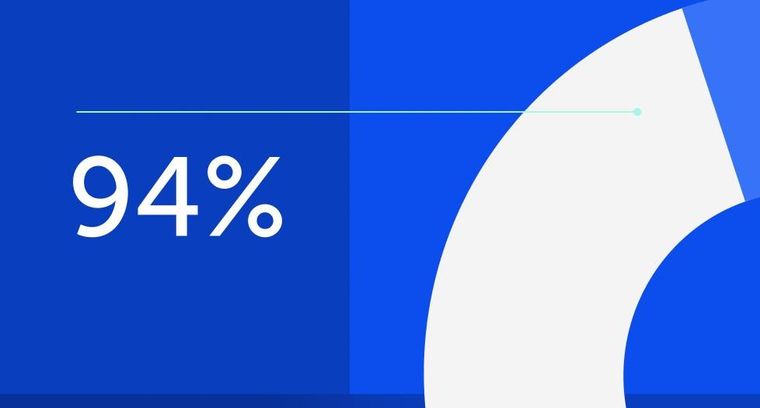
94% of researchers rate our articles as excellent or good
Learn more about the work of our research integrity team to safeguard the quality of each article we publish.
Find out more
ORIGINAL RESEARCH article
Front. Genet., 09 August 2024
Sec. Livestock Genomics
Volume 15 - 2024 | https://doi.org/10.3389/fgene.2024.1409335
This article is part of the Research TopicGenetic Regulation of Reproduction Traits in Livestock SpeciesView all 8 articles
Introduction: Targeted single nucleotide polymorphisms (SNPs) have been used in genomic prediction methodologies to enhance the accuracy of associated genetic transmitting abilities in Holstein cows. The objective of this study was to identify and validate SNPs associated with fertility traits impacting early embryo mortality.
Methods: The mRNA sequencing data from day 16 normal (n = 9) and embryo mortality (n = 6) conceptuses from lactating multiparous Holstein cows were used to detect SNPs. The selection of specific genes with SNPs as preliminary candidates was based on associations with reproductive and fertility traits. Validation of candidate SNPs and genotype-to-phenotype analyses were conducted in a separate cohort of lactating primiparous Holstein cows (n = 500). After genotyping, candidate SNPs were filtered using a quality control pipeline via PLINK software. Continuous numeric and binary models from reproductive traits were evaluated using the mixed procedure for a generalized linear model-one way ANOVA or logistic regression, respectively.
Results: Sixty-nine candidate SNPs were initially identified, but only 23 passed quality control procedures. Ultimately, the study incorporated 466 observations for statistical analysis after excluding animals with missing genotypes or phenotypes. Significant (p <0.05) associations with fertility traits were identified in seven of the 23 SNPs: DSC2 (cows with the A allele were older at first calving); SREBF1 and UBD (cows with the T or G alleles took longer to conceive); DECR1 and FASN (cows with the C allele were less likely to become pregnant at first artificial insemination); SREBF1 and BOLA-DMB (cows with the T allele were less likely to be pregnant at 150 days in milk). It was also determined that two candidate SNPs within the DSC2 gene were tag SNPs. Only DSC2 SNPs had an important allele substitution effect in cows with the G allele, which had a decreased age at first calving by 10 days.
Discussion: Candidate SNPs found in this study could be used to develop genetic selection tools to improve fertility traits in dairy production systems.
Until 2005, Holstein cows were under intense genetic selection for milk yield, which inadvertently led to a decline in fertility traits (García-Ruiz et al., 2016). This was to be expected given the negative genetic correlations that range from 0.35 to 0.60 for these traits (Pritchard et al., 2013). The advances in the use of genomic information have identified associations with economically relevant traits in cattle and revolutionized animal breeding programs (Matukumalli et al., 2009; Amos et al., 2011; Khatkar et al., 2014). Despite the genetic improvement in Holstein cows, the fertility performance of this breed is still considered suboptimal when compared to other dairy cattle breeds (Martinez-Castillero et al., 2020).
Fertility based on daughter pregnancy rate (DPR) has clearly improved over the last 2 decades (García-Ruiz et al., 2016). Regardless, embryo mortality (EM) is still an issue and was recently reported to be as high as 37% from d17–33 of pregnancy in Holstein cows (Domingues et al., 2024). Pregnancies that result in EM are associated with multiple factors, including inadequate interferon tau (IFNT) signaling by the conceptus (Evans et al., 2012), which plays a key role in establishing and maintaining pregnancy by preventing regression of the corpus luteum (Rizos et al., 2012; Hansen et al., 2017; Moraes et al., 2018). As a result, the production of progesterone from the corpus luteum continues to support conceptus growth and maintain a competent uterine environment for the implanting conceptus (Bazer et al., 1975; Bazer et al., 1997). A challenge with EM pregnancies is that they take place during the pre-implantation period, between days 7 and 16 of pregnancy. Therefore, pregnancy status is unknown until determined at day 32 via ultrasound (Lonergan and Forde, 2014). This hinders resynchronizing non-pregnant cows in a timely manner, limits management of a cow’s reproductive performance, and results in an annual loss of $1.6 billion for the dairy industry in the United States and $1.28 trillion worldwide (Suthar and Shah, 2009; Perkel et al., 2015), further providing a rationale for the selection of Holstein cows with superior fertility traits.
Given that single nucleotide polymorphisms (SNPs) are responsible for 84% of the variation in gene expression, their location in DNA could affect protein structure, production, and function and/or cause a phenotype that could vary due to the reproductive status of an animal (El-Sayed et al., 2006; Beltman et al., 2010; Kommadath et al., 2011; Spencer et al., 2014). Consequently, using SNPs associated with reproductive traits in genomic diagnostic panels may improve genomic estimates of predicted transmitting abilities (Cochran et al., 2013a). Diagnostics using SNP genotyping could further aid in the culling of cattle that are reproductively inferior (Singh et al., 2014).
It was hypothesized that pregnancies with EM are associated with missense SNPs that impair maternal-conceptus communication. We identified 69 candidate SNPs within lactating multiparous Holstein cows that had pregnancies with conceptuses that were either normal (N) or EM and aimed to validate SNP associations in a separate population of lactating primiparous Holstein cows (n = 500).
Figure 1 is a flow chart of the methodology from the initial lactating multiparous Holstein cow population (n = 15). Each cow had a day-16 conceptus collected for the discovery of genes with candidate SNPs that may influence pregnancies to become EM. The candidate SNPs were validated in a separate cohort of lactating primiparous Holstein cows (n = 500) in Colorado, United States.
Figure 1. Flowchart of methods used to identify candidate SNPs used in association analysis with reproduction traits in Holstein cows. EM, embryo mortality or N, normal pregnancy: cow’s pregnancy status; IPA, ingenuity pathway analysis.
The initial group of fifteen healthy lactating multiparous Holstein cows was located at a dairy farm in North-Eastern Colorado and had conceptuses collected as per approval of Colorado State University’s Institutional Animal Care and Use Committee (IACUC protocol number 17-7539A). An additional 500 healthy lactating primiparous Holsteins from an organic dairy farm in North-Eastern Colorado had blood samples collected in August 2020 (IACUC protocol number 1036). Both groups of cows were fed and milked twice daily, offered water ad-libitum, and received a total mixed ration according to the guidelines of the National Research Council (Council, 2001). Cows were also housed in a free-stall barn equipped with sprinklers, fans, and sand-beds.
After 60 days postpartum, the initial group of healthy lactating multiparous Holstein cows (n = 15) were subjected to synchronization of the estrous cycle and induced ovulation by the Ovsynch protocol. Cows were exposed to timed artificial insemination (TAI) and the same high-fertile sire’s semen. The day of TAI was considered day 0 for cows in the pregnant group. All were TAI at 16 h after the final intramuscular injection from Ovsynch. If no conceptus was recovered on day 16 after the first TAI, then new cows were assigned to the study.
