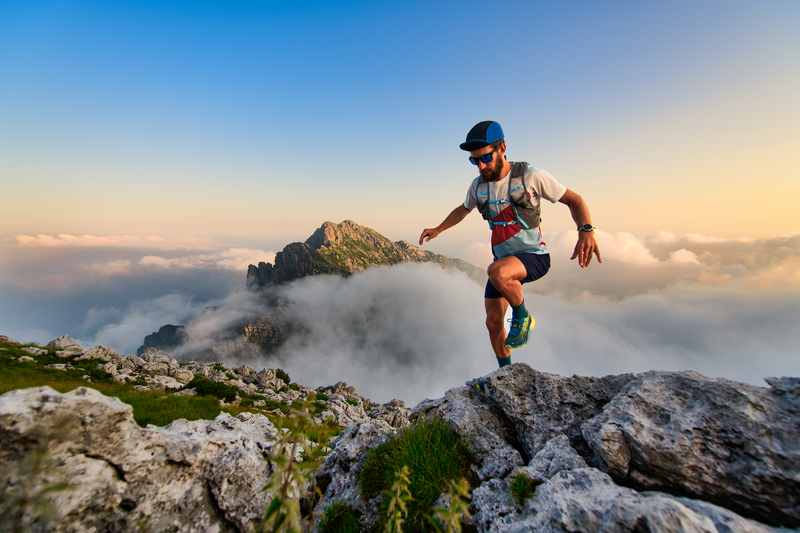
95% of researchers rate our articles as excellent or good
Learn more about the work of our research integrity team to safeguard the quality of each article we publish.
Find out more
REVIEW article
Front. Genet. , 24 August 2023
Sec. Pharmacogenetics and Pharmacogenomics
Volume 14 - 2023 | https://doi.org/10.3389/fgene.2023.1242711
This article is part of the Research Topic Exploring the Impact of Genetics on New Drugs and Potential Drug Targets: A Multi-Omics Approach to Improve Personalized Therapeutics. View all 10 articles
Voriconazole (VRZ) is a broad-spectrum antifungal medication widely used to treat invasive fungal infections (IFI). The administration dosage and blood concentration of VRZ are influenced by various factors, posing challenges for standardization and individualization of dose adjustments. On the one hand, VRZ is primarily metabolized by the liver, predominantly mediated by the cytochrome P450 (CYP) 2C19 enzyme. The genetic polymorphism of CYP2C19 significantly impacts the blood concentration of VRZ, particularly the trough concentration (Ctrough), thereby influencing the drug’s efficacy and potentially causing adverse drug reactions (ADRs). Recent research has demonstrated that pharmacogenomics-based VRZ dose adjustments offer more accurate and individualized treatment strategies for individuals with hepatic insufficiency, with the possibility to enhance therapeutic outcomes and reduce ADRs. On the other hand, the security, pharmacokinetics, and dosing of VRZ in individuals with hepatic insufficiency remain unclear, making it challenging to attain optimal Ctrough in individuals with both hepatic insufficiency and IFI, resulting in suboptimal drug efficacy and severe ADRs. Therefore, when using VRZ to treat IFI, drug dosage adjustment based on individuals’ genotypes and hepatic function is necessary. This review summarizes the research progress on the impact of genetic polymorphisms and hepatic insufficiency on VRZ dosage in IFI individuals, compares current international guidelines, elucidates the current application status of VRZ in individuals with hepatic insufficiency, and discusses the influence of CYP2C19, CYP3A4, CYP2C9, and ABCB1 genetic polymorphisms on VRZ dose adjustments and Ctrough at the pharmacogenomic level. Additionally, a comprehensive summary and analysis of existing studies’ recommendations on VRZ dose adjustments based on CYP2C19 genetic polymorphisms and hepatic insufficiency are provided, offering a more comprehensive reference for dose selection and adjustments of VRZ in this patient population.
Invasive fungal infection (IFI) is a dangerous disease commonly seen in individuals with damaged immune function, such as individuals with acquired immune deficiency syndrome (AIDS), malignancy, and organ transplantation (Kullberg and Arendrup, 2015; Douglas et al., 2021). Individuals with hepatic insufficiency are vulnerable to IFI due to their low immune function and increased intestinal mucosal permeability, and IFI has a high mortality rate, which seriously affects patient prognosis, especially in immunosuppressed individuals, where the mortality rate may reach up to 90% (Yamada et al., 2018; Chen et al., 2021; Jenks et al., 2021). VRZ is a medication with broad-ranging antifungal properties extensively used to treat IFI (Ullmann et al., 2007). However, the pharmacokinetic parameters, efficacy, and safety of VRZ are influenced by various factors, such as genetic polymorphisms, liver function, and drug interactions (Liu and Mould, 2014; Lamoureux et al., 2016).
Gene polymorphism is one of the critical factors in the variability of pharmacokinetic parameters of VRZ. Gene polymorphism is when multiple versions of genes are present in a population, and these versions can result in different enzyme activities (Cheng et al., 2018). VRZ is primarily metabolized in the liver by CYP2C19 enzyme and partially by CYP3C4 and CYP2C9. CYP2C19 gene polymorphism affects the pharmacokinetic parameters and efficacy of VRZ (Hulin et al., 2011; Hamadeh et al., 2017). It has been found that the pharmacokinetic parameters of VRZ in mutant carriers such as CYP2C19*2 are significantly higher than those in wild-type carriers, while enhanced carriers such as CYP2C19*17 show the opposite trend (Pascual et al., 2008; Dolton et al., 2012). Therefore, individualized dose adjustment strategies are needed for different CYP2C19 genotypes to improve the efficacy and safety of VRZ (Pascual et al., 2012).
Hepatic insufficiency can also affect the pharmacokinetic parameters and efficacy of VRZ. Individuals with hepatic insufficiency experience difficulty breaking down and eliminating drugs from their system, and this causes the drug to remain in the body for an extended duration, leading to higher concentrations of the drug (Liu and Mould, 2014). Therefore, individuals with hepatic insufficiency should decrease their VRZ dosage to prevent ADRs caused by a potential overdose (Lamoureux et al., 2016). According to a study, the way VRZ works and its effects differ significantly for individuals with hepatic insufficiency compared to those with normal liver function (Tang et al., 2019; 2021). In addition, VRZ has a small margin of safety and has multiple ADRs, including neurotoxicity, hepatotoxicity, and visual impairment (Levine and Chandrasekar, 2016; Lem et al., 2019). Research has confirmed a meaningful connection between Ctrough and both the effectiveness of the treatment and the adverse drug reactions (Yang et al., 2022). As a result, medical professionals frequently suggest therapeutic drug monitoring (TDM) to enhance patient outcomes (Jin et al., 2016; Luong et al., 2016). The instructions provide dosage adjustment recommendations for individuals with mild to moderate liver impairment, utilizing exposure data from real-world usage of VRZ. However, there is a lack of comprehensive data on the safety, pharmacokinetics, and appropriate dosage for patients with severe hepatic insufficiency. It is crucial to develop individualized dose adjustment strategies for these patients to optimize the efficacy and safety of VRZ treatment.
Based on the above studies, the treatment of IFI involves a significant role for VRZ, but its pharmacokinetic parameters, efficacy and safety are influenced by several factors. Individualized dose adjustment strategies can enhance the effectiveness and safety of VRZ, but there are some differences in the results of different studies. Therefore, when developing dose adjustment strategies, the distribution of genetic polymorphisms and hepatic insufficiency in diverse populations should be considered, and the effects of multiple factors should be taken into account. However, there is not enough large-scale clinical research on personalized dosing for VRZ to confirm whether it is a safe and effective approach for guiding clinical practice. This paper focuses on how the CYP2C19 gene and hepatic insufficiency affect VRZ dose adjustment. From a pharmacogenomic perspective, we further investigate the influence of genetic polymorphisms in CYP3A4, CYP2C9, and ABCB1 on Ctrough. This information can help develop personalized treatment plans for VRZ use in individuals with IFI.
Voriconazole (VRZ) was approved for marketing by the U.S. Food and Drug Administration (FDA) in 2002 and was introduced in China in 2005 (Pallet and Loriot, 2021). VRZ is a synthetic second-generation triazole antifungal drug derived from fluconazole and exhibits broad-spectrum antifungal activity. It is believed that the mechanism of action for triazole antifungal drugs involves inhibiting the fungal enzyme 14α-demethylase, which is responsible for converting lanosterol to ergosterol, thereby disrupting the synthesis of the cell membrane (Thompson and Lewis, 2010). The Infectious Diseases Society of America recommends VRZ as the primary treatment for invasive Aspergillosis (Chen et al., 2018). It is also effective against Candida spp. in treatment and prevention (Xing et al., 2017). The metabolic pathways of VRZ are influenced by multiple enzymes, with its primary circulating metabolite being Voriconazole N-oxide (Theuretzbacher et al., 2006; Voriconazole Pathway, Pharmacokinetics, n.d.) (Figure 1).
FIGURE 1. Metabolic pathways of VRZ. VRZ is primarily metabolized by the enzyme CYP2C19 to form Voriconazole N-oxide, with contributions from members of the CYP3A4, CYP2C9, and FMO families. Subsequently, Voriconazole N-oxide is further metabolized to voriconazole O-glucuronidated derivative and other oxidized metabolites. The second metabolic pathway involves hydroxylation of the methyl group of VRZ, in which VRZ is metabolized by CYP3A4 to 4-Hydroxyvoriconazole, which is then glucuronidated to form 4-Hydroxyvoriconazole 4-glucuronidate. The third pathway involves hydroxylation of the fluconazole ring of VRZ, in which VRZ is metabolized by CYP2C19 to hydroxyvoriconazole, which can further undergo hydroxylation by CYP2C19 to form dihydroxyvoriconazole. Finally, dihydroxyvoriconazole is glucuronidated to form dihydroxyvoriconazole O-glucuronidated. Voriconazole Pathway, Pharmacokinetics (Reproduced from PharmaGKB, licensed under CC BY-SA 4.0).
VRZ is used to treat invasive Aspergillosis, non-neutropenic Candidaemia individuals, and severe invasive infections caused by fluconazole-resistant Candida; however, VRZ may cause side effects such as hepatotoxicity, neurotoxicity, photosensitivity, visual disturbances, and osteochondritis with or without hyperfluorosis (Chau et al., 2014). Psychiatric disorders are common ADRs caused by VRZ, characterized by symptoms such as delirium, hallucinations, and emotional excitement (Benitez and Carver, 2019). A study suggested that these symptoms are associated with the distribution and blood concentration of VRZ in the body; during its distribution in the body, VRZ can penetrate the blood-cerebrospinal fluid barrier, resulting in brain tissue concentrations that are 2–3 times higher than plasma concentrations (Ertem et al., 2022). ADRs associated with Ctrough also include hepatotoxicity and other neurological disorders (Hamada et al., 2012; Moriyama et al., 2017). Any form of VRZ can be excreted as a metabolite (98%) and as a prototype (2%) within 48 h of administration, so although it is not uncommon for VRZ to cause psychiatric disorders, psychiatric symptoms can rapidly improve or even disappear in a brief amount of time after discontinuation of the drug (Yi et al., 2017). In individuals with hepatic insufficiency combined with invasive aspergillosis, VRZ remains the drug of choice, but there is still much controversy regarding dose selection (Chen and Ning, 2022).
