- 1Laboratório de Genética Humana e Médica, Instituto de Ciências Biológicas (ICB), Universidade Federal do Pará (UFPA), Belém, Brazil
- 2Laboratório de Imunologia, Seção de Virologia (SAVIR), Instituto Evandro Chagas, Ananindeua, Brazil
- 3Laboratório de Dermato-Imunologia, Instituto de Ciências Biológicas (ICB), Universidade Federal do Pará (UFPA), Belém, Brazil
Leprosy is an infectious disease primarily caused by the obligate intracellular parasite Mycobacterium leprae. Although it has been considered eradicated in many countries, leprosy continues to be a health issue in developing nations. Besides the social stigma associated with it, individuals affected by leprosy may experience nerve damage leading to physical disabilities if the disease is not properly treated or early diagnosed. Leprosy is recognized as a complex disease wherein socioenvironmental factors, immune response, and host genetics interact to contribute to its development. Recently, a new field of study called epigenetics has emerged, revealing that the immune response and other mechanisms related to infectious diseases can be influenced by noncoding RNAs. This review aims to summarize the significant advancements concerning non-coding RNAs in leprosy, discussing the key perspectives on this novel approach to comprehending the pathophysiology of the disease and identifying molecular markers. In our view, investigations on non-coding RNAs in leprosy hold promise and warrant increased attention from researches in this field.
1 Introduction
Leprosy (also known as Hansen’s disease) is a dermatoneurological disease that progresses to deformities and incapacities if not diagnosed and treated correctly (Britton and Lockwood, 2004; Bhat and Prakash, 2012). The causative agent of leprosy, Mycobacterium leprae, is known for a tropism for the upper respiratory tract, skin macrophages and Schwann cells (SCs) of peripheral nerves, conferring neurodermatological manifestations, including hypopigmented or erythematous cutaneous patches, sensorimotor loss and thickened peripheral nerves (Britton and Lockwood, 2004; Bhat and Prakash, 2012; Fava et al., 2019).
Leprosy is a disease that remains quite stigmatized, constituting a serious public health problem in countries like India, Brazil and Indonesia, where there is still a high prevalence rate (WHO, 2021a). It is important to emphasize that according to WHO data, during the year 2020 there was a decrease in the detection of new cases of the disease by about 37% compared to the previous year due to the pandemic of COVID-19 (WHO, 2021b). As a consequence of the pandemic, leprosy detection and treatment have been seriously affected due to staff shortages, suspension of activities in communities, delayed drug supply, etc. This disruption may result in an increase in cases and individuals with grade 2 disability (G2D) (WHO, 2021b; Paz et al., 2022).
The clinical manifestations of leprosy are presented as a spectrum, being intrinsically related to the host’s immune response against M. leprae (Fitness et al., 2002). Based on clinical, pathological, bacilloscopic and immunological criteria, leprosy can be classified as: tuberculoid (TT), borderline tuberculoid (BT), borderline borderline (BB), borderline lepromatous (BL) and lepromatous (LL) (Ridley and Jopling, 1966). Another type of classification, currently used by the World Health Organization, is based on the number of lesions: patients with up to five lesions are classified as paucibacillary (PB) and, patients with over five lesions, as multibacillary (MB) (WHO, 2019).
Importantly, it is still not entirely clear how immune response initiates in leprosy infection. We know that, at the TT pole, the infection is limited by a strong cell-mediated immunity, guaranteed by Th1 CD4+ cells, that secretes interleukin 2 (IL-2), and interferon (IFN)-γ that enhance macrophages and natural killers (NKs) microbicidal activities; therefore, in TT lesions, a granuloma formation and a low number of bacterial are observed (Modlin, 1994; Misra et al., 1995; Mi et al., 2020). As for LL lesions, the cell-mediated immunity is absent, giving place to Th2 antibody response, that produces IL-4 and IL-5 in abundant levels, which are known to activate B cells to switch antibodies (Mi et al., 2020). However, the humoral immunity against M. leprae is ineffective, allowing the bacilli to multiply in large number and disseminate the disease (Misra et al., 1995; Mi et al., 2020).
It is estimated that only a minority proportion of individuals exposed to M. leprae become infected (Nunzi and Massone, 2012; Alemu Belachew and Naafs, 2019). The explanation for such variability can be addressed to different reasons, including environmental factors, divergence in pathogen burden and human genetic susceptibility (Fava et al., 2019). In the global literature, there is evidence from a wide variety of studies supporting that host genes play an important role in susceptibility to leprosy in its different clinical forms, ranging from a classic twin study in the mid-twentieth century (Brown and Stone, 1958) to more recent Genome-Wide Association Studies (GWAS) (Wang et al., 2016; Gzara et al., 2020), which may elucidate leprosy pathology with the investigation of different immune-related genes (Fitness et al., 2002; Fava et al., 2019; Mi et al., 2020).
Currently, it is known that the immune response and other related mechanisms may be influenced not only by genetic factors but also by epigenetic regulation, which includes the activity of non-coding RNAs (ncRNAs) (Cavalli and Heard, 2019). M. leprae alter host cell functionality to their own advantage to promote survival and generate a suitable environment for replication within the host cell by modifying host epigenome (Niller and Minarovits, 2016; Zhang and Cao, 2019). In the last few years, it has been demonstrated that ncRNAs are broadly involved in the activation or suppression of the expression of distinct gene sets related to leprosy phenotype, which directed to novel knowledge on the role of ncRNAs in immunity generation and disease progression, although much remains to be discovered. Here, we review the recent advances in understanding ncRNAs-mediated regulation on leprosy physiopathology and we discuss their importance as potential biomarkers for this disease.
2 Epigenetics
Epigenetics could be defined as the study of molecules and mechanisms capable of modifying regulation and gene expression, without altering the genomic sequence (Cavalli and Heard, 2019). The main mechanisms related to epigenetic regulation are DNA methylation (Moore et al., 2013), histone modifications (Stillman, 2018) and, as previously mentioned, ncRNAs (Chuang and Jones, 2007; Han and He, 2016). These host mechanisms can become excellent tools for pathogens, providing persistent infections by the downregulation of the immune response through bacterial factors capable of altering various cell signaling pathways (Bierne et al., 2012; O’Connell et al., 2012; Syn et al., 2016).
The ncRNAs are RNA molecules that may have structural, functional and regulatory roles, representing about 80% of the genome (ENCODE Project Consortium, 2012). Non-coding RNAs have the capacity to interact with DNA, RNA, and proteins, and they serve multiple functions. These functions include acting as cis-acting silencers and trans-acting mediators at specific transcriptional loci, as well as participating in post-transcriptional processes, nuclear organization, RNA processing, and the suppression of transposons through sequence complementarity (ENCODE Project Consortium, 2012; Hombach et al., 2016; López-Jiménez and Andrés-León, 2021).
Based on size, biogenesis and structure, ncRNAs are grouped into two major groups: long noncoding RNAs (lncRNAs >200 nt) and small noncoding RNAs (sncRNAs <200 nt) (Charles Richard and Eichhorn, 2018; Bhatti et al., 2021). Currently, there is increasing evidence that deregulation in the transcription and maturation of ncRNAs, incorrect interaction with target mRNAs and mutations in the processing mechanism can increase the risk of neurological, cardiovascular diseases and tumorigenesis (Calin et al., 2004; KAZEMZADEH et al., 2015; Anastasiadou et al., 2018; Sallam et al., 2018; Yan et al., 2018; Grillone et al., 2020). However, there are still few studies on the association of ncRNAs with infectious diseases, such as microRNAs (miRNAs) (Liu et al., 2012a; Jorge et al., 2017; Soares et al., 2017; Salgado et al., 2018), lncRNAs (Fava et al., 2017) and piwi-interacting RNAs (piRNAs) (Pinto et al., 2020) in leprosy (Figure 1) (Cokarić Brdovčak et al., 2018; Schulte et al., 2019; Fathizadeh et al., 2020; Pinto et al., 2020). To date, no original articles have been published addressing the role of ncRNAs in leprosy. Therefore, in the following sessions, we will focus on these classes of ncRNAs and their relation to leprosy. Table 1 presents their main characteristics.
2.1 LncRNAs
Long non-coding RNAs (lncRNAs) are functionally heterogeneous molecules that present a length of at least 200 nt, a lack of protein-coding potential, usually a poly-A tail and that may be spliced, similar to mRNAs (Hombach et al., 2016). They are classified according to the relative location to protein-coding genes as: sense lncRNA, antisense lncRNA, bidirectional lncRNA, intron lncRNA, intergenic lncRNA and enhancer lncRNA (Chi et al., 2019).
LncRNAs have been associated with several functions and represent the largest class of ncRNAs. Contrary to short ncRNAs, which are generally attributed to gene regulation, the mechanistic role of lncRNAs is highly diverse, increasing their functional complexity (Charles Richard and Eichhorn, 2018). At the epigenetic level, lncRNAs can regulate DNA methylation, alter methylation, acetylation, or ubiquitination of histones and reconstruct the chromatin or alter its conformation (Zhang T. et al., 2019). It has been demonstrated that lncRNAs play important roles in stem cell maintenance and differentiation, X-chromosome inactivation, imprinting, maintenance of nuclear architecture, cell autophagy, cell proliferation, apoptosis and embryonic development (Hombach et al., 2016; Qian et al., 2019).