Infusion of flushing media (30 mL of phosphate buffered saline [PBS] and 10 mL of 0.01% of polyvinyl alcohol; Sigma) was performed using a 60 mL plastic syringe (Norm-Ject) connected to a French silicone-coated latex Foley modified catheter (size 22-Bard). The Foley catheter was inserted transcervically into the uterine horn ipsilateral to the corpus luteum. Recovery of conceptuses involved using the same 60 mL syringe and placing the fluid in a sterile petri dish. Each dish was examined under a stereoscope (Stereo Star Zoom, American Optical) at ×7 magnification to find the conceptus. This procedure was repeated up to six times to ensure the recovery of the conceptus, if present. Once the conceptus was located, it was rinsed with sterile PBS and 0.1% (v/v) of polyvinyl alcohol, measured for length (millimeters), and photographed beside a ruler. Pregnancies were re-classified based on conceptus morphology and appearance as N (translucent and elongated [≥100 mm]; n = 9) or undergoing EM (pink, red, opaque and/or restricted elongation [≤60 mm]; n = 6). All conceptuses were snap-frozen with liquid nitrogen and stored at −80°C for RNA extraction.
Total conceptus RNA was extracted using TRIzol reagent (Life Technologies; Carlsbad, California) following the manufacturer’s instructions. The remaining DNA was removed with an RNase-free DNase (Qiagen) and RNAeasy MinElute Cleanup Kit 50 (Qiagen; catalog # 74204). RNA was quantified using a NanoDrop 2000 Spectrophotometer (Thermo Scientific, manufacture: ND2000USCAN). The quality of each RNA sample was determined by dividing the absorbance260 by absorbance280. Values of 2.0 were considered high-quality RNA samples.
Total conceptus RNA was shipped to be processed for cDNA libraries and sequencing using the Illumina HiSeq (2000) system by Zoetis Inc. Files were single-end sequencing with 75 bp. Sequences were trimmed and aligned to the bovine reference genome ARS-UCD 1.2 (http://bovinegenome.elsiklab.missouri.edu/node/61; (Rosen, 2018).
Using R studio (version 4.1.2; R core team, 2021), RNA-Seq data were exported, organized (dplyr and plyr), and filtered (edgeR) to exclude genes with less than 10 mRNA raw counts. The DESeq2 package (Love et al., 2014) in R used the Benjamini–Hochberg method to control for false discovery by adjusting p-values (Hochberg, 1995). Comparisons (1 × 1) for conceptuses (EM vs. N) were performed using a negative binomial distribution model. Consequently, all differentially expressed genes (DEGs) were identified with adjusted p-values <0.05.
The differentially expressed genes (DEGs) from RNA-Seq data were submitted into Ingenuity Pathway Analysis software (IPA; Qiagen) to gain additional biological insight into patterns of gene expression. The core analysis of the data used p < 0.001 and ±2 log2-fold change equivalent to a ±4-fold change in DEGs.
After collecting and processing conceptuses for RNA Seq and aligning their sequences to the bovine reference genome, the Qiagen CLC Genomics Workbench software (version 20.0.1; https://digitalinsights.qiagen.com) was used to identify SNPs within the conceptus RNA-Seq data. The SNP discovery entailed four steps. In the first step, genes with SNPs were selected based on (a) association with reproductive and fertility traits in published genome-wide association studies such as GWAS-SNP (Cochran et al., 2013a; Cochran et al., 2013b; Ortega et al., 2017) or QTLdb (Supplementary Table S3), and (b) DEGs that were identified in the conceptuses of the initial group of Holstein cows using IPA. The second step filtered genes identified in step one by selecting only those genes that were statistically significant in the conceptus RNA-Seq data. The third step separated SNPs into one of two groups: diagnostic (only individuals from one type of pregnancy [N or EM] were used to calculate the frequency of a genotype) or non-diagnostic (individuals from both types of pregnancies [N and EM] were used to calculate the frequency of a genotype). The fourth step consisted of (a) evaluating the function of each gene with SNPs, the region within a gene (i.e., non-synonymous), and the functional classification of SNPs (i.e., missense) via Ensembl Variant Effect Predictor, (b) conducting Sorting Intolerant From Tolerant tool analyses (McLaren et al., 2016) that predicted amino acid substitution affecting protein function by evaluating SNP values (0.0–1.0; closer to zero was predicted to have a significant effect in protein function), (c) verifying if the SNP location within the gene was near (5 centimorgan = 5 million nucleotides) a region/SNP previously associated with a reproductive or fertility trait in the Cattle quantitative trait loci (Cattle QTL; (Hu et al., 2022)) database, and (d) verifying the expression in Log2 fold changes of DEGs with candidate SNPs and their adjusted p-values (<0.05) within the conceptus RNA-Seq data.
The SNPs identified in the conceptus RNA-Seq data from the initial lactating multiparous Holstein cows (n = 15) needed to be validated. A separate group of lactating primiparous Holstein cows (n = 500) were randomly enrolled in the study at a dairy milking approximately 9,790 cows. Data of interest were divided into three categories. The first category consisted of health traits that were divided into two sub-categories: reproductive disease (mastitis, metritis, endometriosis, and pyometra) or non-reproductive (lameness, respiratory, digestive, and ketosis). Sub-categories of health traits were recorded on the date of incidence, number of incidences, and type of incidence (0: disease absence or 1: presence of disease) that occurred at or before 60 days of milk (DIM). The second category was comprised of reproductive trait data that were collected after their first calving and up to four artificial inseminations (AIs), which included age (days) at first calving, breeding, conception, and calving date of second pregnancy, season (warm season: June to September or cool season: October to May) that cow was bred and calved, sire identification used for artificial insemination (AI), AI technician identification, number of AI services, pregnancy outcome (pregnant or non-pregnant) and pregnancy loss (0: no pregnancy loss or 1: pregnancy loss; recorded as an abortion after conception). The third category encompassed production traits that were divided into predicted first lactation milk production at 305 DIM, culling of a cow (0: no culling or 1: culling), reason for culling, date of culling, and unit in which cow was housed (location 1 or location 2).
Blood was collected from the tail vein using evacuated tubes containing K2 EDTA (Vacutainer, Becton Dickinson, Franklin Lakes, NJ) while cows were restrained with individual headlocks. Blood samples were placed on ice for transport to the Animal Reproduction and Biotechnology Laboratory facility at Colorado State University. Upon arrival, samples were centrifuged for 30 min at 2,500 rpm to separate blood components. The buffy coat was extracted, placed in a 1.5 mL microcentrifuge tube, suspended up to 1 mL in 1× phosphate-buffered saline, and stored at −20C.
Genomic DNA was extracted from the buffy coat using the Qiagen DNeasy Blood and Tissue Kit (Cat. No. 69504) according to the manufacturer’s protocol. Sample purity and quality were quantified using a NanoDrop 2000 Spectrophotometer (Thermo Scientific, manufacture: ND2000USCAN) and dividing the absorbance’s wavelength reading, absorbance260 over absorbance280. Values of 2.0 were considered high-quality DNA samples.
A custom SNP genotyping panel for candidate SNPs was designed using the Agena Plex panel (MassARRAY System with 96-well plates) from Neogen® (Lansing, Michigan), which consisted of four steps conducted by Neogen®. The first step was providing a list of candidate SNPs with reference identification (RSID) and location of the SNP (150 base pairs up- and down-stream sequence). The second step involved conducting an in silico assay design to verify the efficiency and robustness of the assay based on the percentage of SNPs without overlap (less than 150 base pairs) of other SNPs. The SNPs that overlapped were separated into different panels. The third step tested the custom candidate SNP primers for robustness/efficiency by genotyping a subset of the primiparous Holstein cow samples (n = 24). The final step consisted of an optimized panel to genotype the remaining samples (n = 476). Thus, all primiparous Holstein samples (n = 500) were genotyped to validate the candidate SNPs.
Candidate SNPs were filtered after all primiparous Holstein samples (n = 500) were genotyped. The candidate SNPs that were not in minor allele frequency (MAF) of >10% and/or were monomorphic were eliminated from the study. The remaining candidate SNPs were evaluated by creating a five-step quality control pipeline implemented using PLINK software (version 1.07, (Purcell et al., 2007). The first step removed SNPs with 20% missing genotypes. The second step removed individual animals that were not genotyped for 10% of candidate SNPs. The third step removed candidate SNPs not in Hardy–Weinberg equilibrium at a level of significance above 1e−15. The fourth step evaluated the remaining candidate SNPs (Table 1) for linkage disequilibrium via r2 and d′ (Supplementary Table S1). The final step identified tag SNPs (Table 2; Figure 2). If more than one tag SNP was identified for a group of SNPs, the tag SNP was selected based on having the highest r2 and d′ values (i.e., strongest relationship to the group of SNPs). Additional animals were removed from the study due to missing reproductive (breeding date, calving of cow, and dystocia score) and production trait (predicted milk yield for 305 DIM during cow’s first lactation) data. Thus, the total number of observations used for the statistical models of the study represented 466 cows.