Reports suggest that VRZ can cause liver injury, but the mechanism of its occurrence is still unclear, and some studies suggest that it is mainly related to VRZ metabolism (Kyriakidis et al., 2017; Zhou et al., 2022). VRZ can be converted to active metabolites in the liver, and such functional products can cause mitochondrial damage directly or through inhibition of CYP proteins, leading to cellular dysfunction or necrosis, thus causing liver injury (Pessayre et al., 2012). There is controversy surrounding the impact of VRZ on liver injury, with some studies suggesting that VRZ-induced liver injury is a dose-dependent ADR, but others suggesting that liver injury does not correlate with drug concentration (Suzuki et al., 2013; Zonios et al., 2014).
The pharmacokinetic profile of VRZ in adults is nonlinear, with a significant increase in Ctrough with increasing medication administration. It is predominantly metabolized oxidatively by cytochrome P450 isoenzymes and secondarily by CYP3A4 and CYP2C9 enzymes, in addition to being a CYP3A4 inhibitor itself, so VRZ has more clinically significant drug interactions (Lee et al., 2022). Some studies have reported that the interaction of VRZ with carbamazepine, efavirenz, ritonavir, rifampin, phenobarbital, rifabutin, and nevirapine affects their pharmacokinetic parameters and efficacy (Chen et al., 2018). Therefore, attention needs to be paid to VRZ interactions with other drugs during treatment. VRZ pharmacokinetics are variable within and between individuals, with influencing factors including age, CYP2C19 gene polymorphisms, hepatic function status, drug interactions, and ingestion, further adding to the concern of clinical response variability (Job et al., 2016; Li et al., 2017; You et al., 2018). In addition, Ctrough can significantly impact clinical response, and routine TDM of VRZ is recommended because of inter-patient variability in VRZ pharmacokinetics to avoid high Ctrough-related toxicity and treatment failure with low Ctrough (Denning et al., 2002).
Hepatic insufficiency refers to the damage of liver cells by various hepatogenic factors, resulting in dysfunction of synthesis, degradation, detoxification, storage, secretion, and immunity, which may lead to jaundice, hemorrhage, infection, renal dysfunction, and hepatic encephalopathy (Verma et al., 2022). The advanced stage of hepatic insufficiency is generally called hepatic failure, and the main clinical manifestations are hepatic encephalopathy and hepatorenal syndrome (Rose et al., 2020; Vasques et al., 2022). Individuals with hepatic insufficiency are prone to complications of various infections due to impaired immune function, dysbiosis of intestinal flora, and reduced number of hepatic Kupffer cells, resulting in low immunity (Yamada et al., 2018). Moreover, the application of broad-spectrum, potent antimicrobial drugs and glucocorticoids, as well as invasive procedures, greatly raises the risk of opportunistic infections, especially in individuals with IFI, which are mostly single-site, but there are also cases of two or even multi-site disseminated infections (Hou et al., 2010; Lahmer et al., 2022). In critically ill individuals with hepatic insufficiency and IFI, the most frequent site of infection is the lung (37.0%–56.0%); other sites are the gastrointestinal tract (1.1%–20.2%), urinary tract (4.3%–15.9%), abdominal cavity (2.9%–14.4%) and bloodstream (0.7%–5.8%), and fungal infections of the thoracic cavity, biliary tract, and central nervous system are also seen (Fernández et al., 2018; Piano et al., 2019; Libera et al., 2023). When the liver is not functioning properly, it can affect the metabolism and excretion of VRZ; this may impact the drug’s absorption and clearance rates from the body (Baririan et al., 2007; Verbeeck, 2008). Their Ctrough levels are significantly higher when they have hepatic insufficiency, which raises the risk of ADRs and can have a detrimental effect on their prognosis (Alffenaar et al., 2009; Ueda et al., 2009).
The most common pathogenic fungi of hepatic insufficiency combined with IFI are Candida spp. and Aspergillus spp. Candida spp. are mainly Candida albicans, accounting for more than 50%, and are the main pathogens of the intestinal tract, bloodstream, abdominal cavity, and urinary tract (Jenks et al., 2020; Silva et al., 2021; Hall et al., 2023). A multicenter study in Europe showed that fungal bloodstream infections were dominated by Candida albicans (54.4%), followed by Candida smooth (14.5%), Candida subsmooth (14.1%), Candida tropicalis (5.8%), Candida graminearum (2.5%), of which 34.9% of individuals suffered from septic shock (Bassetti et al., 2017; Medeiros et al., 2019). Candida albicans was also the main causative agent of fungal peritonitis (48.0%–81.8%), followed by Candida klebsiella (15.0%–25.0%), Candida smoothes (6.6%–20.0%), and novel Cryptococcus spp. were seen in some individuals (Tariq et al., 2019; Feldman et al., 2023). Pulmonary IFI is mainly caused by Aspergillus spp., with Aspergillus fumigatus being the most common, followed by Aspergillus flavus and Aspergillus niger; Aspergillus pyogenes and Aspergillus terreus are less frequently reported (Su et al., 2010; Jović et al., 2019; Lahmer et al., 2019). Cases of severe hepatic insufficiency combined with Pneumocystis pneumonia have also been reported to occur (Hadfield et al., 2019). In severe hepatic insufficiency, both natural and acquired immunity are severely impaired, resulting in decreased immunity, and often accompanied by intestinal dysfunction, intestinal mucosal edema, increased permeability, impaired intestinal barrier leading to flora translocation, intestinal microorganisms can enter the portal vein through the intestinal wall, coupled with serious damage to the liver mononuclear macrophage system, the ability to remove microorganisms is reduced, resulting in infection with bacteria, viruses and fungi and other pathogens the risk of infection with pathogens such as bacteria, viruses and fungi is significantly increased (Matsubara et al., 2016; Andrade et al., 2022). Therefore, individuals with hepatic insufficiency are more susceptible to developing IFI, and IFI usually occurs in the blood circulation and eventually causes systemic fungal infections, leading to conditions such as organ failure, sepsis, and fatal multi-organ dysfunction syndrome, for which VRZ is the first-line drug (Xing et al., 2017).
Severe hepatic insufficiency combined with IFI has an inadequate prognosis, with an upper morbidity and death rate, the clinical manifestations can be atypical, and diagnosing and treating it can be difficult. Antifungal drugs are mostly metabolized in the liver, which can cause highly toxic side effects (Cheong et al., 2009). According to relevant literature, individuals infected with Candida have a 30%–40% morbidity and mortality rate, while individuals infected with Aspergillus have an even higher rate of 50%–100% (Lahmer et al., 2016). Candida infection, invasive Aspergillus infection individuals to increase morbidity and mortality rate (Hwang et al., 2014). During liver transplantation, individuals who receive a new liver have an increased chance of getting fungal infections during and after the procedure (Kang et al., 2020). In recent years, prophylactic use of antifungal medications has helped to bring the overall prevalence of these infections down to 4%–8% (Lum et al., 2020; Khalid et al., 2021).
VRZ is mainly metabolized in the liver and mediated by cytochrome P450 (CYP) 2C19 enzyme (Theuretzbacher et al., 2006). The CYP2C19 gene has genetic polymorphisms that can affect the pharmacokinetic characteristics of VRZ; in fact, these polymorphisms are responsible for 50% of the variability in VRZ. (Amsden and Gubbins, 2017). The gene that codes for CYP2C19 has more than 34 different versions, known as alleles; one of these alleles, called CYP2C19*17, has a mutation in the gene’s promoter region, making it more active than usual (Lee et al., 2022). It was found that mutant genes such as CYP2C19*2 and CYP2C19*3 were connected to pharmacokinetic parameters of VRZ, and individuals carrying mutant genes such as CYP2C19*2 and CYP2C19*3 had a slower clearance of VRZ, higher drug exposure, and greater fluctuations in drug concentration at the same dose (Hamadeh et al., 2017). It has been found that individualized adjustment of VRZ dose according to individuals’ CYP2C19 genotypes can reduce drug exposure and decrease the incidence of ADRs while ensuring drug efficacy (Amsden and Gubbins, 2017; Zhang et al., 2021). Individualized dose adjustment of VRZ may be necessary for different CYP2C19 genotypes. The proper dosage can be determined using TDM in conjunction with pharmacogenetic testing (He et al., 2020; Li et al., 2021).
The Clinical Pharmacogenetics Implementation Consortium (CPIC) categorizes individuals into five groups based on their genotype for CYP2C19 (Moriyama et al., 2017). These groups include CYP2C19 ultrarapid metabolizers (UMs), CYP2C19 rapid metabolizers (RMs), CYP2C19 normal metabolizers (NMs), CYP2C19 intermediate metabolizers (IMs), and CYP2C19 poor metabolizers (PMs) (Lee et al., 2022) (See Table 1: CYP2C19 phenotype classification). The differences in CYP2C19 genes between individuals can greatly affect how they respond to VRZ medication, particularly for those with liver problems. Studies have shown that identifying a patient’s CYP2C19 gene phenotype is essential in determining the appropriate VRZ dosage, as it can vary greatly from UMs to PMs (Ren et al., 2019). The impact of CYP2C19 is noted in the VRZ medication label approved by the FDA. However, there are currently no genetic variant-based dosing instructions available. To avoid potential problems, CPIC suggests utilizing antifungal medications that do not rely on the CYP2C19 enzyme metabolism for individuals with PMs/Ums, while standard VRZ dosing is recommended for other phenotypes, and the Dutch Pharmacogenetics Working Group (DPWG) recommends dose adjustment for both PMs and UMs phenotypes (García-García and Borobia, 2021; Maertens et al., 2021). The inconsistency and ambiguity of these guidelines may hinder clinicians’ practical application of these drugs.
The CYP2C19*1/*17 and *17/*17 genotypes conferred higher enzymatic activity to the RMs and UMs phenotypes, respectively, compared to NMs (Sim et al., 2006). The *2 and *3 alleles were loss-of-function variations. IMs with one such variant had significantly lower enzyme activity compared to NMs. However, PMs with two such variants showed no enzyme activity. According to Hamadeh et al. (2017), CYP2C19 genotype significantly impacts the risk of VRZ underexposure, individuals with *17/*17 genotypes (UMs) and around 50% of those with *1/*17 genotypes (RMs) were unable to achieve a therapeutic Ctrough (2–6 mg/L) when VRZ was administered based on body weight. UMs showed a decrease in VRZ Ctrough, resulting in a delay in reaching the target Ctrough; on the other hand, PMs exhibited an increase in Ctrough, which puts them at a higher risk of ADRs (Walsh et al., 2018). Hence, compared to NMs, UMs and RMs may require an increase in the VRZ dosage, while PMs may necessitate a reduction in the VRZ dosage (Lamoureux et al., 2016) (See Table. 2: Recommendations for dose adjustment for different CYP2C19 phenotypes). It is important to be aware that medications like omeprazole and cimetidine, which inhibit CYP2C19, can increase Ctrough levels in VRZ; conversely, taking certain CYP450 enzyme inducers at the same time can cause Ctrough levels to drop below the necessary therapeutic levels, resulting in clinical failure (Mikus et al., 2006). Existing meta-analyses indicate that PMs taking VRZ are at an upper risk of experiencing ADRs compared to NMs and IMs; however, other meta-analyses have not found a significant correlation between the two (Li et al., 2016; Amsden and Gubbins, 2017). Therefore, we still require extensive, high-quality trials to confirm these findings.
