- 1Department of Biological Pharmacy, Faculty of Pharmacy, Universitas Padjadjaran, Bandung, Indonesia
- 2Center of Excellence for Pharmaceutical Care Innovation, Universitas Padjadjaran, Bandung, Indonesia
- 3Division of Pharmacology and Therapy, Department of Biomedical Sciences, Faculty of Medicine, Universitas Padjadjaran, Bandung, Indonesia
The World Health Organization (WHO) stated that ensuring access to effective and optimal treatment is a key component to eradicate tuberculosis (TB) through the End TB Strategy. Personalized medicine that depends on the genetic profile of an individual is one way to optimize treatment. It is necessary because of diverse drug responses related to the variation in human DNA, such as single-nucleotide polymorphisms (SNPs). Ethambutol (EMB) is a drug widely used as the treatment for Mycobacterium Tuberculosis (Mtb) and/non-tuberculous mycobacteria and has become a potential supplementary agent for a treatment regimen of multidrug-resistant (MDR) and extensively drug-resistant (XDR) TB. In human genetic polymorphism studies of anti-tuberculosis, the majority focus on rifampicin or isoniazid, which discuss polymorphisms related to their toxicity. Whereas there are few studies on EMB, the incidence of EMB toxicity is lower than that of other first-line anti-TB drugs. To facilitate personalized medicine practice, this article summarizes the genetic polymorphisms associated with alterations in the pharmacokinetic profile, resistance incidence, and susceptibility to EMB toxicity. This study includes 131 total human studies from 17 articles, but only eight studies that held in the low-middle income country (LMIC), while the rest is research conducted in developed countries with high incomes. Personalized medicine practices are highly recommended to maintain and obtain the optimal therapeutic effect of EMB.
1 Introduction
Tuberculosis (TB) has high mortality rate of infectious disease in the world, affecting an estimated ten million people per year in 2019. According to the World Health Organization (WHO), tuberculosis (TB) is one of the top 10 causes of death globally, and in 2020, an estimated 10 million people fell ill with TB and 1.5 million died from the disease [(WHO) World Health Organization, 2020a]. In contrast, atypical mycobacterial infections are generally less severe and less widespread, although they can still cause significant morbidity and mortality in certain populations, such as those with weakened immune systems (Falkinham, 2015; Drummond and Kasperbauer, 2019; Winburn and Sharman, 2022). The reason of the importance to handle the Mycobacterium tuberculosis (Mtb) is based on recent article which highlights the ongoing challenges in TB control and the need for new tools and strategies to combat the disease. This article underscores the continued global burden of TB and the urgent need for new approaches to prevention, diagnosis, and treatment (Esmail et al., 2022).
Most drug-susceptible (DS)-TB patients will have a positive treatment response when treated with the right combination of first-line anti-TB drugs and treatment regimen (duration and dose of the drugs). The World Health Organization (WHO) stated that ensuring access to effective and optimal treatment is a key component to eradicate TB through the End TB Strategy, which includes a priority indicator that 90% or more patients should have a successful treatment outcome (World Health Organization, 2020b). According to WHO, high burden countries for TB are consist of 30 Low- and Middle-Income Countries (LMIC) (World Health Organization, 2021). Ongoing delayed of diagnosis and treatment in LMIC still become problems, worsening prognosis and also continuing TB transmission in the community (Teo et al., 2021). Furthermore, this condition is detrimental to resistance of TB therapy. Resistance to the therapy and risk of drugs adverse effects are the things that could detain to achieve optimal therapy (Pradipta et al., 2018). So, personalized treatment that depends on the individual genetic profile can minimize the risk of toxicity and resistance, thus optimize the treatment. It becomes necessary because of the diversity of drug responses related to the variation in human DNA, such as single-nucleotide polymorphisms (SNPs), which are a single substitution of nucleotides for another (Lander et al., 2001; Subramanian et al., 2001; Kurkó et al., 2013). SNPs that occur in genes related to pharmacokinetics (PK) and pharmacodynamics (PD) processes could affect the response, effectiveness, resistance, and toxicity of drugs (Zastrozhin et al., 2018; Calcagno et al., 2019; Xiang et al., 2020).
Ethambutol (EMB) is a drug widely used for TB treatment. It is used as the first-line anti-TB drug together with rifampicin (RIF), pyrazinamide (PZA), and isoniazid (INH) as a six-month regimen. EMB should not be used alone as monotherapy but rather in tandem with at least one other anti-TB drug. EMB shows a specific effectiveness against the Mtb and atypical/non-tuberculous mycobacteria such as Mycobacterium avium complex bacteria (MAC) that cause pulmonary infection non-tuberculosis and lymphadenitis, but not against other bacteria or other pathogens, such as viruses and fungi (Lee and Nguyen, 2020; Winburn and Sharman, 2022) EMB is also a potential supplementary agent for a treatment regimen of multidrug-resistant (MDR) and extensively drug-resistant (XDR) TB (World Health Organization, 2020a). Although as a supplementary agent, EMB is added to the TB regimen as a protection against unrecognized resistance to one of the three core drugs (Horsburgh et al., 2015). However, the WHO recently reported that patients infected with Mtb strain and showing simultaneous resistance to EMB and INH or EMB and RIF had an increased risk of treatment failure and further acquired resistance (Falzon et al., 2011). EMB is a bacteriostatic drug that interferes with the biosynthesis of arabinogalactan in the cell wall of Mtb and inhibits multiplication by bacilli (Palomino and Martin, 2014). However, the exact molecular mechanism of action remains unclear (Schubert et al., 2017). Studies on genetic polymorphisms related to EMB thus far have focused on polymorphisms that occur in the gene of Mtb bacteria (Zenteno-Cuevas et al., 2019). Only a few studies have explored gene polymorphisms in humans that have an impact on the clinical response to EMB, including resistance and toxicity.
In human genetic polymorphism studies of anti-TB, the majority focus on RIF or INH. These studies discussed polymorphisms related to clinical response and toxicity (Baskaran and Sabina, 2017; Bao et al., 2018; Richardson et al., 2018; Yang et al., 2019; Zenteno-Cuevas et al., 2019; Rivière et al., 2020). However, EMB has an important role in the treatment of tuberculosis, such as maintaining the effectiveness of therapeutic regimens (Horsburgh et al., 2015). In addition, the incidence of EMB toxicity is lower than that of other first-line anti-TB drugs, especially in comparison with INH. The toxicity is also thought to be related to polymorphisms in certain drug-metabolizing enzymes (DMEs) (Castro et al., 2015; Sarkar and Ganguly, 2016).
The relatively low toxicity and low incidence of resistance are the reasons why EMB is widely used in both sensitive and resistant TB. Personalized medicine practices are highly recommended to maintain and obtain the optimal therapeutic effect of EMB, but human studies of polymorphisms related to EMB clinical response, which is the basis for personalized medicine-based therapy, are scarce. Mapping out all the polymorphisms that have an impact on EMB efficacy, risk of toxicity, and risk of resistance can help us maintain the effectiveness of EMB as one of the TB drugs for sensitive and resistant TB. This article summarizes the genetic polymorphisms associated with alterations in the pharmacokinetic profile, resistance incidence, and susceptibility to EMB toxicity.
2 Materials and methods
This review summarizes the results of several studies related to the effects of polymorphisms on the EMB clinical response (effectivity, pharmacokinetics, resistance, and susceptibility to toxicity). It includes studies from the PubMed database identified using the keywords “genetic polymorphism” and “ethambutol”. Furthermore, research communications, thesis manuscript, reviews, expert opinions, non-English studies, and unrelated studies were excluded (Figure 1). The thesis manuscript that we have found is the manuscript who have not already been published, and article reviews that were excluded are articles in the form of narrative reviews or mini-reviews that do not contain statistically new conclusions.
A total of 131 reports of human studies were collected; among them, 91 studies of Mtb polymorphism, 11 review studies, 4 thesis publications, and 8 unrelated studies, which discuss genetic polymorphism related to susceptibility of TB, were excluded. Hence, a total of 17 articles were included in this study. Most of the articles discussed the effect of gene polymorphisms on the therapeutic outcome, i.e., effectiveness, resistance to EMB, and side effects or toxicity.
