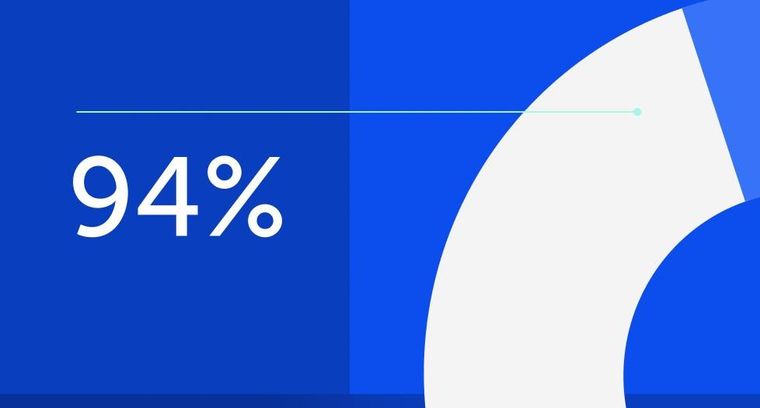
94% of researchers rate our articles as excellent or good
Learn more about the work of our research integrity team to safeguard the quality of each article we publish.
Find out more
BRIEF RESEARCH REPORT article
Front. Genet., 09 April 2024
Sec. Genetics of Common and Rare Diseases
Volume 15 - 2024 | https://doi.org/10.3389/fgene.2024.1390924
Objective: The objective of this study was to pinpoint pathogenic genes and assess the mutagenic pathogenicity in two pediatric patients with hereditary spherocytosis.
Methods: We utilized whole-exome sequencing (WES) for individual analysis (case 1) and family-based trio analysis (case 2). The significance of the intronic mutation was validated through a Minigene splicing assay and supported by subsequent in vitro experiments.
Results: Both probands received a diagnosis of hereditary spherocytosis. WES identified a novel ANK1 c.1504-9G>A mutation in both patients, causing the retention of seven nucleotides at the 5′ end of intron 13, as substantiated by the Minigene assay. This variant results in a premature stop codon and the production of a truncated protein. In vitro studies indicated a reduced expression of the ANK1 gene.
Conclusion: The novel ANK1 c.1504-9G>A variant is established as the causative factor for hereditary spherocytosis, with the c.1504-9G site functioning as a splicing receptor.
Hereditary spherocytosis (HS) or spherocytosis type 1 (MIM: # 182900) is an inherited hemolytic disorder commonly characterized by symptoms of extravascular hemolysis, including anemia, jaundice, and splenomegaly. HS has a global prevalence, with incidences reported as high as 1 in 2,000 in European and North American populations (Bolton-Maggs et al., 2012). In China, a comprehensive review of literature from 1978 to 2013 by Wang et al. (2015) estimated the overall prevalence of HS at approximately 1.37 per 100,000, with a slight gender discrepancy of 1.27 cases per 100,000 in males and 1.49 cases per 100,000 in females, indicating that HS is the most prevalent Mendelian red cell membrane disorder in the country (Tao et al., 2016). Genetic mutations in the ANK1, SPTB, SPTA1, SLC4A1, and EPB42 genes lead to defects in the corresponding ankyrin, β-spectrin, α-spectrin, band 3, and protein 4.2, respectively. These defects result in a decreased erythrocyte membrane surface area, increased osmotic fragility, and ultimately, the transformation of red blood cells from their typical biconcave shape to a spherical morphology. This morphological change predisposes the red blood cells to hemolysis within the spleen (Mohandas and Gallagher, 2008). Currently, China lacks a disease registry system for HS, and there is a significant need for epidemiological data. Although the incidence and detection rates of HS have been on the rise in recent years, misdiagnosis and oversight of the condition remain prevalent (Chen et al., 2020; Gerard et al., 2020; Zhu et al., 2020). In this context, we present two cases of HS attributed to the same novel ANK1 intronic mutation, which we have demonstrated to function as a splicing receptor.
Upon admission, the two unrelated probands displayed varying degrees of anemia. Comprehensive baseline laboratory evaluations were conducted, including assessments of ferritin, iron levels, transferrin, total iron binding capacity, folate, and vitamin B12, glucose-6-phosphate dehydrogenase (G-6-PD) activity, hemoglobin electrophoresis, direct antiglobulin test, thalassemia gene screening, and bone marrow analysis, in accordance with the locally prevalent anemia etiologies. Both cases were thoroughly investigated to rule out common anemia causes such as nutritional deficits, G-6-PD enzyme deficiency, thalassemia, autoimmune hemolytic anemia, and bone marrow hematopoietic disorders.