Even though many studies have shown how the CYP2C19 gene polymorphisms affect VRZ dosage adjustment, specific details and controversies still exist. PMs/IMs lead to elevated VRZ blood levels that may result in toxicity, such as hepatotoxicity or neurotoxicity, but the link between PMs/IMs and hepatotoxicity has not been established (Wang et al., 2014b). Additional investigation is necessary to fully comprehend the effects of CYP2C19*2 and CYP2C19*3 mutations on VRZ therapy response and hepatotoxicity. Differences in CYP2C19 genotype distribution in different populations may affect the applicability of dose adjustment strategies. For example, some studies have found a higher frequency of mutant phenotypes such as CYP2C19*2 in Asian populations, while enhanced phenotypes such as CYP2C19*17 predominate in European and American people, which may affect the accuracy and effectiveness of dose adjustment strategies (Mikus et al., 2011; Lee et al., 2021). Various studies indicate that the impact of CYP2C19 gene variations on the efficiency and security of VRZ may depend on the particular approach used for adjusting the dosage. For example, it has been suggested that individualized dose adjustment strategies may improve the efficacy and safety of VRZ more than conventional dose adjustment strategies in CYP2C19*2 and other mutant carriers (Hamada et al., 2013). Although CYP2C19 gene polymorphisms have an impact on the pharmacokinetic parameters and efficacy of VRZ, other causes, including individuals’ liver and kidney function and drug interactions, need to be considered in actual clinical application. Special attention should be given to the impact of drug-induced enzyme reactions on the alteration of related drug plasma concentrations, particularly when co-administered with hepatic enzyme inducers or inhibitors, to avoid ADRs (Hakkola et al., 2020) (See Table 3: Inhibitors and inducers of CYP2C19, CYP3A4, and CYP2C9). Therefore, the dose adjustment strategy should consider various factors rather than being based solely on CYP2C19 genotype (Moriyama et al., 2017).
CYP3A is the most prevalent metabolic enzyme in the liver and is engaged in the metabolism of 45%–60% of frequently used medications; CYP3A4 and CYP3A5 are the most significant drug-metabolizing enzymes in this regard (Wojnowski, 2004). CYP3A5 accounts for approximately 17%–60% of hepatic CYP3A and has a similar substrate specificity to CYP3A4; however, even with the same substrate, CYP3A4 exerts a higher metabolic efficiency (Klyushova et al., 2022). CYP3A4 is the main metabolic enzyme for VRZ hydroxylation metabolism, while CYP3A5 plays a relatively weak role in VRZ hydroxylation metabolism, and studies have shown that the hydroxylation metabolism of VRZ by CYP3A4 and CYP3A5 is relatively enhanced when CYP2C19 enzyme activity is diminished (Murayama et al., 2007). The CYP3A4 gene is a key enzyme in VRZ metabolism, and its genotype is associated with the pharmacokinetic and pharmacodynamic properties of VRZ. Still, compared with CYP2C19, CYP3A4 affects VRZ metabolism in vivo to a lesser extent, approximately 1/50th of CYP2C19(Hyland et al., 2003). Although CYP3A4 has been addressed in several previous studies, no genotypes explained the phenotype until two SNPs, rs4646437 and rs35599367, were found to be associated with the Ctrough of VRZ. The research findings reveal that the rs4646437 polymorphism significantly influences the mean blood drug concentration of VRZ, with the T variant allele being associated with higher blood drug concentrations (Gautier-Veyret et al., 2015; He et al., 2015). Walsh et al. found that polymorphisms such as CYP3A4*22 and CYP3A4*23 may impact the metabolism of VRZ, and CYP3A4 *22 was associated with higher VRZ concentrations compared to CYP3A4 *1/*1 (Walsh et al., 2018). Meanwhile, some studies indicate that genetic variations of CYP3A4 and CYP3A5 have little impact on the pharmacokinetics of VRZ (Lee et al., 2012; Chuwongwattana et al., 2020). Diverse studies’ findings on how CYP3A4 genotype affects VRZ are equivocal; more research is required to determine how the two are related. Research on how CYP3A5 affects the pharmacokinetics of VRZ has also produced inconsistent results. According to Weiss et al., there is no apparent connection between CYP3A5*3 mutations and VRZ pharmacokinetics (Weiss et al., 2009). According to Levin et al. (2007), the amount of hepatic drug-metabolizing enzymes in the blood may indicate high levels of VRZ plasma concentration-induced liver toxicity; the study also discovered that the liver damage was not related to the CYP3A5*3 allele. However, a study conducted in a laboratory setting has demonstrated that individuals with the genetic variant CYP3A5*3/*3 experience a threefold increase in AUC when taking VRZ compared to those with at least one functional allele (Yamazaki et al., 2010).
Studies have revealed that CYP2C19 is the primary enzyme responsible for the nitrogen-based oxidative metabolism of VRZ; however, CYP2C9 can also contribute to this process to a lesser extent (Dorji et al., 2019). Lee et al. (2002) showed that there is a link between the variability of VRZ blood concentration and the CYP2C9*2 and CYP2C9*3 alleles. The CYP2C9*13 allele is the first novel variant of CYP2C9 identified in Chinese and is important in determining the metabolic capacity of CYP2C9. Some studies indicate that the CYP2C9*13 gene variation can decrease drug clearance from the bloodstream (Si et al., 2004; Zhang et al., 2007). There are few conclusions regarding clinical aspects supporting the impact of different genotypes of CYP2C9 on VRZ metabolism, and there are some indications that CYP2C9 genotypes may not be associated with VRZ pharmacokinetics. The current research has made the function of CYP2C9 in VRZ metabolism somewhat controversial. According to Niwa et al., the CYP2C9 *2 allele results in less effective inhibition of CYP2C9 by VRZ compared to the CYP2C9 *1 and CYP2C9 *3 alleles (Niwa and Hata, 2016). It has been reported that the pharmacokinetic parameters of VRZ were not altered in individuals genotyped as CYP2C9 *2/*2 pure siblings (Liu and Mould, 2014). Furthermore, a study conducted on 35 healthy participants revealed that there was no impact of CYP2C9 on VRZ pharmacokinetic parameters, as determined by a multiple regression analysis of VRZ pharmacokinetics (Weiss et al., 2009). In conclusion, there are varying results from different studies regarding the impact of CYP2C9 genotype on VRZ, further research is necessary to reach a definitive conclusion.
P-glycoprotein (ABCB1) is one of the crucial transporter proteins in the human body and is essential for maintaining biological barriers (Tulsyan et al., 2016). Currently, there have been over 50 SNPs documented in the ABCB1 gene (Tanabe et al., 2001). According to Cascorbi et al., a specific variation, rs1045642, in exon 26 of the ABCB1 gene can lead to a decrease in protein function (Cascorbi et al., 2001). ABCB1 also has genetic polymorphisms that affect its transport activity and, thus, the pharmacokinetic parameters of its transported substrates (Sauna et al., 2007). It was found that VRZ can interact with CaMdr1p, a homolog of yeast P-glycoprotein and that VRZ can mildly inhibit the activity of P-glycoprotein, corroborating that VRZ may be a substrate of ABCB1(Wakieć et al., 2007). Few studies have been conducted on the effects of ABCB1 gene polymorphisms on VRZ metabolism, and no clear conclusions have been obtained. One study found that ABCB1 gene polymorphism has an impact on VRZ clearance (Weiss et al., 2009). It has been shown that the AA allele of the rs1045642 polymorphic locus carrying the ABCB1 gene is associated with reduced VRZ metabolism in healthy individuals compared to the GG genotype (Weiss et al., 2009; Allegra et al., 2018). However, Recent studies have shown that the ABCB1 gene polymorphism does not significantly impact blood concentrations of VRZ (Chuwongwattana et al., 2020). Therefore, To fully understand the impact of ABCB1 gene variations on VRZ metabolism, it is necessary to conduct studies using larger sample sizes encompassing different races.
In addition to the genetic involvement of CYP2C19, CYP3A4, CYP2C9, and ABCB1, several other genetic variants may also broadly affect VRZ concentrations in individuals. According to the study, subjects carrying the rs3781727 variant of the SLCO2B1 gene had reduced and delayed oral absorption of VRZ, and genotype CC + CT was associated with reduced VRZ exposure in healthy individuals compared to genotype TT (Lee et al., 2020). The presence of the AA genotype at the rs2461817 polymorphic site in the NR1I2 gene is associated with a decrease in VRZ concentrations; furthermore, the presence of the GG allele at the rs6785049 polymorphic site and the CC allele at the rs3814057 polymorphic site in the NR1I2 gene, the AA allele at the rs2266780 polymorphic site in the FMO3 gene, and the AA allele at the rs2266780 polymorphic site in the POR gene, as well as the GG allele at the rs10954732 polymorphic site in the POR gene, is correlated with an increase in VRZ concentrations (Zeng et al., 2020). Regression analysis confirmed the potential function of the rs4149117 GT/TT genotype group of the SLCO1B3 gene in predicting Ctrough reduction by VRZ, and one study showed that individuals carrying the GT + TT allele of the rs4149117 polymorphic locus of the SLCO1B3 gene were associated with reduced Ctrough in children (Allegra et al., 2018). The ABCC2 gene encodes a transporter protein that has a tremendous impact on the transport and clearance of VRZ, and the rs717620 polymorphic locus CT + TT allele carrying the ABCC2 gene and the rs13120400 polymorphic locus CC allele carrying the ABCG2 gene were also associated with elevated Ctrough in children (Allegra et al., 2018).
Overall, among the genetic influences on VRZ dose adjustment, CYP2C19 gene polymorphisms were the most influential, accounting for approximately 50% of VRZ variability (Amsden and Gubbins, 2017). Although CYP3A4 is associated with the pharmacokinetic and pharmacodynamic properties of VRZ, the effect of CYP3A4 on the metabolism of VRZ in vivo is less than that of CYP2C19, which is about 1/50 of CYP2C19 (Hyland et al., 2003; Murayama et al., 2007). The effect of CYP2C9 on the dose adjustment of VRZ is more slight, and it is only involved in a small part of the nitrogen oxidation metabolism of VRZ (Dorji et al., 2019). Although several studies have shown that CYP3A5, ABCB1, SLCO2B1, NR1I2, FMO3 and other genes have an effect on VRZ metabolism, these effects are relatively small compared with CYP2C19, CYP3A4, and CYP2C9. Moreover, there is a considerable amount of confounding factors and a lack of consistent conclusions in these studies, warranting further research in this area.
The recommended dose of VRZ for treating IFI in adults varies between countries and regions. Thus, it is important to adjust the dosage for each individual (See Table 4: Comparison of recommended doses in different countries). Overall, the recommended doses varied somewhat between countries. Still, all had the same intravenous loading dose and maintenance dose, and there was less variation between countries in oral dosing, and all recommended inter-individual dose adjustments based on Ctrough.
Different countries have varying Ctrough levels. For instance, Chinese guidelines suggest a minimum of 0.5 mg/L and a maximum of 5 mg/L for VRZ target Ctrough (Chen et al., 2018). The Japanese guidelines state that Ctrough ≥12 mg/L can achieve clinical efficacy, and individuals with Ctrough >4–5 mg/L should be monitored for elevated related indicators (Roberts et al., 2012). According to the 2016 guidelines in the US, individuals should maintain a minimum requirement of 1–1.5 mg/L and a maximum requirement of 5–6 mg/L for Ctrough (Patterson et al., 2016). European 2017 guidelines recommend that the lower limit of Ctrough in individuals should be 1–1.5 mg/L, and the recommended Ctrough for severe infections is 2–6 mg/L (Ullmann et al., 2018). The British Society for Medical Mycology (BSMM) antifungal drug TDM guideline recommendation defines the VRZ treatment window as 2 ∼ 6 mg/L (Ashbee et al., 2014).