Table 1. Candidate single-nucleotide polymorphisms (SNPs; n = 23) that were non-monomorphic and passed the quality control pipeline using PLINK software in primiparous lactating Holstein cows (n = 466).
Table 2. Candidate single-nucleotide polymorphisms (SNPs; n = 8) with a tag SNP using PLINK software in primiparous lactating Holstein cows.
Figure 2. Candidate single-nucleotide polymorphisms (SNPs) and published SNPs associated with quantitative trait loci (QTL, orange lines) within fertility for each chromosome (Chr) in Holstein cows. CR, conception rate; D2C, days to conception; P1AI1, pregnant at first artificial insemination. Orange lines represent published SNPs associated with quantitative trait loci; purple lines represent candidate SNPs. Created with Biorender.com.
Genotype-phenotype statistical association models were evaluated for services per conception, age at first calving, days to conception, days to first AI, pregnant at first AI, pregnant at 150 DIM, and pregnancy loss (Table 3). The independent variables included within each model were genotypes, reproductive disease, non-reproductive disease, and housing unit. Each of the reproductive traits used as models was evaluated for mean, standard deviation, minimum, and maximum via the means procedure of SAS (SAS, 2023) (Table 4). Continuous numeric traits (services per conception, age at first calving, days to conception, and days to first AI) and binary models (i.e., 1: event occurred or 0: event did not occur; pregnant at first AI, pregnant at 150 DIM, and pregnancy loss) were evaluated for statistical significance with and without a single genotype term from candidate SNPs by using the generalized linear model-one way ANOVA or logistic regression, respectively. The r2 was calculated using McFadden’s r squared, which is calculated by the proc glm command and evaluated with and without the genotype term to demonstrate the amount of variation explained by the model’s inputs. Only statistically significant models with a single genotype term from candidate SNPs were evaluated using the means separation test within LSMEANS, which included the Bonferroni adjustment for p-values to minimize false discovery error from the mixed procedure (Weir, 2001). Interactions between genotype terms and other fixed effects were also evaluated but only among those sharing the same reproductive trait model. Furthermore, we evaluated the effect of genotype additive and dominance allele effects on candidate SNPs (Luna-Nevarez et al., 2011). Linear and quadratic contrasts were executed to confirm or reject such additive or dominant allele effects. Statistical procedures were conducted in SAS OnDemand for academic software, and statistical significance was defined as P ≤ 0.05.
Table 3. Basic model, and qualitative and quantitative covariate class variables used to predict the categorical traits.
Table 4. Summary statistics for study populations, including MEANS, GLMa, and Logisticb for conception, age at calving, and pregnancy in primiparous lactating Holstein cows.
Sixty-nine candidate SNPs were discovered within the RNA-Seq data of EM compared to N conceptuses from a multiparous population of Holstein cows (n = 15). All candidate SNPs were validated in a separate population of primiparous Holstein cows (n = 500). Only 30 of the 69 candidate SNPs were in MAF and were non-monomorphic. The remaining candidate SNPs were evaluated through a five-step quality control pipeline using PLINK software. The final number of candidate SNPs and individual animals for this study were 23 and 466, respectively (Table 1). Eight of the 23 candidate SNPs were in linkage disequilibrium via r2 and d′ (Supplementary Table S1), and two within the DSC2 gene were identified as tag SNPs (Table 2; Supplementary Figure S1).
All candidate SNPs were in proximity (<5 centimorgans) of at least one SNP associated with fertility traits reported in the Cattle QTL database (Figure 2). All models were evaluated for reproductive traits with single genotype terms and nine instances that differed (p < 0.05; Table 6; Supplementary Table S2; Figure 3). The R2 of a model was greater when the genotype term was significant (p < 0.05; Table 5).
Figure 3. Seven potential functional single-nucleotide polymorphisms (SNPs) within exons (E) and nucleotide (nt) position in each gene: (A) uridine monophosphate synthetase (UMPS), (B) 2,4-dienoyl-CoA reductase 1 (DECR1), (C) sterol regulatory element-binding transcription factor 1 (SREBF1), (D) fatty acid synthetase (FASN), (E) major histocompatibility complex, class II, DM beta (BOLA-DMB), (F) ubiquitin d (UBD), and (G) desmocollin-2 (DSC2) in their designated chromosome (Chr) for Holstein cows. Created with Biorender.com.
Table 5. Summary of R2 of the model without and with potential functional candidate single-nucleotide polymorphisms (SNPs; n = 7) in primiparous lactating Holstein cows.
Cows that were older at first calving were associated with carrying an A allele in the desmocollin-2 (DSC2; rs211151260; Table 6) SNP. Meanwhile, cows that carried a T allele e in sterol regulatory element-binding transcription factor 1 (SREBF1; rs41912290) or a G allele in ubiquitin d (UBD; rs209518868; Table 6) were associated with having longer intervals to become pregnant after calving. Cows might experience such intervals due to reproductive diseases impacting the health of the animal, the unit in which cows were housed, or their interactions, but that was not the case for both the SREBF1 and UBD SNPs. Moreover, no interactions were found between the SREBF1 and UBD SNPs. For the SNPs of uridine monophosphate synthetase (UMPS; rs110953962) and SREBF1 (rs41912290), cows that carried a T allele were associated with being AI’d later than other cows (Table 6). For both models, age and reproductive diseases were significant (p < 0.001). Age and disease interactions between UMPS or SREBF1 SNPs were not different in days to first AI. Cows associated with being less likely to become pregnant at first AI had a C allele in 2, 4-dienoyl-CoA reductase 1 (DECR1; rs41580472) or an A allele in fatty acid synthetase (FASN; rs41919985; Table 6). No differences were observed for other variables except for the genotype term of the DECR1 and FASN SNPs (p < 0.05). In addition, interactions (p < 0.05) were found between the DECR1 and FASN SNPs. Cows were less likely to become pregnant before or at 150 DIM with a T allele in SREBF1 (rs41912290) or in the major histocompatibility complex, class II, DM beta (BOLA-DMB; rs109032590; Table 6) when compared to those that had at least one C allele. No differences were found for variables except for the genotype terms of SREBF1 and BOLA-DMB SNPs (p < 0.05). Interactions (p < 0.01) were also found between the SREBF1 and BOLA-DMB SNPs in pregnant cows at 150 DIM.
Table 6. Least square means ± standard error for fertility traits among genotypes within genes with candidate single-nucleotide polymorphisms (SNPs) in primiparous lactating Holstein cows.
All of the potential functional SNPs were non-synonymous and classified as missense. Three of the seven potential functional SNPs were predicted to influence the protein function of the gene (p < 0.05; Table 7). All seven potential functional SNPs had an additive effect (p < 0.05) due to a linear trend observed when the genotype term was a fixed effect within the model (Table 8). Conversely, the DSC2 and SREBF1 SNPs had allele substitution effects (p < 0.05; Table 8), while only the DECR1 SNP tended (p < 0.10; Table 8) to yield differing levels among genotypes. Cows with allele G in DSC2 (p < 0.05) had a 10-day decreased age at first calving (Table 8). Meanwhile, cows with a C allele in SREBF1 impaired the trait level and had a 6% decreased (p < 0.05) probability of becoming pregnant at 150 DIM (Table 8).
Table 7. Variant effect predictor analysis for SNPs within genes with candidate single-nucleotide polymorphisms (SNPs) in primiparous lactating Holstein cows.
Table 8. Allele substitution estimates and fixed effect estimates of additive and dominance of the genes with candidate single-nucleotide polymorphisms (SNPs) in primiparous lactating Holstein cows.
The discovery of functional SNPs could contribute to improving breeding value estimation and genetic improvement of Holstein cows despite the low heritability of fertility traits. Improvement of these strategies could also have a direct impact on the economic efficacy and culling practices of the dairy industry (Pryce et al., 1997; Inchaisri et al., 2010). Among the seven reproductive traits (services per conception, age at first calving, days to conception, days to first AI, pregnant at first AI, pregnant at 150 DIM, and pregnancy loss) that were evaluated in this study, five SNPs may be expected to change amino acids and, based on the substitution effects, could be predicted to be damaging to protein function. Additional biochemical research into these proteins is needed to fully understand how these SNPs influence the variance of these traits.