In the immune system, lncRNAs exhibit dynamic expression in cell type, developmental stage, and context-specific manners to coordinate several aspects of immune function (Atianand and Fitzgerald, 2014). The majority of lncRNAs that are associated with infection diseases have been shown to dysregulate expression in different tissues and cells. Several studies have found differentially expressed host lncRNAs in various bacterial infections such as Escherichia coli, Salmonella, Campylobacter concisus and Mycobacterium tuberculosis (Li et al., 2018a; Chi et al., 2019; Ozata et al., 2019; Huang et al., 2020), indicating that lncRNAs can be used as molecular markers of infection associated with pathogenic bacteria.
To date, the only study that has directly related lncRNAs and leprosy was done by Fava and collaborators (Fava et al., 2017). In this genome-wide association study, the authors have identified a lncRNA as a potential risk factor for the development of pathological inflammatory responses in leprosy. Specifically, two risk variants associated with leprosy type-1 reactions (T1R), namely, rs1875147 and rs10826321, are found to be linked to two distinct isoforms of a novel lncRNA. One of these isoforms is encoded by the ENSG00000235140 gene, also known as RP11-135D11.2, while the other is encoded by the uncharacterized LOC105378318 gene. It’s noteworthy that these two genetic variants are situated at positions 6.5 kb and 8.7 kb, respectively, upstream of the transcription start site of the ENSG00000235140 gene. These T1R events manifest as pathological inflammatory responses affecting a specific subgroup of leprosy patients, often leading to peripheral nerve damage. These episodic occurrences significantly contribute to immune-mediated tissue damage in the host and, consequently, play a pivotal role in the development of disability in leprosy (Fava et al., 2017).
Another study, using learning machine to predict leprosy progression amongst household contacts of leprosy patients, identified a group of genes among which three lncRNAs were found (ENSG00000283633, ENSG00000266538 and ENSG00000279227). However, these lncRNAs, along with other pseudogenes were not included for validation of the RNA-Seq signature due the lack of commercially available probes for RTqPCR (Tió-Coma et al., 2021).
Currently, research on lncRNAs in leprosy and other infectious immunity is just beginning when compared to studies on other ncRNAs, such as miRNAs, in this field. One of the major challenges in identifying lncRNAs relates to difficulty in discriminating their pleiotropic functions, as well as understanding the mechanisms by which they interact with other molecules, such as proteins, miRNAs, mRNAs, and circRNAs. Similarly, the interaction of lncRNAs with the immune system has not yet been fully elucidated, being needed more experimental and clinical studies to offer novel approaches for better diagnosis and therapy in the future.
2.2 miRNAs
Among the variety of ncRNAs, the most frequently studied are miRNAs. Discovered as non-coding and post-transcriptional regulators in eukaryotes in the early 1990s (Lee et al., 1993), miRNAs are small, endogenous, stable, and highly conserved among species. MiRNAs are predicted to control the activity of approximately 30% of all protein-coding genes in the human genome (Friedman et al., 2009), and have been shown to participate in the regulation of thousands of genes (Schulte et al., 2019). They are among the most important regulatory molecules of an organism and participate in a variety of biological processes that include the modulation of the immune response during infections (Felekkis et al., 2010; Zhou et al., 2010; Singh RP. et al., 2013; Bhaskaran and Mohan, 2014; Lukasik and Zielenkiewicz, 2019).
However, studies that associate the expression of miRNAs in infectious diseases are still insufficient, especially related to diseases caused by mycobacteria (Singh RP. et al., 2013; Yang and Ge, 2018; Abu-Izneid et al., 2020). It is known that these microorganisms have various routes of infection and can cause diverse immune response based on the cells that are likely infected (Agarwal et al., 2019) For example, the main cell types directly infected by the mycobacteria are macrophages, which are crucial modulators of innate and adaptive immune responses, leading to different immune responses by deregulation of host miRNAs (Singh PK. et al., 2013; Bettencourt et al., 2016).
Macrophages act in the front line of host defense and are a major target of these pathogens (Zhou et al., 2010; Singh RP. et al., 2013). In leprosy, the infection of macrophages results in the recognition by TLR-4 (Polycarpou et al., 2013) and TLR-2/1 (Krutzik et al., 2003), and subsequent direction of adaptive immune responses. Two miRNAs that target PRKCE gene (hsa-miR-1-3p and hsa-miR-31-5p), which in turn is involved in TLR-4 signaling in mycobacterial infections (Hestvik et al., 2005), showed low expression in leprosy tissues of both TT and LL poles through miRNA sequencing (Salgado et al., 2018). On the other hand, Liu et al. (2012b), using microarray analysis, demonstrated that in LL patients, the vitamin D-dependent antimicrobial effects of TLR-2/1 are directly inhibited by the overexpression of hsa-miR-21. This miRNA negatively regulates the expression of IL1B and CYP27B1 genes, activates downstream of TLR-2/1 signaling, and plays a role in the induction of vitamin D immunomodulatory activities. This results in an indirect increase in the production of IL-10, subsequently leading to the inhibition of antimicrobial peptides CAMP and DEFB4A. (Liu et al., 2012b) (Figure 2).
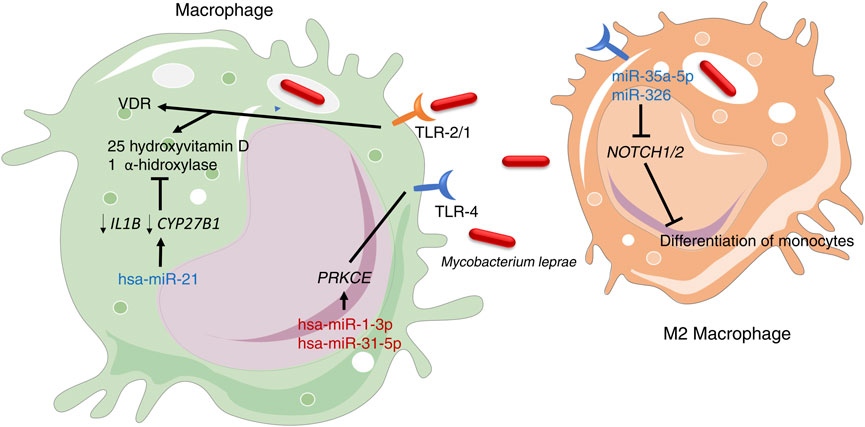
FIGURE 2. TLR-4 and TLR-1/2 are crucial receptors involved in the recognition of M. leprae in macrophages. miRNAs highlighted in blue indicate high expression, while miRNAs in red indicate low expression.
The persistence of M. leprae infection depends on the type of the host immune response and macrophages present in the skin lesions. Macrophages have high plasticity and can polarize to different phenotypes, including M1 (pro-inflammatory profile) and M2 (anti-inflammatory profile), with mostly homeostatic and phagocytic functions (Kibbie et al., 2016). In the skin lesions of LL patients, miR-34a-5p and miR-326 were upregulated, causing the inhibition of NOTCH1/2 expression and preventing the differentiation of monocytes to M1 macrophages (Salgado et al., 2018). Thus, the populations of M2 macrophages were found to be predominant in LL lesions, indicating that the types of macrophages present in lesions are associated with clinical spectrum of leprosy.
To initiate the adaptive response, the interaction between adhesion molecule 1 (CADM1) expressed on the surface of dendritic cells and the molecule associated with MHC class I restricted T cells (CRTAM) coordinates the immunological synapse required for T cell receptor (TCR) activation and polarization in subpopulations of CD4+ T cells (Yeh et al., 2008; Giangreco et al., 2012). MiRNAs can control the bridge between innate and adaptive immune signaling mechanisms and regulatory networks that determine which subpopulations of Th cells will be activated during leprosy (Baumjohann and Ansel, 2013; Kumar et al., 2013). The miR-15a-5 and miR-16-5p, that control the interaction between CADM1 and CRTAM have been shown to be downregulated in all groups of leprosy patients (Salgado et al., 2018). However, the downregulation of miR-181a is associated with the progression of leprosy to BL and LL forms (Kumar et al., 2011). This miRNA is particularly important for TCR signaling for regulating SHP2, a phosphatase that reduces TCR expression and, as a consequence, SHP2 overexpression compromises TCR signaling and specificity of T cells against M. leprae (Kumar et al., 2011).
The regulatory network of miRNAs that operate in differentiation for TCD4+ Th1, TCD4+ Th2, and TCD4+ Th17 cells are still far from being fully comprehended. When overexpressed, miR-125b regulates genes involved in the differentiation of naive TCD4+ cells (e.g., IFNG, IL2RB, IL10RA, and PRDM1); whereas it is downregulated in leprosy patients, it does not seem to prevent the differentiation of TCD4+ in the subpopulations of Th cells (Rossi et al., 2011; Salgado et al., 2018). It has also been previously demonstrated in vitro that the overexpression of miR-155 in TCD4+ cells promote differentiation into Th1 cells, while the its downregulation appears to activate Th2 cells (Banerjee et al., 2010), but, in LL patients, miR-155 was upregulated (Salgado et al., 2018). In a previous study, miR-155 was associated with the repression of SHIP1 and the transcriptional repressor BTB and CNC homology 1 (Bach1), which results in increased activation of Akt (serine/threonine protein kinase B) and favors the survival of mycobacteria inside cells (Kumar et al., 2012; Rothchild et al., 2016). However, these results are still quite controversial, since studies show that miR-155 is also able to promote differentiation into Th17 cells through regulation of the JARID2 gene (Escobar et al., 2014; Xu et al., 2017; Zhu et al., 2020).