In terms of dose adjustment, the “VRZ Personalized Medication Guidelines,” published by the Chinese Pharmacology Society, recommend using a population pharmacokinetic model based on the Chinese public to adjust VRZ dosing. For individuals with a steady-state Ctrough below the lower limit of the target Ctrough or poor efficacy, it is recommended that the VRZ maintenance dose be increased by 50% and then adjusted according to Ctrough; for individuals with a steady-state Ctrough above the upper limit of the target Ctrough and below 10 mg/L, and in the absence of grade 2 or higher adverse events, it is recommended that the VRZ maintenance dose be diminished by 20% and then adjusted according to Ctrough; For individuals with steady-state Ctrough above 10 mg/L or Grade 2 adverse events, then VRZ is recommended to be discontinued for one dose, followed by a maintenance dose reduction of 50%, followed by adjustment based on Ctrough (Chen et al., 2018).
Individuals with hepatic insufficiency may face a higher risk of ADRs due to the potential accumulation of VRZ caused by decreased hepatic blood flow and enzyme activity. Individuals with hepatic insufficiency are advised to follow the VRZ instructions. For those with mild to moderate hepatic insufficiency (Child-Pugh A/B), a standard loading dose and a maintenance dose that is half the usual dosage are recommended (Chen and Chen, 2021). However, it is unclear what the proper dosing of VRZ should be for individuals with serious hepatic insufficiency (Child-Pugh C). Studies have indicated that the recommended VRZ loading dose and maintenance dose halving are not suitable and that reducing the maintenance dose by half can result in perilously high drug levels in these individuals (Wang et al., 2018b; Spernovasilis and Kofteridis, 2018). Therefore, conducting a pharmacokinetic study of VRZ in this particular population is crucial to develop suitable dosage schedules (See Table 5: Recommendations for dose adjustment in hepatic insufficiency).
The 12 studies have examined the use of VRZ in individuals with hepatic insufficiency. All have concluded that the currently recommended dose is unsuitable for these individuals and requires adjustment. Of these, 10 studies provided specific recommendations for dose adjustments, but only 5 gave both loading and maintenance doses, while the other 5 only provided maintenance doses. 8 retrospective multisample studies and 1 case report have shown that the standard loading dose and maintenance dose for individuals with hepatic insufficiency may not be appropriate, particularly for those in Child-Pugh class B and C. This is because of their higher Ctrough levels, which increase the risk of serious ADRs. To prevent elevated Ctrough levels and associated ADRs, it is advisable to consider lower doses, longer dosing intervals, and early TDM for these patients.
In individuals with hepatic dysfunction, total bilirubin has been identified as a crucial factor in predicting the pharmacokinetic parameters of VRZ. Optimizing the VRZ dosage to align with the total bilirubin levels can enhance treatment effectiveness. A prospective observational study categorized individuals with hepatic insufficiency into three levels based on total bilirubin levels and determined the optimal therapeutic dosage of VRZ for each bilirubin level (refer to Table 5); additionally, the study found that the pharmacokinetics of VRZ can be appropriately described using a one-compartment model with first-order absorption and elimination in individuals with hepatic dysfunction (Tang et al., 2021). These findings align with former retrospective studies and the research conducted by Pascual et al. (2012) and Wang et al. (2014a) on the pharmacokinetics of VRZ in individuals. A population-based pharmacokinetic modeling study showed that individuals with Ctrough >5.12 mg/L were more likely to experience VRZ-related ADRs, and individuals with hepatic insufficiency should receive a reduced half-load dose regimen compared with individuals with normal liver function, and the VRZ maintenance dose should be reduced to one-third for Child-Pugh A/B individuals and one-quarter for Child-Pugh C individuals (Wang et al., 2021). CYP2C19 phenotype plays a crucial role in selecting VRZ treatment regimens in individuals with liver insufficiency. When CYP2C19 activity is reduced, individuals with the same degree of liver insufficiency can further reduce the dose of VRZ. The results of a dosing regimen optimization based on MonteCarlo simulation showed that the maintenance dose of VRZ should be decreased to less than 50% in individuals with mild to moderate hepatic insufficiency with extensive CYP2C19 metabolism and o 1/4 in individuals with moderate to severe hepatic insufficiency (Ren et al., 2019). Dote et al. found that taking glucocorticoids alongside VRZ lowers plasma levels, while taking proton pump inhibitors increases plasma levels (Dote et al., 2016). Some studies have indicated that steroids are a hazard element for fungal infections in individuals with liver failure; Liu et al. (2017) found that VRZ is safe in individuals with fungal pneumonia and that low-maintenance doses of VRZ (100 mg/d) can achieve effective Ctrough without causing liver damage, but Ctrough of VRZ should be carefully monitored. A prospective observational study has shown that the regular VRZ dose can be increased by 50 mg in individuals with hepatic insufficiency at a MIC of 1 mg/L, but Ctrough needs to be monitored carefully to avoid severe ADRs; When the MIC is ≥ 2 mg/L, other alternative drugs are recommended, and depending on the type of fungal pathogen and its susceptibility to VRZ, lower doses or longer dosing intervals should be recommended to individuals with hepatic insufficiency (Lin et al., 2022).
VRZ, a widely used broad-spectrum antifungal medication for treating fungal infections, shows significant variability in its pharmacokinetics and pharmacodynamics among individuals. This is due to the involvement of multiple metabolic pathways and influencing factors. More and more research has emphasized the significance of genetic polymorphisms and hepatic insufficiency in determining appropriate VRZ dosage adjustments for individuals with IFI. Recent studies have investigated the potential relationship between genetic variations, such as CYP2C19, CYP3A4, ABCB1, ABCC2, FMO3, and POR, and the pharmacokinetics and pharmacodynamics of VRZ. Among these genes, CYP2C19 has the strongest impact on VRZ metabolism and clearance, followed by CYP3A4. Certain variants, like CYP2C19*2 and CYP2C19*3, reduce the enzymatic activity of CYP2C19, which results in higher drug exposure. On the contrary, variants such as CYP2C19*17 enhance CYP2C19 activity, resulting in faster VRZ metabolism and reduced drug exposure. ABCB1 and ABCC2 genotypes may influence VRZ transport and distribution, while FMO3 and POR genotypes could potentially impact its metabolism and clearance. However, it is important to note that the findings from different studies are not always consistent, warranting further research to understand the specific effects of each genotype on VRZ.
The treatment of patients with hepatic insufficiency complicated by IFI is a clinical challenge and a topic of great interest. While existing pharmacokinetic studies, clinical trials, and post-marketing safety data of available antifungal agents can assist clinicians in optimizing antifungal treatment regimens in patients with mild to moderate hepatic insufficiency and IFI, the recommended dosage adjustments for patients with severe hepatic insufficiency remain unclear in most current guidelines. Furthermore, the majority of dose adjustment studies for VRZ in patients with hepatic insufficiency have primarily focused on maintenance doses, with limited recommendations for loading doses. Moreover, there are discrepancies in the recommended adjustment doses across different studies, highlighting the lack of consensus. Therefore, further pharmacokinetic and clinical research is warranted to guide the use of VRZ in patients with hepatic insufficiency. Additionally, TDM of antifungal agents should be strengthened in clinical practice for patients with hepatic insufficiency and IFI to prevent or promptly identify hepatic and renal impairment, thereby avoiding adverse clinical outcomes. Furthermore, there is limited evidence and research on the dose adjustment of antifungal agents based on genotype and phenotype in patients with hepatic insufficiency, necessitating more extensive investigation in this aspect.
The current recommendations in guidelines and package inserts regarding patients with mild to moderate hepatic insufficiency (Child-Pugh A and B) who are prescribed VRZ suggest standard loading doses and halved maintenance doses, but this approach is likely to result in high Ctrough levels in patients, making it potentially inappropriate. Several ADRs associated with VRZ use have been found to directly correlate with Ctrough levels (Zonios et al., 2008; Tang et al., 2021). A meta-analysis conducted to assess the utility of TDM revealed a significantly higher frequency of toxic adverse events in patients with Ctrough levels ranging from 4.0 to 6.0 mg/L compared to those with lower Ctrough levels (Luong et al., 2016). Furthermore, a review of plasma monitoring studies for VRZ demonstrated that maintaining a treatment window of >1–2 mg/L and <5–5.5 mg/L was associated with improved efficacy and reduced toxicity (Karthaus et al., 2015). Additionally, a randomized controlled trial evaluating the utility of TDM in patients receiving VRZ treatment for IFI found that patients undergoing TDM exhibited a significant increase in complete or partial treatment response, with fewer discontinuations due to adverse events (Park et al., 2012). Moreover, the “VRZ personalized dosing guideline” strongly recommends Ctrough monitoring for patients with hepatic insufficiency, those co-administering drugs that affect VRZ pharmacokinetics, patients with CYP2C19 gene mutations, patients experiencing VRZ-related adverse events or suboptimal treatment efficacy, and critically ill patients with life-threatening fungal infections (Chen et al., 2018). It is evident that conducting Ctrough monitoring in hepatic insufficiency patients using VRZ is highly necessary. TDM should be initiated early when administering VRZ, and if steady-state Ctrough falls below the lower limit or if treatment efficacy is suboptimal, dosage adjustments should be made according to the dose adjustment scheme outlined in the “VRZ personalized dosing guideline.” Additionally, the CYP2C19 gene phenotype plays a crucial role in determining VRZ dosage in patients with hepatic insufficiency. When making dosage adjustments, special attention should be given to the impact of CYP2C19 gene phenotype in hepatic insufficiency patients on VRZ dosage adjustments (Tang et al., 2019).
From an individualized dosing perspective, hepatic insufficiency and genetic polymorphisms are two important factors influencing the administration dosage of VRZ in patients. In terms of the genetic impact on VRZ dose adjustments, the majority of the influence is attributed to the involvement of CYP2C19, CYP3A4, and CYP2C9, with CYP2C19 being particularly significant (accounting for approximately 50% of VRZ variability). Therefore, it is crucial to focus on the impact of CYP2C19, CYP3A4, and CYP2C9 gene phenotypes in hepatic insufficiency patients on VRZ plasma concentrations, as this holds positive implications for the successful treatment of hepatic insufficiency with concomitant invasive fungal infections. Other genes such as CYP3A5, ABCB1, SLCO2B1, NR1I2, and FMO3, which have lesser impact, may also be considered to some extent. To validate the safety and efficacy of VRZ dose adjustment strategies based on genotypes and liver function, future research should further investigate how to optimize the therapeutic approach of VRZ and better utilize genetic testing and clinical practice guidelines to guide VRZ dosage adjustments. This includes expanding the sample size and enhancing comparative studies among different populations, which can further elucidate the influence of genetic polymorphisms on VRZ pharmacokinetics and pharmacodynamics. Furthermore, since hepatic insufficiency patients often present with other diseases and receive concurrent medication, these factors may also impact VRZ pharmacokinetics and dose adjustments. Lastly, further research is necessary to examine the influence of genetic polymorphisms on ADRs, in order to guide clinical drug use and personalized treatment.
In conclusion, pharmacogenomics-based VRZ dose adjustment offers accurate and personalized treatment for hepatic insufficiency, improving outcomes and reducing ADRs. Compared to those with normal liver function, patients with hepatic insufficiency require lower drug doses and longer dosing intervals. Early TDM is crucial to mitigate potential adverse events. Additionally, the impact of CYP2C19, CYP3A4, and CYP2C9 genes on hepatic insufficiency patients with IFI should be carefully considered. Future high-quality pharmacogenomics trials are urgently needed to enhance evidence-based medicine and pharmacology for the diagnosis and treatment of hepatic insufficiency patients with IFI.
GL, QnL, CZ, QY, QiL, XZ, RY, and XY participated in the literature review, analysis, and manuscript writing. HL and YY guided manuscript writing and participated in manuscript revision. All authors contributed to the article and approved the submitted version.
