COVID-19. PLoS ONE. (2020) 15:e0235458. doi: 10.1371/journal.pone.0235458
140. Edler C, Schröder AS, Aepfelbacher M, Fitzek A, Heinemann A, Heinrich F, et al. Dying with SARS-CoV-2 infection-an autopsy study of the first consecutive 80 cases in Hamburg, Germany. Int J Legal Med. (2020) 134:1275–84. doi: 10.1007/s00414-020-02317-w
141. Petrilli CM, Jones SA, Yang J, Rajagopalan H, O'Donnell L, Chernyak Y, et al. Factors associated with hospital admission and critical illness among 5279 people with coronavirus disease 2019 in New York City: prospective cohort study. Bmj. (2020) 369:m1966. doi: 10.1136/bmj.m1966
142. de Leeuw AJM, Oude Luttikhuis MAM, Wellen AC, Müller C, Calkhoven CF. Obesity and its impact on COVID-19. J Mol Med. (2021) 99:1–17. doi: 10.1007/s00109-021-02072-4
143. Richardson S, Hirsch JS, Narasimhan M, Crawford JM, McGinn T, Davidson KW, et al. Presenting characteristics, comorbidities, and outcomes among 5700 patients hospitalized with COVID-19 in the New York City Area. Jama. (2020) 323:2052–9. doi: 10.1001/jama.2020.6775
144. Jin JM, Bai P, He W, Wu F, Liu XF, Han DM, et al. Gender differences in patients with COVID-19: focus on severity and mortality. Front Public Health. (2020) 8:152. doi: 10.3389/fpubh.2020.00152
145. Gebhard C, Regitz-Zagrosek V, Neuhauser HK, Morgan R, Klein SL. Impact of sex and gender on COVID-19 outcomes in Europe. Biol Sex Differ. (2020) 11:29. doi: 10.1186/s13293-020-00304-9
146. Raifman MA, Raifman JR. Disparities in the population at risk of severe illness from COVID-19 by race/ethnicity and income. Am J Prev Med. (2020) 59:137–9. doi: 10.1016/j.amepre.2020.04.003
147. Karmakar M, Lantz PM, Tipirneni R. Association of social and demographic factors with COVID-19 incidence and death rates in the US. JAMA Netw Open. (2021) 4:e2036462. doi: 10.1001/jamanetworkopen.2020.36462
148. Kumar A, Arora A, Sharma P, Anikhindi SA, Bansal N, Singla V, et al. Is diabetes mellitus associated with mortality and severity of COVID-19? A meta-analysis. Diabetes Metab Syndr. (2020) 14:535–45. doi: 10.1016/j.dsx.2020.04.044
149. Barron E, Bakhai C, Kar P, Weaver A, Bradley D, Ismail H, et al. Associations of type 1 and type 2 diabetes with COVID-19-related mortality in England: a whole-population study. Lancet Diabetes Endocrinol. (2020) 8:813–22. doi: 10.1016/S2213-8587(20)30272-2
150. Bode B, Garrett V, Messler J, McFarland R, Crowe J, Booth R, et al. Glycemic Characteristics and Clinical Outcomes of COVID-19 Patients Hospitalized in the United States. J Diabetes Sci Technol. (2020) 14:813–21. doi: 10.1177/1932296820924469
151. Holman N, Knighton P, Kar P, O'Keefe J, Curley M, Weaver A, et al. Risk factors for COVID-19-related mortality in people with type 1 and type 2 diabetes in England: a population-based cohort study. Lancet Diabetes Endocrinol. (2020) 8:823–33. doi: 10.1016/S2213-8587(20)30271-0
152. Zhu L, She ZG, Cheng X, Qin JJ, Zhang XJ, Cai J, et al. Association of Blood Glucose Control and Outcomes in Patients with COVID-19 and Pre-existing Type 2 Diabetes. Cell Metab. (2020) 31:1068–77.e3. doi: 10.1016/j.cmet.2020.04.021
153. Agarwal S, Schechter C, Southern W, Crandall JP, Tomer Y. Preadmission diabetes-specific risk factors for mortality in hospitalized patients with diabetes and coronavirus disease 2019. Diabetes Care. (2020) 43:2339–44. doi: 10.2337/dc20-1543
154. Cariou B, Hadjadj S, Wargny M, Pichelin M, Al-Salameh A, Allix I, et al. Phenotypic characteristics and prognosis of inpatients with COVID-19 and diabetes: the CORONADO study. Diabetologia. (2020) 63:1500–15. doi: 10.1007/s00125-020-05180-x
155. Yu B, Li C, Sun Y, Wang DW. Insulin treatment is associated with increased mortality in patients with COVID-19 and type 2 diabetes. Cell Metab. (2021) 33:65–77.e2. doi: 10.1016/j.cmet.2020.11.014
156. Zhao Q, Meng M, Kumar R, Wu Y, Huang J, Lian N, et al. The impact of COPD and smoking history on the severity of COVID-19: a systemic review and meta-analysis. J Med Virol. (2020) 92:1915–21. doi: 10.1002/jmv.25889
157. Portolés J, Marques M, López-Sánchez P, de Valdenebro M, Muñez E, Serrano ML, et al. Chronic kidney disease and acute kidney injury in the COVID-19 Spanish outbreak. Nephrol Dial Transplant. (2020) 35:1353–61. doi: 10.1093/ndt/gfaa189
158. Liu C, Zhao Y, Okwan-Duodu D, Basho R, Cui X. COVID-19 in cancer patients: risk, clinical features, and management. Cancer Biol Med. (2020) 17:519–27. doi: 10.20892/j.issn.2095-3941.2020.0289
