- 1Molecular Plant Science, Washington State University, Pullman, WA, United States
- 2Department of Plant Pathology, Washington State University, Pullman, WA, United States
- 3Department of Horticultural Sciences, Texas A&M University, College Station, TX, United States
- 4Department of Agronomy, Horticulture and Plant Science, South Dakota State University, Brookings, SD, United States
- 5Grain Legume Genetics Physiology Research, Pullman, WA, United States
- 6Department of Crop and Soil Science, Washington State University, Pullman, WA, United States
Polygalacturonase-inhibiting proteins (PGIPs) are cell wall proteins that inhibit pathogen polygalacturonases (PGs). PGIPs, like other defense-related proteins, contain extracellular leucine-rich repeats (eLRRs), which are required for pathogen PG recognition. The importance of these PGIPs in plant defense has been well documented. This study focuses on chickpea (Cicer arietinum) PGIPs (CaPGIPs) owing to the limited information available on this important crop. This study identified two novel CaPGIPs (CaPGIP3 and CaPGIP4) and computationally characterized all four CaPGIPs in the gene family, including the previously reported CaPGIP1 and CaPGIP2. The findings suggest that CaPGIP1, CaPGIP3, and CaPGIP4 proteins possess N-terminal signal peptides, ten LRRs, theoretical molecular mass, and isoelectric points comparable to other legume PGIPs. Phylogenetic analysis and multiple sequence alignment revealed that the CaPGIP1, CaPGIP3, and CaPGIP4 amino acid sequences are similar to the other PGIPs reported in legumes. In addition, several cis-acting elements that are typical of pathogen response, tissue-specific activity, hormone response, and abiotic stress-related are present in the promoters of CaPGIP1, CaPGIP3, and CaPGIP4 genes. Localization experiments showed that CaPGIP1, CaPGIP3, and CaPGIP4 are located in the cell wall or membrane. Transcript levels of CaPGIP1, CaPGIP3, and CaPGIP4 genes analyzed at untreated conditions show varied expression patterns analogous to other defense-related gene families. Interestingly, CaPGIP2 lacked a signal peptide, more than half of the LRRs, and other characteristics of a typical PGIP and subcellular localization indicated it is not located in the cell wall or membrane. The study’s findings demonstrate CaPGIP1, CaPGIP3, and CaPGIP4’s similarity to other legume PGIPs and suggest they might possess the potential to combat chickpea pathogens.
1 Introduction
Plants deploy a variety of barriers to withstand numerous pathogenic stresses, one of which is the cell wall, a physical barrier that serves as the first line of defense. Pathogens produce enzymes known as cell wall-degrading enzymes (CWDEs) to overcome this plant barrier (Kubicek et al., 2014). Pectin-degrading enzymes called polygalacturonases are among the most important CWDEs. The middle lamella is the plant cell’s outermost layer that connects the primary cell walls of adjacent cells (Daher and Braybrook, 2015). Middle lamella is rich in pectin, which primarily constitutes homogalacturonan, a linear homopolymer of D-galacturonic acid monomers linked by a-(1–4) glycosidic linkage (Mohnen, 1999). Pectin determines the integrity and rigidity of plant tissue (Voragen et al., 2009), and degrading pectin enables quick access to the components within the cell. By breaking down glycosidic linkages between D-galacturonic acid residues, PGs degrade homogalacturonan and subsequently pectin causing cell separation and maceration of the host tissue (Kalunke et al., 2015; Mojsov, 2016). Polygalacturonases (PG) are secreted at the early stages of infection (De Lorenzo et al., 2001). In defense, plants use polygalacturonase inhibiting proteins (PGIPs) to impede PGs’ pectin-depolymerizing activity. Plant PGIPs are located on the cell wall and their potential to suppress PG activity is correlated with plant disease resistance (Ge et al., 2019).
PGIPs are highly conserved proteins (Di Matteoet al., 2003). So far, PGIPs have been reported in every characterized plant species or mutant (Kalunke et al., 2015). Most PGIPs are generally intronless, except a few that include a short intron (Kalunke et al., 2015). PGIPs, like many other resistance gene products, contain extracellular type leucine-rich repeats (eLRRs) (Di Matteo et al., 2003; Kalunke et al., 2014). PGIPs are composed of 10 incomplete LRRs of approximately 24 residues each, which are arranged into two ß-sheets. β1 occupies the inner concave side of the molecules, while β2 occupies the outer convex side. These repeats form ß-sheet/β-turn/α-helix containing LRR motifs. Motifs that occupy the β1 inner concave side are critical for interaction with PGs.
Albersheim and Anderson, 1971 were the first to report PGIP gene activity in 1971. The first PGIP gene, however, was isolated in French beans 20 years later (Toubart et al., 1992). Several PGIP genes have been identified in several crops based on sequence identity since 1971. Except for some members belonging to Brassicaceae (Hegedus et al., 2008), most PGIP genes do not undergo large expansion and may exist as single gene per genome (Di Giovanni et al., 2008), or clustered into small gene families (Ferrari et al., 2003). In legumes, PGIP genes have been characterized in Glycine max, Medicago sativa, Medicago truncatula, Phaseolus acutifolius, Phaseolus coccineus, Phaseolus lunatus, Phaseolus vulgaris, Pisum sativum, and Vigna radiata (Veronico et al., 2011; Gao and Gui, 2015; Kalunke et al., 2015; Matsaunyane et al., 2015; Kaewwongwal et al., 2017). However, only M. sativa, M. truncatula, V. radiata, P. vulgaris, and G. max’s genome have more than one PGIP gene (Kalunke et al., 2015; Wang et al., 2022).
Pathogen PG inhibition by PGIPs is well established. PGIPs like other defense molecules can be used against pathogens and pests (Hamera et al., 2014; Zhou et al., 2017). The majority of the identified legume PGIPs inhibited fungal infections, such as G. max’s GmPGIP7 (D'Ovidio et al., 2006; Frati et al., 2006; Kalunke et al., 2014), M. truncatula’s MtPGIP1, MtPGIP2 (Song and Nam, 2005), P. vulgaris’s PvPGIP1, PvPGIP 2, PvPGIP3, PvPGIP 4 (Desiderio et al., 1997; D'Ovidio et al., 2006; Frati et al., 2006), P. acutifolius’s PaPGIP2, P. coccineus’s PcPGIP2, P. lunatus’s PlPGIP2 (Farina et al., 2009), and Brassica napus, BnPGIPs (Wang et al., 2021). However, PGIPs from V. radiata, VrPGIP1, and VrPGIP2 (Kaewwongwal et al., 2017; Zhang et al., 2021) and Brassica rapa ssp. pekinensis BrPGIPs (Haeger et al., 2021) have shown to inhibit insects and P. sativum PsPGIP inhibited nematodes (Veronico et al., 2011).
Some PGIP genes are expressed in untreated conditions when plants are not stressed, while others respond to external cues. Pathogens and pests such as fungi, oomycetes, insects, and nematodes are known to induce PGIP gene expression, as are phytohormones such as abscisic acid (ABA), indole-3-acetic acid (IAA), salicylic acid (SA), and jasmonic acid (JA) (Ferrari et al., 2003; Hwang et al., 2010; Hou et al., 2015). PGIP gene expression is also triggered by wounding and oligogalacturonic acid treatments (Ferrari et al., 2003; Di Matteo et al., 2006). PGIP genes/gene families expression is tissue-specific and developmentally regulated (Li and Smigocki, 2016), studies conducted with basal transcript levels of B. napus PGIPs (Hegedus, et al., 2008), P. vulgaris PGIPs (Kalunke et al., 2011), and C. papaya PGIPs (Broetto et al., 2015) indicate PGIPs are expressed in untreated conditions when plants are not stressed.
Currently eighteen PGIPs have been either computationally or biochemically characterized from nine legume species, but major legumes such as chickpeas, peanuts, and lentil PGIPs remain uninvestigated. This study focuses on PGIPs of chickpeas because chickpeas are the world’s second most widely produced and consumed leguminous crop, chickpeas have a high protein content (up to 40% protein by weight), are an excellent source of essential vitamins such as riboflavin, niacin, thiamin, folate, and the vitamin A precursor ß-carotene, and have other potential health benefits such as lowering cardiovascular, diabetic, and cancer risks (Jukanti et al., 2012; Sharma et al., 2013; Merga and Haji, 2019). Previous publications only report that the chickpea genome harbors two PGIPs (CaPGIP1 and CaPGIP2) on chromosome 6 (Kalunke et al., 2014; Kalunke et al., 2015). Therefore the goal of this study is to investigate PGIPs in chickpeas to gain a better understanding of their structural features, functional domains, regulatory elements, and genomic organization. CaPGIP genes were cloned, and their sequence features were evaluated in this study. The basal expression of all CaPGIPs was explored. Our findings revealed that CaPGIPs, like other legume PGIPs, had similar characteristics and can play an essential role in plant resistance against pathogens and pests.
2 Materials and methods
2.1 Sequence acquisition, phylogeny, and bioinformatics analysis
To identify PGIP homologs in the chickpea genome, a homology search was performed against the NCBI database using the amino acid sequences of previously known legume PGIPs. SignalP 5.0 was used to identify the presence of signal peptides in the candidate genes identified by the NCBI homology search (Armenteros et al., 2019). The molecular weight and isoelectric point (pI) were determined using ExPASy Server (Gasteiger et al., 2005). NetNGlyc version 1.0 server was used to analyze the putative N-linked glycosylation sites (Gupta & Brunak, 2002). The Swiss-Model server was used to build homology-based 3D models of CaPGIPs (Waterhouse et al., 2018). Protein sequences were aligned using ClustalW through the MEGA X program (Kumar et al., 2018). Jalview was used for multiple sequence alignment with a conservation index of 50% (Waterhouse et al., 2009). A phylogenetic tree was generated using MEGA X (Kumar et al., 2018) with the neighbor-joining phylogenetic statistical method, Poisson model and other settings retained at default. The tree was bootstrapped 1000 times for robustness and Cucumis sativus PGIPs (CsPGIPs) were used as outgroup. MEGA X generated trees were visualized using the iTOL version 6.1.1 online tool (Letunic and Bork., 2021). The 1,500 bp upstream sequence for all CaPGIP sequences was analyzed for the putative cis-acting regulatory DNA elements using New PLACE (Higo et al., 1999).
2.2 Plant materials
Chickpea (Cicer arietinum) cultivar Dwelley was grown in greenhouse conditions. Plants maintained in the greenhouse at 22°C ± 2°C. Leaf, stem, root, flower, pod, and seed tissues were collected at different chickpea growth stages, which are mentioned in Table 1. Tissue samples (100 mg) were collected in three biological replicates and were immediately snap-frozen in liquid nitrogen and stored at −80°C.
