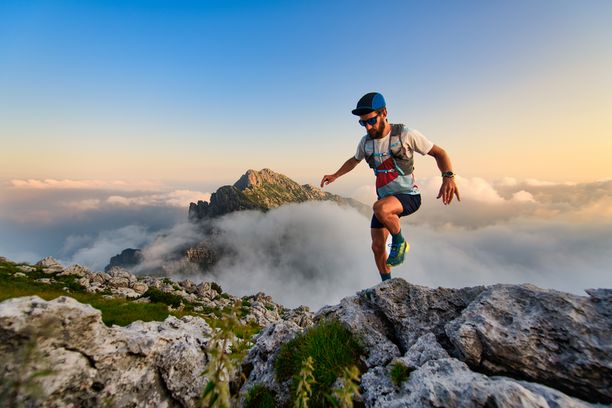
94% of researchers rate our articles as excellent or good
Learn more about the work of our research integrity team to safeguard the quality of each article we publish.
Find out more
ORIGINAL RESEARCH article
Front. Geochem., 07 October 2024
Sec. Biogeochemistry
Volume 2 - 2024 | https://doi.org/10.3389/fgeoc.2024.1436488
The glacier ice algae Ancylonema alaskanum and Ancylonema nordenskiöldii grow in harsh dynamic environments on bare ice surfaces. In these environments, they contribute to the continuous darkening of the ice surface, which in turn accelerates the ice melt. However, investigation into their adaptation and resilience in these environments is necessary in order to understand their robustness and potential for increasing the intensity of blooms. In this study, it was examined how variations in environmental parameters such as pH, salinity, light and temperature impacted the photophysiology of the glacier ice algae during a bloom in Greenland. Through in situ incubations and pulse-amplitude-modulation (PAM) fluorometric measurements, the photophysiological responses of algal cells were assessed. Results suggest that light intensity significantly influences glacier ice algae photophysiology, with cells exhibiting better performance (in terms of a higher theoretical maximum light coefficient and maximum quantum efficiency) under lower light intensity. Moreover, while light emerges as the primary driver of photophysiology, glacier ice algae demonstrate tolerance to a broad range of pH and temperatures four times higher than those experienced during Greenland’s summer.
Glacier ice algae are known to occur in the top centimeters of surface ice in many high-latitude and -altitude areas, such as the Arctic (Yallop et al., 2012; Remias et al., 2012), Alps (Di Mauro et al., 2020), Himalayas (Yoshimura et al., 1997) and Antarctica (Ling and Seppelt, 1990). These algae belong to the Zygnematophyceae (Streptophyta) and are distinct from both snow and sea ice algae. Along the western margin of the Greenland ice sheet (GrIS), extensive algal blooms (
Glacier ice algae, being non-motile (Remias et al., 2009; Dial et al., 2018), must cope with the changes in the environment in which they live. Inhabiting some of the harshest environments on Earth, they must endure extreme conditions promoted by high and fluctuating light intensities, fluctuating pH levels, varying salinity, and temperature extremes (Williamson et al., 2020; Shetty et al., 2019). These environmental factors pose significant challenges to their survival and productivity (Shetty et al., 2019; Baker and Rosenqvist, 2004; Mott and Berry, 1986). For example, during the months of constant light in the Arctic summer season, glacier ice algae are subjected to intense solar radiation, with photosynthetic active radiation (PAR) that can exceed 1700
While light serves as the primary energy source for photosynthesis in glacier algae, excessive radiation levels can induce photoinhibition and increase reactive oxygen species, which can damage DNA and proteins within the cell (Aro et al., 1993; Rippin et al., 2019; Williamson et al., 2020). A reduction in incoming light has been shown to alter the photophysiology of glacier ice algae (Williamson et al., 2020). Similarly, the external pH around algal cells plays a role in their photosynthetic performance (Yadav and Singh, 2021). Fluctuations in pH and salinity can disrupt vital cellular processes for algal growth and survival by, for example, damaging the photosystem or affecting the enzymatic processes involved in photosynthesis (Shetty et al., 2019; Mott and Berry, 1986). Additionally, temperature extremes influence enzymatic reactions and metabolic rates, thereby impacting algal productivity (Baker and Rosenqvist, 2004).