3 Result
3.1 Ethambutol
EMB (C10H24N2O2) is an ethylenediamine derivative used as the dihydrochloride salt. It is a white crystalline powder that is essentially odorless and has a bitter taste. It is sparingly soluble in water (Osol and Hoover, 1976). EMB was first discovered at Lederle Laboratories of the American Cynamid. The discovery of its remarkable stereospecific activity in mice was found in 1961 (Thomas et al., 1961; Shepherd et al., 1966).
3.1.1 Ethambutol and tuberculosis
Early biochemical studies found that EMB works by impairing glycerol metabolism as well as RNA synthesis only on bacilli (Kuck et al., 1962; Kuck et al., 1963; Kuck et al., 1965). Subsequent biochemical studies showed that EMB interferes with the biosynthesis of arabinogalactan, a major component of the bacterial cell wall. The polymerization of cell wall arabinan from arabinogalactan and lipoarabinomannan is inhibited by blockade of arabinosyl-transferases and enchance the accumulation of D-arabinofuranosyl-P-decaprenol, an intermediate in arabinan biosynthesis (National Center for Biotechnology Information, 2021). This results in halting bacterial growth. Genetically, the effect of EMB is related to interactions with three membrane-embedded arabinosyltransferase genes, EmbA, EmbB, and EmbC. Most of pharmacogenomics study on EMB discussing about these genes. EMB has a specific mechanism as a therapy for tuberculosis (Palomino and Martin, 2014; Lee and Nguyen, 2020; Zhang et al., 2020; National Center for Biotechnology Information, 2021). For DS-TB, EMB is used together with other first-line anti-TB drugs, RIF, INH, and PZA, and for drug-resistant (DR)-TB, it is used in combination with other second-line anti-TB drugs. For MDR TB and rifampicin-resistant tuberculosis (RR TB), it is recommended to use EMB with delamanid, PZA, imipenem-CILAStatin/meropenem, amikacin/streptomycin, ethionamide/prothionamide (Pto), and p-amino salicylic acid (Lee and Nguyen, 2020).
A previous study showed that EMB has a synergistic effect with INH against Mtb through a transcriptional repressor of the inhA gene, a target gene of INH that encodes a protein for cell wall integrity. The results indicated that EMB enhances INH sensitivity of the inhA gene and, as a result, might increase the killing effect and toxicity of INH (Zhu et al., 2019). The WHO updated treatment guidelines for DR TB in May 2016 that recommend use of the shorter MDR-TB regimen under specific conditions, including use of high-dose INH and EMB. The addition of high-dose INH or EMB (or both) strengthens the regimen. This new recommendation is expected to be more effective and provide more benefit for most MDR-TB patients worldwide [(WHO) World Health Organization, 2020b].
3.1.2 Pharmacokinetics and pharmacodynamics
Approximately 75%–80% of an orally administered dose of EMB (hydrochloride form) is absorbed in the gastrointestinal tract. Absorption is not substantially affected when the drug is administered with food. EMB is widely distributed in most body tissues and fluids. The highest concentrations of the drug are found in erythrocytes, kidneys, lungs, and saliva; lower drug concentrations are found in ascitic fluid, pleural fluid, brain, and Cerebrospinal Fluid (CSF). In tuberculosis meningitis, only 10%–50% of EMB may penetrate the meninges (Mc Evoy, 2007; United States Pharmacopeial Convention, 2007; World Health Organization, 2020a). The absorption and distribution profile of the drug is affected by many enzymes, and those that play a major role are transporter enzymes, such as the ATP-binding cassette (ABC transporter) and solute carrier family (SLC transporter) (Figure 2) (Parvez et al., 2017; Pontual et al., 2017).
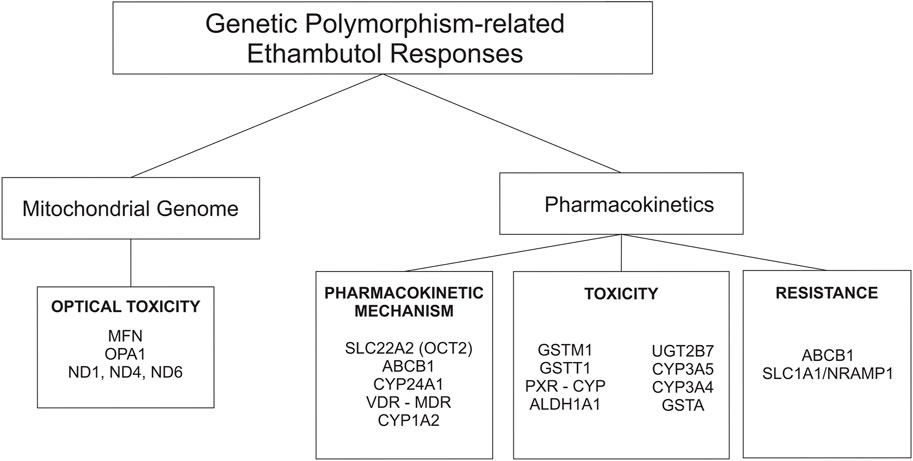
FIGURE 2. Genetic polymorphisms-related ethambutol responses on pharmacokinetic mechanism and mitochondrial genome.
Half of EMB is excreted unchanged in the urine, and a small part is excreted in the feces. An additional 15% changed to the form of metabolites. EMB is partially inactivated by oxidation to an aldehyde intermediate, which is converted to the decarboxylic acid derivative assisted by a metabolic enzyme called aldehyde dehydrogenase 1 (ALDH1) (Figure 2) (PEETS et al., 1965; Peng et al., 2021). Other drug-metabolizing enzymes (DMEs), such as cytochrome (CYP), also play a role in the metabolic rate of EMB. Based on a recent study, the AUC0-8 varied from 6.3 ± 5.5 h mg/L to 10.8 ± 7.6 h mg/L depending on CYP1A2 genetic polymorphisms (Sundell et al., 2020). In addition, the plasma half-life of EMB is approximately 3.3 h in patients with normal renal function. The half-life is prolonged in patients with impaired renal or hepatic function. In patients with renal failure, the half-life can be 7 hours or longer (Mc Evoy, 2007).
EMB is a concentration-dependent bactericidal, where ability to kill bacteria is linked to AUC/MIC. Decreasing the AUC could lead to decreased killing activity and increase the risk of treatment failure (Hall et al., 2012). Therefore, polymorphisms in genes that code for enzymes that play a role in drug pharmacokinetics, will also affect the drugs response. genetic polymorphisms that occur in genes encoding enzymes that play a role in pharmacokinetic processes in turn affect pharmacodynamics processes.
3.1.3 Toxicity and resistance of EMB
One of the most well-known and major adverse effects of EMB is optic neuropathy. It is dose-related, and 40% of adult patients develop optic neuropathy at doses greater than 50 mg/kg (Donald et al., 2006). Non-etheless, EMB is included in the category of TB drugs with mild adverse effects (World Health Organization, 2020b). A previous study conducted on 60 pulmonary TB patients treated with EMB resulted in significant changes in copper (Cu) levels. The study find that the chelating effect of EMB could leads to the decrease in serum levels of Cu as cationic trace elements. This increases the chelation of copper, which is related to the mechanism of EMB-induced optic neuropathy. Blood samples were obtained before treatment (baseline) and 10 days after starting anti-TB therapy, and the amounts of serum Cu were determined in all samples by atomic absorption (Abbasi Nazari et al., 2009). Another factor that could be a susceptible factor for optic neuropathy is genetic polymorphism of the human-mitochondrial gene. Mitochondrial genes encode proteins that play a role in cell survival and can decay as a result of alterations in genetic sequences, such as the presence of SNPs (De Marinis, 2001; Seo et al., 2010) (Figure 2). Apart from the major toxicity of EMB (reversible-optical nerve degradation), EMB may play a role in hepatotoxicity. Some studies found that EMB has adverse effects on hepatotoxicity, but others do not (Ramappa and Aithal, 2013). The fact that genetic polymorphism could increase the susceptibility of EMB toxicity, including hepatotoxicity, is known based on previous studies (De Marinis, 2001; Seo et al., 2010; Ramappa and Aithal, 2013; Fatiguso et al., 2016).
Genetic polymorphisms could be a potential marker of EMB resistance (Ramachandran and Swaminathan, 2012; McCallum and Sloan, 2017; Motta et al., 2018). EMB is added to the TB regimen as protection against unrecognized resistance to one of the three core drugs (Horsburgh et al., 2015). But, as mentioned before, the majority of studies found that the mechanism of resistance to EMB was linked to mutations in the gene embB with mutations at position embB306 (Sreevatsan et al., 1997; Telenti et al., 1997). The primary resistance rates of Mtb to EMB vary widely from 1% to 14% (Nasiri et al., 2016). Patients infected with Mtb strains showed simultaneous resistance to EMB and INH or EMB and RIF, which has been associated with an increased risk of treatment failure and further acquired resistance (Falzon et al., 2011).