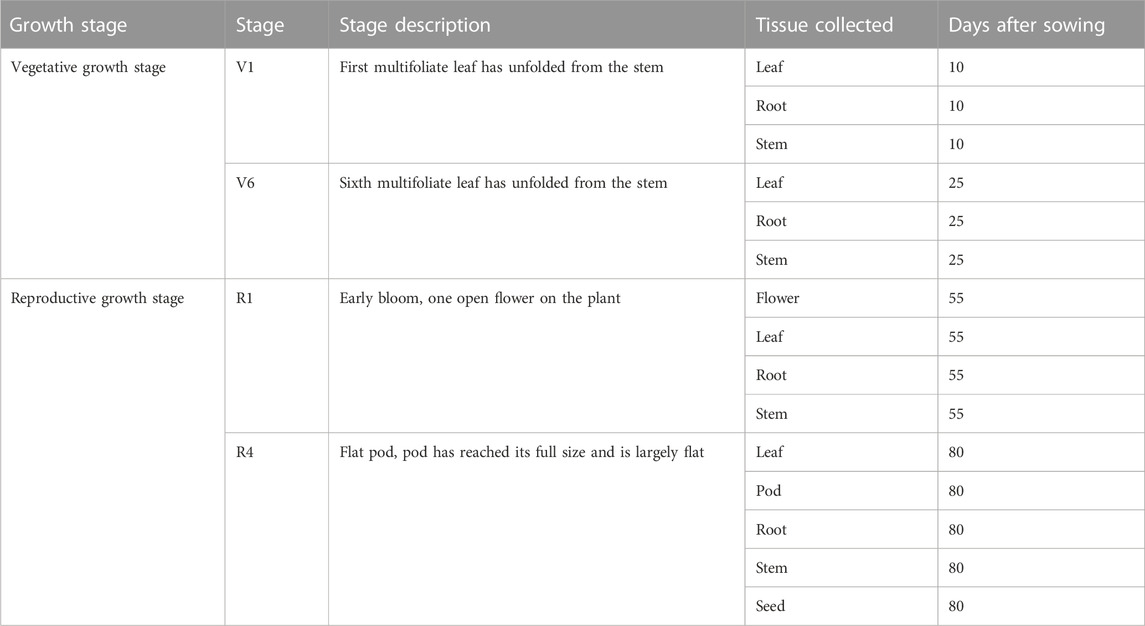
TABLE 1. Chickpea tissues collected during different chickpea growth stages for absolute gene expression quantification.
2.3 Cloning and sequencing
The total RNA was extracted from the leaves of chickpea cultivar Dwelley using the RNeasy Plant Mini Kit (Qiagen, Hilden, Germany) from 100 mg samples in accordance with the manufacturer’s protocol. First strand cDNA synthesis and genomic DNA elimination were performed simultaneously using 5X All-In-One RT MasterMix, containing AccurT Genomic DNA Removal (Applied Biological Materials Inc., Richmond, Canada). cDNA samples were stored at−80 °C until use. Full-length ORFs were amplified with Phusion® High-Fidelity DNA Polymerase (NEB, Ipswich, MA, United States) using gene-specific primer pairs (Supplementary Table S1) using the following protocol: initial denaturation at 98°C for 30 s and 35 cycles of 98°C for 10 s, 60°C and 72°C for 30 s each and followed by a final elongation at 72°C for 8 min. Amplified PCR products with appropriate expected sizes were purified with the Monarch® DNA Gel Extraction Kit (NEB). Purified PCR products were cloned into the pMiniT 2.0 vector (NEB) and transformed into DH10B high-efficiency E. coli competent cells (NEB). The plasmids were recovered from E. coli using PureYield™ Plasmid Miniprep (Promega, Madison, WI, United States), verified using Sanger sequencing (Laboratory of Biotechnology & Bioanalysis, Pullman, WA, United States), and compared to the GenBank sequences of CaPGIP1 (XM_004504675), CaPGIP3 (XM_004493500), and CaPGIP4 (XM_012713804).
2.4 RNA isolation, cDNA conversion and quantitative real-time-PCR
To determine the absolute expression of CaPGIP genes in untreated conditions, total RNA was extracted from various chickpea tissues from the cultivar Dwelley at different growth stages (Table 1). Based on the timing of infection by the major chickpea fungal pathogens, four growth stages (V1, V6, R1, and R4) were selected (Mazur et al., 2002; West et al., 2003; Bretag and Horsham, 2004; Markel et al., 2008; Jiménez-Fernández et al., 2011; Moore et al., 2011; Khan et al., 2012; Wunsch, 2014; Knights and Hobson, 2016). RNA was extracted from 100 mg samples using the RNeasy Plant Mini Kit (Qiagen) according to the manufacturer’s protocol. First-strand cDNA synthesis and genomic DNA elimination were performed simultaneously using 5X All-In-One RT MasterMix, containing AccurT Genomic DNA Removal (Applied Biological Materials, Inc.). To preserve sample integrity, RNA extraction, genomic DNA removal, and cDNA synthesis were performed on the same day. Samples were stored at −80°C until use. Using Primer3Plus (Untergasser et al., 2007) at the default parameters, quantitative PCR primers (Supplementary Table S1) were generated based on the sequences of CaPGIP1, CaPGIP3, and CaPGIP4 and the reference gene 18SrRNA and 25SrRNA. The NCBI Primer-BLAST (ncbi.nlm.nih.gov/tools/primer-blast/) program was used to ensure that primers are unique specifically to the corresponding gene. Primers were referenced against the chickpea genome ASM33114v1. Standard curves generated by serial dilution of cDNA for 18SrRNA, 25SrRNA, CaPGIP1, CaPGIP3, and CaPGIP4 were used to evaluate primer efficiency (Supplementary Figure S1). Transcript levels of chickpea PGIPs (CaPGIP1, CaPGIP3, and CaPGIP4) in chickpea at untreated conditions were evaluated following the Minimum Information for Publication of Quantitative Real-Time PCR Experiments (MIQE) guidelines (Bustin et al., 2009). Each RT-qPCR reaction consisted of 1x SsoAdvancedTM universal Inhibitor-Tolerant SYBR® Green Supermix (Bio-Rad, Hercules, CA, United States), 2.5 μM of each gene-specific primer, and cDNA converted from 100 ng RNA in a final reaction volume of 10 μL. No template control (NTC), no amplification control (NAC), and negative reverse transcription (NRT) controls were included for each primer pair, and all reactions were performed with three separate biological replicates in technical triplicates. qPCR was carried out in the CFX96TM Real-Time PCR Detection System using a two-step amplification and melt curve method with the following protocol: 95°C for 3 min, followed by 40 cycles of 95°C for 10 s; 60°C for 30 s; and 72°C for 30 s. Melt curve readings were taken from 65.0°C to 95.0°C with an increment of 0.5°C every 5 s. The absolute gene expression assays were performed by constructing standard curves of the corresponding cloned coding region of CaPGIPs (Wong and Medrano, 2005). The expression Ct values of CaPGIPs were normalized against the expression Ct values of reference genes 18SrRNA and 25SrRNA. Quantification was done using the relative standard curve method (Supplementary Figure S1) (Pfaffl, 2001). The CaPGIP expression values are given as the mean of the normalized expression values of CaPGIPs normalized against reference genes 18SrRNA and 25SrRNA. Obtained CaPGIP data is shown as gene copy number/microgram of RNA (Forlenza et al., 2012). The statistical significance was determined by one-way analysis of variance (ANOVA) followed by Tukey’s ad hoc testing using the PROC GLM program in Statistical Analysis System (SAS) version 9.4 (SAS Institute Inc, 2013).
2.5 Subcellular localization
DeepLoc-1.0 (Almagro Armenteroset al., 2017) was used to predict subcellular localization based on CaPGIP protein sequences. The complete coding sequences (CDS) of CaPGIP1, CaPGIP2, CaPGIP3, and CaPGIP4 genes were cloned into pEarleyGate 103 (ABRC) using the gateway cloning approach to determine their subcellular localization. After sequencing validation, these gateway plant expression vectors were transformed into Agrobacterium tumefaciens strain EHA105. Using a blunt syringe, transformed EHA105 cultures harboring CaPGIP-mGFP plasmids were infiltrated into 4-week-old N. benthamiana leaves, two biological replicates along with two technical replicates per gene were infiltrated and imaged. A laser scanning confocal microscope (Leica SP-8) was used to examine and capture the fluorescence emitted by fusion proteins 72 h after infiltration. GFP fluorescence was excited at 488 nm.
3 Results
3.1 Insilco characterization of CaPGIPs
CaPGIP1 (LOC101505245) and CaPGIP2 (LOC101504619) are two previously reported chickpea PGIPs (Kalunke et al., 2014; Kalunke et al., 2015). They occupy a 30,150 bp region on chromosome 6. CaPGIP1 has a single exon with no intron as seen in several legume PGIPs. While CaPGIP2 has two exons separated by a 9,825 bp intron. A homology search against the NCBI chickpea genome assembly ASM33114v1 using the amino acid sequences of known legume PGIPs revealed the presence of additional five candidate PGIP sequences (Supplementary Table S2). Only two of them, LOC101499240 and LOC101499557, were suitable for designation as prospective PGIPs since they were the appropriate size, had a signal peptide, and had ten LRR sequences. They were named CaPGIP3 and CaPGIP4 respectively. CaPGIP3 and CaPGIP4 are composed of a single exon with no introns and span a 15,582 bp region on chromosome 3. In addition to the previously known locus of PGIP genes (Kalunke et al., 2014; Kalunke et al., 2015), our analysis identifies a new locus. The occurrence of two PGIP loci in chickpeas, chromosome 3 and chromosome 6, necessitates a new genomic organization of chickpea PGIP genes (Figure 1). Full-length cDNA size (bp), ORF size (bp), predicted protein size (aa), predicted signal peptide size (aa), theoretical molecular mass (kDa), and pI for all four CaPGIPs are presented in Table 2.
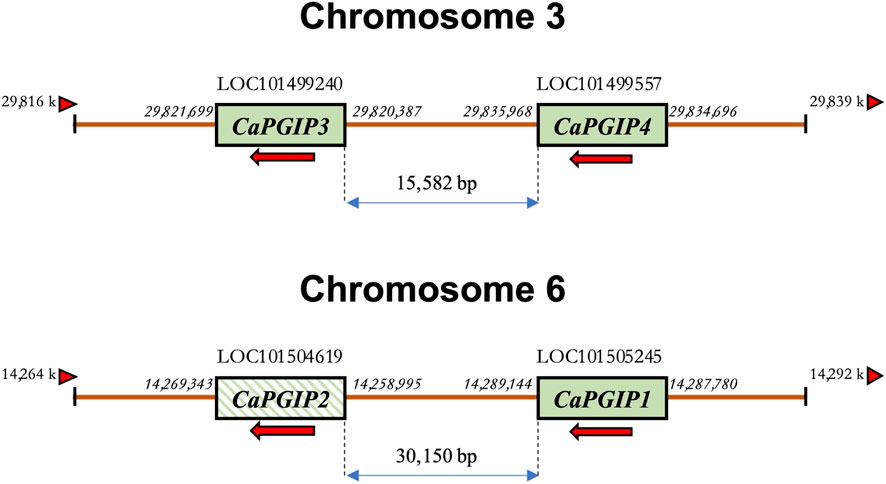
FIGURE 1. Schematic representation of the revised genomic organization of the PGIP gene family in Cicer arietinum showing two loci for CaPGIPs, chromosomes 3 and 6. The numbers on the side of genes represent their start and stop codon location on the genome. The numbers between the genes denote distances in base pairs. Arrows indicate the transcriptional direction of coding regions. The arrowhead triangles indicate the orientation/direction of the DNA strand on which the genes are placed. Crossed box for CaPGIP2 indicates the presence of an intron within the gene.

TABLE 2. NCBI accession number, full-length cDNA size (bp), ORF size (bp), predicted protein size (aa), predicted signal peptide size (aa), theoretical molecular mass (kDa), and pI for all four CaPGIPs.