Despite the ecological significance of glacier ice algae, substantial gaps persist in understanding their responses to environmental perturbations, particularly regarding their photophysiology and productivity. Bridging this knowledge gap is crucial for predicting future dynamics in glacier ice ecosystems and assessing their vulnerability to ongoing environmental changes. A common and nondestructive method for assessing the fitness of plants and algal cells involves measuring chlorophyll fluorescence to estimate photosynthetic performance (Baker and Rosenqvist, 2004; Baker, 2008). This technique can be implemented using pulse-amplitude-modulation (PAM) fluorometry, which allows for in vivo examination of photosystem II (PSII). The electron transport through PSII serves as an indicator of the photosynthetic efficiency of a cell. Additionally, a reduced quantum yield, as indicated by the maximum quantum efficient (
As a result, the aims of this study were to investigate the impact of light, pH, salinity, and temperature on the photophysiology of glacier ice algae via in situ incubations in Greenland, which allows for variable control, and provide valuable insights into their adaptive mechanisms and resilience in the face of changing environmental conditions.
Surface ice sampling was conducted at an elevation of 617 m a.s.l.
Figure 1. An overview of four in situ incubation experiments that manipulated pH, salinity, light intensity and temperature. The ‘control’ samples represent the prevailing environmental conditions at the study site (A), an example of a field incubation for the pH experiment (B), and Copernicus Sentinel-2 optical satellite imagery of the fieldwork site (denoted by a blue star) in southern Greenland (61°06′05″N, 46°50′53″W) (C) during 2021 (The Danish Agency for Data Supply and Infrastructure, 2024).
In order to comprehensively examine the impact of environmental factors on the photophysiology of the microbial community, a series of controlled incubation experiments were conducted, manipulating pH, salinity, light and temperature. For each parameter, a gradient of five points was systematically created by transferring aliquots of 650 mL from one of the four bags to fresh 2,000-mL Whirl-Pak® bags (Figure 1A). The environmental parameter of interest was then amended in all of the fresh bags except for one per replicate, which served as an unmodified “control”, representing the prevailing environmental conditions at the study site.
After determining that the pH of the surface ice was 6.7
Lastly, a temperature gradient (5°C [control], 10°C, 15°C, 20°C, and 25°C) was created by placing the triplicate bags (n = 3) in heated water baths, where the temperature was manually controlled by adding hot water. Samples incubated at 5°C were considered to be controls, as this was the approximate air temperature (Fausto et al., 2021). Due to the challenges associated with manually maintaining the desired temperatures, the temperature experiments were conducted under low light conditions
The photophysiology of the microbial community was assessed immediately within each sample at the end of its incubation period (48 h or 4 h) using the methods described in the following section. Prior to initiating the incubation experiments for pH, salinity, and light, baseline photophysiological measurements were taken for additional control samples that had been prepared using the same methods to establish a reference point after the melting process (
The photophysiology of the microbial community was assessed using pulse-amplitude-modulation techniques following methods described by Perkins et al. (2006). Specifically, variable chlorophyll fluorescence was measured in 3-mL aliquots using a WaterPAM fluorometer combined with a blue emitter/detector cuvette system, with continuous stirring to prevent settling (Walz GmBH, Effeltrich, DE). All samples were dark-adapted for 30 min prior to rapid light curve assessments (RLCs), which consisted of nine 20-s steps of incrementally increasing actinic light intensities that ranged from 33 to 1,306
Statistical analysis of the data was performed in R version 4.0.2 using methods outlined by Crawley (2007). Assumptions of normality and homogeneity of variance were assessed through Shapiro-Wilk and Levene’s tests, respectively. One-way analysis of variance (ANOVA) was used to assess differences in photophysiological parameters across each of the gradients. Post-hoc pairwise comparisons were performed using Tukey’s HSD test when significant variations were detected. When parametric assumptions were met, the Student’s t-test was used to compare means between the
Although, a series of incubation experiments aimed to investigate the individual effects of pH, salinity, light, and temperature on the photophysiology of glacier ice algae in south Greenland, the rapid light curve assessments, performed using PAM fluorometry, were insufficient to fully saturate PSII reaction centers within the microbial community (Supplementary Figures S2, S3). Consequently, it was not possible to derive values for