159. Kovalic AJ, Satapathy SK, Thuluvath PJ. Prevalence of chronic liver disease in patients with COVID-19 and their clinical outcomes: a systematic review and meta-analysis. Hepatol Int. (2020) 14:612–20. doi: 10.1007/s12072-020-10078-2
160. Singh S, Khan A. Clinical characteristics and outcomes of coronavirus disease 2019 among patients with preexisting liver disease in the United States: a multicenter research network study. Gastroenterology. (2020) 159:768–71.e3. doi: 10.1053/j.gastro.2020.04.064
161. Stawiski EW, Diwanji D, Suryamohan K, Gupta R, Fellouse FA, Sathirapongsasuti JF, et al. Human ACE2 receptor polymorphisms predict SARS-CoV-2 susceptibility. bioRxiv. (2020). doi: 10.1101/2020.04.07.024752
162. Hussain M, Jabeen N, Raza F, Shabbir S, Baig AA, Amanullah A, et al. Structural variations in human ACE2 may influence its binding with SARS-CoV-2 spike protein. J Med Virol. (2020) 92:1580–6. doi: 10.1002/jmv.25832
163. Asselta R, Paraboschi EM, Mantovani A, Duga S. ACE2 and TMPRSS2 variants and expression as candidates to sex and country differences in COVID-19 severity in Italy. Aging. (2020) 12:10087–98. doi: 10.18632/aging.103415
164. Nguyen A, David JK, Maden SK, Wood MA, Weeder BR, Nellore A, et al. Human leukocyte antigen susceptibility map for severe acute respiratory syndrome coronavirus 2. J Virol. (2020) 94. doi: 10.1128/JVI.00510-20
165. Wang F, Huang S, Gao R, Zhou Y, Lai C, Li Z, et al. Initial whole-genome sequencing and analysis of the host genetic contribution to COVID-19 severity and susceptibility. Cell Discov. (2020) 6:83. doi: 10.1038/s41421-020-00231-4
166. Zhao J, Yang Y, Huang H, Li D, Gu D, Lu X, et al. Relationship between the ABO Blood Group and the COVID-19 Susceptibility. Clin Infect Dis. (2020). doi: 10.1101/2020.03.11.20031096
167. Liu Y, Häussinger L, Steinacker JM, Dinse-Lambracht A. Association between the dynamics of the COVID-19 epidemic and ABO blood type distribution. Epidemiol Infect. (2021) 149:e19. doi: 10.1017/S0950268821000030
168. Patanavanich R, Glantz SA. Smoking is associated with COVID-19 progression: a meta-analysis. Nicotine Tob Res. (2020) 22:1653–6. doi: 10.1093/ntr/ntaa082
169. Huang W, Zhou H, Hodgkinson C, Montero A, Goldman D, Chang SL. Network meta-analysis on the mechanisms underlying alcohol augmentation of COVID-19 pathologies. Alcohol Clin Exp Res. (2021) 45:675–88. doi: 10.1111/acer.14573
170. Bailey KL, Samuelson DR, Wyatt TA. Alcohol use disorder: a pre-existing condition for COVID-19? Alcohol. (2021) 90:11–7. doi: 10.1016/j.alcohol.2020.10.003
171. Hamer M, Kivimäki M, Gale CR, Batty GD. Lifestyle risk factors, inflammatory mechanisms, and COVID-19 hospitalization: A community-based cohort study of 387,109 adults in UK. Brain Behav Immun. (2020) 87:184–7. doi: 10.1016/j.bbi.2020.05.059
172. da Silveira MP, da Silva Fagundes KK, Bizuti MR, Starck É, Rossi RC, de Resende ESDT. Physical exercise as a tool to help the immune system against COVID-19: an integrative review of the current literature. Clin Exp Med. (2021) 21:15–28. doi: 10.1007/s10238-020-00650-3
173. Schiebinger L. Women's health and clinical trials. J Clin Invest. (2003) 112:973–7. doi: 10.1172/JCI19993
174. Shields KE, Lyerly AD. Exclusion of pregnant women from industry-sponsored clinical trials. Obstet Gynecol. (2013) 122:1077–81. doi: 10.1097/AOG.0b013e3182a9ca67
175. Beery AK, Zucker I. Sex bias in neuroscience and biomedical research. Neurosci Biobehav Rev. (2011) 35:565–72. doi: 10.1016/j.neubiorev.2010.07.002
176. Westerman S, Wenger N. Gender differences in atrial fibrillation: a review of epidemiology, management, and outcomes. Curr Cardiol Rev. (2019) 15:136–44. doi: 10.2174/1573403X15666181205110624
177. Dudley JP, Lee NT. Disparities in age-specific morbidity and mortality from SARS-CoV-2 in China and the Republic of Korea. Clin Infect Dis. (2020) 71:863–5. doi: 10.1093/cid/ciaa354
178. Alghamdi IG, Hussain, II, Almalki SS, Alghamdi MS, Alghamdi MM, El-Sheemy MA. The pattern of Middle East respiratory syndrome coronavirus in Saudi Arabia: a descriptive epidemiological analysis of data from the Saudi Ministry of Health. Int J Gen Med. (2014) 7:417–23. doi: 10.2147/IJGM.S67061