Other possible mechanisms of drug resistance related to the host, human genetic polymorphisms, are SNP on the genes that play a role for pharmakokinetics and pharmacodynamic of EMB (Figure 2).
3.2 Pharmacogenomics
The diversity of drug responses was revealed with the completion of the Human Genome Projects in April 2003. A developing field called personalized medicine has adapted medical care, such as treatment decision-making, to the genetic background of individuals. Pharmacogenomics typically involves the search for variations in multiple genes that are associated with variability in drug response. Understanding genetics of an individual is the key to creating personalized drugs that will optimize therapy (National Human Genome Research Institute, 2018).
3.2.1 Genetic polymorphism related to pharmacokinetic mechanism
Genetic polymorphisms can change the pharmacokinetic profile of EMB, such as creatinine clearance, plasma concentration, and drug exposure, and result in the possibility of toxicity or drug resistance (Table 1). Genes that are included in this category are genes that encode ATP-binding cassette (ABC transporter), solute carrier family (SLC transporter), metabolizer enzymes; cytochrome (CYP), UDP-glucuronosyltransferase (UGT), aldehyde dehydrogenase (ALDH), glutathione S-transferase (GST), vitamin D receptor (VDR), pregnane X receptor (PXR), and genes related to optic neuropathy (Takahashi et al., 2008; Ramachandran and Swaminathan, 2012; Fatiguso et al., 2016; Pontual et al., 2017; Sun et al., 2019; Peng et al., 2021) (Figure 3).
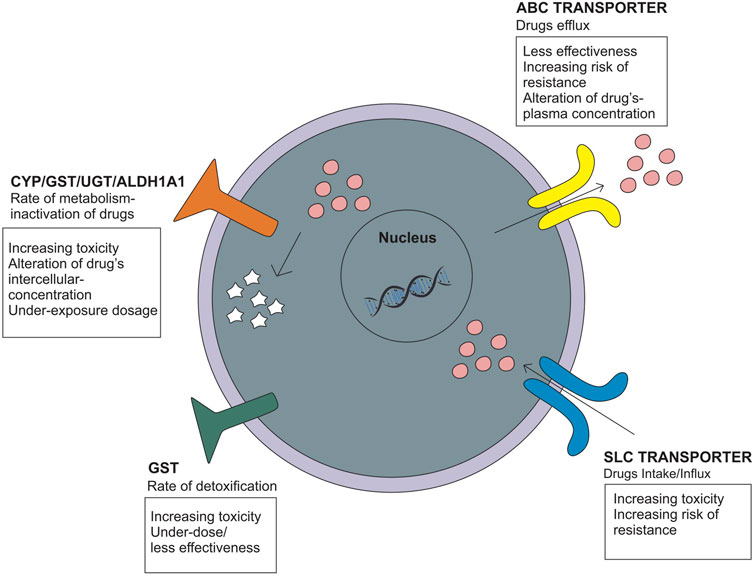
FIGURE 3. Genetic polymorphisms related to the pharmacokinetic mechanism that influences the drug intake/efflux and the rate of drug metabolism.
3.2.1.1 ABC transporter and SLC transporter
The ATP-binding cassette (ABC transporter) and solute carrier family (SLC transporter) genes encode protein transporters that play a role in the efflux and influx/uptake of drugs into cells (Figure 2). Polymorphisms of both genes may alter the activity or function of proteins and have an impact on drug concentration at the target site, leading to alterations in therapeutic efficacy or risk of resistance. Polymorphisms in ABC transporters, especially ABCB1 encoding P-glycoprotein (P-gp), a transmembrane drug efflux pump, may contribute to drug resistance and/or drug effectivity. The ABCB1 rs2032582 AA genotype is specifically associated with EMB resistance. A case–control study in Brazil showed that analyses for EMB resistance revealed an association of the rare allele “A” (OR = 12.91 p = 0.01) (Pontual et al., 2017). The wild-type genotype of ABCB1 is CC, which encodes P-gp and has a physiological function to remove toxic metabolites. Polymorphism in ABCB1 rs1045642 is associated with alteration of EMB plasma concentration with the result of homozygote mutant and heterozygote genotype (TT and CT) on week two and week four has a significant association with plasma concentration through p = 0.023 and p = 0.035, respectively (Fatiguso et al., 2016). Meanwhile, the SLC transporter is a transmembrane influx/uptake pump. Like ABC transporters, polymorphisms of SLC transporters may contribute to drug resistance and/or drug effectivity. An in vitro to in vivo extrapolation study showed that a genetic polymorphism in the SLC22A2 (OCT2) gene affected the EMB pharmacokinetics profile. Mutant genotypes reduced the activity of EMB transport and affect the drug clearance rate and AUC value (Parvez et al., 2017). Another study of SLC11A1/NRAMP1 (natural resistance-associated macrophage protein 1) in patients who used INH, PZA, RIF, EMB, kanamycin, and streptomycin showed that genetic variations in SLC11A1 may affect the incidence of MDR-TB (OR = 5.03 95% CI [1.24-20.62] p = 0.02 and OR = 5.03 CI95% [1.24-20.62] p = 0.02) and clinical features of pulmonary TB, which could be explained by a longer time to sputum culture conversion. EMB most likely contributed to these statistical results because G alleles are likely related to EMB resistance (Takahashi et al., 2008).
3.2.1.2 Cytochrome (CYP), UDP-glucuronosyltransferase (UGT), aldehyde dehydrogenase (ALDH), and glutathione S-transferase (GST)
Genes that encode metabolizer enzymes, such as CYP, GST, UGT, and ALDH, contribute to drug resistance and have been widely studied. Drugs metabolized for the two purposes; converting drugs into an active form and/or inactive drugs so that they can be excreted through the kidney. Changes in the function/activity of drug-metabolizing proteins will also affect the level of the active form of the drug at the target site (Susa and Preuss, 2021). Several studies have been performed to analyze the association between CYP polymorphisms and EMB responses. CYP24A1 rs2585428 is associated with EMB plasma and intracellular concentrations. The AG and GG genotypes of CYP24A1 rs2585428 had a significant association with the decrease of intracellular Ctrough of EMB (p = 0.03) (Fatiguso et al., 2016). Another study conducted in HIV-TB patients with the CYP1A2 rs2472304 polymorphism showed that the GA genotype was associated with a 50% reduction in EMB bioavailability. This SNP affects EMB exposure, and the treatment given may result in underexposure dosage (Sundell et al., 2020). Furthermore, GST polymorphisms are related to susceptibility to drug toxicity. GST are recognized as common detoxifying enzymes, playing an important protective role as they catalyze the conjugation of various reactive drug toxicity metabolites causing cellular damage with glutathione, thereby decreasing drug hepatotoxicity (Mitchell et al., 1973; Meister, 1983; Meister and Anderson, 1983; Andreoli et al., 1986; Li et al., 2013). The GSTM1 rs4025935 null genotype is associated with increased anti-tuberculosis drug-induced hepatotoxicity (ATDIH) (OR = 1.36 CI 95% [1.04-1.79]). Pooled analysis result null allele carriers had significant association with ATDIH risk for INH, RIF, PZA, and EMB regimen (OR = 1.47 CI 95% [1.14-1.9] p = 0.406) and INH, RIF, PZA, EMB, and streptomycin regimen (OR = 1.89 CI 95% [1.09-3.27] p = 0.076). However, there was no significant association in the dual therapy INH and RIF-only regimens (Li et al., 2013).