The Th17 subset is usually found in patients with unstable leprosy, where there is absence of Th1 and Th2 polarization, and is also associated with T1R leprosy reactions (Nath et al., 2015; Saini et al., 2016). Similar to miR-155, the miRNA hsa-miR-326, which is reported to be an important inductor of differentiation of Th17 cells (Du et al., 2009; Karimi et al., 2020) was also found upregulated in LL (Salgado et al., 2018). On the other hand, some studies report the importance of miR-326 as a regulator of apoptosis in neuronal cells, able to inhibit JNK and MAPK signaling pathways when overexpressed (Zhang Y. et al., 2019; He et al., 2020). From an immunological point of view, apoptosis is a form of programmed cell death (PCD) that functions as a defense against infections, involving in the death of infected macrophages (Ottenhoff, 2012). Modulation of apoptosis can influence the course of infection, allowing intracellular pathogens to survive, and may be an important mechanism in the development of the various clinical forms of leprosy, as they are influenced by the bacillary load (Brito de Souza et al., 2010; Galluzzi et al., 2017).
Several miRNAs that target Caspase-8 (CASP-8) inductor are upregulated in the two leprosy extreme poles. Apoptosis-related genes like MYC (miR-34a-5p and miR-155-5p), FAS (miR-196b-5p and miR-146a-5p) and FADD (miR-155-5p and miR-146a-5p) are upregulated only in LL patients, while miR-126-3p, miR-15a-5p, miR-20a-5p and miR-16-5p, that target BCL-2, an antiapoptotic gene, and miR-193a-3p and miR-133a-3p, that target MCL1, a member of BCL-2 family, were downregulated (Salgado et al., 2018). In LL lesions, miRNAs that target the pro-apoptotic gene YAP1 (miR-200a-3p and miR-375) and miRNAs that target AKT1 (miR-199a-3p and miR-708-5p) were all downregulated. AKT1 is a known suppressor of the apoptotic machinery, including YAP1 and FOX03. TP53 also presented its miRNAs (IAmiR-200a-3p and miR-375) downregulated in LL lesions, but it has its functions blocked through activation of RBL1/2, that are activated after inhibition of FOX03, besides, AKT1 also stimulates MDM4, a regulator of TP53 (Figure 3). Therefore, M. leprae can impact macrophages miRNAs expression levels, thus altering apoptosis to save itself from intracellular death (Salgado et al., 2018).
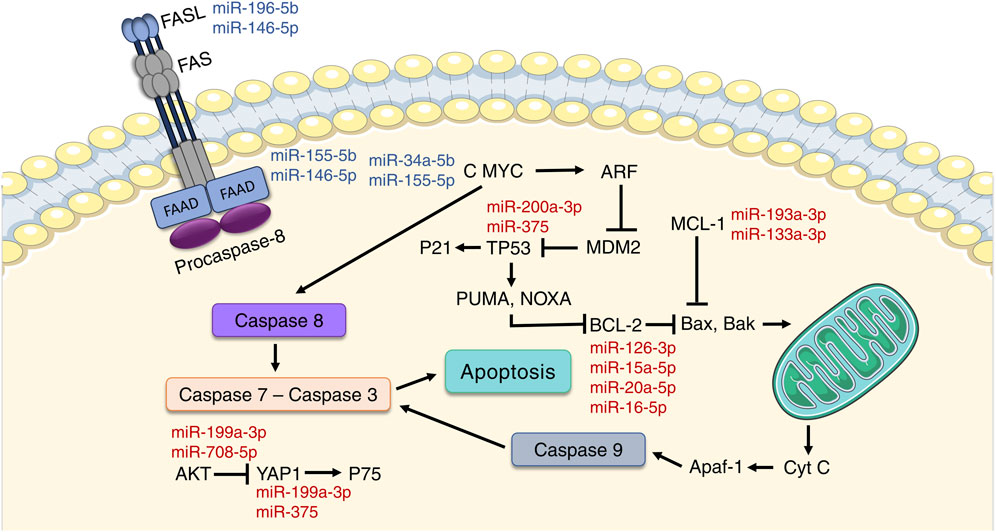
FIGURE 3. MicroRNAs regulates apoptosis-associated genes in leprosy. MicroRNAs are able to modulate both extrinsic and intrinsic apoptosis pathways during the infection process. The miRNAs that regulate the expression of genes associated with the intrinsic apoptotic pathway (FASL, FAS, FADD, and CASP8) are upregulated, while the miRNAs that control intrinsic signaling, highlighting the antiapoptotic gene BCL-2, are downregulated, demonstrating the anti-apoptotic profile of cells infected by M. leprae. miRNAs highlighted in blue indicate high expression, while miRNAs in red indicate low expression.
In addition to understanding about the immunopathophysiology of leprosy, some studies have also sought biomarker miRNAs that are accessible and reliable. Jorge et al. (2017) studied sets of miRNAs differentially expressed by low-density array in skin lesions of LL and TT patients, with the expression levels of seven miRNAs (miR-125b, miR-196b, miR-27b, miR-29c, miR-425-5p, miR-502-3p, and miR-92a), and among these four miRNAs were selected (miR-101, miR-196b, miR-27b, and miR-29c) from evaluation by their ability to distinguish between group of patients and control in the first stage, and group of LL and TT in a second stage. The combination of miRNAs miR-101, miR-196b, miR-27b and miR-29c show 80% sensitivity and 91% specificity (AUC 87%) in discriminating patients with leprosy, and was also able to discriminate between TT and LL with 83% sensitivity and 80% specificity (AUC 83%).
In another study carried out by Soares and collaborators (Soares et al., 2017), comparing clinical forms (TT, BT, BB, BL, and LL) and a control group, twenty miRNAs were found differentially expressed, while in polar forms (TT and LL) only one miRNA was identified (hsa-miR181a). The hsa-miR181a is upregulated across the spectrum of leprosy and reaction forms, with greater expression of the polar forms TT and LL, which may indicate importance in the pathophysiological process of the disease (Soares et al., 2017). It is known that hsa-miR-181a is capable of regulating the sensitivity of T cells, allowing mature T cells to recognize antagonists as agonists (Li et al., 2007; Amado et al., 2020). The higher expression of hsa-miR-181a is correlated with greater sensitivity of T cells in immature T cells, suggesting that this miRNA acts as a rheostat of intrinsic sensitivity to the antigen (Li et al., 2007; Soares et al., 2017). Of the sixty-four miRNAs, eight were validated, seven of which were upregulated (hsa-miR-142-3p, hsa-miR-142-5p, hsa-miR-146b-5p, hsa-miR-342-3p, hsa-miR-361 -3p, hsa-miR-3653, and hsa-miR-484) and one were downregulated (hsa-miR-1290) (Soares et al., 2017).
In other study, da Silva MNS et al. (2022) found association of twenty-five genetic variants in miRNAs and miRNA machinery-related genes (DROSHA and AGO1) with leprosy susceptibility in a population from the Amazon region. In the association analysis between leprosy patients and healthy individuals, they found six significant markers for the risk of developing leprosy in the population studied: rs2505901 (pre-mir938), rs639174 (DROSHA), rs636832 (AGO1), rs10739971 (pri-let -7a1), rs12904 (miR200C) and rs10035440 (DROSHA).
Comparing leprosy patients grouped according to the PB form with the control group, they found a significant association in SNPs rs2505901 (pre-miR938), rs10739971 (pri-let-7a1), rs12904 (miR200C) and rs2168518 (miR4513) (da Silva MNS et al., 2022). The marker rs2505901 (pre-miR938) was associated with a decreased risk of PB leprosy in recessive and dominant models and changes in miR938 biogenesis and stability, in addiction to be associated with regulatory pathways related to cell survival and apoptosis (Nath et al., 2015; Zhu et al., 2020). On the other hand, SNPs rs10739971 (pri-let-7a1) and rs12904 (miR200C) were associated with increased risk of PB in a dominant model and rs2168518 (miR4513) in recessive model (da Silva MNS et al., 2022).
Comparing genotypes of patients grouped according to MB clinical form with the control group, SNPs rs639174 (DROSHA), rs636832 (AGO1) and rs4143815 (miR570) were associated with a reduced risk of MB leprosy using a dominant model (da Silva MNS et al., 2022). The rs639174 variant (DROSHA) and the rs4143815 variant (miR570) is an intronic SNP with a recognized role in transcriptional regulation and are involved in the regulation of the inflammatory response, respectively, and in this study this variants was associated with protection against leprosy per se and MB (Du et al., 2009; Saini et al., 2016; Zhu et al., 2020). On the other hand, SNP rs10035440 (DROSHA), which also plays an important role in the splicing and transcriptional regulation of the DROSHA gene, was associated with increased disease risk in a dominant model (Saini et al., 2016; Zhu et al., 2020).