179. Jia N, Feng D, Fang LQ, Richardus JH, Han XN, Cao WC, et al. Case fatality of SARS in mainland China and associated risk factors. Trop Med Int Health. (2009) 14:21–7. doi: 10.1111/j.1365-3156.2008.02147.x
180. Karlberg J, Chong DS, Lai WY. Do men have a higher case fatality rate of severe acute respiratory syndrome than women do? Am J Epidemiol. (2004) 159:229–31. doi: 10.1093/aje/kwh056
181. Chan-Yeung M, Xu RH. SARS: epidemiology. Respirology. (2003) 8:S9–14. doi: 10.1046/j.1440-1843.2003.00518.x
182. Viveiros A, Rasmuson J, Vu J, Mulvagh SL, Yip CYY, Norris CM, et al. Sex differences in COVID-19: candidate pathways, genetics of ACE2, and sex hormones. Am J Physiol Heart Circ Physiol. (2021) 320:H296–h304. doi: 10.1152/ajpheart.00755.2020
183. Schurz H, Salie M, Tromp G, Hoal EG, Kinnear CJ, Möller M. The X chromosome and sex-specific effects in infectious disease susceptibility. Hum Genomics. (2019) 13:2. doi: 10.1186/s40246-018-0185-z
184. Disteche CM, Berletch JB. X-chromosome inactivation and escape. J Genet. (2015) 94:591–9. doi: 10.1007/s12041-015-0574-1
185. Tukiainen T, Villani AC, Yen A, Rivas MA, Marshall JL, Satija R, et al. Landscape of X chromosome inactivation across human tissues. Nature. (2017) 550:244–8. doi: 10.1038/nature24265
186. Tipnis SR, Hooper NM, Hyde R, Karran E, Christie G, Turner AJ, et al. human homolog of angiotensin-converting enzyme. Cloning and functional expression as a captopril-insensitive carboxypeptidase. J Biol Chem. (2000) 275:33238–43. doi: 10.1074/jbc.M002615200
187. Chen K, Bi J, Su Y, Chappell MC, Rose JC. Sex-specific changes in renal angiotensin-converting enzyme and angiotensin-converting enzyme 2 gene expression and enzyme activity at birth and over the first year of life. Reprod Sci. (2016) 23:200–10. doi: 10.1177/1933719115597760
188. Wang G, Lai FM, Lai KB, Chow KM, Kwan CH, Li KT, et al. Discrepancy between intrarenal messenger RNA and protein expression of ACE and ACE2 in human diabetic nephropathy. Am J Nephrol. (2009) 29:524–31. doi: 10.1159/000185629
189. Swärd P, Edsfeldt A, Reepalu A, Jehpsson L, Rosengren BE, Karlsson MK. Age and sex differences in soluble ACE2 may give insights for COVID-19. Crit Care. (2020) 24:221. doi: 10.1186/s13054-020-02942-2
190. Sama IE, Ravera A, Santema BT, van Goor H, Ter Maaten JM, Cleland JGF, et al. Circulating plasma concentrations of angiotensin-converting enzyme 2 in men and women with heart failure and effects of renin-angiotensin-aldosterone inhibitors. Eur Heart J. (2020) 41:1810–7. doi: 10.1093/eurheartj/ehaa373
191. Kornilov SA, Lucas I, Jade K, Dai CL, Lovejoy JC, Magis AT. Plasma levels of soluble ACE2are associated with sex, Metabolic Syndrome, and its biomarkers in a large cohort, pointing to a possible mechanism for increased severity in COVID-19. Crit Care. (2020) 24:452. doi: 10.1186/s13054-020-03141-9
192. Rahman MM, Hasan M, Ahmed A. Potential detrimental role of soluble ACE2 in severe COVID-19 comorbid patients. Rev Med Virol. (2021) 31:1–2. doi: 10.2139/ssrn.3729704
193. Libert C, Dejager L, Pinheiro I. The X chromosome in immune functions: when a chromosome makes the difference. Nat Rev Immunol. (2010) 10:594–604. doi: 10.1038/nri2815
194. Klein SL, Flanagan KL. Sex differences in immune responses. Nat Rev Immunol. (2016) 16:626–38. doi: 10.1038/nri.2016.90
195. Invernizzi P, Pasini S, Selmi C, Gershwin ME, Podda M. Female predominance and X chromosome defects in autoimmune diseases. J Autoimmun. (2009) 33:12–6. doi: 10.1016/j.jaut.2009.03.005
196. Billi AC, Kahlenberg JM, Gudjonsson JE. Sex bias in autoimmunity. Curr Opin Rheumatol. (2019) 31:53–61. doi: 10.1097/BOR.0000000000000564
197. Akira S, Uematsu S, Takeuchi O. Pathogen recognition and innate immunity. Cell. (2006) 124:783–801. doi: 10.1016/j.cell.2006.02.015
198. Diebold SS, Kaisho T, Hemmi H, Akira S, Reis e Sousa C. Innate antiviral responses by means of TLR7-mediated recognition of single-stranded. RNA Science. (2004) 303:1529–31. doi: 10.1126/science.1093616
199. Souyris M, Cenac C, Azar P, Daviaud D, Canivet A, Grunenwald S, et al. TLR7 escapes X chromosome inactivation in immune cells. Sci Immunol. (2018) 3. doi: 10.1126/sciimmunol.aap8855
200. Jaillon S, Berthenet K, Garlanda C. Sexual dimorphism in innate immunity. Clin Rev Allergy Immunol. (2019) 56:308–21. doi: 10.1007/s12016-017-8648-x
201. Berghöfer B, Frommer T, Haley G, Fink L, Bein G, Hackstein H. TLR7 ligands induce higher IFN-alpha production in females. J Immunol. (2006) 177:2088–96. doi: 10.4049/jimmunol.177.4.2088