Sequence analysis was conducted for all four CaPGIPs. Predicted proteins of CaPGIP1, CaPGIP3, and CaPGIP4 exhibited a typical PGIP sequence identity. SignalP 5.0 (Armenteros et al., 2017) projected 36, 22, and 20 amino acid signal peptides for CaPGIP1, CaPGIP3, and CaPGIP4, respectively (Table 2). As illustrated in Figure 2, the predicted mature protein sequences for CaPGIP1, CaPGIP3, and CaPGIP4 featured an N-terminal domain, a central LRR domain, and a C-terminal domain. These tandemly repeated LRRs fold into a characteristic curved and elongated PGIP shape. As observed in homology 3D models generated using PvPGIP2 as a template for CaPGIP1, CaPGIP3, and CaPGIP4 (Figure 3), the secondary and tertiary structures of CaPGIP1, CaPGIP3, and CaPGIP4 indicate that all 10 LRRs contain an ß-turn motif (xxLxLxx) that folds into β1 sheets. β1 sheets occupy the PGIP scaffold’s inner concave face, which is the site for PG interaction. Aside from that, all LRRs have β2 sheets and 310-helixes. PGIPs are glycoproteins with N-glycosylation sites ((N–x–S/T; where N is asparagine, x can be any amino acid except proline (P), S is serine, and T is threonine). As a result, the NetNGlyc version 1.0 server predicted five, three, and eight N-glycosylation sites in CaPGIP1, CaPGIP3, and CaPGIP4 proteins, respectively. CaPGIPs contain several conserved cysteine residues. CaPGIP1 has eight, CaPGIP3 has ten, and CaPGIP4 has nine cysteine residues.
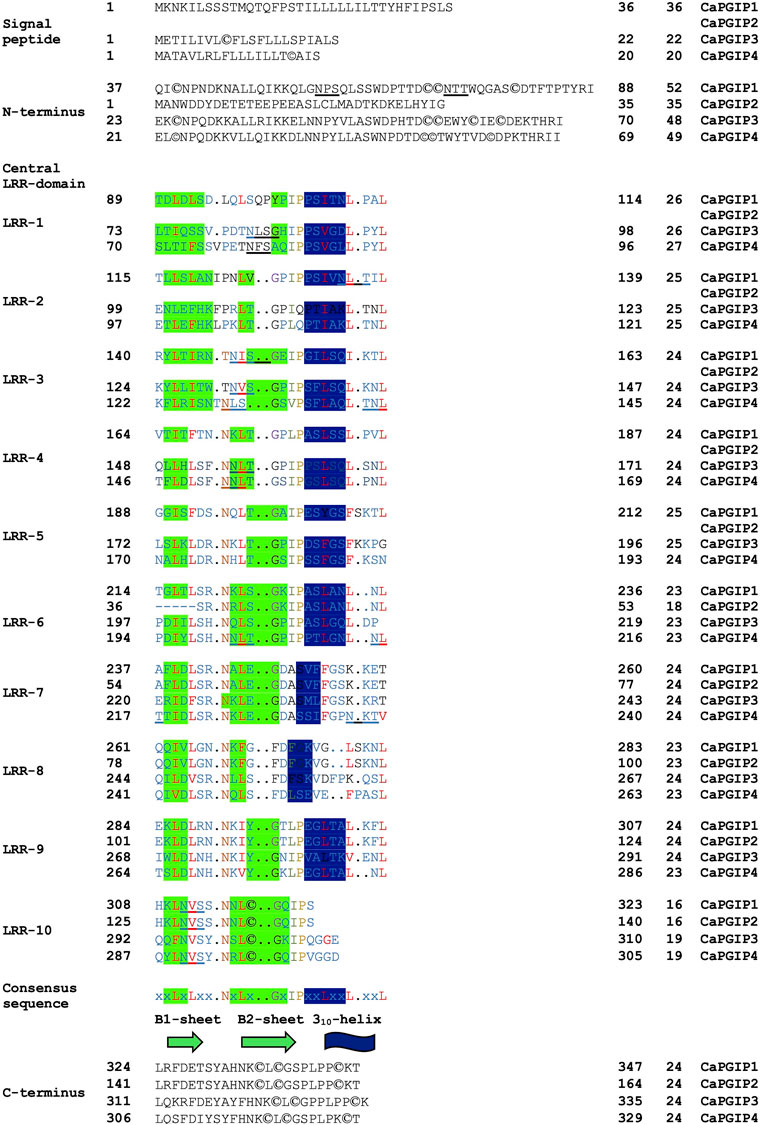
FIGURE 2. Translated structure of CaPGIP1,CaPGIP2,and CaPGIP4 based on PvPGIP2.A)Signal peptide,B)N-terminal domain,C)central LRK domain and D) C-terminal domains are indicated.Secondary structure elements (sheet B1,B2,and 310-helix) are highlighted.Five N-glycosylation sites (N-X-S/T) are underlined,cysteine residues are encircled.
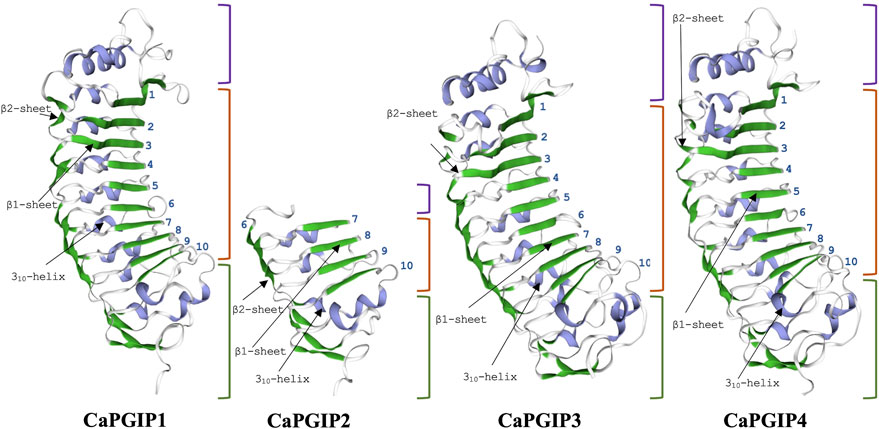
FIGURE 3. Homology 3D model of CaPGP1,CaPGIP2, CaPGIP3 and CaPGIP4 using PvPGIP2 as a template,b1, and b2 sheets are indicated by green color.310-helixes are indicated with purple color. purple-colored brackets indicate N-terminal domains,orange-colored brackets indicate central LRR domain with LRR numbers marked and gren-colored brackets indicate C-terminal domain.
Interestingly, CaPGIP2 (Figures 2, 3), on the contrary, lacked many of the above sequence identities. The absence of signal peptide suggested it is not a secretory protein. Its secondary structure reveals that it lacked more than half of the LRR modules, with just the 6th to 10th LRRs. This short LRR sequence on CaPGIP2’s C-terminal perfectly matches CaPGIP1’s C-terminal. The CaPGIP2 homology 3D model indicated the absence of the distinctive concave face that harbors PG interaction sites (Figure 3). CaPGIP2 has one N-glycosylation site and five cysteine residues, fewer than its counterparts.
3.2 Sequence comparison and phylogenetic analysis of theCaPGIP proteins
Multiple sequence alignment showed that CaPGIP amino acid sequences are highly similar to those of other legumes such as soybean, common bean, runner bean, tepary bean, lima bean, barrel clover, peas, mung bean, and alfalfa, with the presence of five conserved cysteine residues shared by all. The higher similarity was observed in the β2-sheet regions, along with variable portions present in both ß-sheets, as evident in plant-specific LRR proteins (Figure 4). This sequence alignment demonstrates that PGIPs are highly conserved within the legume family.
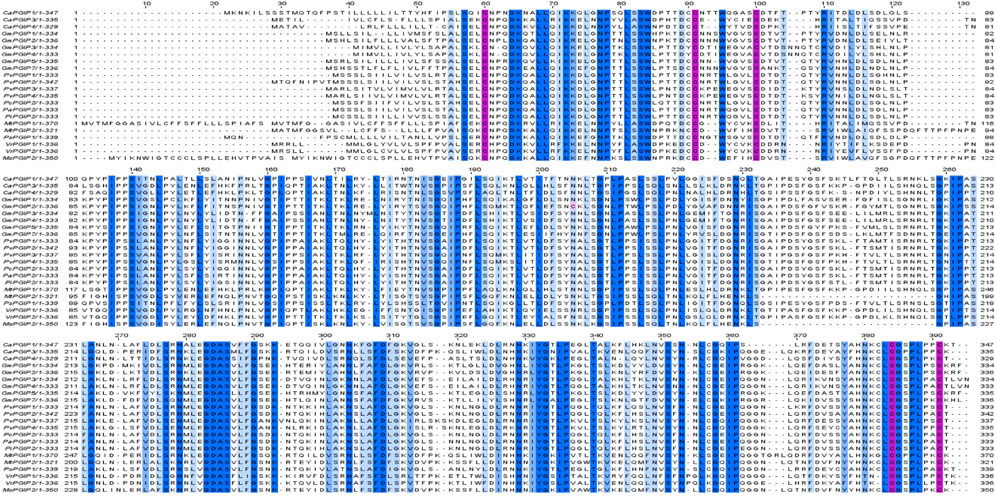
FIGURE 4. Multiple sequence alignment of chickpeas PGIPs[Capgip1(XP_004504732.1),Capgip3(XP_004493557.1),Capgip4(XP_012569258.1)}], with other legume PGIPs such as soybean [GmPGIP1(XP_0035224772.1),GmPGIP2(CAI99393.1), GmPGIP3 (NP_001304551.2), Gm PGIP4(CAI99395.1),GMPGIP5(XP_003524769.1),GmPGIP7(XP_003531070.1)], common bean [PvPGIP1(CAI11357.1),PvPGIP2(P58822.10,PvPGIP3(CAI11359.1),PvPGIP4(CAI11360.1)], runner bean [PcPGIP2(CAR92534.1)], tepary bean[PAPGIP2(CAR92533.1)], lima bean [PiPGIP2(CAR92537.1)], barrel clover [MtPGIP1(XP_003625218.1),MtPGIP2(XP_024626259.1)], peas [PsPGIP1(AJ749705.1)]mung bean[VrPGIP1(ATN23902.1),VrPGIP2(ATN23895.1), and alfalfa[MsPGIP2(ALX18673.1)]Blue color bar indicates conservation more than 50%, brighter the bar more the conservation, pink color bar indicates cysteine residues.
In the phylogenetic analysis (Figure 5), CaPGIPs were compared to 45 other known PGIPs from various crop families. The tree (Figure 5) comprises five main branches that are separated into monocots and dicots. The Poaceae family is represented by one cluster, the majority of the legume PGIPs are represented by a second cluster, three PGIPs from Beta vulgaris are represented by a third cluster. CaPGIP3, and PGIPs from V. radiata are represented by a fourth cluster, and the remaining PGIPs from other families, such as Actinidiaceae, Apocynaceae, Brassicaceae, Caricaceae, Cucurbitaceae, and Malvaceae, form the final cluster. CaPGIP1 and CaPGIP2 are members of the Leguminosae cluster and exhibit significant similarities to pea PGIP, PsPGIP1. CaPGIP3 and CaPGIP4 are outside the Leguminosae cluster, with CaPGIP3 sharing a high degree of similarity with its other legume counterparts, V. radiata PGIPs, VrPGIP1, and VrPGIP2. CaPGIP4 is in a separate cluster that includes PGIPs from various plant families.
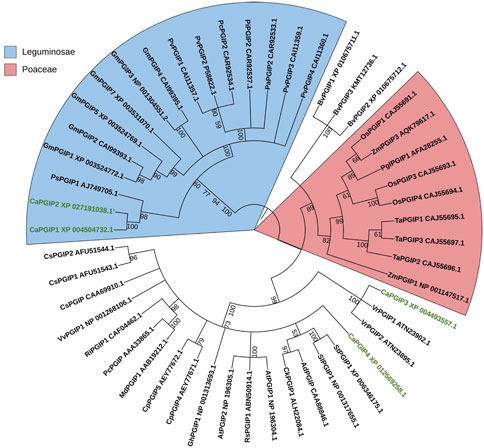
FIGURE 5. Phylogenetic tree illustrating the relationship between several PGIPs from various crop families and CaPGIPs.CaPGIPs are highlighted in green the values on the branches correspond to bootstrap values. Cucumis sativus PGIPs(CsPGIPs) are used as outgroup.