In a genotype comparison analysis between PB and MB patients, only SNP rs10739971 (pri-let-7a1) showed an association with the development of leprosy per se and the PB form, with an association between groups of patients (PB versus MB), with increased risk to the PB form (106). While the rs2910164 SNP (miR146A) was associated with a decreased risk of leprosy in the PB form in a recessive model and consequent susceptibility to risk of MB leprosy (da Silva MNS et al., 2022).
Among ncRNAs, miRNAs are the most studied in leprosy, with studies aiming at basic knowledge of how these molecules act in the immune response and pathophysiology of the disease, aiming at new therapeutic targets aimed at the interaction between bacillus/host and accessible biomarkers. However, many of the miRNAs found require validation and functional analysis to assess their roles in the pathogenesis of leprosy.
2.3 piRNAs
PIWI-interacting RNAs (piRNAs) represent the most abundant and diverse group of sncRNAs (Ku and Lin, 2014), with more than 30,000 piRNAs molecules identified in the human genome (Chalbatani et al., 2019). Several genomic loci, defined as piRNA clusters, can transcribe pri-piRNA sequences (Brennecke et al., 2007), that unlike miRNA and siRNA, are processed through Dicer-independent mechanisms, to mature piRNAs (Vagin et al., 2006). Structurally, piRNAs are single-stranded molecules, typically 24–32 nucleotides in length, characterized by 2′-O-methyl-modified 3′ termini. These sncRNAs interact with proteins of the PIWI family, a sub-group of the AGO proteins, forming the piRNA-induced silencing complex (piRISC) (Siomi et al., 2011).
The involvement of piRNAs in the innate and adaptative immune response is not a well-established concept as for miRNAs, and few studies with controversial results have pointed out a possible relationship between the expression of piRNAs and the response to viral infections (Petit et al., 2016). Despite this, the pioneer work by Pinto et al. (2020) focused on the global changes in the piRNA expression profile (piRNome) of leprosy skin lesions, and detected 337 piRNAs in human skin, of which five piRNAs (piR-hsa-28634, piR-hsa-1580, piR-hsa-27007, piR-hsa- 21131, piR-hsa-12454) were differentially expressed (DE) in leprosy tissues when compared with healthy tissues (HS) (TT + LL vs. HS).
Other piRNAs were exclusively DE to the different leprosy poles: eight DE piRNAs (piR-hsa-23327, piR-hsa-23655, piR-hsa-2153, piR-hsa-12790, piR-hsa-31280, piR-hsa-28394, piR-hsa-27283, piR-hsa-23289) were found only in TT and three DE piRNAs (piR-hsa-23919, piR-hsa-26131, piR-hsa-15215) were found only in LL leprosy (Pinto et al., 2020). This indicates that piRNA expression profiles can distinguish leprosy tissue from a non-leprosy tissue, even more, can distinguish leprosy between its TT and LL poles, therefore, these piRNAs are attractive biomarkers for leprosy.
Under normal physiological conditions, the piRNAs are produced at stable levels (Story et al., 2019), however, in leprosy skin lesions all but one of DE piRNAs (piR-hsa-27283) are downregulated, emphasizing epigenetic alterations in leprosy (Pinto et al., 2020). Considering that, piR-hsa-27283, was the only piRNA upregulated, it may be useful as a risk biomarker of leprosy, more specifically, for TT patients, since it was DE for this pole, and as leprosy poles have different immunological and molecular features, it is suggested that piR-hsa-27283 functions can have a significant impact on progression to TT leprosy. On the other hand, its role in leprosy is unclear, as it may has multiple gene targets, thus making its analysis difficult (Pinto et al., 2020).
To better clarify the functional and mechanistic features of piRNAs in leprosy context, the authors built a piRNA-gene regulatory network, based on DE piRNAs putative target genes, which revealed that, in general, DE piRNAs regulate genes involved in leprosy-related processes, such as, programmed cell death (i.e., apoptosis, autophagic cell death), immune response, neural and epidermal regeneration (Pinto et al., 2020).
Lines of evidences suggest that immunological response generated during M. leprae, induces the apoptosis of infected cells, mainly by the secretion of pro-apoptotic cytokines, such as TNF and IFN, which are markedly dominant in TT lesions (Jin et al., 2018). However, synergistically with miRNAs, the piRNAs regulate anti-apoptotic pathways, via CARF activation, an inhibitor of caspase-dependent apoptosis, allowing a favorable condition to bacterial survival (Pinto et al., 2020).
An important question is if piRNAs are downregulated in leprosy biopsies and considering that SCs of peripheral nerves possess genomic plasticity and high regenerate capacity guaranteed by SOX10 and ERBB gene family, why in leprosy the regrowth of axons and SCs is inhibited? (Tapinos et al., 2006; Hess and Rambukkana, 2019). Recently, Masaki et al. (2013) demonstrated that the leprosy bacterium hijacks this regenerative property and turn-off myelination-associated genes by DNA methylation of SOX10 promotor region, a regulator of SCs differentiation and myelination, thus, the cells remain in an undifferentiated stage and become capable to migrate to other tissues, which facilitated the spread of M. leprae to other niches, such as skeletal and smooth muscle, and also contribute to granuloma formation that subsequently release of M. leprae-laden macrophages.
Another important mechanism for SCs regeneration is the recruitment of pro-regenerative macrophages. The pro-regenerative macrophages function to clear axonal and myelin debris, persisting in nerve microenvironment to guide remyelination and SC differentiation after nerve injury, which mechanism are regulated by growth arrest specific 6 (GAS6) and IL-6 (Stratton et al., 2018). It is proposed that the downregulation of piRNAs (piR-hsa-12454, piR-hsa-1580, piR-hsa-2153, piR-hsa-23289) that target IL6R to culminate in the expression of this receptor in pro-regenerative macrophages and active the IL-6, a well-known neuropathic biomarker in leprosy patients (Pinto et al., 2020). The IL-6 in turn, stimulates pro-regenerative macrophages to produce GAS6 that promotes better SCs remyelination within the injured nerve (Stratton et al., 2018).
Unfortunately, the work of Pinto and collaborators (Pinto et al., 2020) is the only one study available in the specialized literature that aimed to understand the involvement of piRNAs pathway in leprosy. Clearly, there is still a gap in this regard, but as above mentioned these data are important to understand the epigenetic control of genes that participate in leprosy immunophysiopathology. It is believed that future studies may reveal mechanisms to reactivate neural regeneration genes, in this case, the expression of piRNAs should also be modulated to avoid inhibiting regeneration.
2.4 circRNAs
CirRNAs are ncRNAs produced from an alternative splicing of the precursor mRNA (pre-mRNA), performed in the spliceosome, called back-splicing, in which the 3′end of an exon connects to the 5′end of an upstream exon via a 3′, 5′-phosphodiester bond, forming a closed linker structure with a back-splicing junction site (Li et al., 2018b; Chen, 2020; Zhou et al., 2020).
Some circular RNAs play an important role in gene regulation, modulating transcription and splicing, as well as in pathophysiological processes, acting as a miRNA sponge, which further suppress transcription and lead to gene silencing, as well as interacting with proteins and acting as models for polypeptide synthesis (Ojha et al., 2018; Chen, 2020). In addition, CircRNAs are involved in innate immunity, cell proliferation and transformation and neuronal function, and their dysregulation is related to the malfunction of physiological processes, resulting in several pathological conditions, such as the development of cancer, neurodegenerative diseases, tuberculosis and other infectious diseases (Li et al., 2018b; Ojha et al., 2018; Chen, 2020), but its involvement in leprosy is still unclear.
One of the only studies that associated circRNAs with leprosy was the work carried out by Gao et al. (2022) where they developed a circRNA–miRNA–mRNA network using MLO-Y4 murine osteocyte-like cells treated with N-glycosylated MDP (N.g MDP) to elucidate bone remodeling activity in leprosy. In this study, 724 differentially expressed mRNAs and circRNAs were observed between samples treated with control and N.g MDP, with 579 upregulated genes and 145 downregulated genes in differentially expressed mRNAs and 309 upregulated and 415 downregulated circRNAs, in addition to 58 pairs of circRNA–miRNA–mRNA interaction (Gao et al., 2022).
3 Perspectives
Noncoding RNA are implicated in a wide variety of human diseases, including infectious diseases. Recently, the use of RNA technology against COVID-19 has caused a “boom” in the study of ncRNAs as transcription modulators. NcRNAs have also been identified as important novel regulators of infectious disease risk factors and cell functions and are thus important candidates to improve diagnostics assessment. Beyond their application in diagnostic, ncRNA can also be the targets or tools of novel therapeutic strategies.