Identification of at least one QTL associated with fertility traits was found in proximity to this study’s candidate SNPs. Others have shown that QTL has an association with causing an effect over nearby SNPs that control similar traits (Khatkar et al., 2004; Daetwyler et al., 2008). When conducting genotype-to-phenotype association studies, SNPs that are associated with QTL(s) may be helpful when implemented into breeding programs to improve the prediction of traits of low heritability (Spelman and Garrick, 1997; Van Tassell et al., 1999; Abdel-Azim and Freeman, 2002).
All candidate SNPs that were statistically significant (p < 0.05) within the models demonstrated a larger R2 value (Table 5). The optimum age of cows at first calving averages between 23 and 24 months because of the cost of rearing replacement heifers in dairy herds (Heinrichs, 1993; Hoffman, 1997; Gabler et al., 2000; Tozer and Heinrichs, 2001; Boulton AC, 2015). In the context of this study’s DSC2 SNP, cows with the G allele were older during their first calving. The DSC2 gene is known for its role in cell junction and adhesion (Lewin et al., 2022); therefore, it has been studied for its potential role in the development and function of bovine embryos via compaction and cavitation (Wrenzycki et al., 2001). The DSC2 SNP has only been studied in an Iranian Bos taurus breed, and no associations to age at first calving were identified in Holstein cows. Only a different SNP within DSC2 has been associated with fertility traits of daughter pregnancy rate in Holstein cows (Cochran et al., 2013a).
The interval of conception after calving is often used as a parameter to determine the reproductive performance of dairy cows (Harman et al., 1996), and, ideally, conception takes place prior to 85 days post calving (Grimard et al., 2006; Tillard et al., 2008). However, the interval for average days of conception after calving is highly dependent on diverse factors such as season (i.e., summer), peripartum disorders (i.e., metritis and endometritis), and cow management (Grimard et al., 2006; Tillard et al., 2008; Siddiqui et al., 2013). Minimizing the number of days that a cow is not pregnant (open) is crucial to decreasing costs (i.e., food, labor, breeding) through increasing culling rates as well as the number of calves and longevity of the animal (González-Recio et al., 2004; Inchaisri et al., 2010; Cabrera, 2014). Within our data, we identified the T allele in SREBF1 and the G allele in UMD SNP as being associated with cows having longer intervals of conception after calving. The SREBF1 gene function has been documented as an important regulator for the transcription of genes that synthesize milk fat and its secretion in the mammary epithelial cells of dairy cows (Harvatine and Bauman, 2006; Ma and Corl, 2012; Oppi-Williams et al., 2013; Li et al., 2014). The SREBF1 SNP discussed in this study has been submitted to the ENSEMBL SNP database and is associated with fatty acid indexes that influence milk fat and protein percentage in Holstein cows (Rincon et al., 2012; Cochran et al., 2013a). Despite this, associations for the SREBF1 SNP and its impact on days to conception have not been reported in Holstein cows. The UMD gene has general roles in DNA replication, recombination and repair, and cell development, assembly, maintenance, survival, and death (Salehi et al., 2016). Other discovered SNPs within UBD have mainly been reported to regulate responses by the immune system (Russell et al., 2012; Thompson-Crispi et al., 2014). Although our study’s UBD SNP has been reported in fourteen different cow and bull populations, no association with days to conception has been made in Holstein cows.
The average days to first AI measure was reported to range from 67 to 84 (Esslemont, 1992; Darwash et al., 1997; Royal et al., 2000; Waldmann et al., 2001; Yusuf et al., 2011), favoring an extension of DIM due to higher conception rates being obtained in later rather than earlier stages of lactation (Dohoo, 1983; Royal et al., 2000; Quintela Arias et al., 2004; Bouchard E, 2008). Our data revealed that cows that carried a T allele for UMPS or SREBF1 SNPs were associated with being AI’d 10 days later than cows who carried at least 1 C allele (Table 7). Studies on the UMPS gene have suggested that it has a role in the synthesis of nucleotides in both DNA and RNA (Healey and Shanks, 1987). Deficiency (inactivation) of UMPs, also known as DUMPs, is an inherited recessive disorder that causes arrested growth and development of pregnancies, which leads to EM in Holstein cows (Shanks and Robinson, 1990; Shanks and Greiner, 1992; Robinson et al., 1993; Kuhn and Shanks, 1994). Furthermore, carriers of DUMPs have been found to remain non-pregnant for longer periods of time (Čítek and Barbora, 2004). Prevention of this condition consists of early detection of defect carriers by screening herds routinely through haplotype tests (VĂTĂŞEscu-Balcan et al., 2006). The UMP SNPs identified herein have only been reported within an Iranian B. taurus breed, but no associations have been made to days to first AI in Holstein cows. Likewise, no associations of the SREBF1 SNP identified in this study were made in other studies with days to first AI in Holstein cows.
Pregnancy at first AI, which is similar to the trait of days to conception, depends on the time of year, voluntary waiting period, reproductive/peripartum disorders, and reproductive management (Grimard et al., 2006; Tillard et al., 2008; Siddiqui et al., 2013). It has also been proven that dairy cows serviced more than once have reduced pregnancy rates at first AI (77.3% vs. 35.7%) and a 4.5% decreased probability of becoming pregnant for each previously unsuccessful service (Barrett, 1948; Sprott et al., 1998). Our data suggested that the probability of cows becoming pregnant at first AI was lower when they had a C allele in the DECR1 SNP or an A allele in the FASN SNP. The DECR1 gene acts as a mitochondrial enzyme involved in beta-oxidation that regulates the rate of fatty acid metabolism, which contributes to energy production (Kunau and Dommes, 1978; Wathes et al., 2012). The SNP within DECR1 was associated with an effect on lipid metabolism, milk production, back fat thickness, days to first service, and calving interval in beef cattle (Marques et al., 2009; Clempson et al., 2012). Boussaha et al. (2015) submitted the DECR1 SNP within a GWAS data set, but no other publications have associated this submission with pregnancy at first AI in Holstein cows.
The FASN gene role has been associated with catalyzing the production of long-chain fatty acids and has been evaluated as a candidate for improving fat levels in both milk and muscle of cattle (Roy et al., 2006; Schennink et al., 2009; Matsumoto et al., 2012; Li et al., 2016). The SNP within FASN was found to be associated with lactation traits, composition of fatty acids within milk, and reconstitution of body reserves during gestation (Matsumoto et al., 2012; Elis et al., 2013; Mauric et al., 2019) but not with pregnancy at first AI.
Pregnancy that takes place before or at 150 DIM is contingent on reproductive diseases, detection of estrus, voluntary waiting period, and season (Grimard et al., 2006; Tillard et al., 2008; Siddiqui et al., 2013). Timing when pregnancy occurs in dairy cows is critical in sustaining profitability in the industry (Giordano et al., 2011). Both SREBF1 and BOLA-DMB were found to have SNPs in our data associated with a lower probability of becoming pregnant before or at 150 DIM. In the case of the SREBF1 SNP, no associations with pregnancy at 150 DIM have been made. The BOLA-DMB gene is part of the bovine immune system’s major histocompatibility complex in the class IIb region, and it is responsible for aiding with the loading of peptides in antigen-presenting cells (Pathak et al., 2001; La Rocca et al., 2014). Interestingly, the regulation of BOLA-DMB is different in the endometrium of cows that are pregnant compared to non-pregnant cows (Cerri et al., 2012). Associations for this study’s BOLA-DMB SNP were made for daughter pregnancy rate, cow conception rate, heifer pregnancy rate, and milk yield (Cochran et al., 2013a; Ortega et al., 2016; Ortega et al., 2017; Ortega, 2018) but not specifically to pregnancy at 150 DIM.
For allele substitution and additive effects, cows with the beneficial G allele within DSC2 had improved age of first calving, which decreased by 10 days (Table 8). Eastham et al. (2018) reported that cows that were younger during their first calving had an association with udder health, increased longevity, milk yield, improved reproductive performance, and increased probability of calving for a second time. Cows’ age at first AI was demonstrated to be dependent on the management of the herd and was unique to each individual dairy (Esslemont, 1992; Darwash et al., 1997; Royal et al., 2000; Waldmann et al., 2001; Yusuf et al., 2011). Moreover, it has also been shown that cows had higher conception rates when AI occurs at later stages of lactation (Dohoo, 1983; Royal et al., 2000; Quintela Arias et al., 2004; Bouchard E, 2008). Pregnancy occurring before or at 150 DIM is of importance to sustain profitability within the industry, and it optimally occurs between 90 and 130 DIM (Giordano et al., 2011). Thus, the candidate SNPs associated with pregnant at 150 DIM may somewhat be unique to the reproductive management practices of an organic farm, causing longer times for each lactation cycle (Sorge et al., 2016).
