- 1ICAR-Directorate of Poultry Research, Hyderabad, India
- 2EVA.4 Unit, Faculty of Forestry and Wood Sciences, Czech University of Life Sciences Prague, Prague, Czechia
Background: Native chickens are dispersed in a wide geographical range and have hereditary assets that are kept by farmers for various purposes. Mitochondrial DNA (mtDNA) is a widely utilized marker in molecular studies because of its quick advancement, matrilineal legacy, and simple molecular structure.
Method and Results: We performed NGS sequencing to investigate mitochondrial genomes and to evaluate the hereditary connections, diversity, and measure of gene stream estimation in Indian native chicken breeds and Red Jungle fowl. The chicken breeds were genotyped using the D-loop region and 23 haplotypes were identified. When compared to Indian native breeds, more haplotypes were identified in the NADH dehydrogenase subunits, Cytochrome c oxidase, Cytochrome b, ATP synthase subunit 6, and Ribosomal RNA genes. The phylogenetic examination indicated that the analyzed chicken breeds were divided into six significant clades, namely A, B, C, D, E, and F, of which the F clade indicated the domestication of chicken breeds in India. Additionally, our work affirmed that the Indian Red Jungle Fowl is the origin for both reference Red Jungle Fowl as well as all Indian breeds, which is reflected in the dendrogram as well as network analysis based on the whole mtDNA and D-loop region. Indian Red Jungle Fowl is distributed as an outgroup, suggesting that this ancestry was reciprocally monophyletic.
Conclusion: The mtDNA sequences of Indian native chickens provided novel insights into adaptation mechanisms and the significance of important mtDNA variations in understanding the maternal lineages of native birds.
Introduction
Modern-day chicken breeds mostly evolved from Red Jungle fowl (RJF), which is evident from archeological discoveries (Darwin, 1868; Beebe, 1918; Danforth, 1958; Morejohn, 1968; Fumihito et al., 1994). There are also some reports on the contribution of other Jungle fowl in evolving many breeds across the Globe (Eriksson et al., 2008; Lawal, 2017). However, according to the available data, it is unclear when and where the first domestication of chickens took place (Zeuner, 1963; Crawford, 1984; West and Zhou, 1988; Fumihito et al., 1996; Liu et al., 2006; Xiang et al., 2014; Peters et al., 2015). In early 3,200 BC, chicken domestication was observed in the Indus valley, and accepted as the epicenter of chicken domestication (Zeuner, 1963). Wild RJF can be found in the forests of South East Asia and India; when people domesticated the chicken and spread it to different parts of the world, the genome landscape of domestic chickens was molded by natural and artificial selection, bringing about a wide range of breeds and ecotypes (Liu et al., 2006; Stevens, 1991; Peterson and Brisbin, 1999; Silva et al., 2009; Gifford-Gonzalez and Hanotte, 2011; Mwacharo et al., 2011). The domestic chicken has broad phenotypic variations. However, the RJF lack the phenotypic variations that would be the result of domestication, for example, plumage color and other morphological characteristics, behavioral and production traits, adaptation to different agro-ecosystems, and rigid human selection for production as well as aesthetic qualities (Schütz et al., 2001; Keeling et al., 2004; Tixier-Boichard et al., 2011). Following domestication, large-scale breeding programs have resulted in more than sixty chicken breeds representing four particular genealogies: egg-type, game, meat-type, and bantamweight (Moiseyeva et al., 2003). According to the taxonomy, the genus Gallus is composed of four species: G. gallus (Red Jungle Fowl), G. lafayettei (Lafayette’s Jungle Fowl), G. varius (Green Jungle Fowl), and G. sonneratii (Grey Jungle Fowl). At present, RJF has five sub-species based on phenotypic traits and geographic distribution of the populations: G. g. gallus (South East Asia RJF), G. g. spadiceus, G. g. bankiva, G. g. murghi (Indian RJF) and G. g. jabouillei (Niu et al., 2002). The domestic chicken is considered either a subspecies of RJF (G. g. domesticus) or a separate species, G. domesticus. Scientists are concerned about the genetic integrity and conservation status of the wild RJF and those held in avicultural assortments. It was revealed that the domestic chicken is hybridized with the wild RJF, resulting in the erosion of the genetic purity of the wild birds (Peterson and Brisbin, 1999; Brisbin et al., 2002; Nishibori et al., 2005). Because the previous examinations depend on phenotypic characters, small samples were utilized for DNA investigations. Mitochondrial D-loop sequence phylogeny and nuclear gene analyses demonstrated conceivable hybridization between GJF-RJF/domestic birds (Nishibori et al., 2005). Based on these reports, the evaluation of the genetic uniqueness of Indian RJFs is significant for conservation and population studies.
In the study of chicken hereditary diversity, microsatellites have been effectively utilized (Karsli and Balcıoğlu, 2019). For examining the hereditary connections between chicken populations, hereditary assorted diversity estimates utilizing the exceptionally polymorphic variable number of tandem repeat loci have yielded reliable and precise data. Over the previous decade, the utilization of maternally inherited mitochondrial DNA (mtDNA), particularly its complete displacement-loop (D-loop) region, has expanded. To track genetic information about chicken ancestral breeds, demonstrating the phylogenetic relationship, genetic distance, and variability within and between populations, one of the most significant and remarkable molecular tools, the nucleotide sequence of the mitochondrial D-loop region, is used (Moore, 1995; Nishibori, 2004). The mtDNA or an explicit part of mtDNA (e.g. D-loop) sequencing gives precise data on evolution and hereditary diversity (Fumihito et al., 1994; Di Lorenzo et al., 2015). The D-loop region evolves much faster than different areas of the mtDNA and does not encode a protein. A variation study was done utilizing the mtDNA D-loop region and HVI domain of 397 bp fragments for 398 African native chickens from 12 countries; 12.59% polymorphic sites were discovered (Mobegi, 2006). Likewise, 25 individuals from six native Chinese chicken populations recorded polymorphic variation rates between the range of 7.05% and 5.54%, respectively (Niu et al., 2002; Liu et al., 2004). However, there are no reports on Indian native chickens at the mitochondrial genomic level. Examining native chickens at the mitochondrial genomic level can explain the mitochondrial genomic premise of their disparities and the particular attributes of indigenous chickens can be accurately investigated. The comprehension of phylogeography will clarify the demographic history, origin, and extension of chicken species. To defeat the issue of parallel mutations and lineage exchange between different populaces, network analysis has enhanced phylogenetic trees (Bandelt et al., 1999). India is a huge nation that contains a unique scope of altitudes and climates, meaning native chickens have an astounding genetic diversity. As indicated by ICAR-NBAGR, India has 19 indigenous breeds: Ankaleshwar, Aseel, Busra, Chittagong (Malay), Danki, Daothigir, Ghagus, Harringhata Black, Kadaknath, Kalasthi, Kashmir Favorolla, Miri, Nicobari, Punjab Brown, Tellichery, Mewari, Kaunayen chicken, Hansli, and Uttara (Mogilicherla et al., 2022). There is a need to characterize native chicken lines at the molecular level to enact protection and improvement activities to benefit the nation. With the extended focus on genetic preservation, remarkable alleles may be helpful when making choices to keep up with native varieties. Therefore, the current examination aims to assess the hereditary divergence between twenty-two native Asian breeds and seven native Indian chicken breeds (i.e. Aseel, Ghagus, Nicobari Brown, Tellicherry, Kadaknath, Haringhata Black, and Red Jungle Fowl) utilizing mtDNA NGS sequence data.
Materials and methods
Experimental birds and sample collection
The seven Indian native chicken breeds, namely Aseel, Ghagus, Nicobari brown, Kadaknath, Tellicherry, Haringhata black, and Red Jungle fowl, were studied. Blood samples of five female birds each of Aseel, Ghagus, Nicobari brown, Nicobari black, and Kadaknath were collected from the experimental farms of Directorate of Poultry Research, Hyderabad while samples of H. black and Red Jungle fowl were collected from the experimental farms of WBUAFS, West Bengal and CSKHPKVV, Palampur, respectively. The blood samples of Tellicherry were collected from the local farmers of Kerala state (Figure 1; Tables 1, 2). The blood samples of each breed (five individual birds) were pooled and stored at −80°C. The DNA was extracted from pooled blood samples according to the lab-standard phenol-chloroform extraction method (Sambrook and Russell, 2001). The experiment was approved by the Institute Animal Ethics Advisory Committee (IAEC) ICAR-Directorate of Poultry Research, Hyderabad, India.
Primer designing
Primer designing, library preparation, and sequencing were performed at Genotypic Technology’s Genomics facility. Eight primer pairs were designed to cover the entire mitochondrial genome. About 10 ng of DNA was taken for PCR to amplify explicit products 2–3 kb in size. All the products (five individual birds’ pooled DNA PCR samples) were checked using a 1% Agarose gel (Supplementary Figure S1). All products were pooled in equal amounts for sonication utilizing Covaris S220.
DNA template library preparation and sequencing by Ion PGM™ sequencer
Library Preparation was done following the Ion Torrent protocol outlined in the Ion plus fragment library kit (ThermoFisher Scientific, United States, # 4471252). Approximately 500 ng of fragmented and cleaned DNA was taken for library preparation. End-repair and adapter ligation was done and the samples were barcoded in this progression. The samples were cleaned utilizing AMPure XP beads. Samples were size-chosen utilizing a 2% low melting agarose gel. The gel-purified samples were amplified for the enrichment of adapter-ligated fragments as per the protocol. The amplified products were cleaned using AMPure XP beads and quantified using Qubit Fluorometer and then run on Bioanalyzer High sensitivity DNA Assay to assess the quality of the library. The purified libraries were then used to prepare clonally amplified templated Ion Sphere™ Particles (ISPs) for sequencing on an Ion PGM™ Chip to obtain the essential data coverage. Sequencing was performed on an Ion PGM™ sequencer at Genotypic Technology’s Genomics facility, in Bengaluru, India.
Quality control for reads and analysis
The samples were sequenced with Ion PGM Sequencer and analyzed with Torrent suite v 3.6. Once the base-calling was done, the raw reads underwent the in-built process of trimming and filtering to obtain only high-quality reads. Trimming was performed to evacuate any undesired base calls, adapter sequences, and lower-quality reads at the 3′end of the reads. Filtering at the next step removed reads judged to contain low-quality base calls. These two steps ensured the reads taken were of high quality; the clean reads were used for the subsequent analyses. The raw reads obtained were aligned to the reference Gallus gallus mitochondria (NC_001323.1) with the TMAP algorithm. The variants were detected using the inbuilt plugin Variant caller (V4.0) of TS.