This study was supported by research grants from the Chongqing Science and Health Joint Medical Research Project (No. 2022MSXM110).
We acknowledge the support from Sichuan Academy of Medical Sciences & Sichuan Provincial People’s Hospital, China Pharmaceutical University, University of Electronic Science and Technology of China, Southwest Medical University. And thank YY for his contribution to the paper.
The authors declare that the research was conducted in the absence of any commercial or financial relationships that could be construed as a potential conflict of interest.
All claims expressed in this article are solely those of the authors and do not necessarily represent those of their affiliated organizations, or those of the publisher, the editors and the reviewers. Any product that may be evaluated in this article, or claim that may be made by its manufacturer, is not guaranteed or endorsed by the publisher.
Alffenaar, J.-W. C., de Vos, T., Uges, D. R. A., and Daenen, S. M. G. J. (2009). High voriconazole trough levels in relation to hepatic function: how to adjust the dosage? Br. J. Clin. Pharmacol. 67, 262–263. doi:10.1111/j.1365-2125.2008.03315.x
Allegra, S., Fatiguso, G., Francia, S. D., Pirro, E., Carcieri, C., Cusato, J., et al. (2018). Pharmacogenetic of voriconazole antifungal agent in pediatric patients. Pharmacogenomics 19, 913–925. doi:10.2217/pgs-2017-0173
Amsden, J. R., and Gubbins, P. O. (2017). Pharmacogenomics of triazole antifungal agents: implications for safety, tolerability and efficacy. Expert Opin. Drug Metab. Toxicol. 13, 1135–1146. doi:10.1080/17425255.2017.1391213
Andrade, J. C., Kumar, S., Kumar, A., Černáková, L., and Rodrigues, C. F. (2022). Application of probiotics in candidiasis management. Crit. Rev. Food Sci. Nutr. 62, 8249–8264. doi:10.1080/10408398.2021.1926905
Ashbee, H. R., Barnes, R. A., Johnson, E. M., Richardson, M. D., Gorton, R., and Hope, W. W. (2014). Therapeutic drug monitoring (TDM) of antifungal agents: guidelines from the British society for medical Mycology. J. Antimicrob. Chemother. 69, 1162–1176. doi:10.1093/jac/dkt508
Baririan, N., Van Obbergh, L., Desager, J.-P., Verbeeck, R. K., Wallemacq, P., Starkel, P., et al. (2007). Alfentanil-induced miosis as a surrogate measure of alfentanil pharmacokinetics in patients with mild and moderate liver cirrhosis. Clin. Pharmacokinet. 46, 261–270. doi:10.2165/00003088-200746030-00006
Bassetti, M., Peghin, M., Carnelutti, A., Righi, E., Merelli, M., Ansaldi, F., et al. (2017). Clinical characteristics and predictors of mortality in cirrhotic patients with candidemia and intra-abdominal candidiasis: a multicenter study. Intensive Care Med. 43, 509–518. doi:10.1007/s00134-017-4717-0
Benitez, L. L., and Carver, P. L. (2019). Adverse effects associated with long-term administration of azole antifungal agents. Drugs 79, 833–853. doi:10.1007/s40265-019-01127-8
Blanco-Dorado, S., Maroñas, O., Latorre-Pellicer, A., Rodríguez Jato, M. T., López-Vizcaíno, A., Gómez Márquez, A., et al. (2020). Impact of CYP2C19 genotype and drug interactions on voriconazole plasma concentrations: a Spain pharmacogenetic-pharmacokinetic prospective multicenter study. Pharmacotherapy 40, 17–25. doi:10.1002/phar.2351
Cai, X., Li, W., Yang, J., Wu, G., Song, J., Gong, X., et al. (2023). Is halving maintenance of voriconazole safe and efficient in patients suffering from invasive fungal infections with serious hepatic dysfunction? Infect. Drug Resist. 16, 1–8. doi:10.2147/IDR.S390026
Cascorbi, I., Gerloff, T., Johne, A., Meisel, C., Hoffmeyer, S., Schwab, M., et al. (2001). Frequency of single nucleotide polymorphisms in the P-glycoprotein drug transporter MDR1 gene in white subjects. Clin. Pharmacol. Ther. 69, 169–174. doi:10.1067/mcp.2001.114164
Chau, M. M., Kong, D. C. M., van Hal, S. J., Urbancic, K., Trubiano, J. A., Cassumbhoy, M., et al. (2014). Consensus guidelines for optimising antifungal drug delivery and monitoring to avoid toxicity and improve outcomes in patients with haematological malignancy, 2014. Intern. Med. J. 44, 1364–1388. doi:10.1111/imj.12600
Chen, D. L., and Chen, J. (2021). Antifungal therapy for severe liver disease complicated with invasive fungal disease. Liver 26, 804–807. doi:10.14000/j.carolcarrollnki.Issn1008-1704.2021.07.025
Chen, D., Qian, Z., Su, H., Meng, Z., Lv, J., Huang, Y., et al. (2021). Invasive pulmonary aspergillosis in acute-on-chronic liver failure patients: short-term outcomes and antifungal options. Infect. Dis. Ther. 10, 2525–2538. doi:10.1007/s40121-021-00524-5
Chen, K., Zhang, X., Ke, X., Du, G., Yang, K., and Zhai, S. (2018). Individualized medication of voriconazole: a practice guideline of the division of therapeutic drug monitoring, Chinese pharmacological society. Ther. Drug Monit. 40, 663–674. doi:10.1097/FTD.0000000000000561
Chen, T., Ning, Q., Chen, L., Chen, Z., Wang, B., and Zhou, D. (2022). The effects of fructose diphosphate on routine coagulation tests in vitro. J. Clin. Hepatobiliary Dis. 38, 304–310. doi:10.1038/s41598-021-04263-y
Cheng, Y., Wang, L., Iacono, L., Zhang, D., Chen, W., Gong, J., et al. (2018). Clinical significance of CYP2C19 polymorphisms on the metabolism and pharmacokinetics of 11β-hydroxysteroid dehydrogenase type-1 inhibitor BMS-823778. Br. J. Clin. Pharmacol. 84, 130–141. doi:10.1111/bcp.13421
Cheong, H. S., Kang, C. I., Lee, J. A., Moon, S. Y., Joung, M. K., Chung, D. R., et al. (2009). Clinical significance and outcome of nosocomial acquisition of spontaneous bacterial peritonitis in patients with liver cirrhosis. Clin. Infect. Dis. 48 (9), 1230–1236. doi:10.1086/597585
Chuwongwattana, S., Jantararoungtong, T., Prommas, S., Medhasi, S., Puangpetch, A., and Sukasem, C. (2020). Impact of CYP2C19, CYP3A4, ABCB1, and FMO3 genotypes on plasma voriconazole in Thai patients with invasive fungal infections. Pharmacol. Res. Perspect. 8, e00665. doi:10.1002/prp2.665
Denning, D. W., Ribaud, P., Milpied, N., Caillot, D., Herbrecht, R., Thiel, E., et al. (2002). Efficacy and safety of voriconazole in the treatment of acute invasive aspergillosis. Clin. Infect. Dis. 34, 563–571. doi:10.1086/324620
Dolton, M. J., Ray, J. E., Chen, S. C.-A., Ng, K., Pont, L. G., and McLachlan, A. J. (2012). Multicenter study of voriconazole pharmacokinetics and therapeutic drug monitoring. Antimicrob. Agents Chemother. 56, 4793–4799. doi:10.1128/AAC.00626-12
Dorji, P. W., Tshering, G., and Na-Bangchang, K. (2019). CYP2C9, CYP2C19, CYP2D6 and CYP3A5 polymorphisms in south-east and east asian populations: A systematic review. J. Clin. Pharm. Ther. 44, 508–524. doi:10.1111/jcpt.12835
Dote, S., Sawai, M., Nozaki, A., Naruhashi, K., Kobayashi, Y., and Nakanishi, H. (2016). A retrospective analysis of patient-specific factors on voriconazole clearance. J. Pharm. Health Care Sci. 2, 10. doi:10.1186/s40780-016-0044-9
Douglas, A. P., Smibert, O. C., Bajel, A., Halliday, C. L., Lavee, O., McMullan, B., et al. (2021). Consensus guidelines for the diagnosis and management of invasive aspergillosis, 2021. Intern. Med. J. 51 (7), 143–176. doi:10.1111/imj.15591
Ertem, O., Tufekci, O., Oren, H., Tuncok, Y., Ergon, M. C., and Gumustekin, M. (2022). Evaluation of voriconazole related adverse events in pediatric patients with hematological malignancies. J. Oncol. Pharm. Pract. 29, 861–873. doi:10.1177/10781552221086887
Feldman, E. B., Bellinghausen, A. L., Vodkin, I. E., Abeles, S. R., and Kamdar, B. B. (2023). Secondary peritonitis in a patient with cirrhosis involving Hyphopichia burtonii, an emerging fungal pathogen. IDCases 31, e01730. doi:10.1016/j.idcr.2023.e01730
Fernández, J., Acevedo, J., Wiest, R., Gustot, T., Amoros, A., Deulofeu, C., et al. (2018). Bacterial and fungal infections in acute-on-chronic liver failure: prevalence, characteristics and impact on prognosis. Gut 67, 1870–1880. doi:10.1136/gutjnl-2017-314240
Gao, J., Zhang, Q., Wu, Y., Li, Y., Qi, T., Zhu, C., et al. (2018). Improving survival of acute-on-chronic liver failure patients complicated with invasive pulmonary aspergillosis. Sci. Rep. 8, 876. doi:10.1038/s41598-018-19320-2
García-García, I., and Borobia, A. M. (2021). Current approaches and future strategies for the implementation of pharmacogenomics in the clinical use of azole antifungal drugs. Expert Opin. Drug Metab. Toxicol. 17, 509–514. doi:10.1080/17425255.2021.1890715
Gautier-Veyret, E., Fonrose, X., Tonini, J., Thiebaut-Bertrand, A., Bartoli, M., Quesada, J.-L., et al. (2015). Variability of voriconazole plasma concentrations after allogeneic hematopoietic stem cell transplantation: impact of cytochrome p450 polymorphisms and comedications on initial and subsequent trough levels. Antimicrob. Agents Chemother. 59, 2305–2314. doi:10.1128/AAC.04838-14
Hadfield, N. J., Selvendran, S., and Johnston, M. P. (2019). Fatal Pneumocystis jirovecii pneumonia in a non-immunocompromised patient with alcohol-related liver cirrhosis. Scott. Med. J. 64, 148–153. doi:10.1177/0036933019872629
Hakkola, J., Hukkanen, J., Turpeinen, M., and Pelkonen, O. (2020). Inhibition and induction of CYP enzymes in humans: an update. Arch. Toxicol. 94, 3671–3722. doi:10.1007/s00204-020-02936-7
Hall, V. G., Tang, K., Kumar, D., Rotstein, C., Chow, S., Chan, S. M., et al. (2023). Breakthrough invasive fungal infection after coadministration of venetoclax and voriconazole. Open Forum Infect. Dis. 10, ofad134. doi:10.1093/ofid/ofad134
Hamada, Y., Seto, Y., Yago, K., and Kuroyama, M. (2012). Investigation and threshold of optimum blood concentration of voriconazole: a descriptive statistical meta-analysis. J. Infect. Chemother. 18, 501–507. doi:10.1007/s10156-011-0363-6