Aldehyde dehydrogenase 1 family member A1 (ALDH1A1) is the next enzyme after alcohol dehydrogenase in the major pathway of alcohol metabolism (Wu et al., 2020; NCBI, 2021). In addition to alcohol, many drugs can be metabolized by ALDHs, such as EMB, and its L-isomer is metabolized by liver ALDHs to form an aldehyde (oxidative) metabolite. The oxidation of aldehydes is generally considered a detoxification process, and a decrease in the rate of the mechanism could affect the risk of toxicity (Wu et al., 2020). The association between the ALDH1A1 polymorphism and incidence of anti-TB drug-induced liver injury (ATDILI) was investigated (Chang et al., 2018; Zhong et al., 2021). The results showed that rs7852860 variants in the ALDH1A1 gene are associated with susceptibility to ATDILI. The C allele and the CA genotype of rs7852860 were significantly associated with an elevated risk for ATDILI (p = 0.006 and 0.005, respectively). Unfortunately, there were limitations of the study in that it was only conducted on Chinese people with a small number of samples (Peng et al., 2021). Another gene related to the hepatotoxicity of EMB and the anti-TB regimen is UGT. Members of the UGT family probably make the largest contribution to phase II metabolism of drugs implicated in DILI, and it is relevant because of their role in detoxifying reactive metabolites. Loss of function of the UGT gene could increase the active drug level that cannot be excreted out of the body, thereby increasing the risk of toxicity (Daly, 2017). A study on UGT2B7 rs7662029 found that the AG genotype could be a protective factor against ATDILI (p = 0.00 OR = 0.389) (Sun et al., 2019).
3.2.1.3 Vitamin D receptor (VDR) and pregnane X receptor (PXR)
VDR, a ligand-activated transcription factor, controls gene expression. The active form of vitamin D, 25-hydroxyvitamin D, binds to VDR, controlling the synthesis of many different proteins (Abouzid et al., 2018). VDR binds directly to specific sequences located near promoters and recruits a variety of coregulatory complexes that perform the additional functions required to modify transcriptional output (Pike and Meyer, 2010). Transcriptional output alteration can influence the production of RNA, which encodes proteins that are integral to specific biological activities such as the degradation of xenobiotic compounds in several tissues (Bouillon et al., 2008; Carlberg and Campbell, 2013) and the functions of key cell types involved in both innate and adaptive immunity (Cutolo et al., 2011). Pregnane X receptor (PXR) is another ligand-activated nuclear receptor (NR) that mainly controls inducible expression of xenobiotic handling genes, including biotransformation enzymes and drug transporters (Pavek, 2016). Both VDR and PXR play major roles as transcription factors of several genes.
CYP3A4 is considered to be the most important member of the family of drug-metabolizing CYP450 enzymes, contributing importantly to the clearance of therapeutic agents (Guengerich, 1999). The induction of CYP3A4 gene expression is mainly regulated through activation of PXR (Lin, 2006; Wang et al., 2013) and it has been demonstrated that the active form of vitamin D3 (1α,25(OH)2D3) can also enhance the transcription of CYP3A4 by a VDR-mediated pathway (Wang et al., 2013).
A study on VDR BsmI (rs1544410), ApaI (rs7975232), and Cdx2 (rs11568820) suggested a possible role of that SNP in EMB plasma concentration and intracellular concentration (Fatiguso et al., 2016). However, a study on PXR rs2472677 showed that the PXR polymorphism has a significant correlation with INH exposure but not with EMB and other anti-TB agents (Calcagno et al., 2019).
3.2.2 Genetic polymorphism related to optic neuropathy
Mitochondrial function is influenced by several genes. Polymorphism or mutations in mitochondrial genes affect the ability of cells to survive, which in turn has an impact on nerve degeneration, one of which is the optic nerve (Table 2).
3.2.2.1 Mitofusion (MFN) and optic atrophy 1 (OPA1) protein
Mitochondria are cell organelles (mitochondrion, singular) that generate most of the chemical energy needed to power the biochemical reactions of cells (William Gahl, 2021). It plays an important role in cell function and survival (Sedlackova and Korolchuk, 2019). Mitochondrial fusion and fission are mechanisms that promote mitochondrial health and survival via the exchange of mitochondrial proteins, lipids, and genomes. Mitochondrial fusion is mediated by proteins called MFN1 and MFN2 on the outer mitochondrial membrane and OPA1 on the inner mitochondrial membrane (Liesa et al., 2009; Chen et al., 2011) (Figure 4). The MFN gene encodes the mitofusin protein, a protein that helps determine the shape and structure of mitochondria, the energy-producing centers organelle within cells (Chen, Liu and Dorn 2nd, 2011). OPA1 is involved in a process that takes place in mitochondria called oxidative phosphorylation, from which cells derive much of their energy, play a role in the maintenance of the DNA within mitochondria (mtDNA), and are also involved in apoptosis mechanisms (Zanna et al., 2008; Yu-Wai-Man et al., 2010). Loss of function of these mitochondrial fusion proteins (caused by mutations) could lead to degenerative neurological disease (Liesa et al., 2009), such as autosomal dominant optic atrophy (DOA), which is induced by OPA1 mutation (Yu-Wai-Man et al., 2010), and Charcot-Marie-Tooth (CMT), which has a subacute onset of optic atrophy associated with central scotoma and color vision defects (Guerriero et al., 2020).
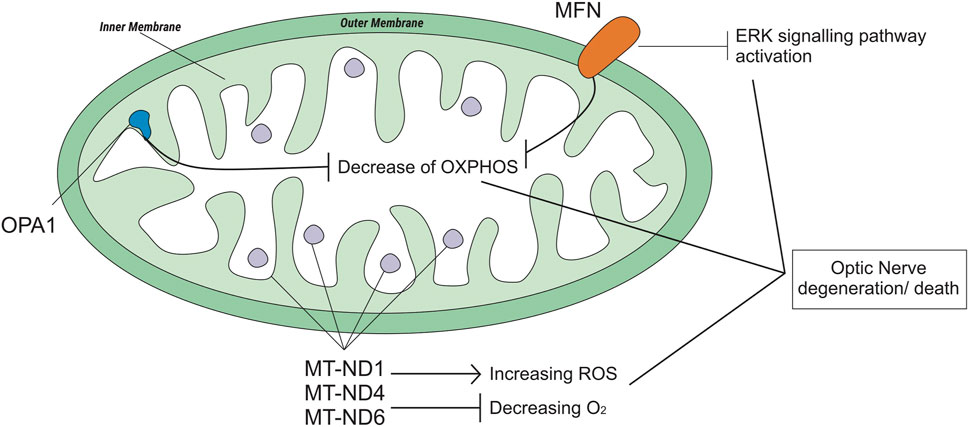
FIGURE 4. Genetic polymorphisms related to optic neuropathy which is regulated by the mitochondrial genome.
The most common form of axonal CMT (CMT2) is CMT2A2. A study on CMT2A2 cases showed that patients with MFN2 polymorphisms and possibly other mitochondrial fusion defects may be uniquely susceptible to EMB-induced neurotoxicity (Fonkem et al., 2013). Otherwise, a case study on the OPA1 rs143319805 polymorphism showed that the basal respiratory rate of the mutant was higher than that of the controls/wild type (Guillet et al., 2010).
3.2.2.2 NADH-ubiquinone oxyreductase core (ND)
The mitochondrial NADH-ubiquinone oxidoreductase core (MT-ND) encodes a NADH dehydrogenase protein, a part of a large enzyme complex known as complex I. MT-ND is activated in mitochondria and plays an important role in producing energy through a process called oxidative phosphorylation, which uses oxygen and simple sugars to create adenosine triphosphate (ATP), the main energy source of the cell. There are several types of MT-ND genes, MT-ND1, MT-ND4, MT-ND6, and polymorphisms in these genes lead to biochemical alterations (Lenaz et al., 2004). Mitochondrial complex I deficiency is also associated with a diverse range of clinical phenotypes (Ng et al., 2020), such as Leber hereditary optic neuropathy (LHON). LHON is a maternally inherited form of central vision loss/degeneration associated with mitochondrial DNA point mutations that affect the ND subunits of the complex (De Marinis, 2001; Lenaz et al., 2004; Seo et al., 2010). A case report from Korea stated that anti-TB medication, specifically EMB, could be a possible epigenetic factor of LHON (Seo et al., 2010). Another case report of subjects with LHON suggested that EMB could have acted as a trigger factor for LHON (De Marinis, 2001).
4 Discussion
From this study we got only eight studies (42.1%) that held in the LMIC, while the rest is research conducted in developed countries with high incomes. In contrast, globally more than 90% of reported tuberculosis infections occur in LMIC. Despite a cumulative reduction in global tuberculosis-related deaths, global progress is still far away from the targets set by the WHO on End TB Strategy and the United Nations on Sustainable Development Strategy. Goals on tuberculosis (SDG 3.3) (WHO, 2015; 2020; UN, 2022), efforts are needed to increase the optimization of tuberculosis therapy, especially in LMIC.