The photophysiological responses of the microbial community to variations in pH, salinity, light, and temperature appeared to differ among the four incubation experiments (Figures 2, 3). Within each specific experiment, both
Figure 2. Maximal quantum yields (
Figure 3. Light utilization efficiencies
In the context of salinity, a 5‰ increase above ambient conditions resulted in a decline in
The photophysiology of the microbial community remained largely stable in response to variations in pH, with
The photophysiology of the microbial community was assessed in all control samples. This evaluation occurred immediately after the melting process (
Figure 4. Maximal quantum yields (
While glacier ice algae have been observed on glaciers and ice sheets globally (Takeuchi et al., 1998; Takeuchi et al., 2006; Takeuchi and Kohshima, 2004; Remias et al., 2012; Lutz et al., 2018; Di Mauro et al., 2020), very few attempts have been made to examine their photophysiology. Where investigations have been conducted, they have almost exclusively focused on the bulk photophysiology of the whole ice community (Yallop et al., 2012; Williamson et al., 2020; McCutcheon et al., 2021; Halbach et al., 2022; Perini et al., 2023; Doting et al., 2024). However, the photophysiology of both the unicellular (A. alaskana) and filamentous (A. nordenskiöldii) algae were analysed separately in a study by Procházková et al. (2021). Photophysiological data from selected studies that utilised PAM fluorometry (Williamson et al., 2020; McCutcheon et al., 2021; Halbach et al., 2022; Doting et al., 2024) have shown that the
Table 1. Overview of photophysiological parameters from other studies on glacier ice algae based on PAM measurements. All samples were without any treatment. The numbers are the average reported from each study.
From this study, it was determined that light history and intensity prior to and during the experiments exert a greater influence on the photophysiology of glacier ice algae. Three observations in this study supporting this statement: 1) the absolute high
The
In this study, no significant differences were identified for the
In addition to growth across a broad range of pH, glacier ice algae were also able to withstand increasing temperatures. No significant differences were observed between the increased temperatures (10°C–25°C) and the control (5°C). However, this study was only conducted over a period of 4 h (Figures 2D, 3D). The algae naturally experience a fluctuation in temperature during a day with temperatures fluctuating between 1.5°C at night and 6° during the summer at the field site (Fausto et al., 2021). The maximum temperature for the summer of 2021 at the field camp was 6.21°C (Fausto et al., 2021), indicating that, for at least a short period, the glacier ice algae are still actively photosynthesizing at a temperature four times higher than the maximum air temperature at the study site. Growth and high photosynthetic performance at high temperatures have also been observed in both Arctic and Antarctic microalgae, suggesting that many polar microalgae are psychrotolerant rather than psychrophilic (Chen et al., 2012; Teoh et al., 2004; Stibal and Elster, 2005). The glacier ice algae, however, was strongly impacted by high salinity. Increasing salt concentrations are a known abiotic stressor, where elevated levels of reactive oxygen species interfere with photosynthesis by damaging photosystem I (PSI) and weakening of PSII (Shetty et al., 2019). Furthermore, transcriptomic data from the green microalgae Chlamydomonas reinhardtii have shown that downregulation of PSI light-harvesting complex genes leads to reduced photosynthetic performance in the cells (Shetty et al., 2019). Some bacterial and algal species have a cross-tolerance between salt stress and stress from freezing since those stressors are both related to osmotic changes within the cell (Wilson et al., 2012; Schmid et al., 2009; Tanaka et al., 2001; Raymond et al., 2020). However, in this study, the
This study was conducted using in situ incubations with a single condition change to examine its affect on the photophysiology of glacier ice algae. With fresh cultures of glacier ice algae (Remias and Procházková, 2023; Jensen et al., 2023), it would be possible to conduct a similar incubation study in a more controlled environment for an extended duration, allowing for the investigation of prolonged effects, such as temperature variations on photophysiology. Furthermore, laboratory studies with these cultures could enable the exploration of interactive effects, where multiple environmental parameters, such as increasing light and temperature, are varied simultaneously to better simulate and understand their combined impact on the algae.