Validation of mtDNA genes with gene-specific primers
The mtDNA gene-specific primers were designed using IDT oligo analyzer software (Table 3). The mtDNA genes were amplified using the Prima-96™ Thermal Cycler (HIMEDIA). PCR amplification was performed in a 25 μL volume containing 1 μL of DNA template, 2.5 μL of 10 × PCR buffer with Mg2+, 2.5 μL of dNTP mixture (2.5 mM each), 1.25 μL of each primer (10 μM), 0.5 μL of Taq DNA polymerase (5 U/μL), and 16 μL of nuclease-free water. The PCR conditions were as follows: 95°C for 10 min; followed by 35 cycles of 94°C for 30 s, 53/58°C for 30 s, and 72°C for 45 s; and a final extension at 72°C for 10 min. The PCR products were detected on 2% agarose gel electrophoresis.
Phylogenetic and molecular evolution analysis
To investigate the evolutionary relationships, a phylogenetic examination was performed using the total mitochondrial DNA and D-loop region sequences of seven native Indian chicken breeds along with twenty-two native Asian chicken breeds. Each of the sequence datasets was aligned by Clustal X and analyzed by neighbor-joining (N-J) in MEGA 10.0, and bootstrap analysis was performed with 1000 replications (Buehler and Baker, 2003; Thompson et al., 1997; Kumar et al., 2004). For building a neighbor-joining phylogenetic tree, Kimura’s two-parameters model was used for calculating the genetic distance of the haplotypes.
Haplotypes diversity
Network analysis was used for haplotype diversity illustrated using NETWORK 10.1 (Bandelt et al., 1999). In chickens, population indices include several segregation sites (S), number of haplotypes (H), haplotype diversity (Hd), and nucleotide diversity (π), determined by the mtDNA D-loop sequences’ diversity and elucidated by the sequence polymorphism and the content of genetic variability (Nei, 1987). The DnaSP software version 10.1 was used for analysis and the alignment gaps arising from a deletion event were excluded from the calculations (Rozas et al., 2003). Between two sequences, the average number of nucleotide differences per site, known as nucleotide diversity (π), was defined as π = n/(n −1) Σijxixjxij or π = Σπij/nc [n = number of DNA sequences examined; xi and xj = frequencies of the ith and jth type of DNA sequences; πij = proportion of nucleotides in the respective types of DNA sequences; and nc = total number of sequence comparisons] (Nei, 1987). According to the Nei formula, the average heterozygosity or haplotype diversity, ℎ, is defined [ℎ = 2n (1—Σxi2)/(2n—1); xi = frequency of haplotype and n = sample size] (Nei, 1987). The gene or haplotype frequencies were used for assessing the level of genetic differentiation among the population. The Fst and Nst significant tests by Arlequin software version 2.000 were used to explore the population’s genetic structure (Wright, 1951; Excoffier et al., 1992; Schneider et al., 2000).
Results
The proposed whole mtDNA sequence analysis was effectively used to characterize the seven indigenous Indian chickens along with twenty-two native Asian chickens. The blood samples were collected and mtDNA was extracted from seven Indian native chicken breeds: Aseel, Ghagus, Nicobari Brown, Tellichery, Kadaknath, Haringhata Black, and Red Jungle Fowl. We successfully amplified mtDNA using an amplification method and NGS sequencing was done using an Ion PGM™ sequencer at Genotypic Technology’s Genomics facility. We obtained 757073, 89859, 105503, 50617, 15764, 264309, and 36685 sequencing reads from seven Indian native chickens, respectively (Table 4; Supplementary Table S1). The assembled complete mitochondrial genomes for seven Indian native chickens were submitted to Genbank under accession numbers, KP211418.1, KP211419.1, KP211422.1, KP211424.1, KP211425.1, KP211420.1, and KP211423.1 for Aseel, Ghagus, Nicobari Brown, Tellichery, Kadaknath, Haringhata Black, and Indian Red Jungle Fowl, respectively.
The complete length of mtDNA of Indian local chickens was 16,775 bp. Like most vertebrates, it contains a common structure, including 2 rRNA genes, 22 tRNA genes, 12 protein-coding genes, and 1 D-loop region (Lin et al., 2016; Gu and Li, 2020). The four nucleotides’ (i.e., A, T, G, and C) overall composition of assessed mtDNA was 30.26%, 23.76%, 32.48%, and 13.50%, in the order G > A > T > C, respectively. The inception codons for all the coding proteins was ATG, aside from COX1 which is GTG (Table 5). The heavy (H) strand of mtDNA encoded all the mtDNA genes and the light (L) strand encoded four sorts of tRNA genes and ND6 genes. Every one of these genes had 15 spaces in the length of 2–227 bp and had 3 overlaps in the length of 1-8 bp. These genes had three sorts of termination codons, namely TAA, TAG, and TGA. The “T– – “ is the 5′terminal of the adjoining gene (Anderson et al., 1981). The lengths of the two rRNA genes were 976 bp and 1621 bp. Among 12 protein-coding genes, the longest one was the ND5 gene (1818 bp) and the most limited one was the ND4L gene (297 bp). As seen in other types of chicken, four tRNA genes were circulated in protein-coding genes, varying from 66 to 135 bp in size (Yu et al., 2016; Liu et al., 2016a; Liu et al., 2016b; Lin et al., 2019). The D-loop region was situated among ND6 and rRNA with a length of 1227 bp (Table 5; Supplementary Table S2).
Pattern of mtDNA D-Loop variability
The 1227 bp mtDNA D-loop fluctuation design uncovered large variations between nucleotides 235 and 1209. In seven local Indian chicken breeds, eight haplotypes were related to variation at 28 destinations, and 8.33% of them were polymorphic (Figure 2). The total arrangement uncovered exceptionally high changeability in the mtDNA D-loop region between 164–360 bases; this variation comprises 23.5% of the seven successions. This rate is incredibly high contrasted with the other local chicken varieties at 5.54%–7.05% (Niu et al., 2002; Liu et al., 2004). This high pace of mtDNA D-loop variation might be credited to the relocation of birds all through the nation and diverse topographical districts in India. The base composition of the native Indian chicken breeds mtDNA D-loop shows that A+T grouping content was 60.39% while G+C was 39.61% (Ruokonen and Kvist, 2002).
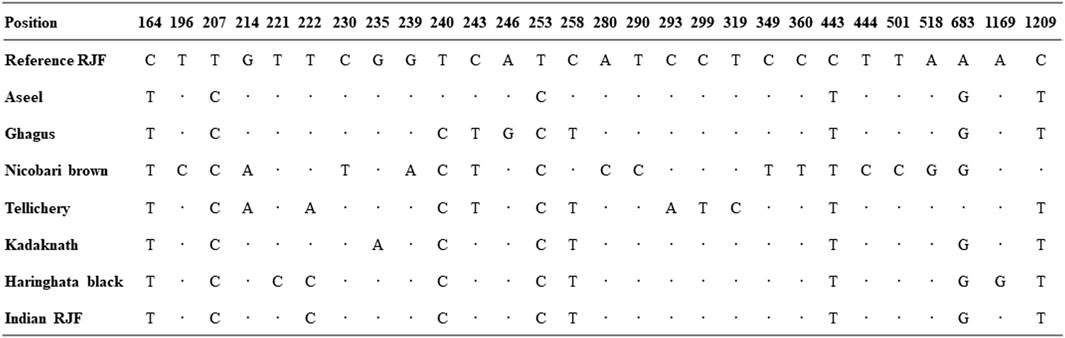
FIGURE 2. Pattern of mtDNA D-loop variability. Nucleotide polymorphisms were observed in the D-loop region of seven native Indian chicken sequences. Vertically oriented numbers indicate the site position and the sequences shown are only the variable sites. Dots (.) indicate identity with the reference sequence and different base letters denote substitution.
Sequence variation and haplotype distribution
Multiple sequence alignment was performed for seven native Indian chicken varieties and recognized eight haplotypes. The alignment of D-loop sequences was finished with a gallus reference sequence (NC_001323.1) utilizing Clustal-X. The accompanying domains and motifs were examined at the 5′end of the D-loop. Two units of invariant tetradecamer 5′- AACTATGAATGGTT-3′ were distinguished at positions 264 to 277 and 325 to 338. An interfered with thymine string (TTTTATTTTTTAA) was observed as conserved in all the individuals contemplated. There was likewise an intrusion of poly-C sequence (5′- CCCCCCCTTTCCCC-3′), which is generally conserved, and downstream to this there was a preserved sequence known as poly-G (5′-AGGGGGGGT-3′). Two moderated 5′- TACAT-3′ and 5′- TATAT-3′ were likewise found in all individuals. There were nine TATAT motifs and four TACAT found inside the D-loop and were, thus, conserved. The initial 163 base sets adjoining tRNA Glu were seen as exceptionally conserved in all individuals. The nucleotide replacements found in the nine variable haplotypes contained one G/T and two C/A transversions and the rest were all changes of which four were A/G transitions and seven were C/T transitions. This exhibits a solid predisposition towards transition. The C/T substitutions are more normal than the A/G substitution (Table 6).
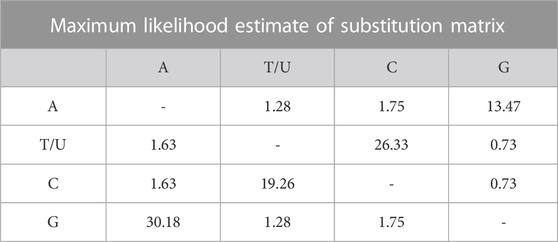
TABLE 6. Each entry is the probability of substitution (r) from one base (row) to another base (column). Substitution pattern and rates were estimated under the Tamura and Nei. (1993) model (Tamura and Nei, 1993). Rates of different transitional substitutions are shown in bold and those of transversionsal substitutions are shown in italics. Relative values of instantaneous r should be considered when evaluating them. For simplicity, sum of r values is made equal to 100, The nucleotide frequencies are A = 30.26%, T/U = 23.76%, C = 32.48%, and G = 13.50%. For estimating ML values, a tree topology was automatically computed. The maximum Log likelihood for this computation was −23504.716. This analysis involved 9 nucleotide sequences. Codon positions included were 1st + 2nd + 3rd + Noncoding. There were a total of 16775 positions in the final dataset. Evolutionary analyses were conducted in MEGA X (Kumar et al., 2018).
Genetic distance among seven native Indian chicken breeds
The genetic distances within and between the seven native Indian chicken breeds were analyzed using the DnaSP program 10.1 (Table 7). The genetic distance within the seven chicken breeds was 0.000655–16.173895. Indian RJF chickens had the most noteworthy within-breed genetic distance, while Ghagus chickens had the least. Between the breeds, the genetic distance estimates went from 0.000655 to 16.188613. The genetic distance between the breeds was most noteworthy for Indian RJF and Tellicherry chickens, while it was least for Ghagus and Aseel chickens. An incredible number of variants and SNPs were recognized in Nicobari Brown and a minimal number of variants and SNPs were distinguished in Tellicherry and Kadaknath, respectively. The most number of INDELs were distinguished in Kadaknath and the least number of INDELs were recognized in Tellicherry (Table 8).