Following informed consent from the family, 4 mL of EDTA-anticoagulated peripheral venous blood from each child and 2 mL from each parent were collected. These samples were then forwarded to Chigene (Beijing) Translational Medical Research Center Co. Ltd. (Beijing, China) for trio whole-exome sequencing (trio-WES) and subsequent bioinformatic analysis.
WES was performed using the xGen Exome Research Panel v2.0 (Integrated DNA Technologies, United States) to construct an exome library. High-throughput sequencing was carried out on the NovaSeq 6000 platform (Illumina, United States). The sequencing process, including data generation, cleaning, and quality control, adhered to the manufacturer’s recommended protocols, achieving an average sequencing depth of 100X and an exomic coverage of no less than 99%. WES data were subjected to automated bioinformatics analysis through the Chigene Comprehensive Genetic Disease Precision Diagnosis Cloud Platform (https://www.chigene.cn/zaixianfenxipingtai/). This analysis generated insertions/deletions (indels) and single nucleotide variant data of ≤50 bp and flagged copy number variations spanning multiple consecutive exons using proprietary algorithms developed by Chigene. The variant database integrated into the Chigene Cloud Platform, including resources such as dbSNP, ClinVar, HGMD pro, gnomAD, and OMIM, provided annotations for the detected gene variants, including minor allele frequency (MAF), reported pathogenicity cases, literature, and associated diseases of the variant genes. The pathogenicity of gene variants was classified according to the clinical practice guidelines of the American College of Medical Genetics and Genomics (ACMG), and categorized as pathogenic, likely pathogenic, of uncertain significance, likely benign, or benign.
Sanger sequencing was conducted utilizing the ABI3730 (Thermo Fisher Scientific, Waltham, United States) sequencer, adhering to the manufacturer’s protocols. The reference for the ANK1 DNA sequence was NCBI transcript version NM_020475.2.
Minigene fishing techniques were employed to construct the recombinant vectors pcMINI-wt/mut and pcDNA3.1-wt/mut, incorporating restriction sites. These vectors were then transiently transfected into Hela and 293T cell lines. Total RNA was extracted from the cell samples, and PCR amplification was performed using primers flanking the minigene. The transcriptional band size was evaluated by agarose gel electrophoresis and confirmed by sequencing.
The p3Xflag-CMV-7.1-wt vector was engineered using the synthesized whole gene ANK1 CDS as a template, with p3Xflag-CMV-7.1-ANK1-EcoRI-F and p3Xflag-CMV-7.1-ANK1-KpnI-R as primers. Similarly, the p3Xflag-CMV-7.1-mut vector was constructed using p3Xflag-CMV-7.1-ANK1-EcoRI-F and CMV-7.1-ANK1-AfeI-R primers. The integrity of the constructed vectors was confirmed by sequencing.
293T cells were propagated in DMEM supplemented with 10% fetal bovine serum. The cells were then transiently transfected with the constructed wild-type and mutant eukaryotic expression vectors using Lipo2000 reagent, as per the manufacturer’s instructions. After 48 h post-transfection, samples were harvested for quantitative PCR (qPCR) and Western blot (WB) analyses.
Cell samples were collected 48 h post-transfection with the recombinant expression vectors. Total RNA was isolated using the Trizol method, followed by DNA digestion and cDNA synthesis. The qPCR technique was utilized to quantify the expression levels of the wild-type and mutant genes.
Cellular precipitates were obtained 48 h after transfection with the expression vectors. Total cellular protein was extracted using RIPA buffer, and protein concentrations were determined using a BSA assay kit. Subsequent to protein denaturation, Western blot analysis was performed to compare the expression levels of the wild-type and mutant proteins.
The comprehensive clinical features of the probands are delineated in Supplementary Material S1 [including a case previously documented by our group (Wu et al., 2021)]. Both unrelated patients were of Han Chinese descent and exhibited varying degrees of anemia upon admission. All diagnostic evaluations were conducted prior to any splenectomy or blood transfusion procedures.
The ANK1 c.1504-9G>A variant was identified in both individuals, a finding first reported by our team (Wu et al., 2021). For case 1, genetic co-segregation analysis was not feasible due to the unavailability of parental samples. In case 2, the mutation emerged de novo and was classified as likely pathogenic according to ACMG standards. Sanger sequencing confirmation for case 2 is depicted in Figure 1.