This study validated the SNPs identified within the conceptuses (EM and N) of the initial herd of Holstein cows by using an independent herd through genotype-to-phenotype associations. Validation of candidate SNPs using blood samples to evaluate gene expression of reproductive phenotypes has proven useful for genetic panels/evaluations performed in cattle, such as expected progeny differences, predictive transmissibility, or genomic breeding values (i.e., Clarifide from Zoetis). Therefore, developing breeding values that encompass fertility traits is of great value when predicting the expected fertility of replacement Holstein heifers and cows that should remain in the herd (Weigel, 2006; García-Ruiz et al., 2016).
Genotype-to-phenotype analysis of 69 candidate SNPs suggested that seven SNPs were associated with fertility traits that are of economic importance within Holstein cows that are reproductively inferior. Additional research should be conducted to determine the utility of these candidate SNPs in genome-enhanced predictive transmissibility abilities estimations and (or) commercial genotyping tools for early life sorting of heifers. Thus, future use of said SNPs might be useful for culling decisions upon validation upon validation if they are predictive.
The datasets presented in this study can be found in online repositories. The names of the repository/repositories and accession number(s) can be found at: https://www.ncbi.nlm.nih.gov/geo/, GSE233492.
The animal study was approved by the Colorado State University IACUC Committee (protocol numbers: 17-7539A and 1036). The study was conducted in accordance with the local legislation and institutional requirements.
CG-B: conceptualization, data curation, formal analysis, funding acquisition, investigation, methodology, project administration, resources, software, validation, visualization, writing–original draft, and writing–review and editing. CB: formal analysis, methodology, software, and writing–review and editing. HS: formal analysis, methodology, software, and writing–review and editing. JB: data curation, formal analysis, and writing–review and editing. HV: data curation, formal analysis, and writing–review and editing. PP: data curation, formal analysis, methodology, and writing–review and editing. TH: conceptualization, data curation, formal analysis, funding acquisition, investigation, methodology, resources, supervision, validation, visualization, and writing–review and editing. MT: conceptualization, data curation, formal analysis, funding acquisition, investigation, methodology, resources, software, supervision, validation, visualization, and writing–review and editing.
The author(s) declare that financial support was received for the research, authorship, and/or publication of this article. This project was funded by the Agriculture and Food Research Initiative Fellowships Programs: Predoctoral Fellowships (2020-67034-31730), National Needs Fellowships Program (2016-38420-25289), and the Hatch/Multi State Research Grant Accession No. 1011648, Project No. COLO0220C, W-4112 awarded by the United States Department of Agriculture-National Institute of Food and Agriculture.
The authors would like to dedicate this manuscript to the memory of MT (23 December 1965—15 February 2024). He was a mentor, colleague, and friend to many who worked in the field of Animal Science. He will be sorely missed. The following dairies provided access to their cows and management records: Blue Sky Dairy, Jim and Josh Docheff and family, Mead, Colorado; Aurora Dairy, Juan Velez, Boulder, Colorado. Tom Rea, DVM, flushed embryos for this study at his clinic, Genetics West, in Berthoud, CO.
The authors declare that the research was conducted in the absence of any commercial or financial relationships that could be construed as a potential conflict of interest.
All claims expressed in this article are solely those of the authors and do not necessarily represent those of their affiliated organizations, or those of the publisher, the editors, and the reviewers. Any product that may be evaluated in this article, or claim that may be made by its manufacturer, is not guaranteed or endorsed by the publisher.
The Supplementary Material for this article can be found online at: https://www.frontiersin.org/articles/10.3389/fgene.2024.1409335/full#supplementary-material
AI, artificial insemination; DEG, differentially expressed gene; DIM, days in milk; EM, embryo mortality; N, normal conceptus; MAF, minor allele frequency; RNA-Seq, RNA sequencing; SNP, single-nucleotide polymorphism; QTL, quantitative trait loci.
Abdel-Azim, G., and Freeman, A. E. (2002). Superiority of QTL-assisted selection in dairy cattle breeding Schemes. J. Dairy Sci. 85 (7), 1869–1880. doi:10.3168/jds.S0022-0302(02)74261-6
Amos, W., Driscoll, E., and Hoffman, J. I. (2011). Candidate genes versus genome-wide associations: which are better for detecting genetic susceptibility to infectious disease?. Proc. Biol. Sci. 278 (1709), 1183–1188. doi:10.1098/rspb.2010.1920
Barrett, J. W., Lloyd, C. A., and Carpenter, R. A. (1948). Order number of insemination and conception rate. J. Dairy Sci. 31, 683.
Bazer, F. W., Chen, T. T., Knight, J. W., Schlosnagle, D., Baldwin, N. J., and Roberts, R. M. (1975). Presence of a progesterone-induced, uterine specific, acid phosphatase in allantoic fluid of gilts. J. Anim. Sci. 41 (4), 1112–1119. doi:10.2527/jas1975.4141112x
Bazer, F. W., Spencer, T. E., and Ott, T. L. (1997). Interferon tau: a novel pregnancy recognition signal. Am. J. Reprod. Immunol. 37 (6), 412–420. doi:10.1111/j.1600-0897.1997.tb00253.x
Beltman, M. E., Forde, N., Furney, P., Carter, F., Roche, J. F., Lonergan, P., et al. (2010). Characterisation of endometrial gene expression and metabolic parameters in beef heifers yielding viable or non-viable embryos on Day 7 after insemination. Reprod. Fertil. Dev. 22 (6), 987–999. doi:10.1071/rd09302
Bouchard, E. T. D. (2008). Dairy herd production and reproduction in Quebec and Canada. Budapest, Hungary: World Buiatrics Congress.
Boulton Ac, R. J., and Wathes, D. C. (2015). A study of dairy heifer rearing practices from birth to weaning and their associated costs on UK dairy farms. Open J. Animal Sci. 05 (5), 185–197. doi:10.4236/ojas.2015.52021
Boussaha, M., Esquerré, D., Barbieri, J., Djari, A., Pinton, A., Letaief, R., et al. (2015). Genome-wide study of structural variants in bovine holstein, Montbéliarde and Normande dairy breeds. PLoS One 10 (8), e0135931. doi:10.1371/journal.pone.0135931
Cabrera, V. E. (2014). Economics of fertility in high-yielding dairy cows on confined TMR systems. Animal 8 (Suppl. 1), 211–221. doi:10.1017/s1751731114000512
Cerri, R. L., Thompson, I. M., Kim, I. H., Ealy, A. D., Hansen, P. J., Staples, C. R., et al. (2012). Effects of lactation and pregnancy on gene expression of endometrium of Holstein cows at day 17 of the estrous cycle or pregnancy. J. Dairy Sci. 95 (10), 5657–5675. doi:10.3168/jds.2011-5114
Čítek, J., and Barbora, B. (2004). Recessive disorders - a serious health hazard?. J. Appl. Biomed. 2doi. doi:10.32725/jab.2004.022
Clempson, A. M., Pollott, G. E., Brickell, J. S., and Wathes, D. C. (2012). Associations between bovine IGFBP2 polymorphisms with fertility, milk production, and metabolic status in UK dairy cows. Anim. Biotechnol. 23 (2), 101–113. doi:10.1080/10495398.2011.650775
Cochran, S. D., Cole, J. B., Null, D. J., and Hansen, P. J. (2013a). Discovery of single nucleotide polymorphisms in candidate genes associated with fertility and production traits in Holstein cattle. BMC Genet. 14, 49. doi:10.1186/1471-2156-14-49
Cochran, S. D., Cole, J. B., Null, D. J., and Hansen, P. J. (2013b). Single nucleotide polymorphisms in candidate genes associated with fertilizing ability of sperm and subsequent embryonic development in cattle. Biol. Reprod. 89 (3), 69. doi:10.1095/biolreprod.113.111260
Council, N. R. (2001). Nutrient Requirements of dairy cattle: Seventh Revised edition, 2001. Washington, DC: The National Academies Press.