Hamada, Y., Tokimatsu, I., Mikamo, H., Kimura, M., Seki, M., Takakura, S., et al. (2013). Practice guidelines for therapeutic drug monitoring of voriconazole: a consensus review of the Japanese Society of Chemotherapy and the Japanese Society of Therapeutic Drug Monitoring. J. Infect. Chemother. 19 (3), 381–392. doi:10.1007/s10156-013-0607-8
Hamadeh, I. S., Klinker, K. P., Borgert, S. J., Richards, A. I., Li, W., Mangal, N., et al. (2017). Impact of the CYP2C19 genotype on voriconazole exposure in adults with invasive fungal infections. Pharmacogenet. Genomics 27, 190–196. doi:10.1097/FPC.0000000000000277
He, H.-R., Sun, J.-Y., Ren, X.-D., Wang, T.-T., Zhai, Y.-J., Chen, S.-Y., et al. (2015). Effects of CYP3A4 polymorphisms on the plasma concentration of voriconazole. Eur. J. Clin. Microbiol. Infect. Dis. 34, 811–819. doi:10.1007/s10096-014-2294-5
He, L., Chen, S., Li, J., Xie, X., Huang, L., Kuang, Y., et al. (2020). Genetic and phenotypic frequency distribution of CYP2C9, CYP2C19 and CYP2D6 in over 3200 Han Chinese. Clin. Exp. Pharmacol. Physiol. 47, 1659–1663. doi:10.1111/1440-1681.13357
Hicks, J. K., Quilitz, R. E., Komrokji, R. S., Kubal, T. E., Lancet, J. E., Pasikhova, Y., et al. (2020). Prospective CYP2C19-guided voriconazole prophylaxis in patients with neutropenic acute myeloid leukemia reduces the incidence of subtherapeutic antifungal plasma concentrations. Clin. Pharmacol. Ther. 107, 563–570. doi:10.1002/cpt.1641
Hou, Z., Tan, D., Liu, G., Xie, Y., Li, C., Xie, J., et al. (2010). Clinical characteristics and therapeutic analysis of invasive fungal infection in chronic severe hepatitis patients. Zhong Nan Da Xue Xue Bao Yi Xue Ban. 35, 537–542. doi:10.3969/j.issn.1672-7347.2010.06.001
Hulin, A., Dailly, E., and Le Guellec, C.Groupe Suivi Therapeutique Pharmacologique de la Societe Francaise de Pharmacologie et de Therapeutique (2011). Level of evidence for therapeutic drug monitoring of voriconazole. Therapie 66, 109–114. doi:10.2515/therapie/2011009
Hwang, S. Y., Yu, S. J., Lee, J. H., Kim, J. S., Yoon, J. W., Kim, Y. J., et al. (2014). Spontaneous fungal peritonitis: a severe complication in patients with advanced liver cirrhosis. Eur. J. Clin. Microbiol. Infect. Dis. 33, 259–264. doi:10.1007/s10096-013-1953-2
Hyland, R., Jones, B. C., and Smith, D. A. (2003). Identification of the cytochrome P450 enzymes involved in the N-oxidation of voriconazole. Drug Metab. Dispos. Biol. Fate Chem. 31, 540–547. doi:10.1124/dmd.31.5.540
Jenks, J. D., Cornely, O. A., Chen, S. C.-A., Thompson, G. R., and Hoenigl, M. (2020). Breakthrough invasive fungal infections: who is at risk? Mycoses 63, 1021–1032. doi:10.1111/myc.13148
Jenks, J. D., Nam, H. H., and Hoenigl, M. (2021). Invasive aspergillosis in critically ill patients: review of definitions and diagnostic approaches. Mycoses 64, 1002–1014. doi:10.1111/myc.13274
Jin, H., Wang, T., Falcione, B. A., Olsen, K. M., Chen, K., Tang, H., et al. (2016). Trough concentration of voriconazole and its relationship with efficacy and safety: a systematic review and meta-analysis. J. Antimicrob. Chemother. 71, 1772–1785. doi:10.1093/jac/dkw045
Job, K. M., Olson, J., Stockmann, C., Constance, J. E., Enioutina, E. Y., Rower, J. E., et al. (2016). Pharmacodynamic studies of voriconazole: informing the clinical management of invasive fungal infections. Expert Rev. Anti Infect. Ther. 14, 731–746. doi:10.1080/14787210.2016.1207526
Jović, Z., Janković, S. M., Ružić Zečević, D., Milovanović, D., Stefanović, S., Folić, M., et al. (2019). Clinical pharmacokinetics of second-generation triazoles for the treatment of invasive aspergillosis and candidiasis. Eur. J. Drug Metab. Pharmacokinet. 44, 139–157. doi:10.1007/s13318-018-0513-7
Kang, W.-H., Song, G.-W., Lee, S.-G., Suh, K.-S., Lee, K.-W., Yi, N.-J., et al. (2020). A multicenter, randomized, open-label study to compare micafungin with fluconazole in the prophylaxis of invasive fungal infections in living-donor liver transplant recipients. J. Gastrointest. Surg. 24, 832–840. doi:10.1007/s11605-019-04241-w
Karthaus, M., Lehrnbecher, T., Lipp, H.-P., Kluge, S., and Buchheidt, D. (2015). Therapeutic drug monitoring in the treatment of invasive aspergillosis with voriconazole in cancer patients—an evidence-based approach. Ann. Hematol. 94, 547–556. doi:10.1007/s00277-015-2333-z
Khalid, M., Neupane, R., Anjum, H., and Surani, S. (2021). Fungal infections following liver transplantation. World J. Hepatol. 13, 1653–1662. doi:10.4254/wjh.v13.i11.1653
Klyushova, L. S., Perepechaeva, M. L., and Grishanova, A. Y. (2022). The role of CYP3A in Health and disease. Biomedicines 10, 2686. doi:10.3390/biomedicines10112686
Kullberg, B. J., and Arendrup, M. C. (2015). Invasive candidiasis. N. Engl. J. Med. 373, 1445–1456. doi:10.1056/NEJMra1315399
Kyriakidis, I., Tragiannidis, A., Munchen, S., and Groll, A. H. (2017). Clinical hepatotoxicity associated with antifungal agents. Expert Opin. Drug Saf. 16, 149–165. doi:10.1080/14740338.2017.1270264
Lahmer, T., Brandl, A., Rasch, S., Baires, G. B., Schmid, R. M., Huber, W., et al. (2019). Prevalence and outcome of invasive pulmonary aspergillosis in critically ill patients with liver cirrhosis: an observational study. Sci. Rep. 9, 11919. doi:10.1038/s41598-019-48183-4
Lahmer, T., Brandl, A., Rasch, S., Schmid, R. M., and Huber, W. (2016). Fungal peritonitis: underestimated disease in critically ill patients with liver cirrhosis and spontaneous peritonitis. PloS One 11, e0158389. doi:10.1371/journal.pone.0158389
Lahmer, T., Peçanha-Pietrobom, P. M., Schmid, R. M., and Colombo, A. L. (2022). Invasive fungal infections in acute and chronic liver impairment: A systematic review. Mycoses 65, 140–151. doi:10.1111/myc.13403
Lamoureux, F., Duflot, T., Woillard, J.-B., Metsu, D., Pereira, T., Compagnon, P., et al. (2016). Impact of CYP2C19 genetic polymorphisms on voriconazole dosing and exposure in adult patients with invasive fungal infections. Int. J. Antimicrob. Agents 47, 124–131. doi:10.1016/j.ijantimicag.2015.12.003
Lee, C. R., Goldstein, J. A., and Pieper, J. A. (2002). Cytochrome P450 2C9 polymorphisms: a comprehensive review of the in-vitro and human data. Pharmacogenetics 12, 251–263. doi:10.1097/00008571-200204000-00010
Lee, C. R., Luzum, J. A., Sangkuhl, K., Gammal, R. S., Sabatine, M. S., Stein, C. M., et al. (2022). Clinical pharmacogenetics implementation Consortium guideline for CYP2C19 genotype and clopidogrel therapy: 2022 update. Clin. Pharmacol. Ther. 112, 959–967. doi:10.1002/cpt.2526
Lee, J., Ng, P., Hamandi, B., Husain, S., Lefebvre, M. J., and Battistella, M. (2021). Effect of therapeutic drug monitoring and cytochrome P450 2C19 genotyping on clinical outcomes of voriconazole: A systematic review. Ann. Pharmacother. 55, 509–529. doi:10.1177/1060028020948174
Lee, S., Kim, B.-H., Nam, W.-S., Yoon, S. H., Cho, J.-Y., Shin, S.-G., et al. (2012). Effect of CYP2C19 polymorphism on the pharmacokinetics of voriconazole after single and multiple doses in healthy volunteers. J. Clin. Pharmacol. 52, 195–203. doi:10.1177/0091270010395510
Lee, S. W., Oh, J., Kim, A. H., Ji, S. C., Park, S.-I., Yoon, S. H., et al. (2020). Oral absorption of voriconazole is affected by SLCO2B1 c*396T>C genetic polymorphism in CYP2C19 poor metabolizers. Pharmacogenomics J. 20, 792–800. doi:10.1038/s41397-020-0166-1
Lem, J., Younus, M., Aram, J. A., Moosavi, S., Freivogel, K., Lewis, A., et al. (2019). Evaluation of the effectiveness of additional risk minimization measures for voriconazole in the EU: findings and lessons learned from a healthcare professional survey. Pharm. Med. 33, 121–133. doi:10.1007/s40290-019-00273-4
Levin, M.-D., den Hollander, J. G., van der Holt, B., Rijnders, B. J., van Vliet, M., Sonneveld, P., et al. (2007). Hepatotoxicity of oral and intravenous voriconazole in relation to cytochrome P450 polymorphisms. J. Antimicrob. Chemother. 60, 1104–1107. doi:10.1093/jac/dkm330
Levine, M. T., and Chandrasekar, P. H. (2016). Adverse effects of voriconazole: over a decade of use. Clin. Transpl. 30, 1377–1386. doi:10.1111/ctr.12834
Li, S., Wu, S., Gong, W., Cao, P., Chen, X., Liu, W., et al. (2021). Application of population pharmacokinetic analysis to characterize CYP2C19 mediated metabolic mechanism of voriconazole and support dose optimization. Front. Pharmacol. 12, 730826. doi:10.3389/fphar.2021.730826
Li, X., Frechen, S., Moj, D., Lehr, T., Taubert, M., Hsin, C.-H., et al. (2020). A physiologically based pharmacokinetic model of voriconazole integrating time-dependent inhibition of CYP3A4, genetic polymorphisms of CYP2C19 and predictions of drug-drug interactions. Clin. Pharmacokinet. 59, 781–808. doi:10.1007/s40262-019-00856-z
Li, X., Yu, C., Wang, T., Chen, K., Zhai, S., and Tang, H. (2016). Effect of cytochrome P450 2C19 polymorphisms on the clinical outcomes of voriconazole: a systematic review and meta-analysis. Eur. J. Clin. Pharmacol. 72, 1185–1193. doi:10.1007/s00228-016-2089-y
Li, Z.-W., Peng, F.-H., Yan, M., Liang, W., Liu, X.-L., Wu, Y.-Q., et al. (2017). Impact of CYP2C19 genotype and liver function on voriconazole pharmacokinetics in renal transplant recipients. Ther. Drug Monit. 39, 422–428. doi:10.1097/FTD.0000000000000425
Libera, R., Dimala, C. A., Oakes, K., and Moss, C. (2023). Cryptococcal peritonitis in a patient with decompensated liver cirrhosis. J. Community Hosp. Intern. Med. Perspect. 13, 51–53. doi:10.55729/2000-9666.1139