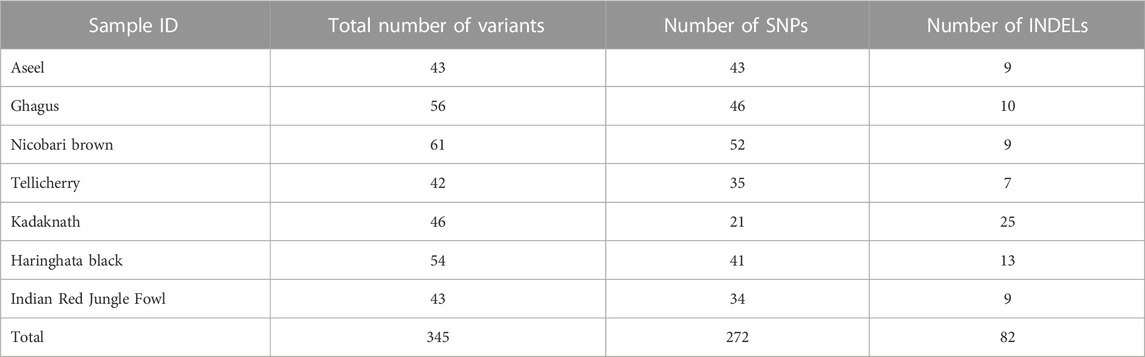
TABLE 8. The number of variants, SNPs and INDELs identified in seven Indian chicken mitochondrial genome.
Phylogenetic analysis of the haplotypes
An N-J tree indicated that the examined Asian native breeds and Indian local varieties were separated into six significant clades: A, B, C, D, E, and F. The native Indian breeds are situated at the base of the tree (Figure 3). This N-J tree produced from the total mitochondrial DNA of twenty-two native Asian breeds and seven local Indian varieties has comparative geographies. The clades A to E shared the native Asian breeds but clade F shared only native Indian breeds. Along these lines, our outcomes affirmed that Kadaknath-H. black and Aseel-Ghagus breeds have a nearby hereditary relationship. In addition, the N-J tree is generated from the NADH dehydrogenase subunit genes, cytochrome c oxidase subunit genes, mitochondrial encoded ATP synthase membrane subunit 6 gene, cytochrome b gene, and ribosomal RNA genes. The results showed a close hereditary relationship with Aseel-Ghagus and Nicobari brown-Reference RJF (Figures 5–8).
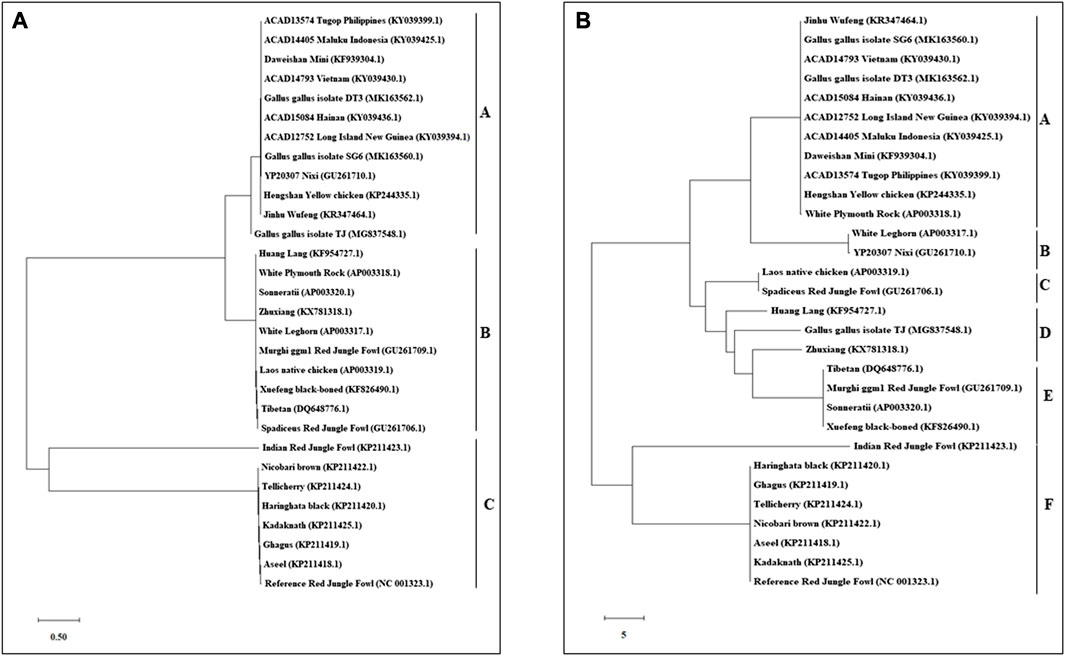
FIGURE 3. An N-J tree was constructed using MEGA 10.1 software. Phylogenetic analysis based on mtDNA D-loop region (A) and complete mtDNA genome sequences (B) of seven Indian native breads along with reference RJF mtDNA and twenty-two native Asian breeds sequences. The mtDNA genome sequences were obtained from the NGS sequencing and submitted in NCBI (Accession numbers: Aseel-KP211418.1; Ghagus-KP211419.1; Haringhata Black-KP211420.1; Kadaknath-KP211425.1; Nicobari Brown-KP211422.1; Indian Red Jungle Fowl-KP211423.1; Tellichery-KP211424.1). The numbers at the nodes represent the percentage bootstrap values for interior branches after 1000 replications.
Network analysis
Median-joining networks were drawn for the eight haplotypes identified from the seven Indian native chickens along with reference RJF mtDNA, based on the variable characters of the complete alignment using the computer program NETWORK 10.1 (Bandelt et al., 1999). The results showed that the mtDNA sequence of Indian Red Jungle Fowl has the highest frequencies and this haplotype is connected to the frequencies of other haplotypes, forming star-like connections. It was also observed that there are mutational links to eight haplotypes with five median vectors (mv)∗ separating clades (Figure 4). The median-joining network examination was completed with the haplotypes from the native Indian breeds. The outcomes show that, out of the seven recognized native breeds haplotypes, just two breeds haplotypes i.e. Kadaknath and Haringhata black, showed uniqueness and fell into a different clade separated from other breeds. Also, median-joining networks were drawn for mtDNA structural genes, such as NADH dehydrogenase subunit genes, cytochrome c oxidase subunit genes, mitochondrial encoded ATP synthase membrane subunit 6 gene, cytochrome b gene, and ribosomal RNA genes. The results showed that three breeds of haplotype i.e. Indian RJF, Kadaknath, and H. black, have uniqueness (Figures 5–8).
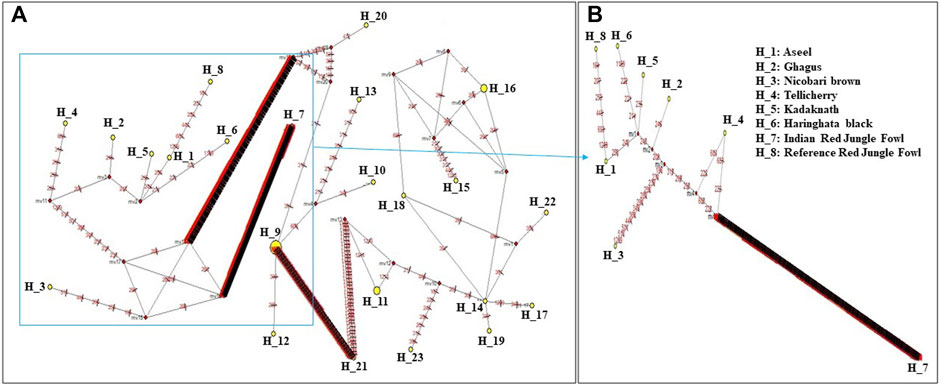
FIGURE 4. A median-joining network was produced by the network software 10.1 for eight haplotypes of native Indian chicken breeds along with reference mtDNA based on the polymorphic site of the mtDNA D-loop region (A) and complete mtDNA sequences (B). The area of each yellow circle is proportional to the frequency of the corresponding haplotype. The pink dots illustrate median vectors (mv) and the numbers on each link line represent mutated positions.
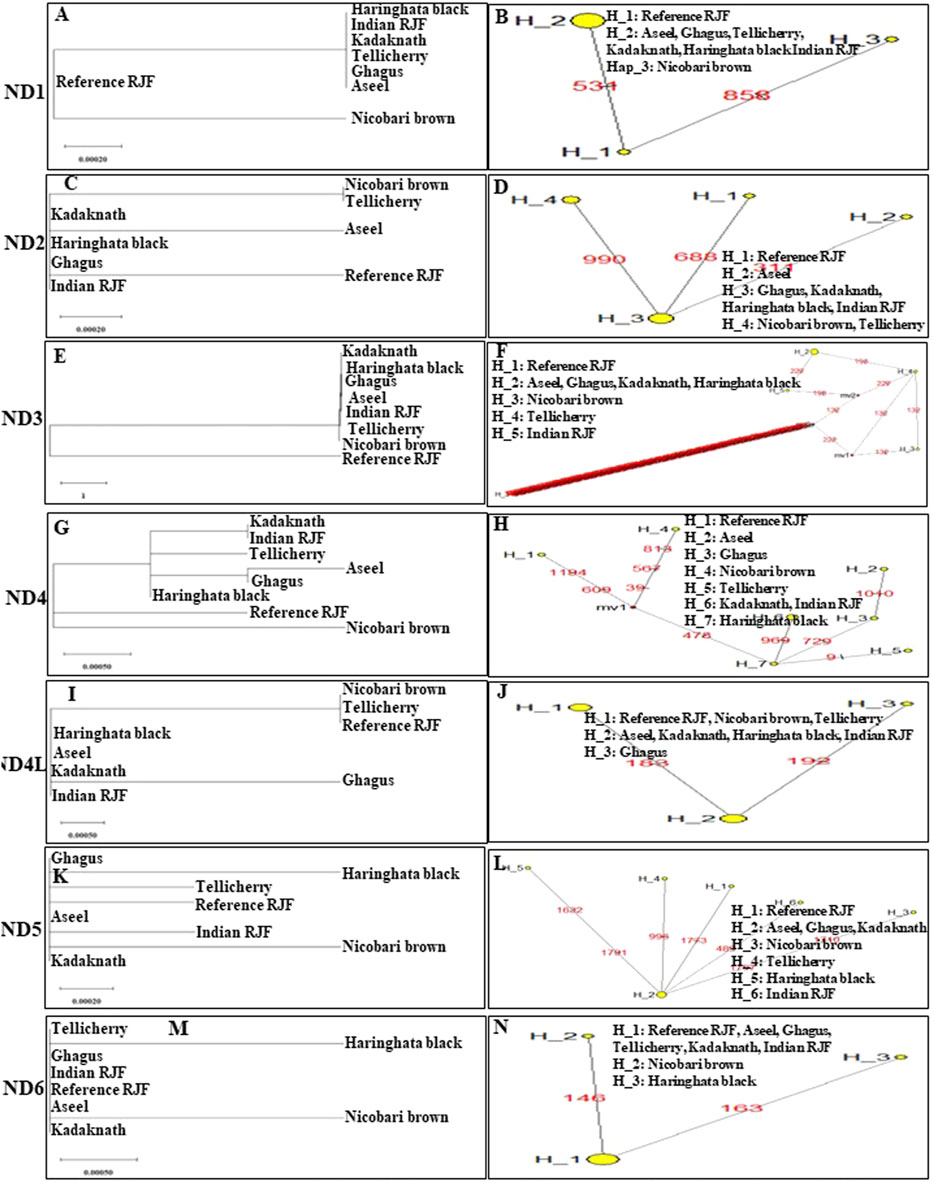
FIGURE 5. An N-J tree was constructed using MEGA 10.1 software. Phylogenetic analysis (A, C, E, G, I, K, and M) based on mtDNA NADH dehydrogenase subunit gene sequences of seven native Indian breeds along with reference RJF. The numbers at the nodes represent the percentage of bootstrap values for interior branches after 1,000 replications. A median-joining network was produced by the network software 10.1 for Indian native chicken breeds along with reference mtDNA based on polymorphic site of the mtDNA NADH dehydrogenase genes (B, D, F, H, J, L, and N). The area of each yellow circle is proportional to the frequency of the corresponding haplotype. The pink dots illustrate median vectors (mv) and the numbers on each link line represent mutated positions.