Daetwyler, H. D., Schenkel, F. S., Sargolzaei, M., and Robinson, J. A. (2008). A genome scan to detect quantitative trait loci for economically important traits in Holstein cattle using two methods and a dense single nucleotide polymorphism map. J. Dairy Sci. 91 (8), 3225–3236. doi:10.3168/jds.2007-0333
Darwash, A. O., Lamming, G. E., and Wooliams, J. A. (1997). The phenotypic association between the interval to post-partum ovulation and traditional measures of fertility in dairy cattle. Animal Sci. 65 (1), 9–16. doi:10.1017/S1357729800016234
Dohoo, I. R. (1983). The effects of calving to first service interval on reproductive performance in normal cows and cows with postpartal disease. Can. Vet. J. 24 (11), 343–346.
Domingues, R. R., Andrade, J. P. N., Cunha, T. O., Madureira, G., Hoppman, A. S., Teixeira, N. N., et al. (2024). Profiles of interferon-stimulated genes in multiple tissues and circulating pregnancy-associated glycoproteins and their association with pregnancy loss in dairy cows†. Biol. Reprod. 110 (3), 558–568. doi:10.1093/biolre/ioad164
Eastham, N. T., Coates, A., Cripps, P., Richardson, H., Smith, R., and Oikonomou, G. (2018). Associations between age at first calving and subsequent lactation performance in UK Holstein and Holstein-Friesian dairy cows. PLoS One 13 (6), e0197764. doi:10.1371/journal.pone.0197764
Elis, S., Coyral-Castel, S., Freret, S., Cognié, J., Desmarchais, A., Fatet, A., et al. (2013). Expression of adipokine and lipid metabolism genes in adipose tissue of dairy cows differing in a female fertility quantitative trait locus. J. Dairy Sci. 96 (12), 7591–7602. doi:10.3168/jds.2013-6615
El-Sayed, A., Hoelker, M., Rings, F., Salilew, D., Jennen, D., Tholen, E., et al. (2006). Large-scale transcriptional analysis of bovine embryo biopsies in relation to pregnancy success after transfer to recipients. Physiol. Genomics 28 (1), 84–96. doi:10.1152/physiolgenomics.00111.2006
Esslemont, R. J. (1992). Measuring dairy herd fertility. Vet. Rec. 131 (10), 209–212. doi:10.1136/vr.131.10.209
Evans, A. C., Mossa, F., Walsh, S. W., Scheetz, D., Jimenez-Krassel, F., Ireland, J. L., et al. (2012). Effects of maternal environment during gestation on ovarian folliculogenesis and consequences for fertility in bovine offspring. Reprod. Domest. Anim. 47 (Suppl. 4), 31–37. doi:10.1111/j.1439-0531.2012.02052.x
Gabler, M. T., Tozer, P. R., and Heinrichs, A. J. (2000). Development of a cost analysis spreadsheet for calculating the costs to raise a replacement dairy heifer. J. Dairy Sci. 83 (5), 1104–1109. doi:10.3168/jds.S0022-0302(00)74975-7
García-Ruiz, A., Cole, J. B., VanRaden, P. M., Wiggans, G. R., Ruiz-López, F. J., and Van Tassell, C. P. (2016). Changes in genetic selection differentials and generation intervals in US Holstein dairy cattle as a result of genomic selection. Proc. Natl. Acad. Sci. U. S. A. 113 (28), E3995–E4004. doi:10.1073/pnas.1519061113
Giordano, J. O., Fricke, P. M., Wiltbank, M. C., and Cabrera, V. E. (2011). An economic decision-making support system for selection of reproductive management programs on dairy farms. J. Dairy Sci. 94 (12), 6216–6232. doi:10.3168/jds.2011-4376
González-Recio, O., Pérez-Cabal, M. A., and Alenda, R. (2004). Economic value of female fertility and its relationship with profit in Spanish dairy cattle. J. Dairy Sci. 87 (9), 3053–3061. doi:10.3168/jds.S0022-0302(04)73438-4
Grimard, B., Freret, S., Chevallier, A., Pinto, A., Ponsart, C., and Humblot, P. (2006). Genetic and environmental factors influencing first service conception rate and late embryonic/foetal mortality in low fertility dairy herds. Anim. Reprod. Sci. 91 (1-2), 31–44. doi:10.1016/j.anireprosci.2005.03.003
Hansen, T. R., Sinedino, L. D. P., and Spencer, T. E. (2017). Paracrine and endocrine actions of interferon tau (IFNT). Reproduction 154 (5), F45-F59–f59. doi:10.1530/rep-17-0315
Harman, J. L., Casella, G., and Gröhn, Y. T. (1996). The application of event-time regression techniques to the study of dairy cow interval-to-conception. Prev. Veterinary Med. 26 (3), 263–274. doi:10.1016/0167-5877(95)00553-6
Harvatine, K. J., and Bauman, D. E. (2006). SREBP1 and thyroid hormone responsive spot 14 (S14) are involved in the regulation of bovine mammary lipid synthesis during diet-induced milk fat depression and treatment with CLA. J. Nutr. 136 (10), 2468–2474. doi:10.1093/jn/136.10.2468
Healey, M. H., and Shanks, R. D. (1987). Performance of females heterozygous for deficiency of uridine monophosphate synthase. J. Dairy Sci. 70 (5), 945–951. doi:10.3168/jds.S0022-0302(87)80098-X
Heinrichs, A. J. (1993). Raising dairy replacements to meet the needs of the 21st century. J. Dairy Sci. 76 (10), 3179–3187. doi:10.3168/jds.S0022-0302(93)77656-0
Hochberg, Y. B. (1995). Controlling the false discovery rate: a practical and Powerful Approach to multiple testing. R. Stat. Soc. 57 (1), 289–300. doi:10.1111/j.2517-6161.1995.tb02031.x
Hoffman, P. C. (1997). Optimum body size of Holstein replacement heifers. J. Anim. Sci. 75 (3), 836–845. doi:10.2527/1997.753836x
Hu, Z. L., Park, C. A., and Reecy, J. M. (2022). Bringing the Animal QTLdb and CorrDB into the future: meeting new challenges and providing updated services. Nucleic Acids Res. 50 (D1), D956–D961. doi:10.1093/nar/gkab1116
Inchaisri, C., Jorritsma, R., Vos, P. L., van der Weijden, G. C., and Hogeveen, H. (2010). Economic consequences of reproductive performance in dairy cattle. Theriogenology 74 (5), 835–846. doi:10.1016/j.theriogenology.2010.04.008
Khatkar, M. S., Randhawa, I. A. S., and Raadsma, H. W. (2014). Meta-assembly of genomic regions and variants associated with female reproductive efficiency in cattle. Livest. Sci. 166, 144–157. doi:10.1016/j.livsci.2014.05.015
Khatkar, M. S., Thomson, P. C., Tammen, I., and Raadsma, H. W. (2004). Quantitative trait loci mapping in dairy cattle: review and meta-analysis. Genet. Sel. Evol. 36 (2), 163–190. doi:10.1186/1297-9686-36-2-163
Kommadath, A., Woelders, H., Beerda, B., Mulder, H. A., de Wit, A. A., Veerkamp, R. F., et al. (2011). Gene expression patterns in four brain areas associate with quantitative measure of estrous behavior in dairy cows. BMC Genomics 12, 200. doi:10.1186/1471-2164-12-200
Kuhn, M. T., and Shanks, R. D. (1994). Association of deficiency of uridine monophosphate synthase with production and reproduction. J. Dairy Sci. 77 (2), 589–597. doi:10.3168/jds.S0022-0302(94)76988-5
Kunau, W. H., and Dommes, P. (1978). Degradation of unsaturated fatty acids. Identification of intermediates in the degradation of cis-4-decenoly-CoA by extracts of beef-liver mitochondria. Eur. J. Biochem. 91 (2), 533–544. doi:10.1111/j.1432-1033.1978.tb12707.x
La Rocca, C., Carbone, F., Longobardi, S., and Matarese, G. (2014). The immunology of pregnancy: regulatory T cells control maternal immune tolerance toward the fetus. Immunol. Lett. 162 (1 Pt A), 41–48. doi:10.1016/j.imlet.2014.06.013