Lin, X.-B., Li, Z.-W., Yan, M., Zhang, B.-K., Liang, W., Wang, F., et al. (2018). Population pharmacokinetics of voriconazole and CYP2C19 polymorphisms for optimizing dosing regimens in renal transplant recipients. Br. J. Clin. Pharmacol. 84, 1587–1597. doi:10.1111/bcp.13595
Lin, X.-B., Lui, K. Y., Guo, P.-H., Liu, X.-M., Liang, T., Hu, X.-G., et al. (2022). Population pharmacokinetic model-guided optimization of intravenous voriconazole dosing regimens in critically ill patients with liver dysfunction. Pharmacotherapy 42, 23–33. doi:10.1002/phar.2634
Liu, P., and Mould, D. R. (2014). Population pharmacokinetic-pharmacodynamic analysis of voriconazole and anidulafungin in adult patients with invasive aspergillosis. Antimicrob. Agents Chemother. 58, 4727–4736. doi:10.1128/AAC.02809-13
Liu, X., Su, H., Tong, J., Chen, J., Yang, H., Xiao, L., et al. (2017). Significance of monitoring plasma concentration of voriconazole in a patient with liver failure: A case report. Med. Baltim. 96, e8039. doi:10.1097/MD.0000000000008039
Lum, L., Lee, A., Vu, M., Strasser, S., and Davis, R. (2020). Epidemiology and risk factors for invasive fungal disease in liver transplant recipients in a tertiary transplant center. Transpl. Infect. Dis. 22, e13361. doi:10.1111/tid.13361
Luong, M.-L., Al-Dabbagh, M., Groll, A. H., Racil, Z., Nannya, Y., Mitsani, D., et al. (2016). Utility of voriconazole therapeutic drug monitoring: a meta-analysis. J. Antimicrob. Chemother. 71, 1786–1799. doi:10.1093/jac/dkw099
Maertens, J. A., Rahav, G., Lee, D.-G., Ponce-de-León, A., Ramírez Sánchez, I. C., Klimko, N., et al. (2021). Posaconazole versus voriconazole for primary treatment of invasive aspergillosis: a phase 3, randomised, controlled, non-inferiority trial. Lancet lond. Engl. 397, 499–509. doi:10.1016/S0140-6736(21)00219-1
Matsubara, V. H., Bandara, H. M. H. N., Mayer, M. P. A., and Samaranayake, L. P. (2016). Probiotics as antifungals in mucosal candidiasis. Clin. Infect. Dis. 62, 1143–1153. doi:10.1093/cid/ciw038
Medeiros, M. A. P., Melo, A. P. V., Bento, A. O., Souza, L. B. F. C., Neto, F. A. B., Garcia, J. B. L., et al. (2019). Epidemiology and prognostic factors of nosocomial candidemia in northeast Brazil: A six-year retrospective study. PloS One 14, e0221033. doi:10.1371/journal.pone.0221033
Miao, Q., Tang, J.-T., van Gelder, T., Li, Y.-M., Bai, Y.-J., Zou, Y.-G., et al. (2019). Correlation of CYP2C19 genotype with plasma voriconazole exposure in South-western Chinese Han patients with invasive fungal infections. Med. Baltim. 98, e14137. doi:10.1097/MD.0000000000014137
Mikus, G., Scholz, I. M., and Weiss, J. (2011). Pharmacogenomics of the triazole antifungal agent voriconazole. Pharmacogenomics 12, 861–872. doi:10.2217/pgs.11.18
Mikus, G., Schöwel, V., Drzewinska, M., Rengelshausen, J., Ding, R., Riedel, K.-D., et al. (2006). Potent cytochrome P450 2C19 genotype-related interaction between voriconazole and the cytochrome P450 3A4 inhibitor ritonavir. Clin. Pharmacol. Ther. 80, 126–135. doi:10.1016/j.clpt.2006.04.004
Moriyama, B., Obeng, A. O., Barbarino, J., Penzak, S. R., Henning, S. A., Scott, S. A., et al. (2017). Clinical pharmacogenetics implementation Consortium (CPIC) guidelines for CYP2C19 and voriconazole therapy. Clin. Pharmacol. Ther. 102, 45–51. doi:10.1002/cpt.583
Murayama, N., Imai, N., Nakane, T., Shimizu, M., and Yamazaki, H. (2007). Roles of CYP3A4 and CYP2C19 in methyl hydroxylated and N-oxidized metabolite formation from voriconazole, a new anti-fungal agent, in human liver microsomes. Biochem. Pharmacol. 73, 2020–2026. doi:10.1016/j.bcp.2007.03.012
Niwa, T., and Hata, T. (2016). The effect of genetic polymorphism on the inhibition of azole antifungal agents against CYP2C9-mediated metabolism. J. Pharm. Sci. 105, 1345–1348. doi:10.1016/j.xphs.2016.01.007
Pallet, N., and Loriot, M. A. (2021). Voriconazole pharmacogenetics. Lancet lond. Engl. 398, 578. doi:10.1016/S0140-6736(21)00879-5
Park, W. B., Kim, N.-H., Kim, K.-H., Lee, S. H., Nam, W.-S., Yoon, S. H., et al. (2012). The effect of therapeutic drug monitoring on safety and efficacy of voriconazole in invasive fungal infections: a randomized controlled trial. Clin. Infect. Dis. 55, 1080–1087. doi:10.1093/cid/cis599
Pascual, A., Calandra, T., Bolay, S., Buclin, T., Bille, J., and Marchetti, O. (2008). Voriconazole therapeutic drug monitoring in patients with invasive mycoses improves efficacy and safety outcomes. Clin. Infect. Dis. 46, 201–211. doi:10.1086/524669
Pascual, A., Csajka, C., Buclin, T., Bolay, S., Bille, J., Calandra, T., et al. (2012). Challenging recommended oral and intravenous voriconazole doses for improved efficacy and safety: population pharmacokinetics-based analysis of adult patients with invasive fungal infections. Clin. Infect. Dis. 55, 381–390. doi:10.1093/cid/cis437
Patterson, T. F., Thompson, G. R., Denning, D. W., Fishman, J. A., Hadley, S., Herbrecht, R., et al. (2016). Practice guidelines for the diagnosis and management of aspergillosis: 2016 update by the infectious diseases society of America. Clin. Infect. Dis. 63, e1–e60. doi:10.1093/cid/ciw326
Pessayre, D., Fromenty, B., Berson, A., Robin, M.-A., Lettéron, P., Moreau, R., et al. (2012). Central role of mitochondria in drug-induced liver injury. Drug Metab. Rev. 44, 34–87. doi:10.3109/03602532.2011.604086
PharmGKB (2017). Voriconazole pathway, pharmacokinetics. Available at: https://www.pharmgkb.org/pathway/PA166160640 (Accessed June 5, 2023).
Piano, S., Singh, V., Caraceni, P., Maiwall, R., Alessandria, C., Fernandez, J., et al. (2019). Epidemiology and effects of bacterial infections in patients with cirrhosis worldwide. Gastroenterology 156, 1368–1380.e10. doi:10.1053/j.gastro.2018.12.005
Ren, Q.-X., Li, X.-G., Mu, J.-S., Bi, J.-F., Du, C.-H., Wang, Y.-H., et al. (2019). Population pharmacokinetics of voriconazole and optimization of dosage regimens based on Monte Carlo simulation in patients with liver cirrhosis. J. Pharm. Sci. 108, 3923–3931. doi:10.1016/j.xphs.2019.09.019
Roberts, J. D., Wells, G. A., Le May, M. R., Labinaz, M., Glover, C., Froeschl, M., et al. (2012). Point-of-care genetic testing for personalisation of antiplatelet treatment (RAPID GENE): a prospective, randomised, proof-of-concept trial. Lancet lond. Engl. 379, 1705–1711. doi:10.1016/S0140-6736(12)60161-5
Rose, C. F., Amodio, P., Bajaj, J. S., Dhiman, R. K., Montagnese, S., Taylor-Robinson, S. D., et al. (2020). Hepatic encephalopathy: novel insights into classification, pathophysiology and therapy. J. Hepatol. 73, 1526–1547. doi:10.1016/j.jhep.2020.07.013
Sauna, Z. E., Kim, I.-W., and Ambudkar, S. V. (2007). Genomics and the mechanism of P-glycoprotein (ABCB1). J. Bioenerg. Biomembr. 39, 481–487. doi:10.1007/s10863-007-9115-9
Si, D., Guo, Y., Zhang, Y., Yang, L., Zhou, H., and Zhong, D. (2004). Identification of a novel variant CYP2C9 allele in Chinese. Pharmacogenetics 14, 465–469. doi:10.1097/01.fpc.0000114749.08559.e4
Silva, J. T., Ruiz-Camps, I., and Aguado, J. M. (2021). Invasive fungal infection over the last 30 years. Rev. Iberoam. Micol. 38, 47–51. doi:10.1016/j.riam.2021.03.003
Sim, S. C., Risinger, C., Dahl, M.-L., Aklillu, E., Christensen, M., Bertilsson, L., et al. (2006). A common novel CYP2C19 gene variant causes ultrarapid drug metabolism relevant for the drug response to proton pump inhibitors and antidepressants. Clin. Pharmacol. Ther. 79, 103–113. doi:10.1016/j.clpt.2005.10.002
Spernovasilis, N., and Kofteridis, D. P. (2018). Pre-existing liver disease and toxicity of antifungals. J. Fungi Basel Switz. 4, 133. doi:10.3390/jof4040133
Su, H.-B., Wang, H.-F., Yan, T., Lin, F., Zhao, H., Li, L., et al. (2010). The clinical characteristics of hepatic failure with aspergillosis. Zhonghua Gan Zang Bing Za Zhi 18, 520–522. doi:10.3760/cma.j.issn.1007-3418.2010.07.012
Suzuki, Y., Tokimatsu, I., Sato, Y., Kawasaki, K., Sato, Y., Goto, T., et al. (2013). Association of sustained high plasma trough concentration of voriconazole with the incidence of hepatotoxicity. Clin. Chim. Acta Int. J. Clin. Chem. 424, 119–122. doi:10.1016/j.cca.2013.05.025
Tanabe, M., Ieiri, I., Nagata, N., Inoue, K., Ito, S., Kanamori, Y., et al. (2001). Expression of P-glycoprotein in human placenta: relation to genetic polymorphism of the multidrug resistance (MDR)-1 gene. J. Pharmacol. Exp. Ther. 297, 1137–1143. doi:10.1023/A:1011583210549
Tanaka, R., Fujioka, T., Suzuki, Y., Iwao, M., and Itoh, H. (2020). A prospective study on the usefulness of initial voriconazole dose adjustment based on CYP2C19 gene polymorphism analysis. Chemotherapy 65, 59–64. doi:10.1159/000509970
Tang, D., Song, B.-L., Yan, M., Zou, J.-J., Zhang, M., Zhou, H.-Y., et al. (2019). Identifying factors affecting the pharmacokinetics of voriconazole in patients with liver dysfunction: a population pharmacokinetic approach. Basic Clin. Pharmacol. Toxicol. 125, 34–43. doi:10.1111/bcpt.13208
Tang, D., Yan, M., Song, B.-L., Zhao, Y.-C., Xiao, Y.-W., Wang, F., et al. (2021). Population pharmacokinetics, safety and dosing optimization of voriconazole in patients with liver dysfunction: a prospective observational study. Br. J. Clin. Pharmacol. 87, 1890–1902. doi:10.1111/bcp.14578
Tariq, T., Irfan, F. B., Farishta, M., Dykstra, B., Sieloff, E. M., and Desai, A. P. (2019). Spontaneous fungal peritonitis: micro-organisms, management and mortality in liver cirrhosis-A systematic review. World J. Hepatol. 11, 596–606. doi:10.4254/wjh.v11.i7.596
Theuretzbacher, U., Ihle, F., and Derendorf, H. (2006). Pharmacokinetic/pharmacodynamic profile of voriconazole. Clin. Pharmacokinet. 45, 649–663. doi:10.2165/00003088-200645070-00002