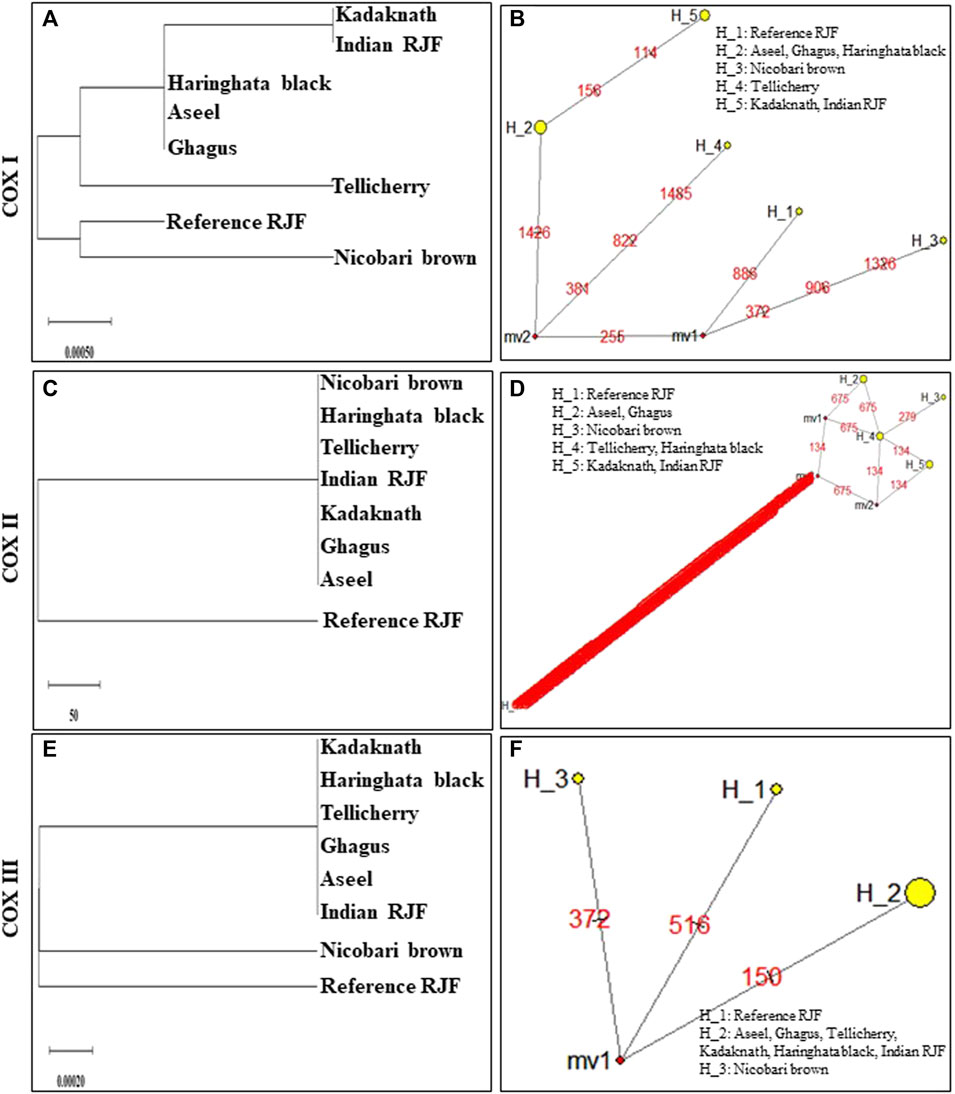
FIGURE 6. An N-J tree was constructed using MEGA 10.1 software. Phylogenetic analysis (A,C,E) based on mtDNA Cytochrome c oxidase subunit gene sequences of seven native Indian breeds along with reference RJF. The numbers at the nodes represent the percentage of bootstrap values for interior branches after 1000 replications. A median-joining network was produced by the network software 10.1 for native Indian chicken breeds along with reference mtDNA based on the polymorphic site of the mtDNA Cytochrome c oxidase subunit genes (B,D,F). The area of each yellow circle is proportional to the frequency of the corresponding haplotype. The pink dots illustrate median vectors (mv) and the numbers on each link line represent mutated positions.
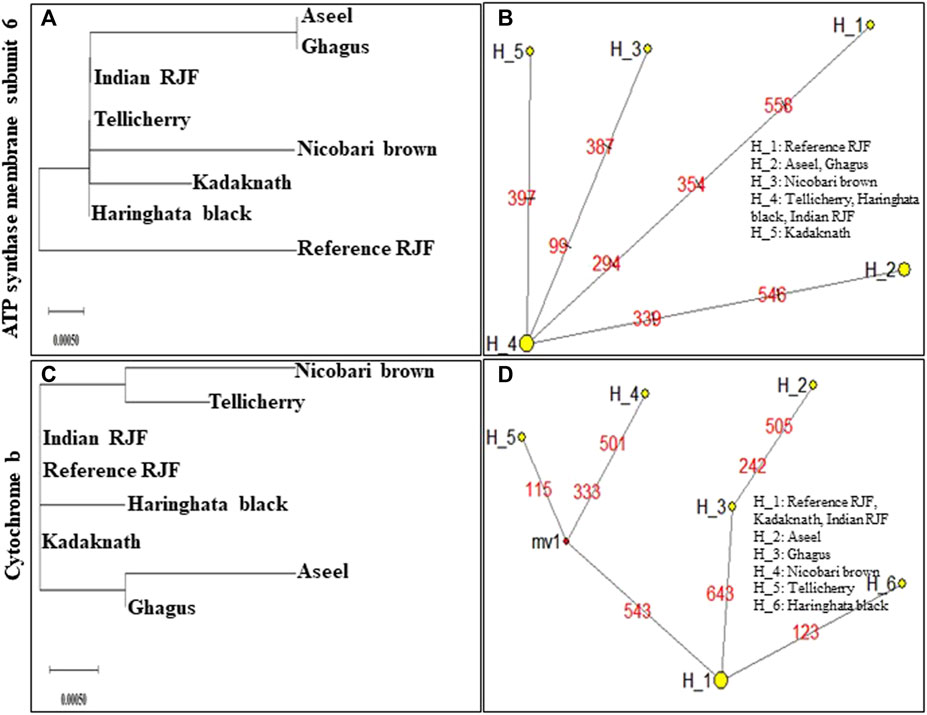
FIGURE 7. An N-J tree was constructed using MEGA 10.1 software. Phylogenetic analysis (A,C) based on mtDNA ATP synthase membrane subunit 6 and Cytochrome b gene sequences of seven native Indian breeds along with reference RJF. The numbers at the nodes represent the percentage of bootstrap values for interior branch after 1000 replications. A median-joining network was produced by the network software 10.1 for native Indian chicken breeds along with reference mtDNA based on the polymorphic site of the mtDNA ATP synthase membrane subunit 6 and Cytochrome b genes (B,D). The area of each yellow circle is proportional to the frequency of the corresponding haplotype. The pink dots illustrate median vectors (mv) and the numbers on each link line represent mutated positions.
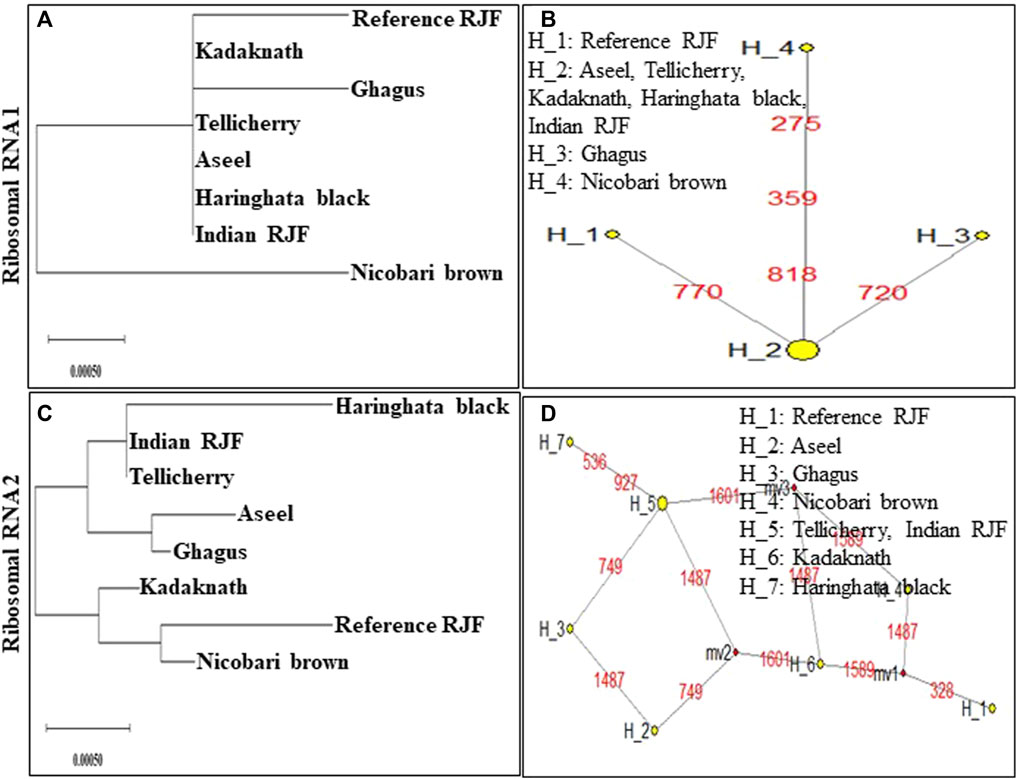
FIGURE 8. An N-J tree was constructed using MEGA 10.1 software. Phylogenetic analysis (A,C) based on mtDNA Ribosomal RNA 1 and 2 gene sequences of seven native Indian breeds along with reference RJF. The numbers at the nodes represent the percentage of bootstrap values for interior branches after 1000 replications. A median-joining network was produced by the network software 10.1 for native Indian chicken breeds along with reference mtDNA based on the polymorphic site of the mtDNA Ribosomal RNA 1 and 2 genes (B,D). The area of each yellow circle is proportional to the frequency of the corresponding haplotype. The pink dots illustrate median vectors (mv) and the numbers on each link line represent mutated positions.