Lewin, T. D., Fouladi-Nashta, A. A., and Holland, P. W. H. (2022). PRD-class Homeobox genes in bovine early embryos: function, Evolution, and overlapping roles. Mol. Biol. Evol. 39 (5), msac098. doi:10.1093/molbev/msac098
Li, C., Sun, D., Zhang, S., Yang, S., Alim, M. A., Zhang, Q., et al. (2016). Genetic effects of FASN, PPARGC1A, ABCG2 and IGF1 revealing the association with milk fatty acids in a Chinese Holstein cattle population based on a post genome-wide association study. BMC Genet. 17, 110. doi:10.1186/s12863-016-0418-x
Li, N., Zhao, F., Wei, C., Liang, M., Zhang, N., Wang, C., et al. (2014). Function of SREBP1 in the milk fat synthesis of dairy cow mammary epithelial cells. Int. J. Mol. Sci. 15 (9), 16998–17013. doi:10.3390/ijms150916998
Lonergan, P., and Forde, N. (2014). Maternal-embryo interaction leading up to the initiation of implantation of pregnancy in cattle. Animal 8 (Suppl. 1), 64–69. doi:10.1017/s1751731114000470
Love, M. I., Huber, W., and Anders, S. (2014). Moderated estimation of fold change and dispersion for RNA-seq data with DESeq2. Genome Biol. 15 (12), 550. doi:10.1186/s13059-014-0550-8
Luna-Nevarez, P., Rincon, G., Medrano, J. F., Riley, D. G., Chase, C. C., Coleman, S. W., et al. (2011). Single nucleotide polymorphisms in the growth hormone-insulin-like growth factor axis in straightbred and crossbred Angus, Brahman, and Romosinuano heifers: population genetic analyses and association of genotypes with reproductive phenotypes. J. Anim. Sci. 89 (4), 926–934. doi:10.2527/jas.2010-3483
Ma, L., and Corl, B. A. (2012). Transcriptional regulation of lipid synthesis in bovine mammary epithelial cells by sterol regulatory element binding protein-1. J. Dairy Sci. 95 (7), 3743–3755. doi:10.3168/jds.2011-5083
Marques, E., Nkrumah, J. D., Sherman, E. L., and Moore, S. S. (2009). Polymorphisms in positional candidate genes on BTA14 and BTA26 affect carcass quality in beef cattle. J. Anim. Sci. 87 (8), 2475–2484. doi:10.2527/jas.2008-1456
Martinez-Castillero, M., Toledo-Alvarado, H., Pegolo, S., Vazquez, A. I., de Los Campos, G., Varona, L., et al. (2020). Genetic parameters for fertility traits assessed in herds divergent in milk energy output in Holstein-Friesian, Brown Swiss, and Simmental cattle. J. Dairy Sci. 103 (12), 11545–11558. doi:10.3168/jds.2020-18934
Matsumoto, H., Inada, S., Kobayashi, E., Abe, T., Hasebe, H., Sasazaki, S., et al. (2012). Identification of SNPs in the FASN gene and their effect on fatty acid milk composition in Holstein cattle. Livest. Sci. 144 (3), 281–284. doi:10.1016/j.livsci.2011.12.003
Matukumalli, L. K., Lawley, C. T., Schnabel, R. D., Taylor, J. F., Allan, M. F., Heaton, M. P., et al. (2009). Development and characterization of a high density SNP genotyping assay for cattle. PLoS One 4 (4), e5350. doi:10.1371/journal.pone.0005350
Mauric, M., Masek, T., Ljoljic, D. B., Grbavac, J., and Starcevic, K. (2019). Effects of different variants of the FASN gene on production performance and milk fatty acid composition in Holstein × Simmental dairy cows. Veterinární medicína 164 (03), 101–108. doi:10.17221/73/2018-vetmed
McLaren, W., Gil, L., Hunt, S. E., Riat, H. S., Ritchie, G. R., Thormann, A., et al. (2016). The Ensembl variant effect predictor. Genome Biol. 17 (1), 122. doi:10.1186/s13059-016-0974-4
Moraes, J. G. N., Behura, S. K., Geary, T. W., Hansen, P. J., Neibergs, H. L., and Spencer, T. E. (2018). Uterine influences on conceptus development in fertility-classified animals. Proc. Natl. Acad. Sci. U. S. A. 115 (8), E1749-E1758–e1758. doi:10.1073/pnas.1721191115
Oppi-Williams, C., Suagee, J. K., and Corl, B. A. (2013). Regulation of lipid synthesis by liver X receptor α and sterol regulatory element-binding protein 1 in mammary epithelial cells. J. Dairy Sci. 96 (1), 112–121. doi:10.3168/jds.2011-5276
Ortega, M. S., Denicol, A. C., Cole, J. B., Null, D. J., and Hansen, P. J. (2016). Use of single nucleotide polymorphisms in candidate genes associated with daughter pregnancy rate for prediction of genetic merit for reproduction in Holstein cows. Anim. Genet. 47 (3), 288–297. doi:10.1111/age.12420
Ortega, M. S., Denicol, A. C., Cole, J. B., Null, D. J., Taylor, J. F., Schnabel, R. D., et al. (2017). Association of single nucleotide polymorphisms in candidate genes previously related to genetic variation in fertility with phenotypic measurements of reproductive function in Holstein cows. J. Dairy Sci. 100 (5), 3725–3734. doi:10.3168/jds.2016-12260
Ortega, S. (2018). Identification of genes associated with reproductive function in dairy cattle. Anim. Reprod. 15, 923–932. doi:10.21451/1984-3143-AR2018-0018
Pathak, S. S., Lich, J. D., and Blum, J. S. (2001). Cutting edge: editing of recycling class II:peptide complexes by HLA-DM. J. Immunol. 167 (2), 632–635. doi:10.4049/jimmunol.167.2.632
Perkel, K. J., Tscherner, A. K., Merrill, C., LaMarre, J., and Madan, P. (2015). The ART of selecting the best embryo: a review of early embryonic mortality and bovine embryo viability assessment methods. Mol. Reproduction Dev. 82, 822–838. doi:10.1002/mrd.22525
Pritchard, T., Coffey, M., Mrode, R., and Wall, E. (2013). Genetic parameters for production, health, fertility and longevity traits in dairy cows. Animal 7 (1), 34–46. doi:10.1017/s1751731112001401
Pryce, J. E., Veerkamp, R. F., Thompson, R., Hill, W. G., and Simm, G. (1997). Genetic aspects of common health disorders and measures of fertility in Holstein Friesian dairy cattle. Animal Sci. 65 (3), 353–360. doi:10.1017/S1357729800008559
Purcell, S., Neale, B., Todd-Brown, K., Thomas, L., Ferreira, M. A., Bender, D., et al. (2007). PLINK: a tool set for whole-genome association and population-based linkage analyses. Am. J. Hum. Genet. 81 (3), 559–575. doi:10.1086/519795
Quintela Arias, L., Peña, A., Taboada, M. J., Alonso, G., Portas, B., Diaz, C., et al. (2004). Risk factors for low pregnancy rate in dairy cattle: a retrospective study in the north west of Spain. Arch. Zootec. 53, 69–76. ISSN 0004-0592.
Rincon, G., Islas-Trejo, A., Castillo, A. R., Bauman, D. E., German, B. J., and Medrano, J. F. (2012). Polymorphisms in genes in the SREBP1 signalling pathway and SCD are associated with milk fatty acid composition in Holstein cattle. J. Dairy Res. 79 (1), 66–75. doi:10.1017/s002202991100080x
Rizos, D., Scully, S., Kelly, A. K., Ealy, A. D., Moros, R., Duffy, P., et al. (2012). Effects of human chorionic gonadotrophin administration on day 5 after oestrus on corpus luteum characteristics, circulating progesterone and conceptus elongation in cattle. Reprod. Fertil. Dev. 24 (3), 472–481. doi:10.1071/rd11139
Robinson, J. L., Popp, R. G., Shanks, R. D., Oosterhof, A., and Veerkamp, J. H. (1993). Testing for deficiency of uridine monophosphate synthase among holstein-friesian cattle of North America and Europe. Livest. Prod. Sci. 36 (4), 287–298. doi:10.1016/0301-6226(93)90046-K
Rosen, B. D., Bickhart, D. M., Schnabel, R. D., Koren, S., Elsik, C. G., Zimin, A., et al. (2018). “Modernizing the bovine reference genome assembly,” in Proceedings of the 11th world congress on genetics applied to livestock production, 11–16.