EMB is widely used in both sensitive TB and resistant TB (Lee and Nguyen, 2020) cases. A global systematic and meta-analysis stated that the resistance and the increasing risk of toxicity were a trend towards increase risk of MDR-TB (Pradipta et al., 2018). It is well known that resistance and risk to toxicity might be affected by the genetic polymorphism. Although only a few studies have explored the association between human genetic polymorphisms and EMB clinical response, we hypothesize that genetic polymorphisms influence the clinical response to EMB by two mechanisms: 1) alteration of the pharmacokinetic profile leads to ineffective therapy due to resistance and an increased risk of drug toxicity, and 2) alteration of the expression or activity of mitochondrial-related genes may lead to an increased risk of EMB optic toxicity. EMB is included in the category of TB drugs with mild adverse effects (World Health Organization, 2020a). The toxicity of EMB is mainly reversible optical nerve degradation, but as a regiment, EMB might also play a role in hepatotoxicity. Some studies have shown that EMB has a hepatotoxic effect, but others have not. It is dose-related; more than 40% of adults develop ototoxicity at doses greater than 50 mg/kg (Donald et al., 2006). EMB binds to TetR, a transcriptional regulator that enhances the INH sensitivity of the inhA gene and leads to increases in the killing effect of INH, thus increasing INH toxicity (Zhu et al., 2019). Genetic polymorphisms in drug metabolic enzyme-encoded genes, such as GST, CYP, and UGT, could affect the risk of EMB hepatotoxicity (Li et al., 2013; Richardson et al., 2018; Sun et al., 2019). Meta-analysis study which conducted on various middle-and high income countries prove that there are significant association between RsaI and 96-bp deletion-insertion SNPs of the CYP2E1 gene to the hepatotoxicity (Richardson et al., 2018). The results of a study on the GSTM1 gene polymorphism (null genotype) showed that the polymorphism was associated with hepatotoxic risk in the EMB-containing regimen, while the non-EMB-containing regimen showed insignificant results. In the GSTT1 gene polymorphism, the null genotype was associated with hepatotoxic risk in the regimen without EMB. However, previous studies have shown that EMB as monotherapy causes rare or no liver toxicity (Richardson et al., 2018). Another study from China resulted in the possibility that EMB induces/increases the risk of hepatotoxicity when used together with other anti-TB drugs. Unfortunately, the study was only conducted on Chinese people with a small number of samples (Peng et al., 2021). However, this information may add to the evidence that the toxicity of EMB to the liver needs to be considered.
For optical toxicity, the mitochondrial genome plays a role in the majority. Mitochondria, important cell organelles, generate most of the chemical energy needed to power the biochemical reactions of the cell (William Gahl, 2021). The health and survival of mitochondria are maintained through fission and fusion mechanisms, which are mediated by MFN1 and MFN2 on the outer mitochondrial membrane and OPA1 on the inner mitochondrial membrane (Liesa et al., 2009; Chen, Liu and Dorn 2nd, 2011). Genetic disorders due to mitochondrial dysfunction are not uncommon, and the majority of these patients will have eye-related manifestations, including visual loss from the optic nerve and retinal disease, that could be irreversible. Defects in mitochondrial genes such as MFN and OPA1 could cause mitochondrial dysfunction that leads to impaired mitochondrial energy production and oxidative stress (Yu-Wai-Man and Newman, 2017). In addition, mt-ND, which is a gene on mitochondrial DNA that functions to code for the NADH dehydrogenase protein, is predicted as an epigenetic factor of LHON in patients with a primary LHON mutation. Some case-report studies on Italy and Korea stated that they believe that their patient had ethambutol optic neuropathy. It cannot be excluded that the heteroplasmic DNA mutation of LHON may have predisposed the patient to toxic neuropathy (De Marinis, 2001; Seo et al., 2010). Several studies and case reports conducted in Italy, England, and United States of America have shown that the risk and susceptibility of ototoxicity-related EMB may be related to genetic polymorphisms of these genes (Guillet et al., 2010; Fonkem et al., 2013). Unfortunately, the authors have not found studies related to mt-DNA, MFN, and OPA1 conducted in LMIC. Hence, need further pharmacogenetic study on these genes in patients with EMB therapy in LMIC.
Genetic polymorphisms related to pharmacokinetic mechanisms could affect drug exposure and efficacy and lead to drug resistance. In brief, the pharmacokinetic mechanism is divided into four stages: absorption, distribution, metabolism, and excretion. Transporter proteins, such as ABC transporters and SLC transporters, work on the efflux and influx/uptake of drugs into cells. Polymorphism of the genes that encode these proteins affect the absorption, distribution, and excretion of the drugs (Parvez et al., 2017; Pontual et al., 2017). Genetic polymorphisms in DMEs, such as CYP, GST, and UGT, affect drug metabolism. All of these genes play a role in altering the pharmacokinetic rate and affect drug concentration exposure at the target site. Hence, it leads to under-treatment if the concentration is below the therapeutic dose or increases the risk of toxicity/adverse effects if the concentration is higher and enters the toxic dose (Fatiguso et al., 2016; Sundell et al., 2020). Long-term effects and under-treatment or non-optimal therapeutic doses increase the risk of drug resistance (Pontual et al., 2017). In addition, the rate of drug clearance, excretion, or detoxification could affect the increased risk of toxicity (Parvez et al., 2017; Richardson et al., 2018; Sun et al., 2019). Individual variations in the clinical response to therapy are known to be influenced by gene polymorphisms, so studies in this regard should be supported and developed. In addition, the practice of personalized medicine, which aims to minimize the rate of resistance, minimize the incidence of toxicity, and increase the effectiveness of treatment, should be recommended for all diseases, especially TB, given that the key to successful treatment of TB is optimal and effective treatment (World Health Organization, 2020b).
The articles included in this review were not limited in period of time due to the limited number of related studies. This review is subject to slight potential bias, including the influence of the personal viewpoints of the author, gaps in literature searching, and selection methods, which may lead to the omission of relevant research.
5 Conclusion
Genetic polymorphisms that occur related to the pharmacokinetics process could alter gene expression or its activities that alter drug concentration (decreased or increased). Therefore, this might be related to the treatment outcome (efficacy and safety/toxicity). Susceptibility to the optic toxicity of EMB could be affected by mitochondrial genetic polymorphism. Personalized medicine is an effort to provide individual therapy based on genetic profiles. Personalized medicine can provide a better and more effective treatment for TB that is efficacious, safe, and prevents drug resistance.
Author contributions
Conceptualization, MB; methodology, MB; writing—original draft, MB and NA; validation, VY; writing—review and editing; VY and RR.
Funding
This work was partially supported by Indonesian Ministry of Education, Culture, Research and Technology for MB and Grant-in Aids from Universitas Padjadjaran (Academic Leadership Grant) for RR.
Conflict of interest
The authors declare that the research was conducted in the absence of any commercial or financial relationships that could be construed as a potential conflict of interest.
Publisher’s note
All claims expressed in this article are solely those of the authors and do not necessarily represent those of their affiliated organizations, or those of the publisher, the editors and the reviewers. Any product that may be evaluated in this article, or claim that may be made by its manufacturer, is not guaranteed or endorsed by the publisher.
References
Abbasi Nazari, M., Kobarfard, F., Tabarsi, P., and Salamzadeh, J. (2009). Serum copper (cu) alterations in pulmonary tuberculosis patients under treatment with ethambutol. Biol. trace Elem. Res. 128 (2), 161–166. doi:10.1007/s12011-008-8267-8
Abouzid, M., Karazniewicz-Lada, M., and Glowka, F. (2018). Genetic determinants of vitamin D-related disorders; focus on vitamin D receptor. Curr. Drug Metab. 19 (12), 1042–1052. doi:10.2174/1389200219666180723143552
Andreoli, S. P., Mallett, C. P., and Bergstein, J. M. (1986). Role of glutathione in protecting endothelial cells against hydrogen peroxide oxidant injury. J. laboratory Clin. Med. 108 (3), 190–198.