In recent years we have seen the growing number of studies related to ncRNAs, however there are still few studies related to leprosy. There is also a knowledge gap regarding the role of host ncRNAs in the etiology, diagnosis and development of vaccines against neglected human diseases. The expression profile of ncRNAs is a key element explored in the development of diagnostic biomarkers with greater sensitivity and specificity and reliable prognoses in leprosy. There is a lot to improve our understanding of leprosy pathophysiology. Therefore, future work should be developed to provide critical information for the development of preventive and therapeutic proposals, using ncRNAs as biomarkers for this neglected disease. We provide an update on recent developments and perspectives for diagnostic use of ncRNAs in leprosy diseases and new therapeutic targets in different forms of leprosy infection.
Author contributions
MS-d-S: Conceptualization, Investigation, Writing–original draft, Writing–review and editing. CS-d-S: Conceptualization, Investigation, Writing–review and editing. MC-D: Conceptualization, Investigation, Writing–review and editing. FS: Conceptualization, Investigation, Writing–review and editing. AG: Writing–review and editing. PP: Supervision, Writing–review and editing. CS: Supervision, Writing–review and editing. SdS: Supervision, Writing–review and editing.
Funding
The author(s) declare financial support was received for the research, authorship, and/or publication of this article. This study was supported by the Brazilian National Research Council (CNPq) 407922/2021-0, the Coordination for Higher Education Personnel Training (CAPES) 3381/2013, the Amazonian Research Foundation (FAPESPA), the VALE S.A. 27756/2019 and by the PROPESP/PROEX/UFPA. None of these organizations had any role in the design of the study, collection or analysis of the data, the decision to publish the manuscript, or its preparation. The contents of this study are the responsibility of the authors.
Conflict of interest
The authors declare that the research was conducted in the absence of any commercial or financial relationships that could be construed as a potential conflict of interest.
Publisher’s note
All claims expressed in this article are solely those of the authors and do not necessarily represent those of their affiliated organizations, or those of the publisher, the editors and the reviewers. Any product that may be evaluated in this article, or claim that may be made by its manufacturer, is not guaranteed or endorsed by the publisher.
References
Abu-Izneid, T., AlHajri, N., Mohammed Ibrahim, A., Noushad Javed, M., Mustafa Salem, K., Hyder Pottoo, F., et al. (2020). Micro-RNAs in the regulation of immune response against SARS COV-2 and other viral infections. J. Adv. Res. 30, 133–145. doi:10.1016/j.jare.2020.11.013
Agarwal, R. G., Sharma, P., and Nyati, K. K. (2019). microRNAs in mycobacterial infection: modulation of host immune response and apoptotic pathways. Immune Netw. 19, e30. doi:10.4110/in.2019.19.e30
Alemu Belachew, W., and Naafs, B. (2019). Position statement: LEPROSY: diagnosis, treatment and follow-up. J. Eur. Acad. Dermatol Venereol. 33, 1205–1213. doi:10.1111/jdv.15569
Amado, T., Amorim, A., Enguita, F. J., Romero, P. V., Inácio, D., de Miranda, M. P., et al. (2020). MicroRNA-181a regulates IFN-γ expression in effector CD8+ T cell differentiation. J. Mol. Med. 98, 309–320. doi:10.1007/s00109-019-01865-y
Anastasiadou, E., Jacob, L. S., and Slack, F. J. (2018). Non-coding RNA networks in cancer. Nat. Rev. Cancer 18, 5–18. doi:10.1038/nrc.2017.99
Atianand, M. K., and Fitzgerald, K. A. (2014). Long non-coding RNAs and control of gene expression in the immune system. Trends Mol. Med. 20, 623–631. doi:10.1016/j.molmed.2014.09.002
Banerjee, A., Schambach, F., DeJong, C. S., Hammond, S. M., and Reiner, S. L. (2010). Micro-RNA-155 inhibits IFN-γ signaling in CD4+ T cells. Eur. J. Immunol. 40, 225–231. doi:10.1002/eji.200939381
Baumjohann, D., and Ansel, K. M. (2013). MicroRNA-mediated regulation of T helper cell differentiation and plasticity. Nat. Rev. Immunol. 13, 666–678. doi:10.1038/nri3494
Berto da Silva Prata, R., Garcia de Mattos Barbosa, M., Jorge de Andrade Silva, B., Araujo da Paixão de Oliveira, J., Lameira Bittencourt, T., and Olmo Pinheiro, R. (2020). “Macrophages in the pathogenesis of leprosy,” in Macrophage activation - biology and disease. Editor K. Hussain Bhat (London, UK: IntechOpen). doi:10.5772/intechopen.88754
Bettencourt, P., Pires, D., and Anes, E. (2016). Immunomodulating microRNAs of mycobacterial infections. Tuberc. (Edinb) 97, 1–7. doi:10.1016/j.tube.2015.12.004
Bhaskaran, M., and Mohan, M. (2014). MicroRNAs: history, biogenesis, and their evolving role in animal development and disease. Vet. Pathol. 51, 759–774. doi:10.1177/0300985813502820
Bhat, R. M., and Prakash, C. (2012). Leprosy: an overview of pathophysiology. Interdiscip. Perspect. Infect. Dis. 2012, 181089. doi:10.1155/2012/181089
Bhatti, G. K., Khullar, N., Sidhu, I. S., Navik, U. S., Reddy, A. P., Reddy, P. H., et al. (2021). Emerging role of non-coding RNA in health and disease. Metab. Brain Dis. 36, 1119–1134. doi:10.1007/s11011-021-00739-y
Bierne, H., Hamon, M., and Cossart, P. (2012). Epigenetics and bacterial infections. Cold Spring Harb. Perspect. Med. 2, a010272. doi:10.1101/cshperspect.a010272
Brennecke, J., Aravin, A. A., Stark, A., Dus, M., Kellis, M., Sachidanandam, R., et al. (2007). Discrete small RNA-generating loci as master regulators of transposon activity in Drosophila. Cell 128, 1089–1103. doi:10.1016/j.cell.2007.01.043
Brito de Souza, V. N., Nogueira, M. E. S., Belone, A. de F. F., and Soares, C. T. (2010). Analysis of apoptosis and Bcl-2 expression in polar forms of leprosy. FEMS Immunol. Med. Microbiol. 60, 270–274. doi:10.1111/j.1574-695X.2010.00746.x
Britton, W. J., and Lockwood, D. N. J. (2004). Leprosy. Lancet 363, 1209–1219. doi:10.1016/S0140-6736(04)15952-7
Brown, J. a. K., and Stone, M. M. (1958). Tuberculoid leprosy in identical twins. Lepr. Rev. 29, 53–55. doi:10.5935/0305-7518.19580005
Calin, G. A., Liu, C.-G., Sevignani, C., Ferracin, M., Felli, N., Dumitru, C. D., et al. (2004). MicroRNA profiling reveals distinct signatures in B cell chronic lymphocytic leukemias. Proc. Natl. Acad. Sci. U. S. A. 101, 11755–11760. doi:10.1073/pnas.0404432101
Carithers, L. J., Ardlie, K., Barcus, M., Branton, P. A., Britton, A., Buia, S. A., et al. (2015). A novel approach to high-quality postmortem tissue procurement: the GTEx Project. Biopreservation Biobanking 13, 311–319. doi:10.1089/bio.2015.0032
Cavalli, G., and Heard, E. (2019). Advances in epigenetics link genetics to the environment and disease. Nature 571, 489–499. doi:10.1038/s41586-019-1411-0
Chalbatani, G. M., Dana, H., Memari, F., Gharagozlou, E., Ashjaei, S., Kheirandish, P., et al. (2019). Biological function and molecular mechanism of piRNA in cancer. Pract. Lab. Med. 13, e00113. doi:10.1016/j.plabm.2018.e00113
Charles Richard, J. L., and Eichhorn, P. J. A. (2018). Platforms for investigating LncRNA functions. SLAS Technol. Transl. Life Sci. Innov. 23, 493–506. doi:10.1177/2472630318780639
Chen, L.-L. (2020). The expanding regulatory mechanisms and cellular functions of circular RNAs. Nat. Rev. Mol. Cell Biol. 21, 475–490. doi:10.1038/s41580-020-0243-y
Chi, Y., Wang, D., Wang, J., Yu, W., and Yang, J. (2019). Long non-coding RNA in the pathogenesis of cancers. Cells 8, 1015. doi:10.3390/cells8091015
Chuang, J. C., and Jones, P. A. (2007). Epigenetics and MicroRNAs. Pediatr. Res. 61, 24R–29R. doi:10.1203/pdr.0b013e3180457684
Cokarić Brdovčak, M., Zubković, A., and Jurak, I. (2018). Herpes simplex virus 1 deregulation of host MicroRNAs. Non-Coding RNA 4, 36. doi:10.3390/ncrna4040036
da Silva Mns, da Veiga Borges Leal, D. F., Sena, C., Pinto, P., Gobbo, A. R., da Silva, M. B., Salgado, C. G., et al. (2022). Association between SNPs in microRNAs and microRNAs-machinery genes with susceptibility of leprosy in the Amazon population. IJMS 23, 10628. doi:10.3390/ijms231810628
Du, C., Liu, C., Kang, J., Zhao, G., Ye, Z., Huang, S., et al. (2009). MicroRNA miR-326 regulates TH-17 differentiation and is associated with the pathogenesis of multiple sclerosis. Nat. Immunol. 10, 1252–1259. doi:10.1038/ni.1798
Encode Project Consortium, (2012). An integrated encyclopedia of DNA elements in the human genome. Nature 489, 57–74. doi:10.1038/nature11247
Escobar, T. M., Kanellopoulou, C., Kugler, D. G., Kilaru, G., Nguyen, C. K., Nagarajan, V., et al. (2014). miR-155 activates cytokine gene expression in Th17 cells by regulating the DNA-binding protein Jarid2 to relieve polycomb-mediated repression. Immunity 40, 865–879. doi:10.1016/j.immuni.2014.03.014
Fathizadeh, H., Hayat, S. M. G., Dao, S., Ganbarov, K., Tanomand, A., Asgharzadeh, M., et al. (2020). Long non-coding RNA molecules in tuberculosis. Int. J. Biol. Macromol. 156, 340–346. doi:10.1016/j.ijbiomac.2020.04.030
Fava, V. M., Dallmann-Sauer, M., and Schurr, E. (2019). Genetics of leprosy: today and beyond. Hum. Genet. 139, 835–846. doi:10.1007/s00439-019-02087-5
Fava, V. M., Manry, J., Cobat, A., Orlova, M., Thuc, N. V., Moraes, M. O., et al. (2017). A genome wide association study identifies a lncRna as risk factor for pathological inflammatory responses in leprosy. PLOS Genet. 13, e1006637. doi:10.1371/journal.pgen.1006637
Felekkis, K., Touvana, E., Stefanou, C., and Deltas, C. (2010). microRNAs: a newly described class of encoded molecules that play a role in health and disease. Hippokratia 14, 236–240.