In this study, it was demonstrated that glacier ice algal photophysiology is highly influenced by solar irradiance while performing well under a wide range of other physicochemical conditions. Solar irradiance significantly impacts the photophysiology and fitness of glacier ice algae, potentially serving as the primary driver of photophysiological variability under natural conditions. Despite their sensitivity to light, as expected from a photosynthetic organism, and increasing salt conditions, glacier ice algae were able to sustain in situ electron transport under a broad range of pH and temperature conditions. Based on the incubation experiments under different temperature conditions, it was shown that the glacier ice algae may not be truly psychrophilic but rather psychrotolerant. However, these experiments were only conducted for a short period of time and thus, it is possible that more pronounced differences in photophysiology under different temperature conditions could emerge over an extended duration. Despite this, it is argued that the adaptation of glacier ice algae to such a broad range of physico-chemical conditions is advantageous, considering their non-motile nature and the dynamic environment of the ice surface, which can undergo rapid changes over short periods.
The raw data supporting the conclusions of this article will be made available by the authors, without undue reservation.
MJ: Conceptualization, Data curation, Formal Analysis, Investigation, Methodology, Validation, Visualization, Writing–original draft, Writing–review and editing. TT-J: Data curation, Formal Analysis, Methodology, Validation, Writing–original draft, Writing–review and editing. MT: Funding acquisition, Project administration, Writing–original draft, Writing–review and editing. LB: Funding acquisition, Project administration, Writing–original draft, Writing–review and editing. AA: Conceptualization, Funding acquisition, Project administration, Supervision, Writing–original draft, Writing–review and editing.
The author(s) declare that financial support was received for the research, authorship, and/or publication of this article. The study was financially supported by the European Research Council Synergy Grant DEEP PURPLE under the European Union’s Horizon 2020 Research and Innovation Programme (grant agreement no. 856416).
The authors would like to thank the 2021 Deep Purple team for their support in the field, Christopher J Williamson for his guidance on processing PAM fluorometry data, and Jonas K Andersen for his assistance with producing the site map and conducting spectrometric measurements. The authors would also like to thank Hans Jakobsen for lending the spectrophotometer.
The authors declare that the research was conducted in the absence of any commercial or financial relationships that could be construed as a potential conflict of interest.
The author(s) declared that they were an editorial board member of Frontiers, at the time of submission. This had no impact on the peer review process and the final decision.
All claims expressed in this article are solely those of the authors and do not necessarily represent those of their affiliated organizations, or those of the publisher, the editors and the reviewers. Any product that may be evaluated in this article, or claim that may be made by its manufacturer, is not guaranteed or endorsed by the publisher.
The Supplementary Material for this article can be found online at: https://www.frontiersin.org/articles/10.3389/fgeoc.2024.1436488/full#supplementary-material
Aro, E. M., Virgin, I., and Andersson, B. (1993). Photoinhibition of Photosystem II. Inactivation, protein damage and turnover. Biochimica Biophysica Acta (BBA) - Bioenergetics 1143, 113–134. doi:10.1016/0005-2728(93)90134-2
Baker, N. R. (2008). Chlorophyll fluorescence: a probe of photosynthesis in vivo. Annu. Rev. Plant Biol. 59, 89–113. doi:10.1146/annurev.arplant.59.032607.092759
Baker, N. R., and Rosenqvist, E. (2004). Applications of chlorophyll fluorescence can improve crop production strategies: an examination of future possibilities. J. Exp. Bot. 55, 1607–1621. doi:10.1093/jxb/erh196
Bamber, J. L., Westaway, R. M., Marzeion, B., and Wouters, B. (2018). The land ice contribution to sea level during the satellite era. Environ. Res. Lett. 13, 1–21. doi:10.1088/1748-9326/aadb2c
Berggren, S. (1871). Alger från Grönlands inlandis. Öfversigt af kongl. Vetenskaps-akademiens Förhandlingar, 293–297.