The mtDNA genes validation
Genomic DNA was extracted from blood samples of seven native Indian chicken breeds and mtDNA genes were validated by PCR using gene-specific primers. PCR was able to amplify the segmental mtDNA region from all seven native breeds. Each expected PCR-amplified product demonstrated the sign band of approximately 67–482 bp in length on 2% agarose gel (Table 3; Supplementary Figures S2, S3). The Aseel-specific ND4 primers (12476–12796 region) amplified all the breeds except Ghagus, whereas Aseel rRNA-specific primers (3916–4253 region) amplified all the breeds except Ghagus and H. black. The Ghagus-specific ND4 primers (11366–11530 region) amplified Indian RJF, Nicobari, Kadaknath, and Ghagus but did not amplify Aseel, H. black, and Tellicherry, whereas Ghagus rRNA-specific primers (1996–2157 region) amplified all the breeds except Tellicherry. The Kadaknath COX1 specific primers (8050–8341 region) amplified all the breeds except Tellicherry.
Discussion
It is hypothesized that chickens (Gallus gallus domesticus) were domesticated from wild jungle fowls in Southeast Asia approximately 10,000 years ago (Ahmed et al., 2017). Native fowl extinction can be improved by modifying cultivation, taking care of the chickens, and better prosperity spread. However, selection and crossbreeding can be used for genetic improvement. Aside from conventional breeding, molecular breeding techniques (Microsatellite markers and SNPs), functional genomics, gene silencing, and genome editing techniques can be used to improve the quality of traits in chickens. Microsatellite markers, SSCP, and sequencing techniques can be used to distinguish proof of polymorphism on genes and assessment of the impact of polymorphism on growth traits in chickens. Molecular genetics has given a few useful assets to exploit the extraordinary abundance of polymorphism at the DNA level, for example, DNA-based genetic markers. The advantage of mtDNA is that it is present in higher copy numbers within the cells and thus can be recovered even from highly degraded specimens (Fumihito et al., 1996). For species identification, forensic studies, and anthropological and evolutionary research, the mitochondrial genome SNPs are assuming a significant role (Yacoub and Fathi, 2013). In 2016, single nucleotide polymorphism was observed, and eleven SNPs in the D-loop region of chicken mitochondrial DNA were reported (Jia et al., 2016).
To set up a phylogenetic relationship, studies were conducted on domestic fowl, different RJF subspecies, and other jungle fowls. In 1994, an investigation proposed that G.g. gallus is a major or sole contributor to a single domestication event, and three further species of RJF mtDNA D-loop region sequences were taken for phylogenetic analysis. This proved G.g. gallus is the real matriarchic origin of all the domestic poultry (Fumihito et al., 1994; Fumihito et al., 1996). In 2009, two ribosomal genes (12S rRNA and 16S rRNA) were used to study the genetic divergence between Indian RJF (G.g. murghi), Gallus gallus subspecies (G.g. spadicus, G.g. gallus, and G.g. bankiva) including G.g. domesticus (domestic fowl), and three other Gallus species (G. varius, G. lafayetei, and G. sonneratii) and found that, compared to other jungle fowls, G.g. murghi was closer to Gallus gallus subspecies, however between Indian RJF and other RJF subspecies, the divergence was very low (Gupta et al., 2009). Furthermore, the study found no noticeable contrasts between G.g. spadicus and G.g. gallus yet G.g. bankiva showed contrasts with both the genetic and phylogenetic relationships among these species (Ulfah et al., 2016). Recent studies confirmed multiple domestications of Indian and other domestic chickens and provided evidence for the domestication of Indian birds from G.g. spadiceus, G.g. gallus, and G.g. murghi (Kanginakudru et al., 2008; Mekchay et al., 2014; Maw et al., 2015). These studies avoided the two different subspecies of red jungle fowl i.e. G.g. murghi and G.g. jabouillei, however, they provided a framework for genetic studies in wild jungle fowls and native and domestic chicken breeds. Considering solid confirmations of the domestication of chicken in the Indus valley, it might be interesting to contemplate the genetic relatedness between Indian RJF and other RJF subspecies including G.g. domesticus and other jungle fowls (Kawabe et al., 2014).
Indian RJFs are widely distributed across 51 × 105 km2 in 21 states of India (Fernandes et al., 2009; Mogilicherla et al., 2022) (Figure 1). We were more interested to explore the phylogenetic relationship of Indian RJF with reference RJF and native Asian breeds along with other native Indian breeds and understand the contribution of Indian RJF, G. g. murghi, to the domestication event. Hence, in the present study, seven native Indian breeds (Aseel, Ghagus, Nicobari brown, Kadaknath, Tellicherry, H. black, and Indian Red Jungle fowl) and twenty-two native Asian breeds were used to study the nucleotide sequence variation in complete mtDNA and the most variable region of mtDNA D-loop region to build up the phylogenetic relationship among the Indian breeds (Table 1; Table 2). Currently, no sequence data is accessible from these seven native Indian breeds. However, one of these breeds is likely to have been a contributor to one of the earliest known chicken domestication events, for example in the Mohanjo-Daro Indus valley.
The preserved traits like Struthioniformes, Falconiformes, and Sphenisciformes have been depicted across a variety of avian species (Haring et al., 2001; Wani et al., 2014). The Indian indigenous chicken D-loop area sequence has cytosines and guanine strings close to each other, making the advancement of a consistent hairpin structure possible (Quinn and Wilson, 1993). In all neighborhood chickens of India, the conserved sequence motifs of TACAT and TATAT were found. Such subjects are depicted as termination-associated sequence segments (TASs) with mtDNA synthesis (Foran et al., 1988). Both Galliformes and mammals have TASs, which may propose a strong auxiliary capacity of the D-loop area of the two genera, while the lack of variety in TASs among the Galliformes may be a direct result of the particular utilitarian constraints. The eight haplotypes recognized from the Indian indigenous chicken and the phylogenetic examination spoke to the formative associations.
A large number of the investigations on domestic chicken origins have focused on the D-Loop; there were additionally a few examinations concentrating on mitochondrial genomics to analyze domestic chicken origins (Miao et al., 2013). Kauai feral chicken mitochondrial phylogenies (D-loop and whole mtDNA) revealed two different clades within their samples (Gering et al., 2015). Maybe the reason for the difference was that the samples for the examination were different between the whole mtDNA phylogeny and mtDNA D-loop phylogeny. We found that the mitochondrial phylogenies (D-loop and whole Mt genome) uncovered a few clades, and their examination re-evaluated the worldwide mtDNA profiles of chickens and encouraged our study of the settlement in India.
From the mtDNA examination, we saw that Indian RJF is the origin of reference RJF as well as Indian breeds (i.e. Aseel, Ghagus, Nicobari brown, Kadaknath, H. black, and Tellicherry). The reference RJF is closely related to the Indian reference RJF, which, in turn, has contributed to the genetic makeup of six native Indian breeds. This is likewise true for the Indian chicken, which originated from independent domestication from Indian RJF and likely from other RJF species. Curiously, the mixing of various Indian breeds with Indian RJF could explain how the current-day Asiatic chicken may have originated from various ancestors through numerous domestication events and such multi-origin breeds could still be seen in a single geographical location. This is in agreement with the current-day perceptions of native Japanese chickens that originated from various regions (Oka et al., 2007). However, all the breeds aside from Indian RJF form a solitary group, suggesting a common ancestor for these birds, including jungle fowls and domestic birds. The separation of the Indian RJF from the main group of breeds shows the possibility of a speciation event. Our examination uncovered that the Indian breeds are relatively pure with uncommon hybridization with reference RJF. In the current investigation of Indian breeds, we did not come across recognizable hybridization at least in the recent past, as shown by a clear separation of Indian RJF clades from reference RJF in mtDNA-based phylogeny. All these results indicate the genetic integrity of the Indian RJF.