Fitness, J., Tosh, K., and Hill, A. V. S. (2002). Genetics of susceptibility to leprosy. Genes Immun. 3, 441–453. doi:10.1038/sj.gene.6363926
Friedman, R. C., Farh, K. K.-H., Burge, C. B., and Bartel, D. P. (2009). Most mammalian mRNAs are conserved targets of microRNAs. Genome Res. 19, 92–105. doi:10.1101/gr.082701.108
Galluzzi, L., Buqué, A., Kepp, O., Zitvogel, L., and Kroemer, G. (2017). Immunogenic cell death in cancer and infectious disease. Nat. Rev. Immunol. 17, 97–111. doi:10.1038/nri.2016.107
Gao, Z.-R., Liu, Q., Zhao, J., Zhao, Y.-Q., Tan, L., Zhang, S.-H., et al. (2022). A comprehensive analysis of the circRNA–miRNA–mRNA network in osteocyte-like cell associated with Mycobacterium leprae infection. PLoS Negl. Trop. Dis. 16, e0010379. doi:10.1371/journal.pntd.0010379
Giangreco, A., Hoste, E., Takai, Y., Rosewell, I., and Watt, F. M. (2012). Epidermal Cadm1 expression promotes autoimmune alopecia via enhanced T cell adhesion and cytotoxicity. J. Immunol. 188, 1514–1522. doi:10.4049/jimmunol.1003342
Grillone, K., Riillo, C., Scionti, F., Rocca, R., Tradigo, G., Guzzi, P. H., et al. (2020). Non-coding RNAs in cancer: platforms and strategies for investigating the genomic “dark matter.”. J. Exp. Clin. Cancer Res. 39, 117. doi:10.1186/s13046-020-01622-x
Gzara, C., Dallmann-Sauer, M., Orlova, M., Van Thuc, N., Thai, V. H., Fava, V. M., et al. (2020). Family-based genome-wide association study of leprosy in Vietnam. PLoS Pathog. 16, e1008565. doi:10.1371/journal.ppat.1008565
Han, Y., and He, X. (2016). Integrating Epigenomics into the understanding of biomedical insight. Bioinform Biol. Insights 10, 267–289. doi:10.4137/BBI.S38427
He, B., Chen, W., Zeng, J., Tong, W., and Zheng, Z. (2020). MicroRNA-326 decreases tau phosphorylation and neuron apoptosis through inhibition of the JNK signaling pathway by targeting VAV1 in Alzheimer’s disease. J. Cell. physiology 235, 480–493. doi:10.1002/jcp.28988
Hess, S., and Rambukkana, A. (2019). Cell biology of intracellular adaptation of Mycobacterium leprae in the peripheral nervous system. Microbiol. Spectr. 7. doi:10.1128/microbiolspec.BAI-0020-2019
Hestvik, A. L. K., Hmama, Z., and Av-Gay, Y. (2005). Mycobacterial manipulation of the host cell. FEMS Microbiol. Rev. 29, 1041–1050. doi:10.1016/j.femsre.2005.04.013
Hombach, S., and Kretz, M. (2016). “Non-coding RNAs: classification, biology and functioning,” in Non-coding RNAs in colorectal cancer. Advances in experimental medicine and biology. Editors O. Slaby, and G. A. Calin (Cham, Germany: Springer International Publishing), 3–17. doi:10.1007/978-3-319-42059-2_1
Huang, A., Zheng, H., Wu, Z., Chen, M., and Huang, Y. (2020). Circular RNA-protein interactions: functions, mechanisms, and identification. Theranostics 10, 3503–3517. doi:10.7150/thno.42174
Iwasaki, Y. W., Siomi, M. C., and Siomi, H. (2015). PIWI-interacting RNA: its biogenesis and functions. Annu. Rev. Biochem. 84, 405–433. doi:10.1146/annurev-biochem-060614-034258
Jin, S.-H., Ahn, K. J., and An, S. (2018). Importance of the immune response to Mycobacterium leprae in the skin. Biomed. Dermatol. 2, 1. doi:10.1186/s41702-017-0012-5
Jorge, KTOS, Souza, R. P., Assis, M. T. A., Araújo, M. G., Locati, M., Jesus, A. M. R., et al. (2017). Characterization of MicroRNA expression profiles and identification of potential biomarkers in leprosy. J. Clin. Microbiol. 55, 1516–1525. doi:10.1128/JCM.02408-16
Kaakoush, N. O., Deshpande, N. P., Man, S. M., Burgos-Portugal, J. A., Khattak, F. A., Raftery, M. J., et al. (2015). Transcriptomic and proteomic analyses reveal key innate immune signatures in the host response to the gastrointestinal pathogen Campylobacter concisus. Infect. Immun. 83, 832–845. doi:10.1128/IAI.03012-14
Karimi, L., Eskandari, N., Shaygannejad, V., Zare, N., Andalib, A., Khanahmad, H., et al. (2020). Comparison of expression levels of miR-29b-3p and miR-326 in T helper-1 and T helper-17 cells isolated from responsive and non-responsive relapsing-remitting multiple sclerosis patients treated with interferon-beta. Iran. J. Allergy, Asthma Immunol. 19, 416–425. doi:10.18502/ijaai.v19i4.4116
Kazemzadeh, M., Safaralizadeh, R., and Orang, A. V. (2015). LncRNAs: emerging players in gene regulation and disease pathogenesis. J. Genet. 94, 771–784. doi:10.1007/s12041-015-0561-6
Kibbie, J., Teles, R. M. B., Wang, Z., Hong, P., Montoya, D., Krutzik, S., et al. (2016). Jagged1 instructs macrophage differentiation in leprosy. PLOS Pathog. 12, e1005808. doi:10.1371/journal.ppat.1005808
Krutzik, S. R., Ochoa, M. T., Sieling, P. A., Uematsu, S., Ng, Y. W., Legaspi, A., et al. (2003). Activation and regulation of Toll-like receptors 2 and 1 in human leprosy. Nat. Med. 9, 525–532. doi:10.1038/nm864
Ku, H.-Y., and Lin, H. (2014). PIWI proteins and their interactors in piRNA biogenesis, germline development and gene expression. Natl. Sci. Rev. 1, 205–218. doi:10.1093/nsr/nwu014
Kumar, R., Halder, P., Sahu, S. K., Kumar, M., Kumari, M., Jana, K., et al. (2012). Identification of a novel role of ESAT-6-dependent miR-155 induction during infection of macrophages with Mycobacterium tuberculosis. Cell Microbiol. 14, 1620–1631. doi:10.1111/j.1462-5822.2012.01827.x
Kumar, S., Ali, R., Khanna, N., and Rao, D. (2011). Disruption of HLA-DR raft, deregulations of Lck–ZAP-70–Cbl-b cross-talk and miR181a towards T cell hyporesponsiveness in leprosy. Mol. Immunol. 48, 1178–1190. doi:10.1016/j.molimm.2011.02.012
Kumar, S., Naqvi, R. A., Ali, R., Rani, R., Khanna, N., and Rao, D. N. (2013). CD4+CD25+ T regs with acetylated FoxP3 are associated with immune suppression in human leprosy. Mol. Immunol. 56, 513–520. doi:10.1016/j.molimm.2013.04.015
Lee, R. C., Feinbaum, R. L., and Ambros, V. (1993). The C. elegans heterochronic gene lin-4 encodes small RNAs with antisense complementarity to lin-14. Cell 75, 843–854. doi:10.1016/0092-8674(93)90529-y