3.3 Promoter analysis of CaPGIPs
To locate regulatory DNA elements, the 1,500 bp upstream sequence for all CaPGIP genes was analyzed. The TATA box and CAAT box motifs were discovered close to the start codons. CAAT and TATA box sequences were found at −40 and −229 upstream of CaPGIP1, respectively, and at −23 and −55 upstream of CaPGIP3. The TATA box was located at a −30 position in the CaPGIP4 upstream sequence, whereas CAAT was at a −37 position. Elements/motifs associated with plant responses to hormones such as abscisic acid, gibberellic acid, jasmonic acid, and salicylic acid were also identified. Motifs for wounding response were identified as well. Crucially, numerous elements associated with pathogenicity responses were identified in the promoter regions of the CaPGIPs. Table 3 lists these putative cis-acting regulatory elements, as well as their locations and roles. Aside from the motifs mentioned above, additional cis-elements known to mediate tissue-specific activity and plant physiological processes were identified. Stress-related cis-acting regulatory elements associated with drought, dehydration, water, high light, and low-temperature stress, were also identified. All these elements are listed in Supplementary Table S3.
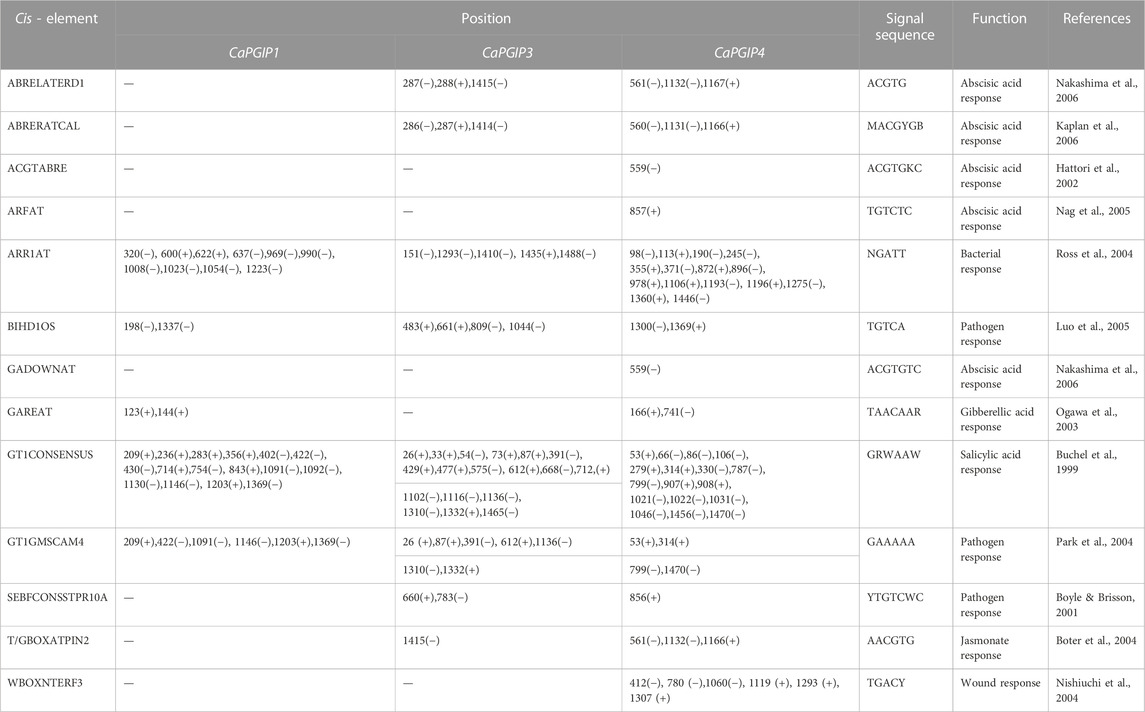
TABLE 3. Putative hormonal and pathogenesis related cis-acting regulatory elements identified in the promoter regions of CaPGIPs.
3.4 Cloning and characterization of CaPGIPs
CaPGIP genes were cloned and sequenced from the chickpea cultivar “Dwelley”. CaPGIP1 sequence matched GenBank sequence XM 004504675. However, a C was replaced by a T at the 720th nucleotide position, which was a synonymous substitution with no change in the coded amino acid. CaPGIP3 sequence matched the GenBank sequence XM 004493500, and CaPGIP4 sequence matched the GenBank sequence XM 012713804. Transcripts for CaPGIP2 could not be amplified even with different sets of primers; hence, all subsequent investigations focused on CaPGIP1, CaPGIP3, and CaPGIP4.
3.5 Subcellular localization of CaPGIPs
DeepLoc-1.0 uses sequencing information to predict the subcellular localization of plant proteins. Based on the presence of signal peptides, it was inferred thatCaPGIP1, CaPGIP3, and CaPGIP4 were secretory and classified as extracellular proteins. CaPGIP2, on the other hand, was predicted to be found in the mitochondrion and cytoplasm. To validate those predictions, Agrobacterium cells carrying binary vectors of CaPGIPs and GFP fusions were infiltrated into N. benthamiana leaves for transient expression of encoded proteins in leaf mesophyll and epidermal cells. According to the excitation curves, the fluorescence of CaPGIPs-GFP fusion proteins was the same as GFP. CaPGIP1, CaPGIP3, and CaPGIP4 fluorescence was visible on the cell boundaries (Figure 6). As a result, they were most likely found on the cell wall or plasma membrane. CaPGIP2-GFP fluorescence was seen inside the cell in the cytoplasm and endoplasmic reticulum, and CaPGIP2 was most likely found in the cytoplasm and endoplasmic reticulum (Figure 6).
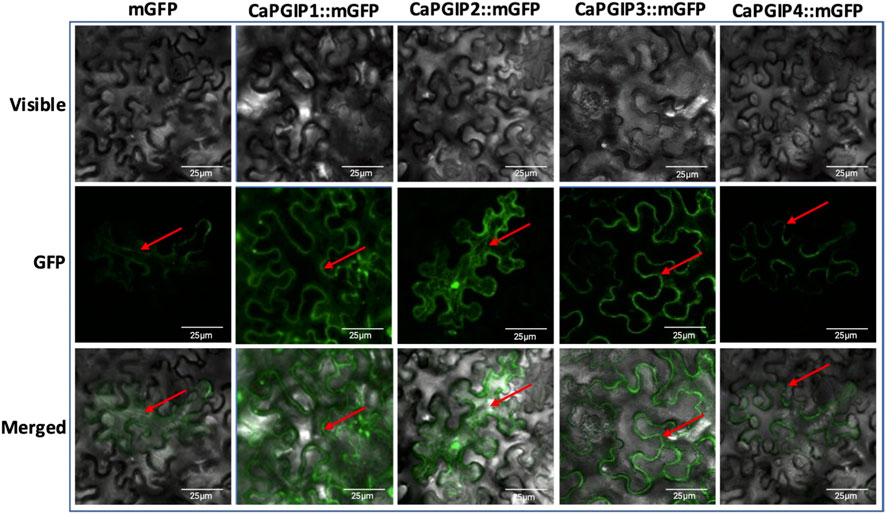
FIGURE 6. Subcellular localization of Capgips. Full-length Capgips fused with a green fluorescent protein (GFP) are transiently expressed in Nicotiana benthamiana leaves by agroinfiltration. The images show the fluorescence emitted by fusion proteins was captured 72 h after infiltartion using a laser scanning confocal microscope as mGFP fluorescence in green color, visible light in brightfield images, merged as merged images of mGFP and visible. Cells transformed with mGFP is the control. Two biological replicates along with two technical replicates per gene were infiltrated and imaged. Red arrows indicate localization.
3.6 Absolute quantification of CaPGIP’s transcripts
CaPGIP transcript levels were investigated at four growth stages using the indeterminate type of Kabuli chickpea variety Dwelley, which matures in 110–120 days. RT-qPCR was utilized to determine the absolute CaPGIPs expression levels (Table 1). Transcripts for CaPGIP1, CaPGIP3, and CaPGIP4 were ubiquitously detected in all the studied tissues. In the vegetative stages V1 and V6 stages were investigated (Figure 7). CaPGIP1, CaPGIP3, and CaPGIP4 transcript levels were higher in the V1 leaf compared to the root and stem. In V1 leaf CaPGIP4 expressed significantly higher, followed by CaPGIP3, then CaPGIP1. For stem in the V1 stage, similar to leaf CaPGIP4 expressed significantly higher, followed by CaPGIP3, then CaPGIP1. Roots in the V1 stage had a different expression pattern, where CaPGIP3 expressed significantly higher, followed by CaPGIP1, then CaPGIP4. CaPGIPs expression was lower in the V6 vegetative stage compared to V1. In contrast to V1, the transcript levels of CaPGIP1, CaPGIP3, and CaPGIP4 were higher in the V6 stem compared to the leaf and root. In V6 stem CaPGIP4 expressed significantly higher, followed by CaPGIP3, then CaPGIP1. For roots in the V6 stage, CaPGIP4 and CaPGIP3 expression levels were statistically similar and higher than CaPGIP1. For V6 leaves, CaPGIP1 and CaPGIP3 expression was statistically similar and lower than CaPGIP4.
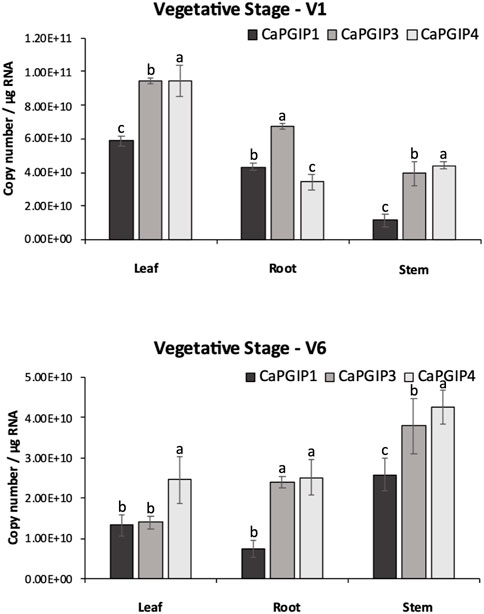
FIGURE 7. Absolute expression of Capgips. The number of transcript copies of Capgip1,Capgip3, Capgip4 was calculated in the leaf, stem, and root at vegetative growth stages V1 and V6. The abundance was normalized by the amount of internal control 18 SrRNA and 25 rRNA.The values are the means of three biological replicates each with three technical replicates. Error bars indicate standard deviation among the biological replicates (N = 3). Different letters indicate significant differences by ANOVA with Tukeys ad hoc test(p < 0.05)
R1 and R4 were investigated in the reproductive stages, and the transcript levels were analyzed for flower, pod, and seed along with leaf, stem, and root (Figure 8). All CaPGIPs in leaves showed higher expression levels in the R1 stage compared to other tissues. In the R1 leaf, CaPGIP3 expressed significantly higher, followed by CaPGIP4, then CaPGIP1. CaPGIP1 and CaPGIP4 expression levels were statistically similar and lower than CaPGIP3 in the R1 root. For stem and flowers in R1, CaPGIP4 had a higher expression, followed by CaPGIP3 and then CaPGIP1. Similar to the R1 stage, all CaPGIPs in R4 leaves expressed at higher levels than other tissues. In the R4 leaf, CaPGIP4 expressed significantly higher, followed by CaPGIP3, then CaPGIP1. In R4 roots, CaPGIP3 expressed significantly higher, followed by CaPGIP1, then CaPGIP1. For the R4 stem, CaPGIP1 and CaPGIP3 expression levels were statistically similar and lower than CaPGIP4. For pods and seeds in R4, CaPGIP4 had a higher expression, followed by CaPGIP3 and then CaPGIP1. Overall, the highest expression levels for all CaPGIPs were found in the leaves. Additionally, CaPGIP4 showed the highest expression among the three genes, with levels about one and a half times higher than CaPGIP3 and double the expression of CaPGIP1.