Bao, Y., Ma, X., Rasmussen, T. P., and Zhong, X. B. (2018). Genetic variations associated with anti-tuberculosis drug-induced liver injury. Curr. Pharmacol. Rep. 4 (3), 171–181. doi:10.1007/s40495-018-0131-8
Baskaran, U. L., and Sabina, E. P. (2017). Clinical and experimental research in antituberculosis drug-induced hepatotoxicity: A review. J. Integr. Med. 15 (1), 27–36. doi:10.1016/S2095-4964(17)60319-4
Bouillon, R., Bischoff-Ferrari, H., and Willett, W. (2008). Vitamin D and health: Perspectives from mice and man. J. bone mineral Res. 23 (7), 974–979. the official journal of the American Society for Bone and Mineral Research. United States. doi:10.1359/jbmr.080420
Calcagno, A., Cusato, J., Sekaggya-Wiltshire, C., von Braun, A., Motta, I., Turyasingura, G., et al. (2019). The influence of pharmacogenetic variants in HIV/tuberculosis coinfected patients in Uganda in the SOUTH study. Clin. Pharmacol. Ther. 106 (2), 450–457. doi:10.1002/cpt.1403
Carlberg, C., and Campbell, M. J. (2013). Vitamin D receptor signaling mechanisms: Integrated actions of a well-defined transcription factor. Steroids 78 (2), 127–136. doi:10.1016/j.steroids.2012.10.019
Castro, A. T., Mendes, M., Freitas, S., and Roxo, P. C. (2015). Incidence and risk factors of major toxicity associated to first-line antituberculosis drugs for latent and active tuberculosis during a period of 10 years. Rev. Port. Pneumol. (English Ed. 21 (3), 144–150. doi:10.1016/j.rppnen.2014.08.004
Chang, T.-E., Huang, Y. S., Chang, C. H., Perng, C. L., Huang, Y. H., and Hou, M. C. (2018). The susceptibility of anti-tuberculosis drug-induced liver injury and chronic hepatitis C infection: A systematic review and meta-analysis. J. Chin. Med. Assoc. 81 (2), 111–118. doi:10.1016/j.jcma.2017.10.002
Chen, Y., Liu, Y., and Dorn, G. W. (2011). Mitochondrial fusion is essential for organelle function and cardiac homeostasis. Circulation Res. 109 (12), 1327–1331. doi:10.1161/CIRCRESAHA.111.258723
Cutolo, M., Plebani, M., Shoenfeld, Y., Adorini, L., and Tincani, A. (2011). “Chapter fourteen - vitamin D endocrine system and the immune response in rheumatic diseases,” in Vitamins and the immune system. Editor G. B. Litwack (Academic Press), 327–351. doi:10.1016/B978-0-12-386960-9.00014-9
Daly, A. K. (2017). Are polymorphisms in genes relevant to drug disposition predictors of susceptibility to drug-induced liver injury? Pharm. Res. 34 (8), 1564–1569. doi:10.1007/s11095-016-2091-1
De Marinis, M. (2001). Optic neuropathy after treatment with anti-tuberculous drugs in a subject with Leber's hereditary optic neuropathy mutation. J. Neural Transm. 108 (8–9), 818–819. doi:10.1007/s004150170103
Donald, P. R., Maher, D., Maritz, J. S., and Qazi, S. (2006). Ethambutol dosage for the treatment of children: Literature review and recommendations. Int. J. Tuberc. lung Dis. 10 (12), 1318–1330. the official journal of the International Union against Tuberculosis and Lung Disease. France.
Drummond, W. K., and Kasperbauer, S. H. (2019). Nontuberculous mycobacteria: Epidemiology and the impact on pulmonary and cardiac disease. Thorac. Surg. Clin. 29 (1), 59–64. doi:10.1016/j.thorsurg.2018.09.006
Esmail, H., Macpherson, L., Coussens, A. K., and Houben, R. M. G. J. (2022). Review Mind the gap À Managing tuberculosis across the disease spectrum. eBioMedicine 78, 103928. doi:10.1016/j.ebiom.2022.103928
Falkinham, J. O. (2015). Environmental sources of nontuberculous mycobacteria. Clin. chest Med. 36 (1), 35–41. doi:10.1016/j.ccm.2014.10.003
Falzon, D., Jaramillo, E., Schünemann, H. J., Arentz, M., Bauer, M., Bayona, J., et al. (2011). WHO guidelines for the programmatic management of drug-resistant tuberculosis: 2011 update. Eur. Respir. J. 38 (3), 516–528. doi:10.1183/09031936.00073611
Fatiguso, G., Allegra, S., Calcagno, A., Baietto, L., Motta, I., Favata, F., et al. (2016). Ethambutol plasma and intracellular pharmacokinetics: A pharmacogenetic study. Int. J. Pharm. 497 (1–2), 287–292. doi:10.1016/j.ijpharm.2015.11.044
Fonkem, E., Skordilis, M. A., Binkley, E. M., Raymer, D. S., Epstein, A., Arnold, W. D., et al. (2013). Ethambutol toxicity exacerbating the phenotype of CMT2A2. Muscle Nerve 48 (1), 140–144. doi:10.1002/mus.23766
Guengerich, F. P. (1999). Cytochrome P-450 3A4: Regulation and role in drug metabolism. Annu. Rev. Pharmacol. Toxicol. 39, 1–17. doi:10.1146/annurev.pharmtox.39.1.1
Guerriero, S., D'Oria, F., Rossetti, G., Favale, R. A., Zoccolella, S., Alessio, G., et al. (2020). CMT2A harboring mitofusin 2 mutation with optic nerve atrophy and normal visual acuity. Int. Med. Case Rep. J. 13, 41–45. doi:10.2147/IMCRJ.S237620
Guillet, V., Chevrollier, A., Cassereau, J., Letournel, F., Gueguen, N., Richard, L., et al. (2010). Ethambutol-induced optic neuropathy linked to OPA1 mutation and mitochondrial toxicity. Mitochondrion 10 (2), 115–124. doi:10.1016/j.mito.2009.11.004
Hall, R. G. 2nd, Swancutt, M. A., Meek, C., Leff, R. D., and Gumbo, T. (2012). Ethambutol pharmacokinetic variability is linked to body mass in overweight, obese, and extremely obese people. Antimicrob. agents Chemother. 56 (3), 1502–1507. doi:10.1128/AAC.05623-11
Horsburgh, C. R. J., Barry, C. E., and Lange, C. (2015). Treatment of tuberculosis. N. Engl. J. Med. 373 (22), 2149–2160. doi:10.1056/NEJMra1413919
Kuck, N. A., Peets, E. A., and Forbes, M. (1962). Mode of action of ethambutol. J. Bacteriol. 84 (5), 1099–1103. doi:10.1128/jb.84.5.1099-1103.1962
Kuck, N. A., Peets, E. A., and Forbes, M. (1963). Mode of action of ethambutol on Mycobacterium tuberculosis, strain H37R V. Am. Rev. Respir. Dis. 87, 905–906. doi:10.1164/arrd.1963.87.6.905
Kuck, N. A., Peets, E. A., and Forbes, M. (1965). Effect of ethambutol on nucleic acid metabolism in MYCOBACTERIUM smegmatis and its reversal by polyamines and divalent cations. J. Bacteriol. 89 (5), 1299–1305. doi:10.1128/jb.89.5.1299-1305.1965
Kurkó, J., Besenyei, T., Laki, J., Glant, T. T., Mikecz, K., and Szekanecz, Z. (2013). Genetics of rheumatoid arthritis - a comprehensive review. Clin. Rev. allergy & Immunol. 45 (2), 170–179. doi:10.1007/s12016-012-8346-7
Lander, E. S., Linton, L. M., Birren, B., Nusbaum, C., Zody, M. C., Baldwin, J., et al. (2001). Initial sequencing and analysis of the human genome. Nature 409 (6822), 860–921. doi:10.1038/35057062
Lee, N., and Nguyen, H. (2020). Ethambutol. Tampa, Florida: StatPearls. [Internet]. Available at: https://www.ncbi.nlm.nih.gov/books/NBK559050/.