202. Torcia MG, Nencioni L, Clemente AM, Civitelli L, Celestino I, Limongi D, et al. Sex differences in the response to viral infections: TLR8 and TLR9 ligand stimulation induce higher IL10 production in males. PLoS ONE. (2012) 7:e39853. doi: 10.1371/journal.pone.0039853
203. Klein SL, Marriott I, Fish EN. Sex-based differences in immune function and responses to vaccination. Trans R Soc Trop Med Hyg. (2015) 109:9–15. doi: 10.1093/trstmh/tru167
204. Garg R, Agrawal P, Gautam A, Pursnani N, Agarwal M, Agarwal A, et al. COVID-19 outcomes in postmenopausal and perimenopausal females: is estrogen hormone attributing to gender differences? J Midlife Health. (2020) 11:250–6. doi: 10.4103/jmh.jmh_287_20
205. Ding T, Zhang J, Wang T, Cui P, Chen Z, Jiang J, et al. Potential influence of menstrual status and sex hormones on female SARS-CoV-2 infection: a cross-sectional study from multicentre in Wuhan, China. Clin Infect Dis. (2021) 9:e240–e248. doi: 10.1093/cid/ciaa1022
206. Pradhan A, Olsson PE. Sex differences in severity and mortality from COVID-19: are males more vulnerable? Biol Sex Differ. (2020) 11:53. doi: 10.1186/s13293-020-00330-7
207. Calabrese EJ. Estrogen and related compounds: biphasic dose responses. Crit Rev Toxicol. (2001) 31:503–15. doi: 10.1080/20014091111785
208. Gaskins AJ, Wilchesky M, Mumford SL, Whitcomb BW, Browne RW, Wactawski-Wende J, et al. Endogenous reproductive hormones and C-reactive protein across the menstrual cycle: the BioCycle study. Am J Epidemiol. (2012) 175:423–31. doi: 10.1093/aje/kwr343
209. Stein B, Yang MX. Repression of the interleukin-6 promoter by estrogen receptor is mediated by NF-kappa B and C/EBP beta. Mol Cell Biol. (1995) 15:4971–9. doi: 10.1128/MCB.15.9.4971
210. Florian M, Magder S. Estrogen decreases TNF-alpha and oxidized LDL induced apoptosis in endothelial cells. Steroids. (2008) 73:47–58. doi: 10.1016/j.steroids.2007.08.010
211. Straub RH. The complex role of estrogens in inflammation. Endocr Rev. (2007) 28:521–74. doi: 10.1210/er.2007-0001
212. Robinson DP, Hall OJ, Nilles TL, Bream JH, Klein SL. 17β-estradiol protects females against influenza by recruiting neutrophils and increasing virus-specific CD8 T cell responses in the lungs. J Virol. (2014) 88:4711–20. doi: 10.1128/JVI.02081-13
213. Peretz J, Pekosz A, Lane AP, Klein SL. Estrogenic compounds reduce influenza A virus replication in primary human nasal epithelial cells derived from female, but not male, donors. Am J Physiol Lung Cell Mol Physiol. (2016) 310:L415–25. doi: 10.1152/ajplung.00398.2015
214. Chedraui P, Pérez-López FR. The severe acute respiratory syndrome due to coronavirus 2 (SARS-CoV-2) infection and the climacteric woman. Climacteric. (2020) 23:525–7. doi: 10.1080/13697137.2020.1837547
215. Seeland U, Coluzzi F, Simmaco M, Mura C, Bourne PE, Heiland M, et al. Evidence for treatment with estradiol for women with SARS-CoV-2 infection. BMC Med. (2020) 18:369. doi: 10.1186/s12916-020-01851-z
216. Neuhauser HK, Adler C, Rosario AS, Diederichs C, Ellert U. Hypertension prevalence, awareness, treatment and control in Germany 1998 and 2008-11. J Hum Hypertens. (2015) 29:247–53. doi: 10.1038/jhh.2014.82
217. Kautzky-Willer A, Harreiter J, Pacini G. Sex and gender differences in risk, pathophysiology and complications of type 2 diabetes mellitus. Endocr Rev. (2016) 37:278–316. doi: 10.1210/er.2015-1137
218. Ntritsos G, Franek J, Belbasis L, Christou MA, Markozannes G, Altman P, et al. Gender-specific estimates of COPD prevalence: a systematic review and meta-analysis. Int J Chron Obstruct Pulmon Dis. (2018) 13:1507–14. doi: 10.2147/COPD.S146390
219. Cai H. Sex difference and smoking predisposition in patients with COVID-19. Lancet Respir Med. (2020) 8:e20. doi: 10.1016/S2213-2600(20)30117-X
220. Erol A, Karpyak VM. Sex and gender-related differences in alcohol use and its consequences: contemporary knowledge and future research considerations. Drug Alcohol Depend. (2015) 156:1–13. doi: 10.1016/j.drugalcdep.2015.08.023
221. Wennman H, Pietilä A, Rissanen H, Valkeinen H, Partonen T, Mäki-Opas T, et al. Gender, age and socioeconomic variation in 24-hour physical activity by wrist-worn accelerometers: the FinHealth 2017 survey. Sci Rep. (2019) 9:6534. doi: 10.1038/s41598-019-43007-x