Björkman, O., and Demmig, B. (1987). Photon yield of O2 evolution and chlorophyll fluorescence characteristics at 77 K among vascular plants of diverse origins. Planta 170, 489–504. doi:10.1007/BF00402983
Chen, Z., He, C., and Hu, H. (2012). Temperature responses of growth, photosynthesis, fatty acid and nitrate reductase in Antarctic and temperate Stichococcus. Extremophiles 16, 127–133. doi:10.1007/s00792-011-0412-1
Cook, J. M., Tedstone, A. J., Williamson, C., McCutcheon, J., Hodson, A. J., Dayal, A., et al. (2020). Glacier algae accelerate melt rates on the south-western Greenland Ice Sheet. Cryosphere 14, 309–330. doi:10.5194/tc-14-309-2020
Dial, R. J., Ganey, G. Q., and Skiles, S. M. K. (2018). What color should glacier algae be? An ecological role for red carbon in the cryosphere. FEMS Microbiol. Ecol. 94, 1–9. doi:10.1093/femsec/fiy007
Di Mauro, B., Garzonio, R., Baccolo, G., Franzetti, A., Pittino, F., Leoni, B., et al. (2020). Glacier algae foster ice-albedo feedback in the European Alps. Sci. Rep. 10, 4739. doi:10.1038/s41598-020-61762-0
Doting, E. L., Jensen, M. B., Peter, E. K., Ellegaard-Jensen, L., Tranter, M., Benning, L. G., et al. (2024). The exometabolome of microbial communities inhabiting bare ice surfaces on the southern Greenland Ice Sheet. Environ. Microbiol. 26, e16574–13doi. doi:10.1111/1462-2920.16574
Eilers, P. H., and Peeters, J. C. (1988). A model for the relationship between light intensity and the rate of photosynthesis in phytoplankton. Ecol. Model. 42, 199–215. doi:10.1016/0304-3800(88)90057-9
Fausto, R. S., van As, D., Mankoff, K. D., Vandecrux, B., Citterio, M., Ahlstrøm, A. P., et al. (2021). Programme for monitoring of the Greenland ice sheet (promice) automatic weather station data. Earth Syst. Sci. Data 13, 3819–3845. doi:10.5194/essd-13-3819-2021
Halbach, L., Chevrollier, L.-A., Doting, E. L., Cook, J. M., Jensen, M. B., Benning, L. G., et al. (2022). Pigment signatures of algal communities and their implications for glacier surface darkening. Sci. Rep. 12, 17643. doi:10.1038/s41598-022-22271-4
IPCC (2023). “Summary for policymakers,” in Climate change 2023: synthesis report. contribution of working groups I, II and III to the sixth assessment report of the intergovernmental panel on climate change, Editor Core Writing Team, H. Lee, and J. Romero (Geneva, Switzerland: IPCC), pp. 1–34. doi:10.59327/IPCC/AR6-9789291691647.001
Jaarsma, A. H., Sipes, K., Zervas, A., Jiménez, F. C., Ellegaard-Jensen, L., Thøgersen, M. S., et al. (2023). Exploring microbial diversity in Greenland Ice Sheet supraglacial habitats through culturing-dependent and -independent approaches. FEMS Microbiol. Ecol. 99, fiad119–16. doi:10.1093/femsec/fiad119
Jensen, M. B., Perini, L., Halbach, L., Jakobsen, H., Haraguchi, L., Ribeiro, S., et al. (2023). The dark art of cultivating glacier ice algae. Bot. Lett., 1–10. doi:10.1080/23818107.2023.2248235
Komárek, O., and Komárek, J. (2001). Contribution to the taxonomy and ecology of green cryosestic algae in the summer season 1995-96 at King George Island, S. Shetland Islands. Nova Hedwig. Beih. 123, 121–140.