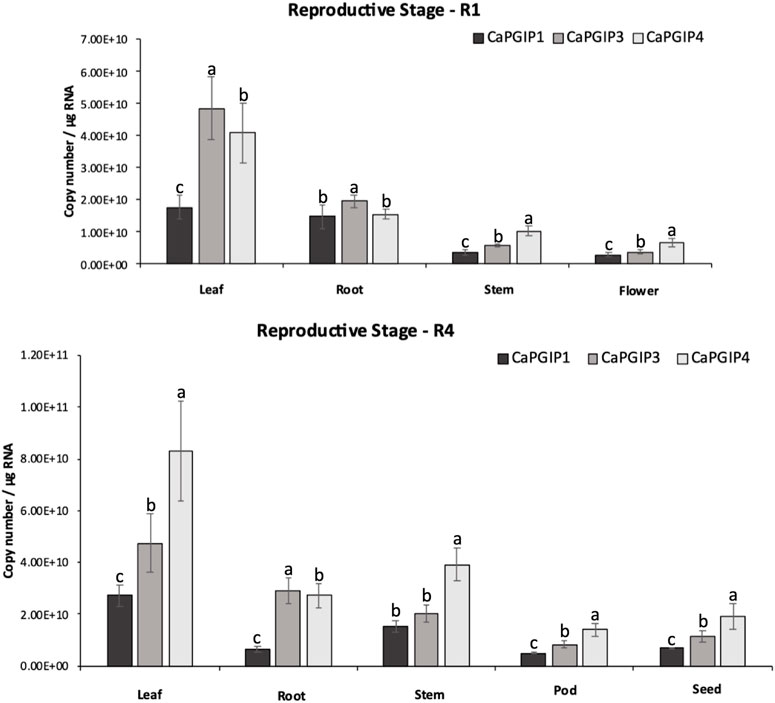
FIGURE 8. Absolute expression of Capgips. The numbers of transcript copies of Capgip1,Capgip3, and Capgip4 were calculated in the leaf, stem, root and flower at reproductive growth stage R1, and leaf, stem,roor, pod and seed at reproductive growth stage R4. The abundance was normalized by the amount of internal control 18 SrRNA and 25 rRNA. The value are the means of three biological replicates each with three technical replicates. Error bars indicate standard deviation among the biological replicates (N = 3) Different letters indicate significant differences by ANOVA with Tukeys ad hoc test (p < 0.005).
4 Discussion
Several plant species have PGIP-encoding small gene families; these multigene families encode proteins with similar LRR domains but different PG-inhibitory capabilities (D’Ovidio et al., 2006). This study demonstrated that the chickpea genome has a PGIP family of potentially three functional genes (CaPGIP1, CaPGIP3, and CaPGIP4) that are present on two chromosomes. Soybean is the only other legume where PGIPs are located on multiple chromosome (D’Ovidio et al., 2006). Like the genomic distribution of CaPGIPs, legume PGIPs are distributed across a broader genomic region, as observed in soybean, where GmPGIP1, GmPGIP2, and GmPGIP5 are located on chromosome 5, and span a ∼ 19.5 kbp region, while GmPGIP3, GmPGIP4, and GmPGIP7, present on chromosome 8, span a ∼ 21 kbp region. In common bean, PvPGIP1, PvPGIP2, PvPGIP3, and PvPGIP4 span an area of ∼ 50 kbp on chromosome 2 (D’Ovidio et al., 2004; D’Ovidio et al., 2006).
So far, over eighteen legume PGIPs have been either computationally or biochemically characterized in 9 species. The reported sequence lengths of these legume PGIPs ranged from 321 amino acids for MtPGIP2 to 342 amino acids for MtPGIP1. All these PGIPs contain signal peptides, with GmPGIP4 having the shortest with 17 amino acids and PvPGIP2 having the longest with 29 amino acids. Protein molecular mass (kDa) ranged from 35.92 for MtPGIP2 to 38.17 for MtPGIP1. And the isoelectric point (pI) ranged from 6.79 for PsPGIP1 to 9.48 for PvPGIP4. Except for MsPGIP2 of alfalfa, all other legume PGIPs had 10 LRRs and all were intronless. Only MsPGIP2’s genomic sequence contains 9 LRRs and a 154-bp intron. CaPGIP1, CaPGIP3, and CaPGIP4 exhibit very similar above-mentioned characteristics, with the exception that CaPGIP1 has the longest signal peptide among the known legume PGIPs so far, with 36 amino acids. Because of selection for transcription efficiency, conserved genes with relatively high levels of expression tend to lose introns (Zou et al., 2011). Intronless genes such as PGIP may play key roles in plant growth, development, or response to biotic or abiotic stresses (Liu et al., 2021).
Like many PGIPs, the central domain of CaPGIP1, CaPGIP3, and CaPGIP4 is comprised of 10 imperfect leucine-rich repeats (LRRs). Each is about 24 amino acids long and perfectly matches the extracellular LRR (eLRRs) consensus sequence xxLxLxx.NxLx. GxIPxxLxxL.xxL (Di Matteo et al., 2003). eLRRs are important in plant defense as they function as receptor-like proteins or receptor-like kinases to recognize diverse pathogen molecules, and plant hormones (van der Hoorn et al., 2005). The presence of xxLxLxxNxL core consensus in eLRRs is responsible for the ß–sheet structure formation (Zambounis et al., 2012). The LRRs are arranged tandemly in the PGIP’s distinctive curved and elongated shape. The ß-sheets are parallelly organized on the inner side of the protein to form the concave face, while the 310-helixes are parallelly organized on the outer side to make the convex face (Kobe and Deisenhofer, 1993). Solvent-exposed residues on the concave-sheet surface bind to pathogen molecules. CaPGIPs, have two types of β–sheets (β1 and β2) and 310-helixes. All LRRs of plant defense proteins have β1 sheet; however, the presence of β2 sheets is unique and seen only in PGIPs (Di Matteo et al., 2003). CaPGIPs are predicted to have several N-glycosylation on these ß-sheets which are vital for ligand binding for disease resistance, and the heterogeneity in ß-sheet residues or the glycosylation patterns contributes to the varying recognition specificities of LRR proteins (Ramanathan et al., 1997; van der Hoorn et al., 2005). The deduced CaPGIP proteins also contain conserved cysteine residues that form disulfide bridges crucial for the maintenance of secondary structures in PGIP (Veronico et al., 2011).
Previous research indicated that truncated variants of functioning NBS-LRR genes can be found within 100 kb of fully functional NBS-LRR genes. These truncated genes are often pseudogenes because of alternative splicing. These pseudogenes have large deletions due to various transposition events (Marone et al., 2013). CaPGIP2 is found within 30 kb of CaPGIP1. CaPGIP2’s C-terminal end matches CaPGIP1 perfectly, implying that the majority of the central LRR domain and N-terminus might be deleted. Also, the presence of a nearly 10-kbp intron is atypical for PGIPs and most functional genes. Since it also lacks a fundamental signal peptide, it is classified as a non-secretory protein. For these reasons, CaPGIP2 was not subjected to investigation in this study beyond the subcellular localization analysis.
Alignment of CaPGIP amino acid sequences with amino acid sequences from various other legume PGIPs revealed that CaPGIPs are similar to those of other characterized PGIPs. Interestingly, alignment also indicated that CaPGIPs along with other legume PGIPs are highly conserved at the β2-sheet sites, which may be because β2-sheet are present only in PGIPs and are absent in other LRR proteins. Even though there is a higher level of similarity in the ß sheet regions, there are also many variable portions present in both ß-sheets. This variability is most likely responsible for the presence of multiple recognition specificities to target broader pathogen PGs (De Lorenzo et al., 2001; Matsushima and Miyashita, 2012). As per the phylogenetic analysis, CaPGIPs have a high degree of similarity with PGIPs from different plant sources. CaPGIP1 shared up to 70% similarity with P. sativum PsPGIP1 (AJI49705.1), and 69% with G. max GmPGIP3 (NP 001304551.2). It has been previously reported that PsPGIP1 and GmPGIP3 potentially inhibit several pathogens. For instance, PsPGIP1 has been identified as a possible defense factor against the pea-cyst nematode Heterodera goettingiana (Veronico et al., 2011). Encoded protein products of GmPGIP3 inhibited PGs from Sclerotinia, Fusarium, and Botrytis (D’Ovidio et al., 2006; Wang et al., 2015). Transgenic wheat expressing GmPGIP3 also showed enhanced resistance to Gaeumannomyces graminis var. tritici, and Bipolaris sorokiniana (D’Ovidio et al., 2006; Wang et al., 2015). Due to CaPGIP1’s high sequence similarity to PsPGIP1 and GmPGIP3, it is likely to have similar functions and be engaged in nematode or fungal disease inhibition.
CaPGIP3 is more similar to the two tightly linked PGIPs of V. radiata, with 64% and 69% similarity to VrPGIP1 and VrPGIP2, respectively. Both VrPGIPs are known to provide resistance to bruchids (Callosobruchus spp) (Kaewwongwal et al., 2017), and CaPGIP3 may play a similar role in chickpeas against bruchids. CaPGIP4 had higher similarity to tree fruit PGIPs, with 72% similarity to Malus domestica MdPGIP1 (AAB19212.1) and 71% similarity to Pyrus communis PcPGIP (AAA33865.1). MdPGIP1 protein inhibited PG production in Colletotrichum lupini and Aspergillus niger (Oelofse et al., 2006). Unlike CaPGIP1 and CaPGIP3, CaPGIP4 may only be effective against pathogenic fungi.
The TATA box and CAAT box motifs are widely found in functional gene’s promoter and enhancer regions (Xue, 2002; Svensson et al., 2006). TATA boxes function as a motif for recruiting transcription initiation machinery and RNA polymerase II, while CAAT boxes improve protein binding (Joubert et al., 2013; Liao et al., 2015). TATA and CAAT boxes are conserved eukaryotic cis-elements that are found in many plant gene promoters, including PGIPs. CaPGIP1, CaPGIP3, and CaPGIP4 all have several TATA and CAAT boxes upstream of the start codon ATG, indicating that they are functioning genes. Plant PGIPs are typically expressed after pathogen infection and wounding response (Kalunke et al., 2015), hence the presence of multiple pathogenicity-related and wounding motifs in the CaPGI1, CaPGIP3, and CaPGIP4 promoter region. Apart from pathogens, PGIPs are triggered by phytohormone treatment in several plant species (Stotz et al., 1993; Yao et al., 1999; Ferrari et al., 2003). PGIP expression in rice, alfalfa, and pepper is induced by abscisic treatment. PGIPs from rapeseed, rice, barrel clover, and pepper are triggered by jasmonic acid and salicylic acid (Song and Nam, 2005; Janni et al., 2006; Hegedus et al., 2008; Lu et al., 2012; Wang et al., 2013). Rice PGIPs are induced by gibberellic acid treatment (Janni et al., 2006; Lu et al., 2012). The presence of multiple cis-acting elements in CaPGIP1, CaPGIP3, and CaPGIP4 that regulate abscisic acid, gibberellic acid, jasmonic acid, and salicylic acid pathways suggests that they may play comparable roles to those seen in many plant PGIPs. Like other families of defense-related genes, PGIPs demonstrate tissue-specific activity. The grapevine VvPGIP1 gene is only expressed in roots and ripening berries, and its expression is developmentally regulated (Joubert et al., 2006). Several cis-acting elements that influence tissue-specific responses, particularly root-specific responses, were found in all CaPGIPs, indicating a role in plant development or resistance to pathogens that enter the plant system through the roots. Brassica juncea PGIPs are associated with high temperature and drought stresses (Bhardwaj et al., 2015), while Arabidopsis AtPGIP1, and apple’s MdPGIP1, are induced in response to cold stress (Kalunke et al., 2015). The presence of regulatory elements associated with drought, dehydration, water, high light, and low-temperature stress in the CaPGIPpromoter suggests that they may play a role in plant stress.