222. Zhang Q, Cong M, Wang N, Li X, Zhang H, Zhang K, et al. Association of angiotensin-converting enzyme 2 gene polymorphism and enzymatic activity with essential hypertension in different gender: a case-control study. Medicine. (2018) 97:e12917. doi: 10.1097/MD.0000000000012917
223. Bukowska A, Spiller L, Wolke C, Lendeckel U, Weinert S, Hoffmann J, et al. Protective regulation of the ACE2/ACE gene expression by estrogen in human atrial tissue from elderly men. Exp Biol Med (Maywood). (2017) 242:1412–23. doi: 10.1177/1535370217718808
224. Gupte M, Thatcher SE, Boustany-Kari CM, Shoemaker R, Yiannikouris F, Zhang X, et al. Angiotensin converting enzyme 2 contributes to sex differences in the development of obesity hypertension in C57BL/6 mice. Arterioscler Thromb Vasc Biol. (2012) 32:1392–9. doi: 10.1161/ATVBAHA.112.248559
225. Wang Y, Shoemaker R, Thatcher SE, Batifoulier-Yiannikouris F, English VL, Cassis LA. Administration of 17β-estradiol to ovariectomized obese female mice reverses obesity-hypertension through an ACE2-dependent mechanism. Am J Physiol Endocrinol Metab. (2015) 308:E1066–75. doi: 10.1152/ajpendo.00030.2015
226. De Paoli M, Werstuck GH. Role of estrogen in type 1 and type 2 diabetes mellitus: a review of clinical and preclinical data. Can J Diabetes. (2020) 44:448–52. doi: 10.1016/j.jcjd.2020.01.003
227. Xu Y, Lin J, Wang S, Xiong J, Zhu Q. Combined estrogen replacement therapy on metabolic control in postmenopausal women with diabetes mellitus. Kaohsiung J Med Sci. (2014) 30:350–61. doi: 10.1016/j.kjms.2014.03.002
228. Stelzig KE, Canepa-Escaro F, Schiliro M, Berdnikovs S, Prakash YS, Chiarella SE. Estrogen regulates the expression of SARS-CoV-2 receptor ACE2 in differentiated airway epithelial cells. Am J Physiol Lung Cell Mol Physiol. (2020) 318:L1280–l1. doi: 10.1152/ajplung.00153.2020
229. Tan EK, Tan EL. Alterations in physiology and anatomy during pregnancy. Best Pract Res Clin Obstet Gynaecol. (2013) 27:791–802. doi: 10.1016/j.bpobgyn.2013.08.001
230. Kohlhepp LM, Hollerich G, Vo L, Hofmann-Kiefer K, Rehm M, Louwen F, et al. Physiological changes during pregnancy. Anaesthesist. (2018) 67:383–96. doi: 10.1007/s00101-018-0437-2
231. Thornburg KL, Jacobson SL, Giraud GD, Morton MJ. Hemodynamic changes in pregnancy. Semin Perinatol. (2000) 24:11–4. doi: 10.1016/S0146-0005(00)80047-6
232. Witry SW. Pulmonary edema in pregnancy. J Obstet Gynecol Neonatal Nurs. (1992) 21:177–84. doi: 10.1111/j.1552-6909.1992.tb02254.x
233. Lee SY, Chien DK, Huang CH, Shih SC, Lee WC, Chang WH. Dyspnea in pregnancy. Taiwan J Obstet Gynecol. (2017) 56:432–6. doi: 10.1016/j.tjog.2017.04.035
234. Thornton P, Douglas J. Coagulation in pregnancy. Best Pract Res Clin Obstet Gynaecol. (2010) 24:339–52. doi: 10.1016/j.bpobgyn.2009.11.010
235. Di Toro F, Gjoka M, Di Lorenzo G, De Santo D, De Seta F, Maso G, et al. Impact of COVID-19 on maternal and neonatal outcomes: a systematic review and meta-analysis. Clin Microbiol Infect. (2021) 27:36–46. doi: 10.1016/j.cmi.2020.10.007
236. Yang Z, Wang M, Zhu Z, Liu Y. Coronavirus disease 2019 (COVID-19) and pregnancy: a systematic review. J Maternal-Fetal Neonatal Med. (2020) 1–4. doi: 10.1080/14767058.2020.1759541
237. Breslin N, Baptiste C, Gyamfi-Bannerman C, Miller R, Martinez R, Bernstein K, et al. Coronavirus disease 2019 infection among asymptomatic and symptomatic pregnant women: two weeks of confirmed presentations to an affiliated pair of New York City hospitals. Am J Obstet Gynecol MFM. (2020) 2:100118. doi: 10.1016/j.ajogmf.2020.100118
238. Allotey J, Stallings E, Bonet M, Yap M, Chatterjee S, Kew T, et al. Clinical manifestations, risk factors, and maternal and perinatal outcomes of coronavirus disease 2019 in pregnancy: living systematic review and meta-analysis. BMJ. (2020) 370:m3320. doi: 10.1136/bmj.m3320
239. Collin J, Byström E, Carnahan A, Ahrne M. Public Health Agency of Sweden's Brief Report: Pregnant and postpartum women with severe acute respiratory syndrome coronavirus 2 infection in intensive care in Sweden. Acta Obstet Gynecol Scand. (2020) 99:819–22. doi: 10.1111/aogs.13901
240. Lokken EM, Walker CL, Delaney S, Kachikis A, Kretzer NM, Erickson A, et al. Clinical characteristics of 46 pregnant women with a severe acute respiratory syndrome coronavirus 2 infection in Washington State. Am J Obstet Gynecol. (2020) 223:911.e1–e14. doi: 10.1016/j.ajog.2020.05.031