Ling, H., and Seppelt, R. (1990). Snow algae of the Windmill Islands, continental Antarctica. Mesotaenium berggrenii (Zygnematales, Chlorophyta) the alga of grey snow. Antarct. Sci. 2, 143–148. doi:10.1017/S0954102090000189
Lutz, S., McCutcheon, J., McQuaid, J. B., and Benning, L. G. (2018). The diversity of ice algal communities on the Greenland Ice Sheet as revealed by oligotyping. Microb. genomics 4, 0001599–e210. doi:10.1099/mgen.0.000159
Masojídek, J., Torzillo, G., and Koblížek, M. (2013). Photosynthesis in microalgae. Handb. Microalgal Cult., 21–36. doi:10.1002/9781118567166.ch2
McCutcheon, J., Lutz, S., Williamson, C., Cook, J. M., Tedstone, A. J., Vanderstraeten, A., et al. (2021). Mineral phosphorus drives glacier algal blooms on the Greenland Ice Sheet. Nat. Commun. 12, 570. doi:10.1038/s41467-020-20627-w
Mott, K. A., and Berry, J. A. (1986). Effects of pH on activity and activation of ribulose 1,5-bisphosphate carboxylase at air level CO 2. Plant Physiol. 82, 77–82. doi:10.1104/pp.82.1.77
Mouginot, J., Rignot, E., Bjørk, A. A., van den Broeke, M., Millan, R., Morlighem, M., et al. (2019). Forty-six years of Greenland Ice Sheet mass balance from 1972 to 2018. Proc. Natl. Acad. Sci. 116, 9239–9244. doi:10.1073/pnas.1904242116
Perini, L., Gostinčar, C., Likar, M., Frisvad, J. C., Kostanjšek, R., Nicholes, M., et al. (2023). Interactions of fungi and algae from the Greenland ice sheet. Microb. Ecol. 86, 282–296. doi:10.1007/s00248-022-02033-5
Perkins, R. G., Mouget, J. L., Lefebvre, S., and Lavaud, J. (2006). Light response curve methodology and possible implications in the application of chlorophyll fluorescence to benthic diatoms. Mar. Biol. 149, 703–712. doi:10.1007/s00227-005-0222-z
Peter, E. K., Jaeger, C., Lisec, J., Peters, R. S., Mourot, R., Rossel, P., et al. (2024). Endometabolic profiling of pigmented glacier ice algae on the Greenland Ice Sheet. Metabolomics 20, 98. doi:10.1007/s11306-024-02147-6
Procházková, L., Řezanka, T., Nedbalová, L., and Remias, D. (2021). Unicellular versus filamentous: The glacial alga ancylonema alaskana comb. et stat. nov. and its ecophysiological relatedness to ancylonema nordenskioeldii (zygnematophyceae, streptophyta). Microorganisms 9, 1103. doi:10.3390/microorganisms9051103
Raymond, J. A., Morgan-Kiss, R., and Stahl-Rommel, S. (2020). Glycerol is an osmoprotectant in two antarctic Chlamydomonas species from an ice-covered saline lake and is synthesized by an unusual bidomain enzyme. Front. Plant Sci. 11, 1259–1268. doi:10.3389/fpls.2020.01259
Remias, D., Holzinger, A., Aigner, S., and Lütz, C. (2011). Ecophysiology and ultrastructure of ancylonema nordenskiöldii (zygnematales, streptophyta), causing brown ice on glaciers in svalbard (high arctic). Polar Biol. 35, 899–908. doi:10.1007/s00300-011-1135-6