Bioinformatic analysis and subcellular localization confirmed thatCaPGIP1, CaPGIP3, and CaPGIP4 are secretory proteins, and they are located in the plasma membrane or the cell wall. DeepLoc-1.0 analysis indicated CaPGIP2 might be found in the mitochondrion or the cytoplasm, and localization experiments revealed CaPGIP2 was found in the cytoplasm and endoplasmic reticulum. The locations of CaPGIPs within the plasma membrane/cell wall were consistent with the locations of other legume PGIPs. Localization on the plasma membrane is crucial as these proteins play a role in defense responses as cell surface receptors to detect pathogen PGs in the apoplast (Rodriguez-Palenzuela et al., 1991). CaPGIP2 localization to the cytoplasm and endoplasmic reticulum might be because of the lack of signal peptide in CaPGIP2 and suggests it might not be involved with the PG interaction in the apoplast.
Several studies investigatedPGIP gene expression in response to external stimuli. On the other hand, analyzing gene expression in the absence of external stimuli or treatment enables the correlation of the expression of different PGIP genes within a crop. Furthermore, pathogens can infect plants at any stage of their life cycle, and PGIP gene families, like other defense-related gene families, have been demonstrated to exhibit variable expression patterns (Kalunke et al., 2015). Because PGIP genes exhibit functional redundancy and sub-functionalization at the protein level (De Lorenzo et al., 2001; Federici et al., 2006), tissue-specific expression of PGIP genes is feasible, allowing them to respond more effectively to a variety of environmental stimuli (D’Ovidio et al., 2004). In terms of pathogen PG specificity, plant PGIP genes can express at higher levels in distinct growth stages and tissues that correspond to pathogen infection (Cantu et al., 2008). Absolute expression analysis showed CaPGIP genes has higher expression in leaf tissue and the least in pod tissues. B. vulgaris’s BvPGIP genes were reported to be highly expressed in roots in comparison to leaf tissue during normal growth and development (Li and Smigocki, 2016). Carica papaya’s CpPGIP4 and CpPGIP6 genes were shown to be ubiquitously expressed in root, stem, leaf, seed fruit pulp and peel. However, the CpPGIP gene transcripts were most abundant in fruit pulp and peel and decreased during ripening (Broetto et al., 2015). Tissue-specific differences have been reported in apples, where higher transcript abundance was in leaves and fruit, least in the stem (Zhang et al., 2010). In blackberry PGIP gene expression was more abundant in young leaves and fruit compared to old leaves and ripe fruit (Hu et al., 2012). In raspberries, PGIP transcripts were detected in fruit but not in flowers (Johnston et al., 1993). The varying expression levels of CaPGIP genes in different tissues at different growth stages indicates that CaPGIP genes might respond to different external stimuli.
5 Conclusion
In conclusion, this study is the first to characterize chickpea PGIPs. Two additional PGIPs on chromosome 3, CaPGIP3 and CaPGIP4 were identified in addition to the previously reported CaPGIP1 and CaPGIP2 on chromosome 6 and this necessitated modifying the genomic organization of CaPGIPs. CaPGIP1, CaPGIP3, and CaPGIP4 displayed a typical PGIP sequence identity with an N-terminal domain, a central LRR domain with ten imperfect LRRs, and a C-terminal domain. Multiple sequence alignment shows that CaPGIP amino acid sequences are highly similar to those of other described legumes. The phylogenetic study of CaPGIPs indicated that CaPGIP1 and CaPGIP3 are similar to legume PGIPs, and CaPGIP4 falls outside the legume PGIP cluster. CaPGIP’s promoter sequences harbor cis-elements that regulate response to various external stimuli. CaPGIP1, CaPGIP3, and CaPGIP4 are localized to the cell wall or plasm membrane. Absolute quantification of the CaPGIP transcript levels under untreated conditions demonstrates that CaPGIPs have tissue-specific expression. Interestingly, CaPGIP2 lacked most of the characteristics typical of a PGIP and warrants further investigations.
Data availability statement
The raw data supporting the conclusion of this article will be made available by the authors, without undue reservation.
Author contributions
VE and WC conceived the study and designed the experiments. VE performed the experiments. VE analyzed the data. VE wrote the manuscript with contributions from all co-authors. All authors contributed to the article and approved the submitted version.
Acknowledgments
We would like to thank Dan Mullendore of Franceschi Microscopy and Imaging Center, Washington State University, Pullman, WA for assistance with confocal microscopy. We would also like to thank Sheri McGrew, Grain Legume Genetics Physiology Research, USDA ARS, Pullman, WA for assistance with maintaining plants in the green house. We would also like to thank Tony Chen, Grain Legume Genetics Physiology Research, USDA ARS, Pullman, WA for assistance in laboratory work. We would also like to thank Derek Pouchnik and Weiwei Du of Laboratory for Biotechnology and Bioanalysis Washington State University, Pullman, WA for their assistance with plasmid and DNA sequencing. Financial support from the Indian Council for Agricultural Sciences (ICAR) is gratefully acknowledged.
Conflict of interest
The authors declare that the research was conducted in the absence of any commercial or financial relationships that could be construed as a potential conflict of interest.
Publisher’s note
All claims expressed in this article are solely those of the authors and do not necessarily represent those of their affiliated organizations, or those of the publisher, the editors and the reviewers. Any product that may be evaluated in this article, or claim that may be made by its manufacturer, is not guaranteed or endorsed by the publisher.
Supplementary material
The Supplementary Material for this article can be found online at: https://www.frontiersin.org/articles/10.3389/fgene.2023.1189329/full#supplementary-material
SUPPLEMENTARY TABLE S1 | Genes identified through a homology database search in Cicer arietinum genome that exhibited PGIP features.
SUPPLEMENTARY TABLE S2 | Primers used in this study to isolate, clone, and for the RT-qPCR expression analysis of the Capgips.
SUPPLEMENTARY TABLE S3 | Other putative cis-acting regulatory elements identified in the promoter regions of Capgips.
References
Albersheim, P., and Anderson, A. J. (1971). Proteins from plant cell walls inhibit polygalacturonases secreted by plant pathogens. Proc. Natl. Acad. Sci. U. S. A. 68 (8), 1815–1819. doi:10.1073/pnas.68.8.1815
Armenteros, J. J. A., Sønderby, C. K., Sønderby, S. K., Nielsen, H., and Winther, O. (2017). DeepLoc: Prediction of protein subcellular localization using deep learning. Bioinforma. Oxf. Engl. 33 (21), 3387–3395. doi:10.1093/bioinformatics/btx431
Armenteros, J. J. A., Tsirigos, K. D., Sønderby, C. K., Petersen, T. N., Winther, O., Brunak, S., et al. (2019). SignalP 5.0 improves signal peptide predictions using deep neural networks. Nat. Biotechnol. 37, 420–423. doi:10.1038/s41587-019-0036-z
Bhardwaj, A. R., Joshi, G., Kukreja, B., Malik, V., Arora, P., Pandey, R., et al. (2015). Global insights into high temperature and drought stress regulated genes by RNA-Seq in economically important oilseed crop Brassica juncea. BMC plant Biol. 15, 9. doi:10.1186/s12870-014-0405-1
Broetto, S. G., Fabi, J. P., and Nascimento, J. R. O. do. (2015). Cloning and expression analysis of two putative papaya genes encoding polygalacturonase-inhibiting proteins. Postharvest Biol. Technol. 104, 48–56. doi:10.1016/j.postharvbio.2015.03.002
Bustin, S. A., Benes, V., Garson, J. A., Hellemans, J., Huggett, J., Kubista, M., et al. (2009). The MIQE guidelines: Minimum information for publication of quantitative real-time PCR experiments. Clin. Chem. 55 (4), 611–622. doi:10.1373/clinchem.2008.112797
Cantu, D., Vicente, A. R., Greve, L. C., Dewey, F. M., Bennett, A. B., Labavitch, J. M., et al. (2008). The intersection between cell wall disassembly, ripening, and fruit susceptibility to Botrytis cinerea. Proc. Natl. Acad. Sci. U. S. A. 105 (3), 859–864. doi:10.1073/pnas.0709813105
D'Ovidio, R., Roberti, S., Di Giovanni, M., Capodicasa, C., Melaragni, M., Sella, L., et al. (2006). The characterization of the soybean polygalacturonase-inhibiting proteins (Pgip) gene family reveals that a single member is responsible for the activity detected in soybean tissues. Planta 224 (3), 633–645. doi:10.1007/s00425-006-0235-y
Daher, F. B., and Braybrook, S. A. (2015). How to let go: Pectin and plant cell adhesion. Front. plant Sci. 6, 523. doi:10.3389/fpls.2015.00523
De Lorenzo, G., D’Ovidio, R., and Cervone, F. (2001). The role of polygalacturonase-inhibiting proteins (PGIPs) in defense against pathogenic fungi. Annu. Rev. Phytopathology 39, 313–335. doi:10.1146/annurev.phyto.39.1.313
Desiderio, A., Aracri, B., Leckie, F., Mattei, B., Salvi, G., Tigelaar, H., et al. (1997). Polygalacturonase-inhibiting proteins (PGIPs) with different specificities are expressed in Phaseolus vulgaris. Phaseolus Vulgaris. Mol. Plant Microbe Interact. 10, 852–860. doi:10.1094/MPMI.1997.10.7.852
Di Giovanni, M., Cenci, A., Janni, M., and D'Ovidio, R. (2008). A LTR copia retrotransposon and Mutator transposons interrupt PGIP genes in cultivated and wild wheats. Theor. Appl. Genet. 116, 859–867. doi:10.1007/s00122-008-0719-1
Di Matteo, A., Bonivento, D., Tsernoglou, D., Federici, L., and Cervone, F. (2006). Polygalacturonase-inhibiting protein (PGIP) in plant defence: A structural view. Phytochemistry 67 (6), 528–533. doi:10.1016/j.phytochem.2005.12.025
Di Matteo, A., Federici, L., Mattei, B., Salvi, G., Johnson, K. A., Savino, C., et al. (2003). The crystal structure of polygalacturonase-inhibiting protein (PGIP), a leucine-rich repeat protein involved in plant defense. Proc. Natl. Acad. Sci. U. S. A. 100 (17), 10124–10128. doi:10.1073/pnas.1733690100
D’Ovidio, R., Mattei, B., Roberti, S., and Bellincampi, D. (2004). Polygalacturonases, polygalacturonase-inhibiting proteins and pectic oligomers in plant–pathogen interactions. Biochimica Biophysica Acta (BBA) - Proteins Proteomics 1696 (2), 237–244. doi:10.1016/j.bbapap.2003.08.012
Farina, A., Rocchi, V., Janni, M., Benedettelli, S., De Lorenzo, G., and D'Ovidio, R. (2009). The bean polygalacturonase-inhibiting protein 2 (PvPGIP2) is highly conserved in common bean (Phaseolus vulgaris L) germplasm and related species. Theor. Appl. Genet. 118 (7), 1371–1379. doi:10.1007/s00122-009-0987-4
Federici, L., Di Matteo, A., Fernandez-Recio, J., Tsernoglou, D., and Cervone, F. (2006). Polygalacturonase inhibiting proteins: Players in plant innate immunity? Trends plant Sci. 11 (2), 65–70. doi:10.1016/j.tplants.2005.12.005
Ferrari, S., Vairo, D., Ausubel, F. M., Cervone, F., and De Lorenzo, G. (2003). Tandemly duplicated Arabidopsis genes that encode polygalacturonase-inhibiting proteins are regulated coordinately by different signal transduction pathways in response to fungal infection. Plant Cell 15 (1), 93–106. doi:10.1105/tpc.005165
Forlenza, M., Kaiser, T., Savelkoul, H. F. J., and Wiegertjes, G. F. (2012). in The use of real-time quantitative PCR for the analysis of cytokine mRNA levels BT” in cytokine protocols. Editor M. De Ley (Totowa, NJ: Humana Press), 7–23. doi:10.1007/978-1-61779-439-1_2
Frati, F., Galletti, R., De Lorenzo, G., Salarino, G., and Conti, E. (2006). Activity of endo-polygalacturonases in mirid bugs (heteroptera: Miridae) and their inhibition by plant cell wall proteins (PGIPs). Eur. J. Entomology 103, 515–522. doi:10.14411/eje.2006.067
Gao, J., Gui, Z., Wang, Y., Liu, H., Xin, N., Zhang, X., et al. (2015). Polymorphism of polygalacturonase-inhibiting protein genes by fluorescence-based PCR single-strand conformation profiling and sequencing in autotetraploid alfalfa (Medicago sativa) populations. Euphytica 206, 567–577. doi:10.1007/s10681-015-1455-7
Gasteiger, E., Hoogland, C., Gattiker, A., Duvaud, S., Wilkins, M. R., Appel, R. D., et al. (2005). in Protein identification and analysis tools on the ExPASy server” in the proteomics protocols handbook. Editor J. M. Walker Totowa, NJ: Humana Press Inc, 571–608. doi:10.1385/1-59259-890-0:571
Ge, T., Huang, X., and Xie, R. (2019). Recent advances in polygalacturonase gene in fruit tree species. Plant Physiology J. 55, 1075–1088.