241. Torres-Torres J, Martinez-Portilla RJ, Espino YSS, Estrada-Gutierrez G, Solis-Paredes JM, Villafan-Bernal JR, et al. Comorbidities, poverty and social vulnerability as risk factors for mortality in pregnant women with confirmed SARS-CoV-2 infection: analysis of 13 062 positive pregnancies including 176 maternal deaths in Mexico. Ultrasound Obstet Gynecol. (2022) 59:76–82. doi: 10.1002/uog.24797
242. Eberle C, James-Todd T, Stichling S. SARS-CoV-2 in diabetic pregnancies: a systematic scoping review. BMC Pregnancy Childbirth. (2021) 21:573. doi: 10.1186/s12884-021-03975-3
243. Sculli MA, Formoso G, Sciacca L. COVID-19 vaccination in pregnant and lactating diabetic women. Nutr Metab Cardiovasc Dis. (2021) 31:2151–5. doi: 10.1016/j.numecd.2021.04.012
244. Roberts J, Pritchard AL, Treweeke AT, Rossi AG, Brace N, Cahill P, et al. Why is COVID-19 more severe in patients with diabetes? The role of angiotensin-converting enzyme 2, endothelial dysfunction and the immunoinflammatory system. Front Cardiovasc Med. (2020) 7:629933. doi: 10.3389/fcvm.2020.629933
245. Lim S, Bae JH, Kwon HS, Nauck MA. COVID-19 and diabetes mellitus: from pathophysiology to clinical management. Nat Rev Endocrinol. (2021) 17:11–30. doi: 10.1038/s41574-020-00435-4
246. Chi J, Gong W, Gao Q. Clinical characteristics and outcomes of pregnant women with COVID-19 and the risk of vertical transmission: a systematic review. Arch Gynecol Obstet. (2021) 303:337–45. doi: 10.1007/s00404-020-05889-5
247. Salem D, Katranji F, Bakdash T. COVID-19 infection in pregnant women: Review of maternal and fetal outcomes. Int J Gynaecol Obstet. (2021) 152:291–8. doi: 10.1002/ijgo.13533
248. Arora N, Sadovsky Y, Dermody TS, Coyne CB. Microbial vertical transmission during human pregnancy. Cell Host Microbe. (2017) 21:561–7. doi: 10.1016/j.chom.2017.04.007
249. Hughes BL, Gyamfi-Bannerman C. Diagnosis and antenatal management of congenital cytomegalovirus infection. Am J Obstet Gynecol. (2016) 214:B5–b11. doi: 10.1016/j.ajog.2016.02.042
250. Adams Waldorf KM, McAdams RM. Influence of infection during pregnancy on fetal development. Reproduction. (2013) 146:R151–62. doi: 10.1530/REP-13-0232
251. Lamont RF, Sobel JD, Vaisbuch E, Kusanovic JP, Mazaki-Tovi S, Kim SK, et al. Parvovirus B19 infection in human pregnancy. Bjog. (2011) 118:175–86. doi: 10.1111/j.1471-0528.2010.02749.x
252. Karimi-Zarchi M, Neamatzadeh H, Dastgheib SA, Abbasi H, Mirjalili SR, Behforouz A, et al. Vertical transmission of coronavirus disease 19 (COVID-19) from infected pregnant mothers to neonates: a review. Fetal Pediatr Pathol. (2020) 39:246–50. doi: 10.1080/15513815.2020.1747120
253. Capobianco G, Saderi L, Aliberti S, Mondoni M, Piana A, Dessole F, et al. COVID-19 in pregnant women: a systematic review and meta-analysis. Eur J Obstet Gynecol Reprod Biol. (2020) 252:543–58. doi: 10.1016/j.ejogrb.2020.07.006
254. Zeng H, Xu C, Fan J, Tang Y, Deng Q, Zhang W, et al. Antibodies in infants born to mothers with COVID-19 pneumonia. Jama. (2020) 323:1848–9. doi: 10.1001/jama.2020.4861
255. Dong L, Tian J, He S, Zhu C, Wang J, Liu C, et al. Possible vertical transmission of SARS-CoV-2 from an infected mother to her newborn. Jama. (2020) 323:1846–8. doi: 10.1001/jama.2020.4621
256. Palmeira P, Quinello C, Silveira-Lessa AL, Zago CA, Carneiro-Sampaio M. IgG placental transfer in healthy and pathological pregnancies. Clin Dev Immunol. (2012) 13. doi: 10.1155/2012/985646
257. Ben-Hur H, Gurevich P, Elhayany A, Avinoach I, Schneider DF, Zusman I. Transport of maternal immunoglobulins through the human placental barrier in normal pregnancy and during inflammation. Int J Mol Med. (2005) 16:401–7. doi: 10.3892/ijmm.16.3.401
258. Hecht JL, Quade B, Deshpande V, Mino-Kenudson M, Ting DT, Desai N, et al. SARS-CoV-2 can infect the placenta and is not associated with specific placental histopathology: a series of 19 placentas from COVID-19-positive mothers. Mod Pathol. (2020) 33:2092–103. doi: 10.1038/s41379-020-0639-4
259. Chen W, Yuan P, Yang M, Yan Z, Kong S, Yan J, et al. SARS-CoV-2 Entry Factors: ACE2 and TMPRSS2 Are Expressed in Peri-Implantation Embryos and the Maternal-Fetal Interface. Engineering (Beijing). (2020) 6:1162–9. doi: 10.1016/j.eng.2020.07.013
260. Pique-Regi R, Romero R, Tarca AL, Luca F, Xu Y, Alazizi A, et al. Does the human placenta express the canonical cell entry mediators for SARS-CoV-2? Elife. (2020) 9. doi: 10.7554/eLife.58716