In high-altitude birds, hypoxia is an unavoidable environmental stress; in natural selection, every step of aerobic respiration had to be experienced to improve the adaptability to hypoxia (Altshuler and Dudley, 2006; Scott, 2011; Scott and Milsom, 2006). Mitochondria play an important role in aerobic respiration through oxidative phosphorylation, because the majority of produced cell ATP is consumed for cellular oxygen uptake (Chandel and Schumacker, 2000; Xu et al., 2007). To determine the role of mitochondrial genes in high-altitude adaptation, six high-altitude Phasianidae birds and 16 low-altitude relatives’ mitochondrial genomes were analyzed, and four lineages were found for this high-altitude habitat (Zhou et al., 2014). Their results strongly suggest that the adaptive evolution of mitochondrial genes, i.e. ND2, ND4, and ATP6, played a critical role during the independent acclimatization to high altitude by galliform birds. In Tibet, a chicken breed was found to have a missense mutation in the MT-ND5 subunit of the NADH dehydrogenase gene for high-altitude adaptation (Bao et al., 2007). To explore the regulatory mechanisms for hypoxia adaptability, the ATP-6 gene was sequenced from 28 Tibetan chickens and 29 Chinese domestic chickens; six SNPs were detected (Zhao et al., 2015). In high-altitude adaption, cytochrome c oxidase (COX) was the key mitochondrial gene and plays an important role in oxidative phosphorylation regulation and oxygen sensing transfer. For identifying the COX gene SNP, the Tibet Chicken and four lowland chicken breeds (Dongxiang Chicken, Silky Chicken, Hubbard ISA White broiler, and Leghorn layer) were used and 13 haplotypes were defined for the 14 SNPs. It was concluded that the significant difference in MT-CO3 gene mutation might have a relationship with the high-altitude adaptation (Bao et al., 2008). MT-CO3 gene was sequenced by using 125 Tibetan chickens and 144 Chinese domestic chickens; eight SNPs were identified, and were defined into nine haplotypes. They found positive and negative haplotype associations with high-altitude adaptation (Sun et al., 2013). However, the MT-COI gene was sequenced from 29 Tibetan chickens and 30 Chinese domestic chickens, and nine SNPs were detected (Zhao et al., 2015). In our study, ND3, ND4, ND5, ATP6, COX1, and COX2 showed high-altitude lineages between Nicobari brown and Reference RJF birds which may help chickens to evolve to adapt to Nicobari Island environments (Figures 5–8).
mtDNA mutations contribute to enclosing both tissue-specific and multiple-system disorders in human diseases (Taylor and Turnbull, 2005; Tuppen et al., 2010). The spindle-associated chromosomal exchange did not show antagonistic consequences for fertilization on subsequent embryo/fetal development in the rhesus monkey (Tachibana et al., 2009). Subsequently, this method may speak to another dependable restorative way to deal with the transmission of mtDNA mutations in influenced families. Mitochondrial heterogeneity is the presence of at least two kinds of mitochondrial (mt) DNA in the same individual/tissue/cell and it is firmly related to animal health and disease. In mtDNA, ND2 is a protein-coding gene and it partakes in the mitochondrial respiratory chain and oxidative phosphorylation. In cloned sequencing of the ND2 region, numerous potential heteroplasmic locales were recognized, which possibly reflected bountiful heteroplasmy in the chicken mitochondrial genome (Yang et al., 2020). These outcomes give a significant reference for further research on heteroplasmy in chicken mitochondria. Recently, an examination utilized complete mtDNA from tuberculosis patients’ blood samples and explored the conceivable mtDNA variations (Tonsing et al., 2020). Twenty-eight non-synonymous variants were found and most of the variations lie in the D-loop of the non-protein-coding region of the mitochondrial DNA. Runting and stunting syndrome (RSS) generally happens early in life and causes low body weight and extensive economic losses in the commercial broiler industry (Kang et al., 2012). In sex-linked dwarf (SLD) chickens, the RSS is related to mitochondria dysfunction, and mutations in the TWNK gene are one reason for mtDNA exhaustion (Li et al., 2019; Hu et al., 2020). We recommend that mutations in the mitochondrial genome should be validated further to fully understand their relationship with animal diseases.
Limitations of the work
Deep sampling using the NGS technique offers a straightforward, high-throughput, and cost-effective platform for effectively detecting and measuring mitochondrial heteroplasmy in complete mitochondrial genomes. The main limitation of this research is that only seven native Indian breeds were used. Nevertheless, the information that was collected will be useful in finding heteroplasmy in various chicken breeds both domestically and internationally.
Conclusion
In summary, the eight haplotypes of the native chicken population showed a relatively rich genetic pool, and molecular information on genetic diversity revealed may help in developing genetic improvement and conservation strategies to better utilize precious genetic reserves. The phylogenetic relationship of native Indian chicken breeds using entire mtDNA and D-loop sequences showed that the South East Asian RJF is distant from the Indian RJF but genetically close to the Indian breeds, whereas the Indian Aseel breed was more closely related to the reference RJF than the Indian RJF. The grouping of Indian RJF separated from Indian native chickens and the presence of subcontinent explicit haplogroups gives additional proof for an independent domestication event of chickens in the subcontinent. For high-altitude hypoxic adaptation, it is important to improve the efficiency of oxygen usage instead of enhancing oxygen uptake and transport. Thus, in natural selection, the mitochondrial genome encoded 12 essential structural genes (6 NADH dehydrogenase genes, cytochrome b subunit, 3 cytochrome c oxidase, and ATP synthase subunit), which must have mutated during adaptation to high-altitude hypoxic conditions.
Data availability statement
The datasets presented in this study can be found in online repositories. The names of the repository/repositories and accession number(s) can be found in the article/Supplementary Material.
Ethics statement
The animal study was reviewed and approved by Institute Animal Ethics Committee.
Author contributions
MK analyzed the data and prepared the draft manuscript. TB developed the idea, planned the research work, carried out the wet lab experiment, and edited the draft. RC analyzed the data and prepared the tables and graphs, MR collected the samples of chicken breeds and MD isolated DNA samples. All authors contributed to the article and approved the submitted version.
Funding
The work was funded by the Department of Science and Technology (SERB) (Project No. CRG/2018/002246), the Government of India.
Acknowledgments
The authors acknowledge the WBUAFS, West Bengal, and CSKHPKVV, Palampur for providing blood samples to carry out the research work. The corresponding author also extends thanks and gratitude to the Department of Science and Technology (SERB), Government of India (Project No. CRG/2018/002246) for providing financial support to carry out the research work.
Conflict of interest
The authors declare that the research was conducted in the absence of any commercial or financial relationships that could be construed as a potential conflict of interest.
Publisher’s note
All claims expressed in this article are solely those of the authors and do not necessarily represent those of their affiliated organizations, or those of the publisher, the editors and the reviewers. Any product that may be evaluated in this article, or claim that may be made by its manufacturer, is not guaranteed or endorsed by the publisher.
Supplementary material
The Supplementary Material for this article can be found online at: https://www.frontiersin.org/articles/10.3389/fgene.2023.1083976/full#supplementary-material
SUPPLEMENTARY FIGURE S1 | PCR products are loaded on 1% agarose gel. Samples A1-A11-Aseel; A13-A23-Ghagus; B1-B11-Nicobari brown; B13-B23-Nicobari black; C1-C11-Tellichery; C13-C23-Kadaknath; D1-D11-Haringhata; D13-D23-Red Jungle Fowl; A12, B12, C12, D12 are ladder.
SUPPLEMENTARY FIGURE S2 | Validation of mtDNA D-loop (A) and NADH dehydrogenase gene (B) regions with D-loop and NADH gene-specific primers, respectively. F-Female; M-Male; 1-100 bp DNA ladder; 2-negative control; 3- Indian RJF; 4- Nicobari; 5- Kadaknath; 6- Ghagus; 7- Aseel; 8- Haringhata black; 9- Tellicherry.
SUPPLEMENTARY FIGURE S3 | Validation of mtDNA COX1, tRNA, rRNA, and CYTB gene regions with gene-specific primers, respectively. F-Female; M-Male; 1-100 bp DNA ladder; 2-negative control; 3- Indian RJF; 4- Nicobari; 5- Kadaknath; 6- Ghagus; 7- Aseel; 8- Haringhata black; 9- Tellicherry.
SUPPLEMENTARY TABLE S1 | Single nucleotide variants (SNVs) called in whole mtDNA sequencing analysis of samples from seven native Indian chicken breeds. The results from sequencing 500ng total mtDNA extracted from whole blood.
SUPPLEMENTARY TABLE S2 | Organization of the mitochondrial genome in seven native Indian chicken breeds.
Abbreviations
ATP6, mitochondrial encoded ATP synthase membrane subunit 6; COX1, cytochrome c oxidase subunit I; COX2, cytochrome c oxidase subunit II; COX3, cytochrome c oxidase subunit III; CYTB, Cytochrome b; INDELs, Insertions, and deletions; ICAR-NBAGR, Indian council for the agricultural research-National bureau of animal genetic resources; mtDNA, Mitochondrial DNA, NGS, Next generation sequencing; NAD1, NADH dehydrogenase subunit 1; NAD2, NADH dehydrogenase subunit 2; NAD3, NADH dehydrogenase subunit 3; NAD4, NADH dehydrogenase subunit 4; NAD5, NADH dehydrogenase subunit 5; NAD6, NADH dehydrogenase subunit 6; OXPHOS, oxidative phosphorylation; RJF, Red Jungle Fowl; rRNA, Ribosomal RNA; RSS, Runting and stunting syndrome; SNP, Single-nucleotide polymorphism; SEA, South East Asia; SLD, sex-linked dwarf; TWNK, Twinkle mtDNA helicase.
References
Ahmed, S. U., Shukla, S., and Das, H. (2017). Molecular Characterization of Mitochondrial D Loop of Indian Red Jungle Fowl. Int. J. Livest. Res. 7 (6), 1–152. doi:10.5455/ijlr.20170423033604
Altshuler, D. L., and Dudley, R. (2006). The physiology and biomechanics of avian flight at high altitude. Integr. Comp. Biol. 46, 62–71. doi:10.1093/icb/icj008
Anderson, S., Bankier, A. T., Barrell, B. G., de Bruijn, M. H., Coulson, A. R., Drouin, J., et al. (1981). Sequence and organization of the human mitochondrial genome. Nature 290 (5806), 457–465. doi:10.1038/290457a0
Bandelt, H. J., Forster, P., and Rohl, A. (1999). Median-joining networks for inferring intraspecific phylogenies. Mol. Biol. Evol. 16 (1), 37–48. doi:10.1093/oxfordjournals.molbev.a026036
Bao, H., Zhao, C., Li, J., and Wu, C. (2007). Association of MT-ND5 gene variation with mitochondrial respiratory control ratio and NADH dehydrogenase activity in Tibet chicken embryos. Anim. Genet. 38, 514–516. doi:10.1111/j.1365-2052.2007.01622.x
Bao, H., Zhao, C., Li, J., and Wu, C. (2008). Sequencing and alignment of mitochondrial genomes of Tibetan chicken and two lowland chicken breeds. Sci. China Life Sci. 51, 47–51. doi:10.1007/s11427-008-0005-0
Brisbin, I. L., Peterson, A. T., Okimoto, R., and Amato, R. G. (2002). Characterization of the genetic status of populations of red jungle fowl. J. Bombay Nat. Hist. Soc. 99, 217–223.
Buehler, D. M., and Baker, A. J. (2003). Characterization of the red knot (Calidris canutus) mitochondrial control region. Genome 46 (4), 565–572. doi:10.1139/g03-034
Chandel, N. S., and Schumacker, P. T. (2000). Cellular oxygen sensing by mitochondria: old questions, new insight. J. Appl. Physiol. 88, 1880–1889. doi:10.1152/jappl.2000.88.5.1880
Crawford, R. (1984). “Domestic fowl,” in Evolution of Domesticated Animals. Editor L. L. Mason (London: Longman), 298–311.
Danforth, C. H. (1958). Gallus sonnerati and the domestic fowl. J. Hered. 49, 167–170. doi:10.1093/oxfordjournals.jhered.a106797
Darwin, C. (1868). The Variation of Animals and Plants Under Domestication. London, UK: John Murray.