Gupta, R., and Brunak, S. (2002). Prediction of glycosylation across the human proteome and the correlation to protein function. Pac. Symposium Biocomput., 310–322.
Haeger, W., Wielsch, N., Shin, N. R., Gebauer-Jung, S., Pauchet, Y., and Kirsch, R. (2021). New players in the interaction between beetle polygalacturonases and plant polygalacturonase-inhibiting proteins: Insights from proteomics and gene expression analyses. Front. plant Sci. 12, 660430. doi:10.3389/fpls.2021.660430
Hamera, S., Mural, R. V., Liu, Y., and Zeng, L. (2014). The tomato ubiquitin-conjugating enzyme variant Suv, but not SlUev1C and SlUev1D regulates Fen-mediated programmed cell death in Nicotiana benthamiana. Plant Signal. Behav. 9 (10), e973814. doi:10.4161/15592324.2014.973814
Hegedus, D. D., Li, R., Buchwaldt, L., Parkin, I., Whitwill, S., Coutu, C., et al. (2008). Brassica napus possesses an expanded set of polygalacturonase inhibitor protein genes that are differentially regulated in response to Sclerotinia sclerotiorum infection, wounding and defense hormone treatment. Planta 228 (2), 241–253. doi:10.1007/s00425-008-0733-1
Higo, K., Ugawa, Y., Iwamoto, M., and Korenaga, T. (1999). Plant cis-acting regulatory DNA elements (PLACE) database. Nucleic Acids Res. 27 (1), 297–300. doi:10.1093/nar/27.1.297
Hou, W., Mu, J., Li, A., Wang, H., and Kong, L. (2015). Identification of a wheat polygalacturonase-inhibiting protein involved in Fusarium head blight resistance. Eur. J. Plant Pathology 141 (4), 731–745. doi:10.1007/s10658-014-0574-7
Hu, D., Dai, R., Wang, Y., Zhang, Y., Liu, Z., Fanf, R., et al. (2012). Molecular cloning, sequence analysis, and expression of the polygalacturonase-inhibiting protein (PGIP) gene in mulberry. Plant Mol. Biol. Rep. 30, 176–186. doi:10.1007/s11105-011-0324-3
Hwang, B. H., Bae, H., Lim, H. S., Kim, K. B., Kim, S. J., Im, M. H., et al. (2010). Overexpression of polygalacturonase-inhibiting protein 2 (PGIP2) of Chinese cabbage (Brassica rapa ssp. pekinensis) increased resistance to the bacterial pathogen Pectobacterium carotovorum ssp. Carotovorum. Plant Cell, Tissue, Organ Cult. 103, 293–305. doi:10.1007/s11240-010-9779-4
Janni, M., Di Giovanni, M., Roberti, S., Capodicasa, C., and D'Ovidio, R. (2006). Characterization of expressed Pgip genes in rice and wheat reveals similar extent of sequence variation to dicot PGIPs and identifies an active PGIP lacking an entire LRR repeat. Theor. Appl. Genet. 113 (7), 1233–1245. doi:10.1007/s00122-006-0378-z
Jiménez-Fernández, D., Montes-Borrego, M., Jiménez-Díaz, R. M., Navas-Cortés, J. A., and Landa, B. B. (2011). In planta and soil quantification of Fusarium oxysporum f. sp. ciceris and evaluation of Fusarium wilt resistance in chickpea with a newly developed quantitative polymerase chain reaction assay. Phytopathology 101 (2), 250–262. doi:10.1094/phyto-07-10-0190
Johnston, D. J., Ramanathan, V., and Williamson, B. (1993). A protein from immature raspberry fruits which inhibits endopolygalacturonases from Botrytis cinerea and other micro-organisms. J. Exp. Bot. 44 (5), 971–976. doi:10.1093/jxb/44.5.971
Joubert, D. A., de Lorenzo, G., and Vivier, M. A. (2013). Regulation of the grapevine polygalacturonase-inhibiting protein encoding gene: Expression pattern, induction profile and promoter analysis. J. Plant Res. 126 (2), 267–281. doi:10.1007/s10265-012-0515-5
Joubert, D. A., Slaughter, A. R., Kemp, G., Becker, J. V. W., Krooshof, G. H., Bergmann, C., et al. (2006). The grapevine polygalacturonase-inhibiting protein (VvPGIP1) reduces Botrytis cinerea susceptibility in transgenic tobacco and differentially inhibits fungal polygalacturonases. Transgenic Res. 15 (6), 687–702. doi:10.1007/s11248-006-9019-1
Jukanti, A. K., Gaur, P. M., Gowda, C. L., and Chibbar, R. N. (2012). Nutritional quality and health benefits of chickpea (cicer arietinum L) A review. Br. J. Nutr. 108, S11–S26. doi:10.1017/S0007114512000797
Kaewwongwal, A., Chen, J., Somta, P., Kongjaimun, A., Yimram, T., Chen, X., et al. (2017). Novel alleles of two tightly linked genes encoding polygalacturonase-inhibiting proteins (VrPGIP1 and VrPGIP2) associated with the Br locus that confer bruchid (callosobruchus spp) resistance to mungbean (Vigna radiata) accession V2709. Front. plant Sci. 8, 1692. doi:10.3389/fpls.2017.01692
Kalunke, R. M., Cenci, A., Volpi, C., O’Sullivan, D. M., Sella, L., Favaron, F., et al. (2014). The PGIP family in soybean and three other legume species: Evidence for a birth-and-death model of evolution. BMC Plant Biol. 14, 189. doi:10.1186/s12870-014-0189-3
Kalunke, R. M., Janni, M., Sella, L., David, P., Geffroy, V., Favaron, F., et al. (2011). Transcript analysis of the bean polygalacturonase inhibiting protein gene family reveals that PVPGIP2 is expressed in the whole plant and is strongly induced by pathogen infection. J. Plant Pathology 93 (1), 141–148.
Kalunke, R. M., Tundo, S., Benedetti, M., Cervone, F., De Lorenzo, G., and D'Ovidio, R. (2015). An update on polygalacturonase-inhibiting protein (PGIP), a leucine-rich repeat protein that protects crop plants against pathogens. Front. Plant Sci. 6, 146. doi:10.3389/fpls.2015.00146
Khan, R. A., Bhat, A. T., and Kumar, K. (2012). Management of chickpea (cicer arietinum L) dry root rot caused by rhizoctonia bataticola (taub) butler. Int. J. Res. Pharm. Biomed. Sci. 3 (4), 1539–1548.
Knights, E. J., and Hobson, K. B. (2016). Chickpea overview. Retrieved from https://www.sciencedirect.com/science/article/pii/B9780081005965000354.
Kobe, B., and Deisenhofer, J. (1993). Crystal structure of porcine ribonuclease inhibitor, a protein with leucine-rich repeats. Nature 366 (6457), 751–756. doi:10.1038/366751a0
Kubicek, C. P., Starr, T. L., and Glass, N. L. (2014). Plant cell wall-degrading enzymes and their secretion in plant-pathogenic fungi. Annu. Rev. phytopathology 52, 427–451. doi:10.1146/annurev-phyto-102313-045831
Kumar, S., Stecher, G., Li, M., Knyaz, C., and Tamura, K. (2018). Mega X: Molecular evolutionary Genetics analysis across computing platforms. Mol. Biol. Evol. 35 (6), 1547–1549. doi:10.1093/molbev/msy096
Letunic, I., and Bork, P. (2021). Interactive tree of life (iTOL) v5: An online tool for phylogenetic tree display and annotation. Nucleic acids Res. 49 (W1), W293–W296. doi:10.1093/nar/gkab301
Li, H., and Smigocki, A. C. (2016). Wound induced Beta vulgaris polygalacturonase-inhibiting protein genes encode a longer leucine-rich repeat domain and inhibit fungal polygalacturonases. Physiological Mol. Plant Pathology 96, 8–18. doi:10.1016/j.pmpp.2016.06.004
Liao, Y. L., 1,2 Shen, Y. B., Chang, J., Zhang, W. W., Cheng, S. Y., and Xu, F. (2015). Isolation, expression, and promoter analysis of GbWRKY2: A novel transcription factor gene from ginkgo biloba. Int. J. Genomics 2015, 607185. doi:10.1155/2015/607185
Liu, H., Lyu, H.-M., Zhu, K., Peer, Y. Van de., and Cheng, Z.-M. (2021). The emergence and evolution of intron-poor and intronless genes in intron-rich plant gene families. Plant J. 105, 1072–1082. doi:10.1111/tpj.15088
Lu, L., Zhou, F., Zhou, Y., Fan, X., Ye, S., Wang, L., et al. (2012). Expression profile analysis of the polygalacturonase-inhibiting protein genes in rice and their responses to phytohormones and fungal infection. Plant Cell Rep. 31 (7), 1173–1187. doi:10.1007/s00299-012-1239-7
Markel, S., Wise, K., McKay, K., Goswami, R., and Gudmestad, N. (2008). Ascochyta blight of chickpea. Retrieved from North Dakota State University website //efaidnbmnnnibpcajpcglclefindmkaj/https://www.ndsu.edu/pubweb/∼goswami/Publications/Aschochyta%20Blight%20fact-sheet.pdf.