Remias, D., Holzinger, A., and Lütz, C. (2009). Physiology, ultrastructure and habitat of the ice alga Mesotaenium berggrenii (Zygnemaphyceae, Chlorophyta) from glaciers in the European Alps. Phycologia 48, 302–312. doi:10.2216/08-13.1
Remias, D., and Procházková, L. (2023). The first cultivation of the glacier ice alga Ancylonema alaskanum (Zygnematophyceae, Streptophyta): differences in morphology and photophysiology of field vs laboratory strain cells. J. Glaciol. 69, 1080–1084. doi:10.1017/jog.2023.22
Remias, D., Schwaiger, S., Aigner, S., Leya, T., Stuppner, H., and Lütz, C. (2012). Characterization of an UV- and VIS-absorbing, purpurogallin-derived secondary pigment new to algae and highly abundant in Mesotaenium berggrenii (Zygnematophyceae, Chlorophyta), an extremophyte living on glaciers. FEMS Microbiol. Ecol. 79, 638–648. doi:10.1111/j.1574-6941.2011.01245.x
Rippin, M., Pichrtová, M., Arc, E., Kranner, I., Becker, B., and Holzinger, A. (2019). Metatranscriptomic and metabolite profiling reveals vertical heterogeneity within a Zygnema green algal mat from Svalbard (High Arctic). Environ. Microbiol. 21, 4283–4299. doi:10.1111/1462-2920.14788
Schmid, B., Klumpp, J., Raimann, E., Loessner, M. J., Stephan, R., and Tasara, T. (2009). Role of cold shock proteins in growth of Listeria monocytogenes under cold and osmotic stress conditions. Appl. Environ. Microbiol. 75, 1621–1627. doi:10.1128/AEM.02154-08
Shetty, P., Gitau, M. M., and Maróti, G. (2019). Salinity stress responses and adaptation mechanisms in eukaryotic green microalgae. Cells 8, 1657–1716. doi:10.3390/cells8121657
Shimada, R., Takeuchi, N., and Aoki, T. (2016). Inter-annual and geographical variations in the extent of bare ice and dark ice on the Greenland ice sheet derived from MODIS satellite images. Front. Earth Sci. 4, 1–10. doi:10.3389/feart.2016.00043
Stibal, M., and Elster, J. (2005). Growth and morphology variation as a response to changing environmental factors in two Arctic species of Raphidonema (Trebouxiophyceae) from snow and soil. Polar Biol. 28, 558–567. doi:10.1007/s00300-004-0709-y
Stibal, M., Elster, J., Šabacká, M., and Kaštovská, K. (2007). Seasonal and diel changes in photosynthetic activity of the snow alga Chlamydomonas nivalis (Chlorophyceae) from Svalbard determined by pulse amplitude modulation fluorometry. FEMS Microbiol. Ecol. 59, 265–273. doi:10.1111/j.1574-6941.2006.00264.x
Takeuchi, N. (2001). The altitudinal distribution of snow algae on an Alaska glacier (Gulkana Glacier in the Alaska Range). Hydrol. Process. 15, 3447–3459. doi:10.1002/hyp.1040
Takeuchi, N., and Kohshima, S. (2004). A snow algal community on tyndall glacier in the southern patagonia icefield, Chile. Arct. Antarct. Alp. Res. 36, 92–99. doi:10.1657/1523-0430(2004)036[0092:asacot]2.0.co;2
Takeuchi, N., Kohshima, S., and Fujita, K. (1998). Snow algae in Himalyan glaciers, Glacier AX010 East Nepal: relationship with glacier summer mass balance. Bullettin Glaciol. Res. 16, 43–50.