Di Lorenzo, P., Ceccobelli, S., Panella, F., Attard, G., and Lasagna, E. (2015). The role of mitochondrial DNA to determine the origin of domestic chicken. World's Poult. Sci. J. 71 (2), 311–318. doi:10.1017/S0043933915000318
Eriksson, J., Larson, G., Gunnarsson, U., Bed'Hom, B., Tixier-Boichard, M., Strömstedt, L., et al. (2008). Identification of the yellow skin gene reveals a hybrid origin of the domestic chicken. PLoS Genet 4, e1000010. doi:10.1371/journal.pgen.1000010
Excoffier, L., Smouse, P., and Quattro, J. (1992). Analysis of molecular variance inferred from metric distances among DNA haplotypes: application to human mitochondrial DNA restriction data. Genetics 131 (2), 479–491. doi:10.1093/genetics/131.2.479
Fernandes, M., Mukesh, S. S., Sathyakumar, S., Kaul, R., Kalsi, R. S., and Sharma, D. (2009). Conservation of red junglefowl Gallus gallus in India. Int. J. Galli. Conserv. 1, 94–101.
Foran, D. R., Hixson, J. E., and Brown, W. M. (1988). Comparisons of ape and human sequences that regulate mitochondrial DNA transcription and D-loop DNA synthesis. Nucleic Acids Res 16 (13), 5841–5861. doi:10.1093/nar/16.13.5841
Fumihito, A., Miyake, T., Sumi, S., Takada, M., Ohno, S., and Kondo, N. (1994). One subspecies of the red junglefowl (Gallus gallus gallus) suffices as the matriarchic ancestor of all domestic breeds. Proc. Natl. Acad. Sci. U. S. A. 91 (26), 12505–12509. doi:10.1073/pnas.91.26.12505
Fumihito, A., Miyake, T., Takada, M., Shingu, R., Endo, T., Gojobori, T., et al. (1996). Monophyletic origin and unique dispersal patterns of domestic fowls. Proc. Natl. Acad. Sci. U. S. A. 93 (13), 6792–6795. doi:10.1073/pnas.93.13.6792
Gering, E., Johnsson, M., Willis, P., Getty, T., and Wright, D. (2015). Mixed ancestry and admixture in Kauai's feral chickens: invasion of domestic genes into ancient Red Junglefowl reservoirs. Mol. Ecol. 24 (9), 2112–24. doi:10.1111/mec.13096
Gifford-Gonzalez, D., and Hanotte, O. (2011). Domesticating animals in Africa: implications of genetic and archaeological findings. J. World Prehist. 24, 1–23. doi:10.1007/s10963-010-9042-2
Gu, J., and Li, S. (2020). Next-generation sequencing of the complete mitochondrial genome of the Piao chicken (Gallus gallus). Mit. DNA Part B 5 (3), 2870–2871. doi:10.1080/23802359.2020.1791755
Gupta, J., Mathew, J., Shukla, S. J. K., Mehra, S., Mehra, M., Sharma, A., et al. (2009). Mitochondrial DNA variation within and between the Gallus species. Asian J. Microbiol. Biotechnol. Environ. Sci. 11 (4), 717–721.
Haring, E., Kruckenhauser, L., Gamauf, A., Riesing, M. J., and Pinsker, W. (2001). The complete sequence of the mitochondrial genome of Buteo buteo (Aves, Accipitridae) indicates an early split in the phylogeny of raptors. Mol. Biol. Evol. 18 (10), 1892–904. doi:10.1093/oxfordjournals.molbev.a003730
Hu, B., Yang, M., Liao, Z., Wei, H., Zhao, C., Li, D., et al. (2020). Mutation of TWNK Gene Is One of the Reasons of Runting and Stunting Syndrome Characterized by mtDNA Depletion in Sex-Linked Dwarf Chicken. Front. Cell Dev. Biol. 8, 581. doi:10.3389/fcell.2020.00581
Jia, X-X., Tang, X-J., Lu, J-X., Fan, Y-F., Chen, D-W., Tang, M-J., et al. (2016). The investigation of genetic diversity and evolution of Daweishan Mini chicken based on the complete mitochondrial (mt) DNA D-loop region sequence. Mit. DNA Part A 27 (4), 3001–3004. doi:10.3109/19401736.2015.1060478
Karsli, T., and Balcıoğlu, M. S. (2019). Genetic characterization and population structure of six brown layer pure lines using microsatellite markers. Asian-Australas. J. Anim. Sci. 32 (1), 49–57. doi:10.5713/ajas.17.0870
Kanakachari, M., Rahman, H., Chatterjee, R. N., and Bhattacharya, T. K. (2022). Signature of Indian native chicken breeds: a perspective. World's Poult. Sci. J. 78 (2), 421–445. doi:10.1080/00439339.2022.2026201
Kang, K. I., El-Gazzar, M., Sellers, H. S., Dorea, F., Williams, S. M., Kim, T., et al. (2012). Investigation into the aetiology of runting and stunting syndrome in chickens. Avian Pathol 41 (1), 41–50. doi:10.1080/03079457.2011.632402
Kanginakudru, S., Metta, M., Jakati, R. D., and Nagaraju, J. (2008). Genetic evidence from Indian red jungle fowl corroborates multiple domestication of modern day chicken. BMC Evol. Biol. 8, 174–178. doi:10.1186/1471-2148-8-174
Kawabe, K., Worawut, R., Taura, S., Shimogiri, T., Nishida, T., and Okamoto, S. (2014). Genetic Diversity of mtDNA D-loop Polymorphisms in Laotian Native Fowl Populations. Asian-Australas. J. Anim. Sci. 27 (1), 19–23. doi:10.5713/ajas.2013.13443
Keeling, L., Andersson, L., Schütz, K. E., Kerje, S., Fredriksson, R., Carlborg, Ö., et al. (2004). Chicken genomics: feather-pecking and victim pigmentation. Nature 431, 645–646. doi:10.1038/431645a
Kumar, S., Stecher, G., Li, M., Knyaz, C., and Tamura, K. (2018). MEGA X: molecular evolutionary genetics analysis across computing platforms. Mol. Biol. Evol. 35, 1547–1549. doi:10.1093/molbev/msy096
Kumar, S., Tamura, K., and Nei, M. (2004). MEGA3: integrated software for molecular evolutionary genetics analysis and sequence alignment. Brief. Bioinformatics 5 (2), 150–163. doi:10.1093/bib/5.2.150
Lawal, R. A. (2017). “Signatures of Selection and Introgression in the Genus Gallus,”. Ph.D. thesis (Nottingham: University of Nottingham).
Li, H., Hu, B., Luo, Q., Hu, S., Luo, Y., Zhao, B., et al. (2019). Runting and stunting syndrome is associated with mitochondrial dysfunction in sex-linked dwarf chicken. Front. Genet. 10, 1337. doi:10.3389/fgene.2019.01337
Lin, Q., Cao, R., Jiang, G. T., Qiu, L., Hu, G. B., Dai, Q. Z., et al. (2016). The complete mitochondrial genome of the Xupu goose. Mit. DNA Part A 27 (2), 1010–1011. doi:10.3109/19401736.2014.926528
Lin, Q., Jiang, G. T., Li, Y. H., Yan, H. F., Liu, X., and Lu, B. (2019). Removal of bisphenol A from aqueous solution via host-guest interactions based on beta-cyclodextrin grafted cellulose bead. Braz. J. Poult. Sci. 21, 1–9. doi:10.1016/j.ijbiomac.2019.08.116
Liu, L. L., Xie, H. B., Yang, Y. S., Yu, Q. F., and He, J. H. (2016a). The complete mitochondrial genome of the Xuefeng black-boned chicken. Mit. DNA Part A 27 (1), 30–31. doi:10.3109/19401736.2013.869679
Liu, L. L., Xie, H. B., Yu, Q. F., He, S. P., and He, J. H. (2016b). Determination and analysis of the complete mitochondrial genome sequence of Taoyuan chicken. Mit. DNA part A 27 (1), 371–372. doi:10.3109/19401736.2014.895991
Liu, Y. P., Wu, G. S., Yao, Y. G., Miao, Y. W., Luikart, G., Baig, M., et al. (2006). Multiple maternal origins of chickens: out of the Asian jungles. Mol. Phylogenet. Evol. 38 (1), 12–19. doi:10.1016/j.ympev.2005.09.014
Liu, Z. G., Lei, C. Z., Luo, J., Ding, C., Chen, G. H., Chang, H., et al. (2004). Genetic variability of mtDNA sequences in Chinese native chicken breeds. Asian-Australs. J. Anim. Sci. 17 (7), 903–909. doi:10.5713/ajas.2004.903
Maw, A. A., Kawabe, K., Shimogiri, T., Rerkamnuaychoke, W., Kawamoto, Y., Masuda, S., et al. (2015). Genetic diversity and population structure in native chicken populations from Myanmar, Thailand and Laos by using 102 indels markers. Asian-Australas. J. Anim. Sci. 8 (1), 14–19. doi:10.5713/ajas.14.0212
Mekchay, S., Supakankul, P., Assawamakin, A., Wilantho, A., Chareanchim, W., and Tongsima, S. (2014). Population structure of four Thai indigenous chicken breeds. BMC Genet 15, 40–45. doi:10.1186/1471-2156-15-40
Miao, Y. W., Peng, M. S., Wu, G. S., Ouyang, Y. N., Yang, Z. Y., Yu, N., et al. (2013). Chicken domestication: an updated perspective based on mitochondrial genomes. Heredity 110 (3), 277–282. doi:10.1038/hdy.2012.83
Mobegi, V. A. (2006). Genetic characterization of African chicken using mitochondrial DNA D-loop sequences [M.S. thesis]. Nairobi, Kenya: University of Nairobi.
Moiseyeva, I. G., Romanov, M. N., Nikiforov, A. A., Sevastyanova, A. A., and Semyenova, S. K. (2003). Evolutionary relationships of Red Jungle Fowl and chicken breeds. Genet. Sel. Evol. 35 (4), 403–423. doi:10.1186/1297-9686-35-5-403
Moore, W. S. (1995). Inferring phylogenies from mtDNA variation: mitochondrial-gene trees versus nuclear-gene trees. Evolution 49 (4), 718–726. doi:10.1111/j.1558-5646.1995.tb02308.x
Morejohn, G. V. (1968). Breakdown of isolation mechanisms in two species of captive junglefowl (Gallus gallus and Gallus sonneratii). Evolution 22, 576–582. doi:10.1111/j.1558-5646.1968.tb03993.x
Mwacharo, J. M., Bjørnstad, G., Mobegi, V., Nomura, K., Hanada, H., Amano, T., et al. (2011). Mitochondrial DNA reveals multiple introductions of domestic chicken in East Africa. Mol. Phylogenet. Evol. 58, 374–382. doi:10.1016/j.ympev.2010.11.027
Nishibori, M. (2004). Complete sequence of mitochondrial D-loop region of red jungle fowls (Gallus gallus) and their genetic diversity in Myanmar and its neighbor countries. Report of the Society for Researches on Native Livestock 21, 213–223.