Figure 1. Verification of the ANK1 gene variant c.1504-9G>A in Case 2. The red arrow highlights the mutation site confirmed by Sanger sequencing.
The pcMINI-ANK1-wt/mut minigene construct was designed to incorporate a segment of intron 13 (397bp), exon 14 (198bp), and part of intron 14 (207bp) into the pcMINI vector. Post-transfection analysis revealed a spliced sequence spanning ANK1 exon A through exon 14 to exon B. RT-PCR results indicated the presence of a band of the predicted size (587bp), termed band a, in the wild type, and an additional band, referred to as band b, in the mutant type. Sequencing of bands a and b confirmed band a as a normally spliced sequence, following the pattern exon A-exon 14 (198nt)-exon B. Conversely, band b exhibited a 7 nucleotide retention on the right side of intron 13, with a splicing pattern of exon A-▽ intron 13 (7nt)-exon 14 (198nt)-exon B (Figure 2).
Figure 2. Results from the pcMINI constructs. (A) Sequencing chromatograms of minigene constructs, with the wild type (WT) on top and the mutant (MUT) below. (B) Agarose gel electrophoresis of RT-PCR products for transcript analysis. (C) Illustration of the minigene construction strategy and expected splicing products, with bands observed in Hela and 293T cells denoted as a and b, respectively. (D) The sequencing results corresponding to the spliced products. Red * indicates mutation location.
The pcDNA3.1-ANK1-wt/mut minigene strategy involved inserting a fragment encompassing exon 13 (99bp), intron 13 (1103bp), and exon 14 (198bp) into the pcDNA3.1 vector. Transfection was followed by observation of the exon 13-exon 14 splicing pattern for abnormalities. RT-PCR findings revealed a band corresponding to the expected size (507bp), designated as band a in the wild type, and a mutant-specific band b. Sequencing of these bands showed that band a represented a normal splicing sequence, exon 13 (99nt)-exon 14 (198nt). Band b, however, retained an additional 7 nucleotides at the right side of intron 13, with a splicing sequence of exon 13 (99nt)-▽ intron 13 (7nt)-exon 14 (198nt) (Figure 3).
Figure 3. Results from the pcDNA3.1 constructs. (A) Sequencing chromatograms of minigene constructs, with the wild type (WT) on top and the mutant (MUT) below. (B) Agarose gel electrophoresis of RT-PCR products for transcript analysis, with bands in Hela and 293T cells labeled as a and b, respectively. (C) Schematic representation of the minigene construction strategy and expected splicing patterns. (D) The sequencing results corresponding to the spliced products. Red * indicates mutation location.
The eukaryotic expression vectors p3Xflag-CMV-7.1-ANK1-wt/mut were transfected into 293T cells, which were subsequently harvested after 48 h for analysis. Quantitative PCR (qPCR) was utilized to measure the expression levels of ANK1 in the cells, with primers ANK1-3xFLAG-QPCR-F and -R specifically designed for the wild-type and mutant genes. The qPCR data indicated that the expression of the mutant ANK1 gene was reduced to 67% of that observed in the wild-type. Western blot analysis confirmed the presence of proteins at the anticipated molecular weights: 212 kDa for the wild-type construct and 59 kDa for the mutant, indicating the synthesis of a truncated protein. These findings are illustrated in Figure 4.
Figure 4. Gene expression analysis using the p3Xflag-CMV-7.1 vector. (A) Sequencing confirmation of the successful construction of the mutant variant: c.1503_1504insggtccag p.D502Gfs*4. (B) Quantitative PCR (qPCR) detection of mRNA expression levels. (C) Western blot (WB) analysis for the assessment of protein expression.
In this study, both patients exhibited the classic hereditary spherocytosis (HS), as known as spherocytosis type 1 (MIM: # 182900), phenotypes, as outlined in Supplementary Material S1. The etiology of HS is linked to defects in erythrocyte membrane proteins, which lead to a diminished surface area of red blood cells, altered sphericity, increased membrane fragility, and compromised elasticity and stability, all of which are associated with genetic mutations (Manciu et al., 2017). Deficiencies or dysfunctions in these membrane proteins disrupt the vertical connectivity of the membrane’s bilayer skeleton. HS is predominantly caused by mutations in the ANK1, SPTB, SPTA1, SLC4A1, and EPB42 genes, which impact the integrity of ankyrin, β-spectrin, α-spectrin, band 3, and protein 4.2, respectively. Consequently, erythrocytes assume an abnormal spherical shape that predisposes them to hemolysis (Mohandas and Gallagher, 2008).