Marone, D., Russo, M. A., Laidò, G., De Leonardis, A. M., and Mastrangelo, A. M. (2013). Plant nucleotide binding site-leucine-rich repeat (NBS-LRR) genes: Active guardians in host defense responses. Int. J. Mol. Sci. 14 (4), 7302–7326. doi:10.3390/ijms14047302
Matsaunyane, L. B., Oelofse, D., and Dubery, I. A. (2015). In silico analysis of the polygalacturonase inhibiting protein 1 from apple, Malus domestica. BMC Res. Notes 8 (1), 76.
Matsushima, N., and Miyashita, H. (2012). Leucine-rich repeat (LRR) domains containing intervening motifs in plants. Biomolecules 2, 288–311. doi:10.3390/biom2020288
Mazur, S., Nawrocki, J., and Kućmierz, J. (2002). Fungal diseases of chickpea (cicer arietinum L) cultivated in the south region of Poland. Plant Prot. Sci. 38 (Special Issue 2), 332–335. doi:10.17221/10483-pps
Merga, B., and Haji, J. (2019). Economic importance of chickpea: Production, value, and world trade. Cogent Food & Agric. 5 (1), 1615718. doi:10.1080/23311932.2019.1615718
Mohnen, D. (1999). “3.15 biosynthesis of pectins and galactomannans,” in Comprehensive natural products chemistry, 497–527. doi:10.1016/b978-0-08-091283-7.00099-0
Mojsov, K. D. (2016). “Chapter 16 - Aspergillus enzymes for food industries,” in New and future developments in microbial Biotechnology and bioengineering. Editor V. K. Gupta (Amsterdam: Elsevier), 215–222. doi:10.1016/B978-0-444-63505-1.00033-6
Moore, M., Ryley, T., Knights, P., Nash, G., and Chiplin, G., and , Cumming. (2011). Chickpeas—varietal selection, paddock planning and disease management in 2011. Northern region (Goondiwindi). GRDC Update Papers April 2011.
Oelofse, D., Dubery, I. A., Meyer, R., Arendse, M. S., Gazendam, I., and Berger, D. K. (2006). Apple polygalacturonase inhibiting protein1 expressed in transgenic tobacco inhibits polygalacturonases from fungal pathogens of apple and the anthracnose pathogen of lupins. Phytochemistry 67 (3), 255–263. doi:10.1016/j.phytochem.2005.10.029
Pfaffl, M. W. (2001). A new mathematical model for relative quantification in real-time RT-PCR. Nucleic acids Res. 29 (9), e45. doi:10.1093/nar/29.9.e45
Ramanathan, V., Simpson, C. G., Thow, G., Iannetta, P. P. M., McNicol, R. J., and Williamson, B. (1997). cDNA cloning and expression of polygalacturonase inhibiting proteins (PGIPs) from red raspberry (Rubus idaeus). J. Exp. Bot. 48 (6), 1185–1193. doi:10.1093/jxb/48.6.1185
Rodriguez-Palenzuela, P., Burr, T. J., and Collmer, A. (1991). Polygalacturonase is a virulence factor in Agrobacterium tumefaciens biovar 3. J. Bacteriol. 173 (20), 6547–6552. doi:10.1128/jb.173.20.6547-6552.1991
SAS Institute Inc (2013). SAS® 9.4 guide to software updates and product changes. Cary, NC: SAS Institute Inc.
Sharma, S., Upadhyaya, H. D., Roorkiwal, M., Varshney, R. K., and Gowda, C. L. L. (2013). “4 - chickpea,” in Genetic and genomic resources of Grain legume improvement. Editors M. Singh, H. D. Upadhyaya, and I. S. Bisht (Oxford: Elsevier), 81–111. doi:10.1016/B978-0-12-397935-3.00004-9
Song, K. H., and Nam, Y. W. (2005). Genomic organization and differential expression of two polygalacturonase-inhibiting protein genes from Medicago truncatula. J. Plant Biol. 48, 467–478. doi:10.1007/BF03030589
Stotz, H. U., Powell, A. L., Damon, S. E., Greve, L. C., Bennett, A. B., and Labavitch, J. M. (1993). Molecular characterization of a polygalacturonase inhibitor from Pyrus communis L. cv Bartlett. Plant physiol. 102 (1), 133–138. doi:10.1104/pp.102.1.133
Svensson, J. T., Crosatti, C., Campoli, C., Bassi, R., Stanca, A. M., Close, T. J., et al. (2006). Transcriptome analysis of cold acclimation in barley albina and xantha mutants. Plant physiol. 141 (1), 257–270. doi:10.1104/pp.105.072645
Toubart, P., Desiderio, A., Salvi, G., Cervone, F., Daroda, L., and De Lorenzo, G. (1992). Cloning and characterization of the gene encoding the endopolygalacturonase-inhibiting protein (PGIP) of Phaseolus vulgaris L. Plant J. Cell Mol. Biol. 2 (3), 367–373. doi:10.1046/j.1365-313x.1992.t01-35-00999.x
Untergasser, A., Nijveen, A., Rao, X., Bisseling, T., Geurts, R., and Leunissen, J. A. M. (2007). Primer3Plus, an enhanced web interface to Primer3. Nucleic Acids Res. 35, W71–W74. doi:10.1093/nar/gkm306
van der Hoorn, R. A., Wulff, B. B., Rivas, S., Durrant, M. C., van der Ploeg, A., de Wit, P. J., et al. (2005). Structure-function analysis of cf-9, a receptor-like protein with extracytoplasmic leucine-rich repeats. plant Cell 17 (3), 1000–1015. doi:10.1105/tpc.104.028118
Veronico, P., Melillo, M. T., Saponaro, C., Leonetti, P., Picardi, E., and Jones, J. T. (2011). A polygalacturonase-inhibiting protein with a role in pea defence against the cyst nematode Heterodera goettingiana. Mol. plant Pathol. 12 (3), 275–287. doi:10.1111/j.1364-3703.2010.00671.x
Voragen, A. G. J., Coenen, G. J., Verhoef, R. P., and Schols, H. A. (2009). Pectin, a versatile polysaccharide present in plant cell walls. Struct. Chem. 20, 263–275. doi:10.1007/s11224-009-9442-z
Wang, A., Wei, X., Rong, W., Dang, L., Du, L. P., Qi, L., et al. (2015). GmPGIP3 enhanced resistance to both take-all and common root rot diseases in transgenic wheat. Funct. Integr. genomics 15 (3), 375–381. doi:10.1007/s10142-014-0428-6
Wang, X., Zhu, X., Tooley, P., and Zhang, X. (2013). Cloning and functional analysis of three genes encoding polygalacturonase-inhibiting proteins fromCapsicum annuum and transgenic CaPGIP1 in tobacco in relation to increased resistance to two fungal pathogens. Plant Mol. Biol. 81, 379–400. doi:10.1007/s11103-013-0007-6
Wang, Y., Zhang, P., Li, L., Li, D., Liang, Z., Cao, Y., et al. (2022). Proteomic analysis of alfalfa (Medicago sativa L) roots in response to rhizobium nodulation and salt stress. Genes 13 (11). doi:10.3390/genes13112004
Wang, Z., Wan, L., Zhang, X., Xin, Q., Song, Y., Hong, D., et al. (2021). Interaction between Brassica napus polygalacturonase inhibition proteins and Sclerotinia sclerotiorum polygalacturonase: Implications for rapeseed resistance to fungal infection. Planta 253 (2), 34. doi:10.1007/s00425-020-03556-2
Waterhouse, A., Bertoni, M., Bienert, S., Studer, G., Tauriello, G., Gumienny, R., et al. (2018). SWISS-MODEL: Homology modelling of protein structures and complexes. Nucleic Acids Res. 46 (W1), W296-W303–W303. doi:10.1093/nar/gky427
Waterhouse, A. M., Procter, J. B., Martin, D. M. A., Clamp, M., and Barton, G. J. (2009). Jalview Version 2-A multiple sequence alignment editor and analysis workbench. Bioinformatics 25, 1189–1191. doi:10.1093/bioinformatics/btp033
West, V. P., Appiah, A. A., and Gow, A. R. N. (2003). Advances in research on oomycete root pathogens. Physiological Mol. Plant Pathology 62 (2), 99–113. doi:10.1016/s0885-5765(03)00044-4
Wong, M. L., and Medrano, J. F. (2005). Real-time PCR for mRNA quantitation. BioTechniques 39 (1), 75–85. doi:10.2144/05391RV01
Wunsch, M. (2014). Management of Ascochyta blight of chickpea. Retrieved from Montana State University website http://pspp.msuextension.org/documents/NDSUCHICKPEA%20Ascochyta%20mgmt.pdf.
Xue, G. P. (2002). Characterisation of the DNA-binding profile of barley HvCBF1 using an enzymatic method for rapid, quantitative and high-throughput analysis of the DNA-binding activity. Nucleic acids Res. 30 (15), e77. doi:10.1093/nar/gnf076
Yao, C., Conway, W. S., Ren, R., Smith, D., Ross, G. S., and Sams, E. (1999). Gene encoding polygalacturonase inhibitor in apple fruit is developmentally regulated and activated by wounding and fungal infection. Plant Mol. Biol. 39, 1231–1241. Available at: https://naldc.nal.usda.gov/download/31319/PDF. doi:10.1023/a:1006155723059
Zambounis, A., Elias, M., Sterck, L., Maumus, F., and Gachon, C. M. (2012). Highly dynamic exon shuffling in candidate pathogen receptors. what if Brown algae were capable of adaptive immunity? Mol. Biol. Evol. 29 (4), 1263–1276. doi:10.1093/molbev/msr296
Zhang, Q., Yan, Q., Yuan, X., Lin, Y., Chen, J., Wu, R., et al. (2021). Two polygalacturonase-inhibiting proteins (VrPGIP) of Vigna radiata confer resistance to bruchids (Callosobruchus spp). J. plant physiology 258-259, 153376. doi:10.1016/j.jplph.2021.153376
Zhou, B., Mural, R. V., Chen, X., Oates, M. E., Connor, R. A., Martin, G. B., et al. (2017). A subset of ubiquitin-conjugating enzymes is essential for plant immunity. Plant Physiol. 173 (2), 1371–1390. doi:10.1104/pp.16.01190
Keywords: polygalacturonase inhibitory proteins (PGIPs), gene family, defense-related gene, biotic stress response, leucine-rich repeats (LRRs), promoter analysis, constitutive gene expression, subcellular localization
Citation: Ellur V, Wei W, Ghogare R, Solanki S, Vandemark G, Brueggeman R and Chen W (2023) Unraveling the genomic reorganization of polygalacturonase-inhibiting proteins in chickpea. Front. Genet. 14:1189329. doi: 10.3389/fgene.2023.1189329
Received: 19 March 2023; Accepted: 26 May 2023;
Published: 05 June 2023.
Edited by:
Balaji Aravindhan Pandian, Enko Chem Inc., United StatesReviewed by:
Roy Kirsch, Max Planck Institute for Chemical Ecology, GermanySilvio Tundo, University of Padua, Italy
Karthik Putta, ENKOCHEM, United States
Copyright © 2023 Ellur, Wei, Ghogare, Solanki, Vandemark, Brueggeman and Chen. This is an open-access article distributed under the terms of the Creative Commons Attribution License (CC BY). The use, distribution or reproduction in other forums is permitted, provided the original author(s) and the copyright owner(s) are credited and that the original publication in this journal is cited, in accordance with accepted academic practice. No use, distribution or reproduction is permitted which does not comply with these terms.
*Correspondence: Weidong Chen, d2VpZG9uZy5jaGVuQHVzZGEuZ292