Nishibori, M., Shimogiri, T., Hayashi, T., and Yasue, H. (2005). Molecular evidence for hybridization of species in the genus Gallus except for Gallus varius. Anim. Genet. 36 (5), 367–375. doi:10.1111/j.1365-2052.2005.01318.x
Niu, D., Fu, Y., Luo, J., Ruan, H., Yu, X-P., Chen, G., et al. (2002). The origin and genetic diversity of Chinese native chicken breeds. Biochem. Genet. 40 (5-6), 163–174. doi:10.1023/a:1015832108669
Oka, T., Ino, Y., Nomura, K., Kawashima, S., Kuwayama, T., Hanada, H., et al. (2007). Analysis of mtDNA sequences shows Japanese native chickens have multiple origins. Anim. Genet. 38 (3), 287–293. doi:10.1111/j.1365-2052.2007.01604.x
Padhi, M. K. (2016). Importance of indigenous breeds of chicken for rural economy and their improvements for higher production performance. Scientifica 2016, 2604685. doi:10.1155/2016/2604685
Peters, J., Lebrasseur, O., Best, J., Miller, H., Fothergill, T., Dobney, K., et al. (2015). Questioning new answers regarding Holocene chicken domestication in China. Proc. Natl. Acad. Sci. U. S. A. 12, E2415. doi:10.1073/pnas.1503579112
Peterson, A. T., and Brisbin, I. L. (1999). Genetic endangerment of wild red jungle fowl (Gallus gallus)?. Bird Conserv. Int. 9, 387–394. doi:10.1017/S0959270900002148
Quinn, T. W., and Wilson, A. C. (1993). Sequence evolution in and around the mitochondrial control region in birds. J. Mol. Evol. 37 (4), 417–425. doi:10.1007/BF00178871
Rozas, J., Sanchez-DelBarrio, J. C., Messeguer, X., and Rozas, R. (2003). DnaSP, DNA polymorphism analyses by the coalescent and other methods. Bioinformatics 19 (18), 2496–2497. doi:10.1093/bioinformatics/btg359
Ruokonen, M., and Kvist, L. (2002). Structure and evolution of the avian mitochondrial control region. Mol. Phylogenet. Evol. 23 (3), 422–432. doi:10.1016/S1055-7903(02)00021-0
Sambrook, J., and Russell, D. W. (2001). Molecular cloning: A laboratory manual. 3rd. New York, NY: Cold Spring Harbor Laboratory Press, Cold Spring Harbor.
Schneider, S., Roessli, D., and Excoffier, L. (2000). Arlequin Version 2.000: Software for Population Genetics and Data Analysis. Geneva, Switzerland: Genetics and Biometry Laboratory, University of Geneva, 1–11.
Schütz, K. E., Forkman, B., and Jensen, P. (2001). Domestication effects on foraging strategy, social behaviour, and different fear responses: a comparison between the red junglefowl (Gallus gallus) and a modern layer strain. Appl. Anim. Behav. Sci. 74, 1–14. doi:10.1016/S0168-1591(01)00156-3
Scott, G. R., and Milsom, W. K. (2006). Flying high: A theoretical analysis of the factors limiting exercise performance in birds at altitude. Respir. Physiol. Neurobiol. 154, 284–301. doi:10.1016/j.resp.2006.02.012
Scott, G. R. (2011). Elevated performance: the unique physiology of birds that fly at high altitudes. J. Exp. Biol. 214, 2455–2462. doi:10.1242/jeb.052548
Silva, P., Guan, X., Ho-Shing, O., Jones, J., Xu, J., Hui, D., et al. (2009). Mitochondrial DNA-based analysis of genetic variation and relatedness among Sri Lankan indigenous chickens and the Ceylon junglefowl (Gallus lafayetti). Anim. Genet. 40 (1), 1–9. doi:10.1111/j.1365-2052.2008.01783.x
Stevens, L. (1991). Genetic and evolution of the domestic fowl. New York, NY: Cambridge University Press.
Sun, J., Zhong, H., Chen, S. Y., Yao, Y. G., and Liu, Y. P. (2013). Association between MT-CO3 haplotypes and high-altitude adaptation in Tibetan chicken. Gene 529, 131–137. doi:10.1016/j.gene.2013.06.075
Tachibana, M., Sparman, M., Sritanaudomchai, H., Ma, H., Clepper, L., Woodward, J., et al. (2009). Mitochondrial gene replacement in primate offspring and embryonic stem cells. Nature 461 (7262), 367–372. doi:10.1038/nature08368
Tamura, K., and Nei, M. (1993). Estimation of the number of nucleotide substitutions in the control region of mitochondrial DNA in humans and chimpanzees. Mol. Biol. Evol. 10, 512–526. doi:10.1093/oxfordjournals.molbev.a040023
Taylor, R. W., and Turnbull, D. M. (2005). Mitochondrial DNA mutations in human disease. Nat. Rev. Genet. 6 (5), 389–402. doi:10.1038/nrg1606
Thompson, J. D., Gibson, T. J., Plewniak, F., Jeanmougin, F., and Higgins, D. G. (1997). The CLUSTAL_X windows interface: flexible strategies for multiple sequence alignment aided by quality analysis tools. Nucleic Acids Res 25 (24), 4876–4882. doi:10.1093/nar/25.24.4876
Tixier-Boichard, M., Bed’hom, B., and Rognon, X. (2011). Chicken domestication: from archeology to genomics. C. R. Biol. 334, 197–204. doi:10.1016/j.crvi.2010.12.012
Tonsing, M. V., Sailo, C. V., Chhakchhuak, L., Chhakchhuak, Z., Pandit, B., Kumar, D., et al. (2020). Analysis of variants in mitochondrial genome and their putative pathogenicity in Tuberculosis patients from Mizoram, North east India. Mitochondrion 54, 21–25. doi:10.1016/j.mito.2020.06.012
Tuppen, H. A., Blakely, E. L., Turnbull, D. M., and Taylor, R. W. (2010). Mitochondrial DNA mutations and human disease. BBA-Bioenergetics 1797 (2), 113–128. doi:10.1016/j.bbabio.2009.09.005
Ulfah, M., Kawahara-Miki, R., Farajalllah, A., Muladno, M., Dorshorst, B., Martin, A., et al. (2016). Genetic features of red and green jungle fowls and relationship with Indonesian native chickens Sumatera and Kedu Hitam. BMC Genomics 17, 320–325. doi:10.1186/s12864-016-2652-z
Wani, C. E., Yousif, I. A., Ibrahim, M. E., and Musa, H. H. (2014). Molecular characterization of Sudanese and Southern Sudanese chicken breeds using mtDNA D-Loop. Genet. Res. Int. 2014, 928420. doi:10.1155/2014/928420
West, B., and Zhou, B-X. (1988). Did chicken go north? New evidence for domestication. J. Archaeol. Sci. 15, 515–533. doi:10.1016/0305-4403(88)90080-5
Wright, S. (1951). The genetical structure of populations. Ann. Eugenics 15 (1), 323–354. doi:10.1111/j.1469-1809.1949.tb02451.x
Xiang, H., Gao, J., Yu, B., Zhou, H., Cai, D., Zhang, Y., et al. (2014). Early Holocene chicken domestication in northern China. Proc. Natl. Acad. Sci. U. S. A. 111, 17564–17569. doi:10.1073/pnas.1411882111
Xu, S., Luosang, J., Hua, S., He, J., Ciren, A., Wang, W., et al. (2007). High altitude adaptation and phylogenetic analysis of Tibetan horse based on the mitochondrial genome. J. Genet. Genom. 34 (8), 720–729. doi:10.1016/S1673-8527(07)60081-2
Yacoub, H. A., and Fathi, M. M. (2013). Phylogenetic analysis using d-loop marker of mtDNA of Saudi native chicken strains. Mitochondrial DNA 24 (5), 538–551. doi:10.3109/19401736.2013.770494
Yang, S., Huo, Y., Wang, H., Ji, J., Chen, W., and Huang, Y. (2020). The spatio-temporal features of chicken mitochondrial ND2 gene heteroplasmy and the effects of nutrition factors on this gene. Sci. Rep. 10 (1), 2972–9. doi:10.1038/s41598-020-59703-y
Yu, Q. F., Liu, L. L., Fu, C. X., He, S. P., Li, S., and He, J. H. (2016). The complete mitochondrial genome of the Huang Lang chicken. Mitochondrial DNA Part A 27 (1), 216–217. doi:10.3109/19401736.2014.880895
Zeuner, F. E. (1963). A History of Domesticated Animals. London: Hutchinson and Co. (Publishers) Ltd.
Zhao, X., Wu, N., Zhu, Q., Gaur, U., Gu, T., and Li, D. (2015). High-altitude adaptation of Tibetan chicken from MT-COI and ATP-6 perspective. Mitochondrial DNA Part A 27 (5), 3280–3288. doi:10.3109/19401736.2015.1015006
Keywords: chicken, mitochondrial DNA, next-generation sequencing, SNPs, mutations and variants, molecular phylogeny
Citation: Kanakachari M, Chatterjee RN, Reddy MR, Dange M and Bhattacharya TK (2023) Indian Red Jungle fowl reveals a genetic relationship with South East Asian Red Jungle fowl and Indian native chicken breeds as evidenced through whole mitochondrial genome sequences. Front. Genet. 14:1083976. doi: 10.3389/fgene.2023.1083976
Received: 29 October 2022; Accepted: 18 July 2023;
Published: 09 August 2023.
Edited by:
Guillermo Giovambattista, CONICET Institute of Veterinary Genetics (IGEVET), ArgentinaReviewed by:
Ranjit Singh Kataria, National Bureau of Animal Genetic Resources (NBAGR), IndiaXiaolong Yuan, South China Agricultural University, China
Copyright © 2023 Kanakachari, Chatterjee, Reddy, Dange and Bhattacharya. This is an open-access article distributed under the terms of the Creative Commons Attribution License (CC BY). The use, distribution or reproduction in other forums is permitted, provided the original author(s) and the copyright owner(s) are credited and that the original publication in this journal is cited, in accordance with accepted academic practice. No use, distribution or reproduction is permitted which does not comply with these terms.
*Correspondence: T. K. Bhattacharya, YmhhdHRhY2hhcnlhdGtAZ21haWwuY29t