Li, Q.-J., Chau, J., Ebert, P. J. R., Sylvester, G., Min, H., Liu, G., et al. (2007). miR-181a is an intrinsic modulator of T cell sensitivity and selection. Cell 129, 147–161. doi:10.1016/j.cell.2007.03.008
Li, X., Yang, L., and Chen, L.-L. (2018a). The biogenesis, functions, and challenges of circular RNAs. Mol. Cell 71, 428–442. doi:10.1016/j.molcel.2018.06.034
Li, X., Yang, L., and Chen, L.-L. (2018b). The biogenesis, functions, and challenges of circular RNAs. Mol. Cell 71, 428–442. doi:10.1016/j.molcel.2018.06.034
Liu, P. T., Wheelwright, M., Teles, R., Komisopoulou, E., Edfeldt, K., Ferguson, B., et al. (2012a). MicroRNA-21 targets the vitamin D-dependent antimicrobial pathway in leprosy. Nat. Med. 18, 267–273. doi:10.1038/nm.2584
Liu, P. T., Wheelwright, M., Teles, R., Komisopoulou, E., Edfeldt, K., Ferguson, B., et al. (2012b). MicroRNA-21 targets the vitamin D-dependent antimicrobial pathway in leprosy. Nat. Med. 18, 267–273. doi:10.1038/nm.2584
López-Jiménez, E., and Andrés-León, E. (2021). The implications of ncRNAs in the development of human diseases. Noncoding RNA 7, 17. doi:10.3390/ncrna7010017
López-López, E., Gutiérrez-Camino, Á., Piñán, M. Á., Sánchez-Toledo, J., Uriz, J. J., Ballesteros, J., et al. (2014). Pharmacogenetics of MicroRNAs and MicroRNAs biogenesis machinery in pediatric acute lymphoblastic leukemia. PLoS ONE 9, e91261. doi:10.1371/journal.pone.0091261
Lukasik, A., and Zielenkiewicz, P. (2019). An overview of miRNA and miRNA target analysis tools. Methods Mol. Biol. 1932, 65–87. doi:10.1007/978-1-4939-9042-9_5
Masaki, T., Qu, J., Cholewa-Waclaw, J., Burr, K., Raaum, R., and Rambukkana, A. (2013). Reprogramming adult Schwann cells to stem cell-like cells by leprosy bacilli promotes dissemination of infection. Cell 152, 51–67. doi:10.1016/j.cell.2012.12.014
Mi, Z., Liu, H., and Zhang, F. (2020). Advances in the immunology and genetics of leprosy. Front. Immunol. 11, 567. doi:10.3389/fimmu.2020.00567
Misra, N., Murtaza, A., Walker, B., Narayan, N. P., Misra, R. S., Ramesh, V., et al. (1995). Cytokine profile of circulating T cells of leprosy patients reflects both indiscriminate and polarized T-helper subsets: T-helper phenotype is stable and uninfluenced by related antigens of Mycobacterium leprae. Immunology 86, 97–103.
Modlin, R. L. (1994). Th1-Th2 paradigm: insights from leprosy. J. Invest. Dermatol 102, 828–832. doi:10.1111/1523-1747.ep12381958
Moore, L. D., Le, T., and Fan, G. (2013). DNA methylation and its basic function. Neuropsychopharmacology 38, 23–38. doi:10.1038/npp.2012.112
Nath, I., Saini, C., and Valluri, V. L. (2015). Immunology of leprosy and diagnostic challenges. Clin. Dermatol 33, 90–98. doi:10.1016/j.clindermatol.2014.07.005
Niller, H. H., and Minarovits, J. (2016). Patho-epigenetics of infectious diseases caused by intracellular bacteria. Adv. Exp. Med. Biol. 879, 107–130. doi:10.1007/978-3-319-24738-0_6
Nunzi, E., and Massone, C. (2012). in Leprosy: a practical guide (Mailand, MA, USA: Springer-Verlag). doi:10.1007/978-88-470-2376-5
OConnell, R. M., Rao, D. S., and Baltimore, D. (2012). microRNA regulation of inflammatory responses. Annu. Rev. Immunol. 30, 295–312. doi:10.1146/annurev-immunol-020711-075013
Ojha, R., Nandani, R., Chatterjee, N., and Prajapati, V. K. (2018). “Emerging role of circular RNAs as potential biomarkers for the diagnosis of human diseases,” in Circular RNAs. Advances in experimental medicine and biology. Editor J. Xiao (Singapore: Springer), 141–157. doi:10.1007/978-981-13-1426-1_12
Ottenhoff, T. H. M. (2012). New pathways of protective and pathological host defense to mycobacteria. Trends Microbiol. 20, 419–428. doi:10.1016/j.tim.2012.06.002
Ozata, D. M., Gainetdinov, I., Zoch, A., O’Carroll, D., and Zamore, P. D. (2019). PIWI-interacting RNAs: small RNAs with big functions. Nat. Rev. Genet. 20, 89–108. doi:10.1038/s41576-018-0073-3
Paz, W. S. da, Souza, M., Tavares, D. dos S., Jesus, A. R. de, Santos, A. D. dos, do, C. R. F., et al. (2022). Impact of the COVID-19 pandemic on the diagnosis of leprosy in Brazil: an ecological and population-based study. Lancet Regional Health – Am., 9. doi:10.1016/j.lana.2021.100181
Petit, M., Mongelli, V., Frangeul, L., Blanc, H., Jiggins, F., and Saleh, M.-C. (2016). piRNA pathway is not required for antiviral defense in Drosophila melanogaster. Proc. Natl. Acad. Sci. U. S. A. 113, E4218–E4227. doi:10.1073/pnas.1607952113
Pinheiro, R. O., Schmitz, V., Silva, B. J. de A., Dias, A. A., de Souza, B. J., de Mattos Barbosa, M. G., et al. (2018). Innate immune responses in leprosy. Front. Immunol. 9, 518. doi:10.3389/fimmu.2018.00518
Pinto, P., da Silva, M. B., Moreira, F. C., Bouth, R. C., Gobbo, A. R., Sandoval, T. V., et al. (2020). Leprosy piRnome: exploring new possibilities for an old disease. Sci. Rep. 10, 12648. doi:10.1038/s41598-020-69355-7
Polycarpou, A., Walker, S. L., and Lockwood, D. N. (2013). New findings in the pathogenesis of leprosy and implications for the management of leprosy. Curr. Opin. Infect. Dis. 26, 413–419. doi:10.1097/QCO.0b013e3283638b04
Qian, X., Zhao, J., Yeung, P. Y., Zhang, Q. C., and Kwok, C. K. (2019). Revealing lncRNA structures and interactions by sequencing-based approaches. Trends Biochem. Sci. 44, 33–52. doi:10.1016/j.tibs.2018.09.012
Quinn, J. J., and Chang, H. Y. (2016). Unique features of long non-coding RNA biogenesis and function. Nat. Rev. Genet. 17, 47–62. doi:10.1038/nrg.2015.10
Ramchandran, R., and Chaluvally-Raghavan, P. (2017). “miRNA-mediated RNA activation in mammalian cells,” in RNA activation. Advances in experimental medicine and biology. Editor L.-C. Li (Singapore: Springer), 81–89. doi:10.1007/978-981-10-4310-9_6
Ridley, D. S., and Jopling, W. H. (1966). Classification of leprosy according to immunity. A five-group system. Int. J. Lepr. Other Mycobact. Dis. 34, 255–273.