HS is characterized by a wide range of phenotypic and genotypic variability, with distinct prevalence rates and molecular patterns across various ethnicities and geographic regions. A review by Wang et al. (2021) of the global literature on HS in Chinese patients from 2000 to 2020, which included genetic and clinical data, revealed that ANK1 (46%) and SPTB (42%) mutations are the most common genetic causes of HS, followed by SLC4A1 (11%) and SPTA1 (1%). Notably, no EPB42 mutations were reported in Han Chinese individuals. Among ANK1 defects, most mutations previously identified were frameshift, nonsense, or located at canonical splicing sites (defined as the two nucleotides at both the 5′ donor and 3′ acceptor splice sites), with mutations near splice sites being relatively rare (Aggarwal et al., 2020; Qin et al., 2020; Tole et al., 2020; Wang et al., 2018; Wang et al., 2020; Xie et al., 2021). The discovery of the novel c.1504-9G>A mutation in two unrelated HS patients within this study underscores its pathogenic significance.
The ANK1 gene, comprised of 42 exons and located on chromosome 8p11.2, encodes the ankyrin protein, which consists of 1881 amino acids including the N-terminal 89 kD domain, the central 62 kD domain, and a variable C-terminal regulatory region (Park et al., 2016). Ankyrin plays a pivotal role in membrane stability by anchoring the β-spectrin tail at one end and the band 3 protein at the other, thereby securing the membrane skeleton within the lipid bilayer (Ipsaro et al., 2009). Alterations in the quantity or quality of ankyrin can undermine the connection between the membrane skeleton and the lipid bilayer, causing instability in the lipid bilayer, vesicle formation and lipid loss, reduction in erythrocyte membrane surface area, red blood cell sphericity (Barcellini et al., 2011), and ultimately hemolysis in the spleen.
In our research, we implemented two distinct molecular systems to validate the impact of intronic mutations on gene splicing, providing dual confirmation of our findings. The pcMINI-wt/mut system was utilized to monitor intron retention and exon skipping phenomena, whereas the pcDNA3.1-wt/mut system was primarily focused on detecting intron retention. The results from both systems consistently demonstrated that the ANK1 c.1504-9G>A mutation leads to the retention of 7 nucleotides downstream of intron 13 during the splicing process. Consequently, we speculated that this retention of 7 nucleotides may result in either RNA degradation or the insertion of seven extraneous codons between exons 13 and 14 in the mature mRNA. It is noteworthy that frameshift mutations occurring between exons 13 and 14 have been known to induce premature translation termination, thereby producing a truncated ANK1 protein.
The in vitro experiments conducted as part of this study have provided further insights into the cellular consequences of this particular variant. Our observations revealed that the analogous variant, c.1503_1504insggtccag p.D502Gfs*4, culminates in the generation of a prematurely truncated protein that terminates within exon 14, rather than being subjected to degradation. This truncated protein may potentially possess undefined biological functions. Additionally, the observed downregulation of ANK1 mRNA expression underscores a deficiency in the production of the wild-type ANK1 protein, corroborating the pathogenic nature of the mutation.
In summary, our study uncovered a novel ANK1 c.1504-9G>A variant and established that it leads to the production of a truncated ANK1 protein. Identifying this intronic mutation in proximity to the canonical splicing sites of the ANK1 gene enhances our comprehension of the genotype-phenotype correlations in ANK1-associated hereditary spherocytosis. These findings pave the way for future research into the regulatory mechanisms of ANK1 expression.
The original contributions presented in the study are publicly available. This data can be found here: https://databases.lovd.nl/shared/variants/0000971537#00025918.
The studies involving humans were approved by Ethics Committee of Jiangxi Provincial Children’s Hospital. The studies were conducted in accordance with the local legislation and institutional requirements. Written informed consent for participation in this study was provided by the participants’ legal guardians/next of kin. Written informed consent was obtained from the individual(s), and minor(s)’ legal guardian/next of kin, for the publication of any potentially identifiable images or data included in this article.
CW: Writing–original draft, Writing–review and editing, Conceptualization, Methodology, Funding acquisition, Supervision, Project administration. ZX and FC: Writing–original draft, Writing–review and editing, Formal analysis. YY, QW and HW: Writing–original draft, Writing–review and editing, Investigation. TX: Writing–original draft, Writing–review and editing, Data curation, Validation, Formal analysis.