Lenaz, G., Baracca, A., Carelli, V., D'Aurelio, M., Sgarbi, G., and Solaini, G. (2004). Bioenergetics of mitochondrial diseases associated with mtDNA mutations. Biochimica biophysica acta 1658 (1–2), 89–94. doi:10.1016/j.bbabio.2004.03.013
Li, C., Long, J., Hu, X., and Zhou, Y. (2013). GSTM1 and GSTT1 genetic polymorphisms and risk of anti-tuberculosis drug-induced hepatotoxicity: An updated meta-analysis. Eur. J. Clin. Microbiol. Infect. Dis. 32 (7), 859–868. doi:10.1007/s10096-013-1831-y
Liesa, M., Palacín, M., and Zorzano, A. (2009). Mitochondrial dynamics in mammalian health and disease. Physiol. Rev. 89 (3), 799–845. doi:10.1152/physrev.00030.2008
Lin, J. H. (2006). CYP induction-mediated drug interactions: In vitro assessment and clinical implications. Pharm. Res. 23 (6), 1089–1116. doi:10.1007/s11095-006-0277-7
G. Mc Evoy (Editor) (2007). American hospital formulary service. AHFS drug information (American Society of Health-System Pharmacists).
McCallum, A. D., and Sloan, D. J. (2017). The importance of clinical pharmacokinetic–pharmacodynamic studies in unraveling the determinants of early and late tuberculosis outcomes. Int. J. Pharmacokinet. 2 (3), 195–212. doi:10.4155/ipk-2017-0004
Meister, A., and Anderson, M. E. (1983). Glutathione. Annu. Rev. Biochem. 52, 711–760. doi:10.1146/annurev.bi.52.070183.003431
Meister, A. (1983). Selective modification of glutathione metabolism. Sci. (New York, N.Y.) 220 (4596), 472–477. doi:10.1126/science.6836290
Mitchell, J. R., Jollow, D. J., Potter, W. Z., Gillette, J. R., and Brodie, B. B. (1973). Acetaminophen-induced hepatic necrosis. IV. Protective role of glutathione. J. Pharmacol. Exp. Ther. 187 (1), 211–217.
Motta, I., Calcagno, A., and Bonora, S. (2018). Pharmacokinetics and pharmacogenetics of anti-tubercular drugs: A tool for treatment optimization? Expert Opin. Drug Metabolism Toxicol. 14 (1), 59–82. doi:10.1080/17425255.2018.1416093
Nasiri, M. J., Imani Fooladi, A. A., Dabiri, H., Pormohammad, A., Salimi Chirani, A., Dadashi, M., et al. (2016). Primary ethambutol resistance among Iranian pulmonary tuberculosis patients: A systematic review. Ther. Adv. Infect. Dis. 3 (5), 133–138. doi:10.1177/2049936116661962
National Center for Biotechnology Information (2021). Ethambutol, PubChem compound summary for CID 14052.
NCBI (2021). ALDH1A1 aldehyde dehydrogenase 1 family member A1 [ Homo sapiens (human) ]. Available at: https://www.ncbi.nlm.nih.gov/gene/216.
Ng, Y. S., Thompson, k., Loyer, D., Hopton, A., Falkous, G., Hardy, S. A., et al. (2020). Novel MT-ND gene variants causing adult-onset mitochondrial disease and isolated complex I deficiency. Front. Genet. 11, 24. doi:10.3389/fgene.2020.00024
Osol, A., and Hoover, J. E. (1976). Remington’s pharmaceutical sciences. 15th Editi. Mack Publishing Co. 15th Editi. John Wiley & Sons, Ltd. doi:10.1002/jps.2600650641
Palomino, J. C., and Martin, A. (2014). Drug resistance mechanisms in Mycobacterium tuberculosis. Antibiotics 3 (3), 317–340. doi:10.3390/antibiotics3030317
Parvez, M. M., Kaisar, N., Lee, Y., Shin, H., Jung, J., and Shin, J. G. (2017). Effect of organic cation transporter genetic polymorphism on ethambutol pharmacokinetics using physiologically based pharmacokinetic (PBPK) model. Clin. Ther. 39 (8), e48. doi:10.1016/j.clinthera.2017.05.149
Pavek, P. (2016). Pregnane X receptor (PXR)-Mediated gene repression and cross-talk of PXR with other nuclear receptors via coactivator interactions. Front. Pharmacol. 7, 456. doi:10.3389/fphar.2016.00456
Peets, E. A., Sweeney, W. M., Place, V. A., and Buyske, D. A. (1965). The absorption, excretion, and metabolic fate of ethambutol in man. Am. Rev. Respir. Dis. 91, 51–58. doi:10.1164/arrd.1965.91.1.51
Peng, W., Zhao, Z. Z., Jiao, L., Wu, T., Chen, H., Zhang, C. Y., et al. (2021). Prospective study of ALDH1A1 gene polymorphisms associated with antituberculosis drug-induced liver injury in Western Chinese Han population. Microbiol. Immunol. 65 (4), 143–153. doi:10.1111/1348-0421.12877
Pike, J. W., and Meyer, M. B. (2010). The vitamin D receptor: New paradigms for the regulation of gene expression by 1,25-dihydroxyvitamin D(3). Endocrinol. metabolism Clin. N. Am. 39 (2), 255–269. doi:10.1016/j.ecl.2010.02.007
Pontual, Y., Pacheco, V. S. S., Monteiro, S. P., Quintana, M. S. B., Costa, M. J. M., Rolla, V. C., et al. (2017). ABCB1 gene polymorphism associated with clinical factors can predict drug-resistant tuberculosis. Clin. Sci. 131 (15), 1831–1840. doi:10.1042/CS20170277
Pradipta, I. S., Forsman, L. D., Bruchfeld, J., Hak, E., and Alffenaar, J. W. (2018). Risk factors of multidrug-resistant tuberculosis: A global systematic review and meta-analysis. J. Infect. 77 (6), 469–478. doi:10.1016/j.jinf.2018.10.004
Ramachandran, G., and Swaminathan, S. (2012). Role-of-pharmacogenomics-in-the-treatment-of-tuberculosis-a review. Pharmgenomics Pers. Med. 5, 89–98. doi:10.2147/PGPM.S15454
Ramappa, V., and Aithal, G. P. (2013). Hepatotoxicity related to anti-tuberculosis drugs: Mechanisms and management. J. Clin. Exp. hepatology 3 (1), 37–49. doi:10.1016/j.jceh.2012.12.001
Richardson, M., Kirkham, J., Dwan, K., Sloan, D. J., Davies, G., and Jorgensen, A. L. (2018). CYP genetic variants and toxicity related to anti-tubercular agents: A systematic review and meta-analysis. Syst. Rev. 7 (1), 204. doi:10.1186/s13643-018-0861-z
Rivière, E., Whitfield, M. G., Nelen, J., Heupink, T. H., and Van Rie, A. (2020). Identifying isoniazid resistance markers to guide inclusion of high-dose isoniazid in tuberculosis treatment regimens. Clin. Microbiol. Infect. 26 (10), 1332–1337. doi:10.1016/j.cmi.2020.07.004
Sarkar, S., and Ganguly, A. (2016). Current overview of anti-tuberculosis drugs: Metabolism and toxicities. Mycobact. Dis. 6 (2). doi:10.4172/2161-1068.1000209
Schubert, K., Sieger, B., Meyer, F., Giacomelli, G., Böhm, K., Rieblinger, A., et al. (2017). The antituberculosis drug ethambutol selectively blocks apical growth in CMN group bacteria. mBio 8 (1), 1–21. doi:10.1128/mBio.02213-16
Sedlackova, L., and Korolchuk, V. I. (2019). Mitochondrial quality control as a key determinant of cell survival. Biochimica Biophysica Acta (BBA) - Mol. Cell. Res. 1866 (4), 575–587. doi:10.1016/j.bbamcr.2018.12.012
Seo, J. H., Hwang, J. M., and Park, S. S. (2010). Antituberculosis medication as a possible epigenetic factor of Leber’s hereditary optic neuropathy. Clin. Exp. Ophthalmol. 38 (4), 363–366. doi:10.1111/j.1442-9071.2010.02240.x
Shepherd, R. G., Baughn, C., Cantrall, M. L., Goodstein, B., Thomas, J. P., and Wilkinson, R. G. (1966). Structure-activity studies leading to ethambutol, a new type of antituberculous compound. Ann. N. Y. Acad. Sci. 135 (2), 686–710. doi:10.1111/j.1749-6632.1966.tb45516.x
Sreevatsan, S., Stockbauer, K. E., Pan, X., Kreiswirth, B. N., Moghazeh, S. L., Jacobs, W. R., et al. (1997). Ethambutol resistance in Mycobacterium tuberculosis: Critical role of embB mutations. Antimicrob. agents Chemother. 41 (8), 1677–1681. doi:10.1128/AAC.41.8.1677
Subramanian, G., Adams, M. D., Venter, J. C., and Broder, S. (2001). Implications of the human genome for understanding human biology and medicine. JAMA 286 (18), 2296–2307. doi:10.1001/jama.286.18.2296
Sun, S.-F., Ren, Q., Zheng, G., Han, T., and Feng, F. (2019). The relationship between metabolic enzyme genetic polymorphisms and anti-tuberculosis drug-induced hepatotoxicity. Int. J. Clin. Exp. Med. 12 (4), 4321–4329.