Thompson, G. R., and Lewis, J. S. (2010). Pharmacology and clinical use of voriconazole. Expert Opin. Drug Metab. Toxicol. 6, 83–94. doi:10.1517/17425250903463878
Tulsyan, S., Mittal, R. D., and Mittal, B. (2016). The effect of ABCB1 polymorphisms on the outcome of breast cancer treatment. Pharmacogenomics Pers. Med. 9, 47–58. doi:10.2147/PGPM.S86672
Ueda, K., Nannya, Y., Kumano, K., Hangaishi, A., Takahashi, T., Imai, Y., et al. (2009). Monitoring trough concentration of voriconazole is important to ensure successful antifungal therapy and to avoid hepatic damage in patients with hematological disorders. Int. J. Hematol. 89, 592–599. doi:10.1007/s12185-009-0296-3
Ullmann, A. J., Aguado, J. M., Arikan-Akdagli, S., Denning, D. W., Groll, A. H., Lagrou, K., et al. (2018). Diagnosis and management of Aspergillus diseases: executive summary of the 2017 ESCMID-ECMM-ERS guideline. Clin. Microbiol. Infect. 24 (1), e1–e38. doi:10.1016/j.cmi.2018.01.002
Ullmann, A. J., Lipton, J. H., Vesole, D. H., Chandrasekar, P., Langston, A., Tarantolo, S. R., et al. (2007). Posaconazole or fluconazole for prophylaxis in severe graft-versus-host disease. N. Engl. J. Med. 356, 335–347. doi:10.1056/NEJMoa061098
Vasques, F., Cavazza, A., and Bernal, W. (2022). Acute liver failure. Curr. Opin. Crit. Care 28, 198–207. doi:10.1097/MCC.0000000000000923
Verbeeck, R. K. (2008). Pharmacokinetics and dosage adjustment in patients with hepatic dysfunction. Eur. J. Clin. Pharmacol. 64, 1147–1161. doi:10.1007/s00228-008-0553-z
Verma, N., Singh, S., Singh, M., Chauhan, A., Pradhan, P., Jaiswal, N., et al. (2022). Global epidemiological burden of fungal infections in cirrhosis patients: a systematic review with meta-analysis. Mycoses 65, 266–284. doi:10.1111/myc.13387
Wakieć, R., Prasad, R., Morschhäuser, J., Barchiesi, F., Borowski, E., and Milewski, S. (2007). Voriconazole and multidrug resistance in Candida albicans. Mycoses 50, 109–115. doi:10.1111/j.1439-0507.2006.01327.x
Walsh, T. J., Moriyama, B., Penzak, S. R., Klein, T. E., and Caudle, K. E. (2018). Response to “impact of CYP3A4 genotype on voriconazole exposure: new insights into the contribution of CYP3A4*22 to metabolism of voriconazole”. Clin. Pharmacol. Ther. 103, 187. doi:10.1002/cpt.811
Wang, T., Chen, S., Sun, J., Cai, J., Cheng, X., Dong, H., et al. (2014a). Identification of factors influencing the pharmacokinetics of voriconazole and the optimization of dosage regimens based on Monte Carlo simulation in patients with invasive fungal infections. J. Antimicrob. Chemother. 69, 463–470. doi:10.1093/jac/dkt369
Wang, T., Yan, M., Tang, D., Dong, Y., Zhu, L., Du, Q., et al. (2021). Using child-pugh class to optimize voriconazole dosage regimens and improve safety in patients with liver cirrhosis: insights from a population pharmacokinetic model-based analysis. Pharmacotherapy 41, 172–183. doi:10.1002/phar.2474
Wang, T., Yan, M., Tang, D., Xue, L., Zhang, T., Dong, Y., et al. (2018a). A retrospective, multicenter study of voriconazole trough concentrations and safety in patients with Child-Pugh class C cirrhosis. J. Clin. Pharm. Ther. 43, 849–854. doi:10.1111/jcpt.12724
Wang, T., Yan, M., Tang, D., Xue, L., Zhang, T., Dong, Y., et al. (2018b). Therapeutic drug monitoring and safety of voriconazole therapy in patients with child–pugh class B and C cirrhosis: a multicenter study. Int. J. Infect. Dis. 72, 49–54. doi:10.1016/j.ijid.2018.05.009
Wang, T., Zhu, H., Sun, J., Cheng, X., Xie, J., Dong, H., et al. (2014b). Efficacy and safety of voriconazole and CYP2C19 polymorphism for optimised dosage regimens in patients with invasive fungal infections. Int. J. Antimicrob. Agents 44, 436–442. doi:10.1016/j.ijantimicag.2014.07.013
Weiss, J., Ten Hoevel, M. M., Burhenne, J., Walter-Sack, I., Hoffmann, M. M., Rengelshausen, J., et al. (2009). CYP2C19 genotype is a major factor contributing to the highly variable pharmacokinetics of voriconazole. J. Clin. Pharmacol. 49, 196–204. doi:10.1177/0091270008327537
Wojnowski, L. (2004). Genetics of the variable expression of CYP3A in humans. Ther. Drug Monit. 26, 192–199. doi:10.1097/00007691-200404000-00019
Xing, Y., Chen, L., Feng, Y., Zhou, Y., Zhai, Y., and Lu, J. (2017). Meta-analysis of the safety of voriconazole in definitive, empirical, and prophylactic therapies for invasive fungal infections. BMC Infect. Dis. 17, 798. doi:10.1186/s12879-017-2913-8
Yamada, T., Imai, S., Koshizuka, Y., Tazawa, Y., Kagami, K., Tomiyama, N., et al. (2018). Necessity for a significant maintenance dosage reduction of voriconazole in patients with severe liver cirrhosis (Child-Pugh class C). Biol. Pharm. Bull. 41, 1112–1118. doi:10.1248/bpb.b18-00164
Yamazaki, H., Nakamoto, M., Shimizu, M., Murayama, N., and Niwa, T. (2010). Potential impact of cytochrome P450 3A5 in human liver on drug interactions with triazoles. Br. J. Clin. Pharmacol. 69, 593–597. doi:10.1111/j.1365-2125.2010.03656.x
Yang, L., Wang, C., Zhang, Y., Wang, Q., Qiu, Y., Li, S., et al. (2022). Central nervous system toxicity of voriconazole: risk factors and threshold - a retrospective cohort study. Infect. Drug Resist. 15, 7475–7484. doi:10.2147/IDR.S391022
Yi, W. M., Schoeppler, K. E., Jaeger, J., Mueller, S. W., MacLaren, R., Fish, D. N., et al. (2017). Voriconazole and posaconazole therapeutic drug monitoring: a retrospective study. Ann. Clin. Microbiol. Antimicrob. 16, 60. doi:10.1186/s12941-017-0235-8
You, H., Dong, Y., Zou, Y., Zhang, T., Lei, J., Chen, L., et al. (2018). Voriconazole therapeutic drug monitoring: factors associated with supratherapeutic and subtherapeutic voriconazole concentrations. Int. J. Clin. Pharmacol. Ther. 56, 239–246. doi:10.5414/CP203184
Zeng, G., Wang, L., Shi, L., Li, H., Zhu, M., Luo, J., et al. (2020). Variability of voriconazole concentrations in patients with hematopoietic stem cell transplantation and hematological malignancies: influence of loading dose, procalcitonin, and pregnane X receptor polymorphisms. Eur. J. Clin. Pharmacol. 76, 515–523. doi:10.1007/s00228-020-02831-1
Zhang, Y., Hou, K., Liu, F., Luo, X., He, S., Hu, L., et al. (2021). The influence of CYP2C19 polymorphisms on voriconazole trough concentrations: systematic review and meta-analysis. Mycoses 64, 860–873. doi:10.1111/myc.13293
Zhang, Y., Si, D., Chen, X., Lin, N., Guo, Y., Zhou, H., et al. (2007). Influence of CYP2C9 and CYP2C19 genetic polymorphisms on pharmacokinetics of gliclazide MR in Chinese subjects. Br. J. Clin. Pharmacol. 64, 67–74. doi:10.1111/j.1365-2125.2007.02846.x
Zhao, Q. G., Ren, Q. X., Du, C. H., and Wang, Y. H. (2019). Analysis of monitoring results of voriconazole plasma concentration in Child-Pugh class C patients. Drug Use Monit. China 16, 11–14. doi:10.3969/j.issn.1672-8157.2019.01.003
Zhao, Y., Hou, J., Xiao, Y., Wang, F., Zhang, B., Zhang, M., et al. (2021). Predictors of voriconazole trough concentrations in patients with child-pugh class C cirrhosis: a prospective study. Antibiot. Basel Switz. 10, 1130. doi:10.3390/antibiotics10091130
Zhou, Z.-X., Yin, X.-D., Zhang, Y., Shao, Q.-H., Mao, X.-Y., Hu, W.-J., et al. (2022). Antifungal drugs and drug-induced liver injury: a real-world study leveraging the FDA adverse event reporting system database. Front. Pharmacol. 13, 891336. doi:10.3389/fphar.2022.891336
Zonios, D. I., Gea-Banacloche, J., Childs, R., and Bennett, J. E. (2008). Hallucinations during voriconazole therapy. Clin. Infect. Dis. 47, e7–e10. doi:10.1086/588844
Zonios, D., Yamazaki, H., Murayama, N., Natarajan, V., Palmore, T., Childs, R., et al. (2014). Voriconazole metabolism, toxicity, and the effect of cytochrome P450 2C19 genotype. J. Infect. Dis. 209, 1941–1948. doi:10.1093/infdis/jiu017
Keywords: voriconazole, genetic polymorphism, hepatic insufficiency, CYP2C19, invasive fungal infections, dose adjustment
Citation: Li G, Li Q, Zhang C, Yu Q, Li Q, Zhou X, Yang R, Yang X, Liu H and Yang Y (2023) The impact of gene polymorphism and hepatic insufficiency on voriconazole dose adjustment in invasive fungal infection individuals. Front. Genet. 14:1242711. doi: 10.3389/fgene.2023.1242711
Received: 19 June 2023; Accepted: 10 August 2023;
Published: 24 August 2023.
Edited by:
Jian Gao, Shanghai Children’s Medical Center, ChinaReviewed by:
Chen Qi, Guizhou Provincial People’s Hospital, ChinaCopyright © 2023 Li, Li, Zhang, Yu, Li, Zhou, Yang, Yang, Liu and Yang. This is an open-access article distributed under the terms of the Creative Commons Attribution License (CC BY). The use, distribution or reproduction in other forums is permitted, provided the original author(s) and the copyright owner(s) are credited and that the original publication in this journal is cited, in accordance with accepted academic practice. No use, distribution or reproduction is permitted which does not comply with these terms.
*Correspondence: Hailin Liu, bGl1aGFpbGluYWFAMTI2LmNvbQ==; Yong Yang, eXl4cG93ZXJAMTYzLmNvbQ==
†These authors have contributed equally to this work and share first authorship
‡These authors have contributed equally to this work and share last authorship
Disclaimer: All claims expressed in this article are solely those of the authors and do not necessarily represent those of their affiliated organizations, or those of the publisher, the editors and the reviewers. Any product that may be evaluated in this article or claim that may be made by its manufacturer is not guaranteed or endorsed by the publisher.
Research integrity at Frontiers
Learn more about the work of our research integrity team to safeguard the quality of each article we publish.