The authors declare that financial support was received for the research, authorship, and/or publication of this article. Funding for this project was provided by the Jiangxi Provincial Education Department Science and Technology Research Project (Grant No. GJJ2203510) and the Jiangxi Provincial Health Commission Science and Technology Project (Grant No. 202211164).
We are very grateful to the Chigene (Beijing) Translational Medical Research Center Co. Ltd. (Beijing, China) and Zhejiang Saiweisi Biotechnology Co., Ltd. for their assistance with gene technology and experimental procedures.
The authors declare that the research was conducted in the absence of any commercial or financial relationships that could be construed as a potential conflict of interest.
All claims expressed in this article are solely those of the authors and do not necessarily represent those of their affiliated organizations, or those of the publisher, the editors and the reviewers. Any product that may be evaluated in this article, or claim that may be made by its manufacturer, is not guaranteed or endorsed by the publisher.
The Supplementary Material for this article can be found online at: https://www.frontiersin.org/articles/10.3389/fgene.2024.1390924/full#supplementary-material
Aggarwal, A., Jamwal, M., Sharma, P., Sachdeva, M., Bansal, D., Malhotra, P., et al. (2020). Deciphering molecular heterogeneity of indian families with hereditary spherocytosis using targeted next-generation sequencing: first south asian study. Br. J. Haematol. 188 (5), 784–795. [Clinical Trial; Journal Article; Research Support, Non-U.S. Gov't]. doi:10.1111/bjh.16244
Barcellini, W., Bianchi, P., Fermo, E., Imperiali, F. G., Marcello, A. P., Vercellati, C., et al. (2011). Hereditary red cell membrane defects: diagnostic and clinical aspects. Blood Transf. 9 (3), 274–277. [Journal Article; Review]. doi:10.2450/2011.0086-10
Bolton-Maggs, P. H., Langer, J. C., Iolascon, A., Tittensor, P., and King, M. J.General Haematology Task Force of the British Committee for Standards in Haematology (2012). Guidelines for the diagnosis and management of hereditary spherocytosis--2011 update. Br. J. Haematol. 156 (1), 37–49. [Journal Article; Practice Guideline]. doi:10.1111/j.1365-2141.2011.08921.x
Chen, M., Ye, Y. P., Liao, L., Deng, X. L., Qiu, Y. L., and Lin, F. Q. (2020). Hereditary spherocytosis overlooked for 7 years in a pediatric patient with β-thalassemia trait and novel compound heterozygous mutations of SPTA1 gene. Hematology. 25 (1), 438–445. [Case Reports; Journal Article; Video-Audio Media]. doi:10.1080/16078454.2020.1846874
Gerard, D., Bourin, S., Phulpin, A., Picard, V., Steschenko, D., and Perrin, J. (2020). Previously misdiagnosed red cell membrane disorder and familial consequences. Br. J. Haematol. 190 (6), 810. [Case Reports; Journal Article]. doi:10.1111/bjh.16803
Ipsaro, J. J., Huang, L., and Mondragon, A. (2009). Structures of the spectrin-ankyrin interaction binding domains. Blood 113 (22), 5385–5393. [Journal Article; Research Support, N.I.H., Extramural; Research Support, Non-U.S. Gov't; Research Support, U.S. Gov't, Non-P.H.S.]. doi:10.1182/blood-2008-10-184358
Manciu, S., Matei, E., and Trandafir, B. (2017). Hereditary spherocytosis - diagnosis, surgical treatment and outcomes. A literature review. Chirurgia. 112 (2), 110–116. [Journal Article; Review]. doi:10.21614/chirurgia.112.2.110
Mohandas, N., and Gallagher, P. G. (2008). Red cell membrane: past, present, and future. Blood 112 (10), 3939–3948. [Journal Article; Review]. doi:10.1182/blood-2008-07-161166
Park, J., Jeong, D. C., Yoo, J., Jang, W., Chae, H., Kim, J., et al. (2016). Mutational characteristics of ank1 and sptb genes in hereditary spherocytosis. Clin. Genet. 90 (1), 69–78. [Journal Article]. doi:10.1111/cge.12749
Qin, L., Nie, Y., Zhang, H., Chen, L., Zhang, D., Lin, Y., et al. (2020). Identification of new mutations in patients with hereditary spherocytosis by next-generation sequencing. J. Hum. Genet. 65 (4), 427–434. [Clinical Trial; Journal Article]. doi:10.1038/s10038-020-0724-z