261. Henarejos-Castillo I, Sebastian-Leon P, Devesa-Peiro A, Pellicer A, Diaz-Gimeno P. SARS-CoV-2 infection risk assessment in the endometrium: viral infection-related gene expression across the menstrual cycle. Fertil Steril. (2020) 114:223–32. doi: 10.1016/j.fertnstert.2020.06.026
262. Zang R, Gomez Castro MF, McCune BT, Zeng Q, Rothlauf PW, Sonnek NM, et al. TMPRSS2 and TMPRSS4 promote SARS-CoV-2 infection of human small intestinal enterocytes. Sci Immunol. (2020) 5. doi: 10.1126/sciimmunol.abc3582
263. Egloff C, Vauloup-Fellous C, Picone O, Mandelbrot L, Roques P. Evidence and possible mechanisms of rare maternal-fetal transmission of SARS-CoV-2. J Clin Virol. (2020) 128:104447. doi: 10.1016/j.jcv.2020.104447
264. Wang W, Xu Y, Gao R, Lu R, Han K, Wu G, et al. Detection of SARS-CoV-2 in different types of clinical specimens. Jama. (2020) 323:1843–4. doi: 10.1001/jama.2020.3786
265. Facchetti F, Bugatti M, Drera E, Tripodo C, Sartori E, Cancila V, et al. SARS-CoV2 vertical transmission with adverse effects on the newborn revealed through integrated immunohistochemical, electron microscopy and molecular analyses of Placenta. EBioMedicine. (2020) 59:102951. doi: 10.1016/j.ebiom.2020.102951
266. Valdespino-Vázquez MY, Helguera-Repetto CA, León-Juárez M, Villavicencio-Carrisoza O, Flores-Pliego A, Moreno-Verduzco ER, et al. Fetal and placental infection with SARS-CoV-2 in early pregnancy. J Med Virol. (2021) 93:4480–4487. doi: 10.1002/jmv.26965
267. Shende P, Gaikwad P, Gandhewar M, Ukey P, Bhide A, Patel V, et al. Persistence of SARS-CoV-2 in the first trimester placenta leading to transplacental transmission and fetal demise from an asymptomatic mother. Hum Reprod. (2021) 36:899–906. doi: 10.1093/humrep/deaa367
268. Resta L, Vimercati A, Cazzato G, Mazzia G, Cicinelli E, Colagrande A, et al. SARS-CoV-2 and placenta: new insights and perspectives. Viruses. (2021) 13. doi: 10.3390/v13050723
269. Shanes ED, Mithal LB, Otero S, Azad HA, Miller ES, Goldstein JA. Placental pathology in COVID-19. Am J Clin Pathol. (2020) 154:23–32. doi: 10.1093/ajcp/aqaa089
270. Sharps MC, Hayes DJL, Lee S, Zou Z, Brady CA, Almoghrabi Y, et al. A structured review of placental morphology and histopathological lesions associated with SARS-CoV-2 infection. Placenta. (2020) 101:13–29. doi: 10.1016/j.placenta.2020.08.018
271. Azinheira Nobrega Cruz N, Stoll D, Casarini DE, Bertagnolli M. Role of ACE2 in pregnancy and potential implications for COVID-19 susceptibility. Clin Sci (Lond). (2021) 135:1805–24. doi: 10.1042/CS20210284
272. Knöfler M, Haider S, Saleh L, Pollheimer J, Gamage T, James J. Human placenta and trophoblast development: key molecular mechanisms and model systems. Cell Mol Life Sci. (2019) 76:3479–96. doi: 10.1007/s00018-019-03104-6
273. Hsu AL, Guan M, Johannesen E, Stephens AJ, Khaleel N, Kagan N, et al. Placental SARS-CoV-2 in a pregnant woman with mild COVID-19 disease. J Med Virol. (2021) 93:1038–44. doi: 10.1002/jmv.26386
274. Jaiswal N, Puri M, Agarwal K, Singh S, Yadav R, Tiwary N, et al. COVID-19 as an independent risk factor for subclinical placental dysfunction. Eur J Obstet Gynecol Reprod Biol. (2021) 259:7–11. doi: 10.1016/j.ejogrb.2021.01.049
275. Xia YQ, Zhao KN, Zhao AD, Zhu JZ, Hong HF, Wang YL, et al. Associations of maternal upper respiratory tract infection/influenza during early pregnancy with congenital heart disease in offspring: evidence from a case-control study and meta-analysis. BMC Cardiovasc Disord. (2019) 19:277. doi: 10.1186/s12872-019-1206-0
276. Joma M, Fovet CM, Seddiki N, Gressens P, Laforge M. COVID-19 and Pregnancy: Vertical Transmission and Inflammation Impact on Newborns. Vaccines. (2021) 9. doi: 10.3390/vaccines9040391
277. Lebel C, MacKinnon A, Bagshawe M, Tomfohr-Madsen L, Giesbrecht G. Elevated depression and anxiety symptoms among pregnant individuals during the COVID-19 pandemic. J Affect Disord. (2020) 277:5–13. doi: 10.1016/j.jad.2020.07.126
278. Wu Y, Zhang C, Liu H, Duan C, Li C, Fan J, et al. Perinatal depressive and anxiety symptoms of pregnant women during the coronavirus disease 2019 outbreak in China. Am J Obstet Gynecol. (2020) 223:240.e1–e9. doi: 10.1016/j.ajog.2020.05.009