Takeuchi, N., Uetake, J., Fujita, K., Aizen, V. B., and Nikitin, S. D. (2006). A snow algal community on Akkem glacier in the Russian Altai mountains. Ann. Glaciol. 43, 378–384. doi:10.3189/172756406781812113
Tanaka, S., Ikeda, K., and Miyasaka, H. (2001). Enhanced tolerance against salt-stress and freezing-stress of Escherichia coli cells expressing algal bbc1 gene. Curr. Microbiol. 42, 173–177. doi:10.1007/s002840010199
Teoh, M. L., Chu, W. L., Marchant, H., and Phang, S. M. (2004). Influence of culture temperature on the growth, biochemical composition and fatty acid profiles of six Antarctic microalgae. J. Appl. Phycol. 16, 421–430. doi:10.1007/s10811-004-5502-3
Williamson, C. J., Anesio, A. M., Cook, J., Tedstone, A., Poniecka, E., Holland, A., et al. (2018). Ice algal bloom development on the surface of the Greenland Ice Sheet. FEMS Microbiol. Ecol. 94, fiy025–10. doi:10.1093/femsec/fiy025
Williamson, C. J., Cook, J., Tedstone, A., Yallop, M., McCutcheon, J., Poniecka, E., et al. (2020). Algal photophysiology drives darkening and melt of the Greenland Ice Sheet. Proc. Natl. Acad. Sci. 117, 5694–5705. doi:10.1073/pnas.1918412117
Williamson, C. J., Turpin-Jelfs, T., Nicholes, M. J., Yallop, M. L., Anesio, A. M., and Tranter, M. (2021). Macro-nutrient stoichiometry of glacier algae from the southwestern margin of the Greenland ice sheet. Front. Plant Sci. 12, 673614–673618. doi:10.3389/fpls.2021.673614
Wilson, S. L., Frazer, C., Cumming, B. F., Nuin, P. A., and Walker, V. K. (2012). Cross-tolerance between osmotic and freeze-thaw stress in microbial assemblages from temperate lakes. FEMS Microbiol. Ecol. 82, 405–415. doi:10.1111/j.1574-6941.2012.01404.x
Yadav, N., and Singh, D. P. (2021). Photosynthetic efficiency and compositional alterations in microalgae Chlorella vulgaris in response to changes in the pH condition. Vegetos 34, 119–126. doi:10.1007/s42535-021-00186-1
Yallop, M. L., Anesio, A. M., Perkins, R. G., Cook, J., Telling, J., Fagan, D., et al. (2012). Photophysiology and albedo-changing potential of the ice algal community on the surface of the Greenland ice sheet. ISME J. 6, 2302–2313doi. doi:10.1038/ismej.2012.107
Keywords: glacier ice algae, Greenland ice sheet, ancylonema, photophysiology, microalgae, arctic, adaptation, stress
Citation: Jensen MB, Turpin-Jelfs T, Tranter M, Benning LG and Anesio AM (2024) Photophysiological response of glacier ice algae to abiotic stressors. Front. Geochem. 2:1436488. doi: 10.3389/fgeoc.2024.1436488
Received: 22 May 2024; Accepted: 16 September 2024;
Published: 07 October 2024.
Edited by:
Yiliang Li, The University of Hong Kong, Hong Kong SAR, ChinaReviewed by:
July Ziret Florez, Universidad de Playa Ancha, ChileCopyright © 2024 Jensen, Turpin-Jelfs, Tranter, Benning and Anesio. This is an open-access article distributed under the terms of the Creative Commons Attribution License (CC BY). The use, distribution or reproduction in other forums is permitted, provided the original author(s) and the copyright owner(s) are credited and that the original publication in this journal is cited, in accordance with accepted academic practice. No use, distribution or reproduction is permitted which does not comply with these terms.
*Correspondence: Marie Bolander Jensen, bWFiakBlbnZzLmF1LmRr; Alexandre M. Anesio, YW1hQGVudnMuYXUuZGs=
Disclaimer: All claims expressed in this article are solely those of the authors and do not necessarily represent those of their affiliated organizations, or those of the publisher, the editors and the reviewers. Any product that may be evaluated in this article or claim that may be made by its manufacturer is not guaranteed or endorsed by the publisher.
Research integrity at Frontiers
Learn more about the work of our research integrity team to safeguard the quality of each article we publish.