Tao, Y. F., Deng, Z. F., Liao, L., Qiu, Y. L., Deng, X. L., Chen, W. Q., et al. (2016). Evaluation of a flow-cytometric osmotic fragility test for hereditary spherocytosis in Chinese patients. Acta Haematol. 135 (2), 88–93. [Evaluation Study; Journal Article; Research Support, Non-U.S. Gov't]. doi:10.1159/000438738
Tole, S., Dhir, P., Pugi, J., Drury, L. J., Butchart, S., Fantauzzi, M., et al. (2020). Genotype-phenotype correlation in children with hereditary spherocytosis. Br. J. Haematol. 191 (3), 486–496. [Journal Article; Research Support, Non-U.S. Gov't]. doi:10.1111/bjh.16750
Wang, C., Cui, Y., Li, Y., Liu, X., and Han, J. (2015). A systematic review of hereditary spherocytosis reported in Chinese biomedical journals from 1978 to 2013 and estimation of the prevalence of the disease using a disease model. INTRACTABLE RARE Dis. Res. 4 (2), 76–81. [Journal Article]. doi:10.5582/irdr.2015.01002
Wang, D., Song, L., Shen, L., Zhang, K., Lv, Y., Gao, M., et al. (2021). Mutational characteristics of causative genes in Chinese hereditary spherocytosis patients: a report on fourteen cases and a review of the literature. Front. Pharmacol. 12, 644352. [Journal Article]. doi:10.3389/fphar.2021.644352
Wang, R., Yang, S., Xu, M., Huang, J., Liu, H., Gu, W., et al. (2018). Exome sequencing confirms molecular diagnoses in 38 Chinese families with hereditary spherocytosis. Sci. China-Life Sci. 61 (8), 947–953. [Journal Article; Research Support, Non-U.S. Gov't]. doi:10.1007/s11427-017-9232-6
Wang, X., Zhang, A., Huang, M., Chen, L., Hu, Q., Lu, Y., et al. (2020). Genetic and clinical characteristics of patients with hereditary spherocytosis in hubei province of China. Front. Genet. 11, 953. [Journal Article]. doi:10.3389/fgene.2020.00953
Wu, C., Xiong, T., Xu, Z., Zhan, C., Chen, F., Ye, Y., et al. (2021). Preliminary study on the clinical and genetic characteristics of hereditary spherocytosis in 15 Chinese children. Front. Genet. 12, 652376. [Journal Article]. doi:10.3389/fgene.2021.652376
Xie, F., Lei, L., Cai, B., Gan, L., Gao, Y., Liu, X., et al. (2021). Clinical manifestation and phenotypic analysis of novel gene mutation in 28 Chinese children with hereditary spherocytosis. Mol. Genet. Genom. Med. 9 (4), e1577. [Journal Article]. doi:10.1002/mgg3.1577
Keywords: ANK1, hereditary spherocytosis, intron retention, whole-exome sequencing, minigene splicing assay
Citation: Xiong T, Xu Z, Wan Q, Chen F, Ye Y, Wang H and Wu C (2024) Identification of a novel ANK1 gene variant c.1504-9G>A and its mechanism of intron retention in hereditary spherocytosis. Front. Genet. 15:1390924. doi: 10.3389/fgene.2024.1390924
Received: 24 February 2024; Accepted: 26 March 2024;
Published: 09 April 2024.
Edited by:
Ammar Husami, Cincinnati Children’s Hospital Medical Center, United StatesReviewed by:
Sitara Roy, Harvard Medical School, United StatesCopyright © 2024 Xiong, Xu, Wan, Chen, Ye, Wang and Wu. This is an open-access article distributed under the terms of the Creative Commons Attribution License (CC BY). The use, distribution or reproduction in other forums is permitted, provided the original author(s) and the copyright owner(s) are credited and that the original publication in this journal is cited, in accordance with accepted academic practice. No use, distribution or reproduction is permitted which does not comply with these terms.
*Correspondence: Chongjun Wu, d3VjaG9uZ2p1bm1lZEAxNjMuY29t
Disclaimer: All claims expressed in this article are solely those of the authors and do not necessarily represent those of their affiliated organizations, or those of the publisher, the editors and the reviewers. Any product that may be evaluated in this article or claim that may be made by its manufacturer is not guaranteed or endorsed by the publisher.
Research integrity at Frontiers
Learn more about the work of our research integrity team to safeguard the quality of each article we publish.