279. Toth B, Jeschke U, Rogenhofer N, Scholz C, Würfel W, Thaler CJ, et al. Recurrent miscarriage: current concepts in diagnosis and treatment. J Reprod Immunol. (2010) 85:25–32. doi: 10.1016/j.jri.2009.12.006
280. Nepomnaschy PA, Welch KB, McConnell DS, Low BS, Strassmann BI, England BG. Cortisol levels and very early pregnancy loss in humans. Proc Natl Acad Sci U S A. (2006) 103:3938–42. doi: 10.1073/pnas.0511183103
281. Lucas DN, Bamber JH. Pandemics and maternal health: the indirect effects of COVID-19. Anaesthesia. (2021) 76:69–75. doi: 10.1111/anae.15408
282. Yirmiya K, Yakirevich-Amir N, Preis H, Lotan A, Atzil S, Reuveni I. Women's depressive symptoms during the COVID-19 pandemic: the role of pregnancy. Int J Environ Res Public Health. (2021) 18. doi: 10.3390/ijerph18084298
283. Pariente G, Wissotzky Broder O, Sheiner E, Lanxner Battat T, Mazor E, Yaniv Salem S, et al. Risk for probable post-partum depression among women during the COVID-19 pandemic. Arch Womens Ment Health. (2020) 23:767–73. doi: 10.1007/s00737-020-01075-3
284. D'Souza R, Ashraf R, Rowe H, Zipursky J, Clarfield L, Maxwell C, et al. Pregnancy and COVID-19: pharmacologic considerations. Ultrasound Obstet Gynecol. (2021) 57:195–203. doi: 10.1002/uog.23116
285. Li L, Wang X, Wang R, Hu Y, Jiang S, Lu X. Antiviral agent therapy optimization in special populations of COVID-19 patients. Drug Des Devel Ther. (2020) 14:3001–13. doi: 10.2147/DDDT.S259058
286. Rasmussen SA, Kelley CF, Horton JP, Jamieson DJ. Coronavirus disease 2019 (COVID-19) vaccines and pregnancy: what obstetricians need to know. Obstet Gynecol. (2021) 137:408–14. doi: 10.1097/AOG.0000000000004290
287. Giles ML, Gunatilaka A, Palmer K, Sharma K, Roach V. Alignment of national COVID-19 vaccine recommendations for pregnant and lactating women. Bull World Health Organ. (2021) 99:739–46. doi: 10.2471/BLT.21.286644
288. Shamshirsaz AA, Hessami K, Morain S, Afshar Y, Nassr A, Arian SE, et al. Intention to receive COVID-19 vaccine during pregnancy: a systematic review and meta-analysis. Am J Perinatol. (2021) 39. doi: 10.1055/a-1674-6120 [Epub ahead of print].
289. Shook LL, Fallah PN, Silberman JN, Edlow AG. COVID-19 vaccination in pregnancy and lactation: current research and gaps in understanding. Front Cell Infect Microbiol. (2021) 11:735394. doi: 10.3389/fcimb.2021.735394
290. Shimabukuro TT, Kim SY, Myers TR, Moro PL, Oduyebo T, Panagiotakopoulos L, et al. Preliminary findings of mRNA Covid-19 vaccine safety in pregnant persons. N Engl J Med. (2021) 384:2273–82. doi: 10.1056/NEJMoa2104983
291. Collier AY, McMahan K, Yu J, Tostanoski LH, Aguayo R, Ansel J, et al. Immunogenicity of COVID-19 mRNA vaccines in pregnant and lactating women. Jama. (2021) 325:2370–80. doi: 10.1001/jama.2021.7563
292. Beharier O, Plitman Mayo R, Raz T, Nahum Sacks K, Schreiber L, Suissa-Cohen Y, et al. Efficient maternal to neonatal transfer of antibodies against SARS-CoV-2 and BNT162b2 mRNA COVID-19 vaccine. J Clin Invest. (2021) 131. doi: 10.1172/JCI154834
293. Gray KJ, Bordt EA, Atyeo C, Deriso E, Akinwunmi B, Young N, et al. Coronavirus disease 2019 vaccine response in pregnant and lactating women: a cohort study. Am J Obstetr Gynecol. (2021) 225:303.e1–e17. doi: 10.1016/j.ajog.2021.03.023
Keywords: COVID-19, SARS-CoV-2, pregnancy, vertical transmission, decidualization, ACE2, women
Citation: Liu A, Raja xavier J, Singh Y, Brucker SY and Salker MS (2022) Molecular and Physiological Aspects of SARS-CoV-2 Infection in Women and Pregnancy. Front. Glob. Womens Health 3:756362. doi: 10.3389/fgwh.2022.756362
Received: 10 August 2021; Accepted: 01 February 2022;
Published: 24 February 2022.
Edited by:
Lakshmi Surya Prabha Manem, Dr. NTR University of Health Sciences, IndiaReviewed by:
Daisy Motta-Santos, Federal University of Minas Gerais, BrazilKatie Harris, University of New South Wales, Australia
Copyright © 2022 Liu, Raja xavier, Singh, Brucker and Salker. This is an open-access article distributed under the terms of the Creative Commons Attribution License (CC BY). The use, distribution or reproduction in other forums is permitted, provided the original author(s) and the copyright owner(s) are credited and that the original publication in this journal is cited, in accordance with accepted academic practice. No use, distribution or reproduction is permitted which does not comply with these terms.
*Correspondence: Madhuri S. Salker, bWFkaHVyaS5zYWxrZXJAbWVkLnVuaS10dWViaW5nZW4uZGU=