Sundell, J., Bienvenu, E., Birgersson, S., Äbelö, A., and Ashton, M. (2020). Population pharmacokinetics and pharmacogenetics of ethambutol in adult patients coinfected with tuberculosis and HIV. Antimicrob. agents Chemother. 64 (2), e01583–19. doi:10.1128/AAC.01583-19
Susa, S. T., and Preuss, C. V. (2021). Drug metabolism in StatPearls [internet] (Tampa, Florida: StatPearls Publishing). Available at: https://www.ncbi.nlm.nih.gov/books/NBK442023/.
Takahashi, K., Hasegawa, Y., Abe, T., Yamamoto, T., Nakashima, K., Imaizumi, K., et al. (2008). SLC11A1 (formerly NRAMP1) polymorphisms associated with multidrug-resistant tuberculosis. Tuberculosis 88 (1), 52–57. doi:10.1016/j.tube.2007.08.008
Telenti, A., Philipp, W. J., Sreevatsan, S., Bernasconi, C., Stockbauer, K. E., Wieles, B., et al. (1997). The emb operon, a gene cluster of Mycobacterium tuberculosis involved in resistance to ethambutol. Nat. Med. 3 (5), 567–570. doi:10.1038/nm0597-567
Teo, A. K. J., Singh, S. R., Prem, K., Hsu, L. Y., and Yi, S. (2021). Duration and determinants of delayed tuberculosis diagnosis and treatment in high-burden countries: A mixed-methods systematic review and meta-analysis. Respir. Res. 22 (1), 251. doi:10.1186/s12931-021-01841-6
Thomas, J. P., Baughn, C. O., Wilkinson, R. G., and Shepherd, R. G. (1961). A new synthetic compound with antituberculous activity in mice: Ethambutol (dextro-2,2’-(ethylenediimino)-di-l-butanol). Am. Rev. Respir. Dis. 83, 891–893. doi:10.1164/arrd.1961.83.6.891
UN (2022). Transforming our world: The 2030 agenda for sustainable development. Resolution adopted by the general assembly on 25 september 2015 [without reference to a main committee (A/70/L. 1)]. New York, US: UN.
United States Pharmacopeial Convention (2007). Micromedex-drug information for the health care professional, Vol. 1. Colorado, US: Greenwood Village.
Wang, Z., Schuetz, E. G., Xu, Y., and Thummel, K. E. (2013). Interplay between vitamin D and the drug metabolizing enzyme CYP3A4. J. steroid Biochem. Mol. Biol. 136, 54–58. doi:10.1016/j.jsbmb.2012.09.012
WHO (2015). Implementing the end TB strategy: The essentials. Geneva: WHO. World Health organization (WHO). WHO/HTM/TB/2015.31.
William Gahl (2021). Mitochondria, national human genome research Institute. Available at: https://www.genome.gov/genetics-glossary/Mitochondria.
Winburn, B., and Sharman, T. (2022). “Atypical mycobacterial disease,” in StatPearls [internet] (Tampa, Florida: StatPearls Publishing).
World Health Organization (2020a). WHO consolidated guidelines on tuberculosis. Module 4: Treatment - drug-resistant tuberculosis treatment. Geneva: WHO report. Online annexes.
World Health Organization (2020b). Consolidated guidelines on tuberculosis treatment. Geneve, Switzerland: WHO.
World Health Organization (2021). Fact-sheet tuberculosis. Geneve, Switzerland: WHO. Available at: https://www.who.int/news-room/fact-sheets/detail/tuberculosis.
Wu, B., Lu, D., and Dong, D. (2020). Circadian pharmacokinetics. Springer Nature. Available at: https://books.google.co.id/books?id=9voFEAAAQBAJ&dq=aldh1a1+ethambutol&source=gbs_navlinks_s.
Xiang, Q., Wu, W., Zhao, N., Li, C., Xu, J., Ma, L., et al. (2020). The influence of genetic polymorphisms in drug metabolism enzymes and transporters on the pharmacokinetics of different fluvastatin formulations. Asian J. Pharm. Sci. 15 (2), 264–272. doi:10.1016/j.ajps.2019.06.002
Yang, S., Hwang, S. J., Park, J. Y., Chung, E. K., and Lee, J. I. (2019). Association of genetic polymorphisms of CYP2E1, NAT2, GST and SLCO1B1 with the risk of anti-tuberculosis drug-induced liver injury: A systematic review and meta-analysis. BMJ Open 9 (8), e027940. doi:10.1136/bmjopen-2018-027940
Yu-Wai-Man, P., and Newman, N. J. (2017). Inherited eye-related disorders due to mitochondrial dysfunction. Hum. Mol. Genet. 26 (R1), R12–R20. doi:10.1093/hmg/ddx182
Yu-Wai-Man, P., Griffiths, P. G., Gorman, G. S., Lourenco, C. M., Wright, A. F., Auer-Grumbach, M., et al. (2010). Multi-system neurological disease is common in patients with OPA1 mutations. Brain a J. neurology 133 (3), 771–786. doi:10.1093/brain/awq007
Zanna, C., Ghelli, A., Porcelli, A. M., Karbowski, M., Youle, R. J., Schimpf, S., et al. (2008). OPA1 mutations associated with dominant optic atrophy impair oxidative phosphorylation and mitochondrial fusion. Brain a J. neurology 131 (2), 352–367. doi:10.1093/brain/awm335
Zastrozhin, M., Sychev, D., Baymeeva, N., Miroshnichenko, I., Grishina, E., and Bryun, E. (2018). Pharmacodynamics and pharmacokinetics related gene polymorphisms affect adverse drug reactions of haloperidol. Eur. Neuropsychopharmacol. 28, S4–S5. doi:10.1016/j.euroneuro.2017.12.021
Zenteno-Cuevas, R., Fernandez, E., Viveros, D., Mayo, C., Comas, I., Munoz, I., et al. (2019). Characterization of polymorphisms associated with multidrug-resistant tuberculosis by whole genomic sequencing: A preliminary report from Mexico. Microb. Drug Resist. 26 (7), 732–740. doi:10.1089/mdr.2019.0054
Zhang, L., Zhao, Y., Gao, Y., Wu, L., Gao, R., Zhang, Q., et al. (2020). Structures of cell wall arabinosyltransferases with the anti-tuberculosis drug ethambutol. Science 368 (6496), 1211–1219. doi:10.1126/science.aba9102
Zhong, T., Fan, Y., Dong, X. L., Guo, X., Wong, K. H., Wong, W. T., et al. (2021). An investigation of the risk factors associated with anti-tuberculosis drug-induced liver injury or abnormal liver functioning in 757 patients with pulmonary tuberculosis. Front. Pharmacol. 12, 708522–708529. doi:10.3389/fphar.2021.708522
Keywords: ethambutol, genetic polymorphism, personalized medicine, tuberculosis, metabolism
Citation: Barliana MI, Afifah NN, Yunivita V and Ruslami R (2023) Genetic polymorphism related to ethambutol outcomes and susceptibility to toxicity. Front. Genet. 14:1118102. doi: 10.3389/fgene.2023.1118102
Received: 07 December 2022; Accepted: 10 April 2023;
Published: 20 April 2023.
Edited by:
Raspail Carrel Founou Zangue, University of Dschang, CameroonReviewed by:
Maria Guadalupe Moreno Treviño, University of Monterrey, MexicoChristophe Orssaud, Assistance Publique Hopitaux de Paris, France
Copyright © 2023 Barliana, Afifah, Yunivita and Ruslami. This is an open-access article distributed under the terms of the Creative Commons Attribution License (CC BY). The use, distribution or reproduction in other forums is permitted, provided the original author(s) and the copyright owner(s) are credited and that the original publication in this journal is cited, in accordance with accepted academic practice. No use, distribution or reproduction is permitted which does not comply with these terms.
*Correspondence: Melisa Intan Barliana, bWVsaXNhLmJhcmxpYW5hQHVucGFkLmFjLmlk