Roy, R., Ordovas, L., Zaragoza, P., Romero, A., Moreno, C., Altarriba, J., et al. (2006). Association of polymorphisms in the bovine FASN gene with milk-fat content. Anim. Genet. 37 (3), 215–218. doi:10.1111/j.1365-2052.2006.01434.x
Royal, M. D., Darwash, A. O., Flint, A. P. F., Webb, R., Woolliams, J. A., and Lamming, G. E. (2000). Declining fertility in dairy cattle: changes in traditional and endocrine parameters of fertility. Animal Sci. 70 (3), 487–501. doi:10.1017/S1357729800051845
Russell, G. C., Benavides, J., Grant, D. M., Todd, H., Thomson, J., Puri, V., et al. (2012). Host gene expression changes in cattle infected with Alcelaphine herpesvirus 1. Virus Res. 169 (1), 246–254. doi:10.1016/j.virusres.2012.08.011
Salehi, R., Colazo, M. G., Tsoi, S., Behrouzi, A., Tsang, B. K., Dyck, M. K., et al. (2016). Morphologic and transcriptomic assessment of bovine embryos exposed to dietary long-chain fatty acids. Reproduction 152 (6), 715–726. doi:10.1530/rep-16-0093
SAS (2023). SAS Institute Inc. User’s Guide: Statistic, SAS/STAT®15, 3. Cary, NC: SAS Institute Inc.
Schennink, A., Bovenhuis, H., Léon-Kloosterziel, K. M., van Arendonk, J. A., and Visker, M. H. (2009). Effect of polymorphisms in the FASN, OLR1, PPARGC1A, PRL and STAT5A genes on bovine milk-fat composition. Anim. Genet. 40 (6), 909–916. doi:10.1111/j.1365-2052.2009.01940.x
Shanks, R. D., and Greiner, M. M. (1992). Relationship between genetic merit of Holstein bulls and deficiency of uridine-5’-monophosphate synthase. J. Dairy Sci. 75 (7), 2023–2029. doi:10.3168/jds.S0022-0302(92)77962-4
Shanks, R. D., and Robinson, J. L. (1990). Deficiency of uridine monophosphate synthase among Holstein cattle. Cornell Vet. 80 (2), 119–122.
Siddiqui, M. A., Das, Z. C., Bhattacharjee, J., Rahman, M. M., Islam, M. M., Haque, M. A., et al. (2013). Factors affecting the first service conception rate of cows in smallholder dairy farms in Bangladesh. Reprod. Domest. Anim. 48 (3), 500–505. doi:10.1111/rda.12114
Singh, U., Deb, R., Alyethodi, R. R., Alex, R., Kumar, S., Chakraborty, S., et al. (2014). Molecular markers and their applications in cattle genetic research: a review. Biomarkers Genomic Med. 6 (2), 49–58. doi:10.1016/j.bgm.2014.03.001
Sorge, U. S., Moon, R., Wolff, L. J., Michels, L., Schroth, S., Kelton, D. F., et al. (2016). Management practices on organic and conventional dairy herds in Minnesota. J. Dairy Sci. 99 (4), 3183–3192. doi:10.3168/jds.2015-10193
Spelman, R., and Garrick, D. (1997). Utilisation of marker assisted selection in a commercial dairy cow population. Livest. Prod. Sci. 47 (2), 139–147. doi:10.1016/S0301-6226(96)01405-4
Spencer, T. E., Hansen Peter, J., Cole John, B., Joseph, D., and Holly, N. (2014). Genomic selection and reproductive efficiency in dairy cattle in: dairy cattle reproduction Salt Lake City, Utah, 16–31.
Sprott, L. R., Harris, M. D., Richardson, J. W., Gray, A. W., Forrest, D. W., Northcutt-Dolezal, S., et al. (1998). Pregnancy to artificial insemination in beef cows as affected by body Condition and number of services. Prof. Animal Sci. 14 (4), 231–235. doi:10.15232/S1080-7446(15)31835-0
Suthar, V. S., and Shah, R. G. (2009). Bovine embryo production: an Overview. Vet. World 2 (12), 478–479.
Thompson-Crispi, K. A., Sargolzaei, M., Ventura, R., Abo-Ismail, M., Miglior, F., Schenkel, F., et al. (2014). A genome-wide association study of immune response traits in Canadian Holstein cattle. BMC Genomics 15 (1), 559. doi:10.1186/1471-2164-15-559
Tillard, E., Humblot, P., Faye, B., Lecomte, P., Dohoo, I., and Bocquier, F. (2008). Postcalving factors affecting conception risk in Holstein dairy cows in tropical and sub-tropical conditions. Theriogenology 69 (4), 443–457. doi:10.1016/j.theriogenology.2007.10.014
Tozer, P. R., and Heinrichs, A. J. (2001). What affects the costs of raising replacement dairy heifers: a multiple-component analysis. J. Dairy Sci. 84 (8), 1836–1844. doi:10.3168/jds.S0022-0302(01)74623-1
Van Tassell, C. P., Wiggans, G. R., and Norman, H. D. (1999). Method R estimates of heritability for milk, fat, and protein yields of United States dairy cattle. J. Dairy Sci. 82 (10), 2231–2237. doi:10.3168/jds.S0022-0302(99)75470-6
Vătăşescu-Balcan, R.-A., Georgescu, S., Manea, M.-A., Dinischiotu, A., Tesio, C.-D., and Costache, M. (2006). A method for diagnosis of deficiency of uridine monphosphate synthase in cattle. Romanian J. Biochem. 43-44, 81–85.
Waldmann, A., Reksen, O., Landsverk, K., Kommisrud, E., Dahl, E., Refsdal, A. O., et al. (2001). Progesterone concentrations in milk fat at first insemination--effects on non-return and repeat-breeding. Anim. Reprod. Sci. 65 (1-2), 33–41. doi:10.1016/s0378-4320(00)00227-x
Wathes, D. C., Clempson, A. M., and Pollott, G. E. (2012). Associations between lipid metabolism and fertility in the dairy cow. Reprod. Fertil. Dev. 25 (1), 48–61. doi:10.1071/rd12272
Weigel, K. A. (2006). Prospects for improving reproductive performance through genetic selection. Anim. Reprod. Sci. 96 (3-4), 323–330. doi:10.1016/j.anireprosci.2006.08.010
Wrenzycki, C., Wells, D., Herrmann, D., Miller, A., Oliver, J., Tervit, R., et al. (2001). Nuclear transfer protocol affects messenger RNA expression patterns in cloned bovine blastocysts. Biol. Reprod. 65 (1), 309–317. doi:10.1095/biolreprod65.1.309
Keywords: cattle, health traits, pregnancy, Holstein cows, early embryo mortality, single-nucleotide polymorphisms, linkage disequilibrium
Citation: Gonzalez Berrios CL, Bowden CF, Saad HM, Bishop JV, Van Campen H, Pinedo P, Hansen TR and Thomas MG (2024) Identification of candidate SNPs associated with embryo mortality and fertility traits in lactating Holstein cows. Front. Genet. 15:1409335. doi: 10.3389/fgene.2024.1409335
Received: 29 March 2024; Accepted: 16 July 2024;
Published: 09 August 2024.
Edited by:
Ning Song, Anhui Agricultural University, ChinaReviewed by:
Liu Yang, Agricultural Research Service (USDA), United StatesCopyright © 2024 Gonzalez Berrios, Bowden, Saad, Bishop, Van Campen, Pinedo, Hansen and Thomas. This is an open-access article distributed under the terms of the Creative Commons Attribution License (CC BY). The use, distribution or reproduction in other forums is permitted, provided the original author(s) and the copyright owner(s) are credited and that the original publication in this journal is cited, in accordance with accepted academic practice. No use, distribution or reproduction is permitted which does not comply with these terms.
*Correspondence: Thomas R. Hansen, dGhvbWFzLmhhbnNlbkBjb2xvc3RhdGUuZWR1
†Present address: Courtney F. Bowden, National Wildlife Research Center, Wildlife Services, Animal and Plant Health Inspection Service, United States Department of Agriculture, Fort Collins, CO, United States
Disclaimer: All claims expressed in this article are solely those of the authors and do not necessarily represent those of their affiliated organizations, or those of the publisher, the editors and the reviewers. Any product that may be evaluated in this article or claim that may be made by its manufacturer is not guaranteed or endorsed by the publisher.
Research integrity at Frontiers
Learn more about the work of our research integrity team to safeguard the quality of each article we publish.