Rossi, R. L., Rossetti, G., Wenandy, L., Curti, S., Ripamonti, A., Bonnal, R. J. P., et al. (2011). Distinct microRNA signatures in human lymphocyte subsets and enforcement of the naive state in CD4+ T cells by the microRNA miR-125b. Nat. Immunol. 12, 796–803. doi:10.1038/ni.2057
Rothchild, A. C., Sissons, J. R., Shafiani, S., Plaisier, C., Min, D., Mai, D., et al. (2016). MiR-155-regulated molecular network orchestrates cell fate in the innate and adaptive immune response to Mycobacterium tuberculosis. Proc. Natl. Acad. Sci. U. S. A. 113, E6172–E6181. doi:10.1073/pnas.1608255113
Saini, C., Siddiqui, A., Ramesh, V., and Nath, I. (2016). Leprosy reactions show increased Th17 cell activity and reduced FOXP3+ tregs with concomitant decrease in TGF-β and increase in IL-6. PLoS Negl. Trop. Dis. 10, e0004592. doi:10.1371/journal.pntd.0004592
Salgado, C. G., Pinto, P., Bouth, R. C., Gobbo, A. R., Messias, A. C. C., Sandoval, T. V., et al. (2018). miRNome expression analysis reveals new players on leprosy immune physiopathology. Front. Immunol. 9, 463. doi:10.3389/fimmu.2018.00463
Sallam, T, Jaspreet, S., and Peter, T. (2018). Long noncoding RNA discovery in cardiovascular disease: decoding form to function. Circulation Res. 122, 155–166. doi:10.1161/CIRCRESAHA.117.311802
Schulte, L. N., Bertrams, W., Stielow, C., and Schmeck, B. (2019). ncRNAs in inflammatory and infectious diseases. Methods Mol. Biol. 1912, 3–32. doi:10.1007/978-1-4939-8982-9_1
Singh, P. K., Singh, A. V., and Chauhan, D. S. (2013b). Current understanding on micro RNAs and its regulation in response to Mycobacterial infections. J. Biomed. Sci. 20, 14. doi:10.1186/1423-0127-20-14
Singh, R. P., Massachi, I., Manickavel, S., Singh, S., Rao, N. P., Hasan, S., et al. (2013a). The role of miRNA in inflammation and autoimmunity. Autoimmun. Rev. 12, 1160–1165. doi:10.1016/j.autrev.2013.07.003
Siomi, M. C., Sato, K., Pezic, D., and Aravin, A. A. (2011). PIWI-interacting small RNAs: the vanguard of genome defence. Nat. Rev. Mol. Cell Biol. 12, 246–258. doi:10.1038/nrm3089
Soares, C. T., Trombone, A. P. F., Fachin, L. R. V., Rosa, P. S., Ghidella, C. C., Ramalho, R. F., et al. (2017). Differential expression of MicroRNAs in leprosy skin lesions. Front. Immunol. 8, 1035. doi:10.3389/fimmu.2017.01035
Stellato, C., Fang, X., Abdelmohsen, K., Gorospe, M., and Ishmael, F. T. (2011). Glucocorticoid (GC) modulation of global miRNA profile in human airway epithelial cells. J. Allergy Clin. Immunol. 127, AB64. doi:10.1016/j.jaci.2010.12.267
Stillman, B. (2018). Histone modifications: insights into their influence on gene expression. Cell 175, 6–9. doi:10.1016/j.cell.2018.08.032
Story, B., Ma, X., Ishihara, K., Li, H., Hall, K., Peak, A., et al. (2019). Defining the expression of piRNA and transposable elements in Drosophila ovarian germline stem cells and somatic support cells. Life Sci. Alliance 2 (5), e201800211. doi:10.26508/lsa.201800211
Stratton, J. A., Holmes, A., Rosin, N. L., Sinha, S., Vohra, M., Burma, N. E., et al. (2018). Macrophages regulate Schwann cell maturation after nerve injury. Cell Rep. 24, 2561–2572. doi:10.1016/j.celrep.2018.08.004
Syn, G., Blackwell, J. M., and Jamieson, S. E. (2016). “Epigenetics in infectious diseases,” in Epigenetic biomarkers and diagnostics (Amsterdam, Netherlands: Elsevier), 377–400. doi:10.1016/B978-0-12-801899-6.00019-X
Tapinos, N., Ohnishi, M., and Rambukkana, A. (2006). ErbB2 receptor tyrosine kinase signaling mediates early demyelination induced by leprosy bacilli. Nat. Med. 12, 961–966. doi:10.1038/nm1433
Tió-Coma, M., Kiełbasa, S. M., van den Eeden, S. J. F., Mei, H., Roy, J. C., Wallinga, J., et al. (2021). Blood RNA signature RISK4LEP predicts leprosy years before clinical onset. EBioMedicine 68, 103379. doi:10.1016/j.ebiom.2021.103379
Vagin, V. V., Sigova, A., Li, C., Seitz, H., Gvozdev, V., and Zamore, P. D. (2006). A distinct small RNA pathway silences selfish genetic elements in the germline. Science 313, 320–324. doi:10.1126/science.1129333
Wang, Z., Sun, Y., Fu, X., Yu, G., Wang, C., Bao, F., et al. (2016). A large-scale genome-wide association and meta-analysis identified four novel susceptibility loci for leprosy. Nat. Commun. 7, 13760. doi:10.1038/ncomms13760
Westermann, A. J., Förstner, K. U., Amman, F., Barquist, L., Chao, Y., Schulte, L. N., et al. (2016). Dual RNA-seq unveils noncoding RNA functions in host–pathogen interactions. Nature 529, 496–501. doi:10.1038/nature16547
Who, (2019). WHO. Classification of leprosy. https://www.who.int/lep/classification/en/.
Who, (2021a). WHO Global leprosy strategy 2021–2030. https://ilepfederation.org/global-leprosy-%e2%80%8ehansens-disease%e2%80%8e-strategy-2021-2030-towards-zero-leprosy/ (Accessed June 14, 2022).
Who, (2021b). Global leprosy (Hansen disease) update. https://www.who.int/publications/i/item/who-wer9736-429-450.
Xu, M., Zuo, D., Liu, X., Fan, H., Chen, Q., Deng, S., et al. (2017). MiR-155 contributes to Th17 cells differentiation in dextran sulfate sodium (DSS)-induced colitis mice via Jarid2. Biochem. Biophysical Res. Commun. 488, 6–14. doi:10.1016/j.bbrc.2017.04.143
Yan, W., Chen, Z.-Y., Chen, J.-Q., and Chen, H.-M. (2018). LncRNA NEAT1 promotes autophagy in MPTP-induced Parkinson’s disease through stabilizing PINK1 protein. Biochem. Biophysical Res. Commun. 496, 1019–1024. doi:10.1016/j.bbrc.2017.12.149
Yang, R., Huang, F., Fu, J., Dou, B., Xu, B., Miao, L., et al. (2016). Differential transcription profiles of long non-coding RNAs in primary human brain microvascular endothelial cells in response to meningitic Escherichia coli. Sci. Rep. 6, 38903. doi:10.1038/srep38903
Yang, T., and Ge, B. (2018). miRNAs in immune responses to Mycobacterium tuberculosis infection. Cancer Lett. 431, 22–30. doi:10.1016/j.canlet.2018.05.028
Yao, R.-W., Wang, Y., and Chen, L.-L. (2019). Cellular functions of long noncoding RNAs. Nat. Cell Biol. 21, 542–551. doi:10.1038/s41556-019-0311-8
Yeh, J.-H., Sidhu, S. S., and Chan, A. C. (2008). Regulation of a late phase of T cell polarity and effector functions by crtam. Cell 132, 846–859. doi:10.1016/j.cell.2008.01.013
Yi, Z., Li, J., Gao, K., and Fu, Y. (2014). Identifcation of differentially expressed long non-coding RNAs in CD4+ T cells response to latent tuberculosis infection. J. Infect. 69, 558–568. doi:10.1016/j.jinf.2014.06.016
Zhang, Q., and Cao, X. (2019). Epigenetic regulation of the innate immune response to infection. Nat. Rev. Immunol. 19, 417–432. doi:10.1038/s41577-019-0151-6
Zhang, T., Hu, H., Yan, G., Wu, T., Liu, S., Chen, W., et al. (2019a). Long non-coding RNA and breast cancer. Technol. Cancer Res. Treat. 18, 1533033819843889. doi:10.1177/1533033819843889
Zhang, Y., Xu, W., Nan, S., Chang, M., and Fan, J. (2019b). MicroRNA-326 inhibits apoptosis and promotes proliferation of dopaminergic neurons in Parkinson’s disease through suppression of KLK7-mediated MAPK signaling pathway. J. Mol. Neurosci. 69, 197–214. doi:10.1007/s12031-019-01349-1
Zhou, M., Liu, Z., Zhao, Y., Ding, Y., Liu, H., Xi, Y., et al. (2010). MicroRNA-125b confers the resistance of breast cancer cells to paclitaxel through suppression of pro-apoptotic Bcl-2 antagonist killer 1 (Bak1) expression. J. Biol. Chem. 285, 21496–21507. doi:10.1074/jbc.M109.083337
Zhou, W.-Y., Cai, Z.-R., Liu, J., Wang, D.-S., Ju, H.-Q., and Xu, R.-H. (2020). Circular RNA: metabolism, functions and interactions with proteins. Mol. Cancer 19, 172. doi:10.1186/s12943-020-01286-3
Keywords: leprosy, ncRNAs, miRNAs, Mycobacterium leprae, infection, immunity
Citation: Santana-da-Silva MN, Sena-dos-Santos C, Cáceres-Durán MÁ, Souza FGd, Gobbo AR, Pinto P, Salgado CG and dos Santos SEB (2023) ncRNAs: an unexplored cellular defense mechanism in leprosy. Front. Genet. 14:1295586. doi: 10.3389/fgene.2023.1295586
Received: 16 September 2023; Accepted: 24 November 2023;
Published: 04 December 2023.
Edited by:
Naoko Hattori, Hoshi University, JapanReviewed by:
Deepak Parashar, Medical College of Wisconsin, United StatesDaniela Ferreira, University of Trás-os-Montes and Alto Douro, Portugal
Copyright © 2023 Santana-da-Silva, Sena-dos-Santos, Cáceres-Durán, Souza, Gobbo, Pinto, Salgado and dos Santos. This is an open-access article distributed under the terms of the Creative Commons Attribution License (CC BY). The use, distribution or reproduction in other forums is permitted, provided the original author(s) and the copyright owner(s) are credited and that the original publication in this journal is cited, in accordance with accepted academic practice. No use, distribution or reproduction is permitted which does not comply with these terms.
*Correspondence: Mayara Natália Santana-da-Silva, bWF5YXJhc2lsdmFAaWVjLmdvdi5icg==