- 1Department of Pathobiology, College of Veterinary Medicine, Auburn University, Auburn, AL, United States
- 2Alabama Agricultural Experiment Station, Auburn University Center for Advanced Science, Innovation, and Commerce, Auburn, AL, United States
- 3Division of Laboratory Animal Health, College of Veterinary Medicine, Auburn University, Auburn, AL, United States
- 4Department of Human Genetics, School of Medicine, South Texas Diabetes and Obesity Institute, The University of Texas Rio Grande Valley, Brownsville, TX, United States
- 5HudsonAlpha Institute for Biotechnology, Huntsville, AL, United States
Introduction: Perfluoroalkyl and poly-fluoroalkyl substances (PFASs) are widely used in industrial and consumer products. Due to their environmental persistence and bioaccumulation, PFASs can be found in the blood of humans and wild animals all over the world. Various fluorinated alternatives such as GenX have been developed to replace the long-chain PFASs, but there is limited information about their potential toxicity.
Methods:The current study developed blood culture protocols to assess the response to toxic compounds in the marsupial, Monodelphis domestica. After whole-blood culture conditions were tested and optimized, changes in gene expression in response to PFOA and GenX treatment were assessed.
Results: More than 10,000 genes were expressed in the blood transcriptomes with and without treatment. Both PFOA and GenX treatment led to significant changes in the whole blood culture transcriptomes. A total of 578 and 148 differentially expressed genes (DEGs) were detected in the PFOA and GenX treatment groups, 32 of which overlapped. Pathway enrichment analysis revealed that DEGs involved in developmental processes were upregulated after PFOA exposure, while those enriched for metabolic and immune system processes were downregulated. GenX exposure upregulated genes associated with fatty acid transport pathways and inflammatory processes, which is consistent with previous studies using rodent models.
Discussion: To our knowledge, this study is the first to investigate the effect of PFASs in a marsupial model. The findings provide supportive evidence for significant transcriptomic alterations, suggesting that this mammalian model may provide a mechanism for exploring the potential toxicity of PFOA and GenX.
Introduction
Perfluoroalkyl and poly-fluoroalkyl substances (PFASs) are manufactured chemicals that have been widely used in the manufacture of cosmetics, firefighting foams, food contact materials, textiles, and other industrial and consumer products (Giesy and Kannan, 2002). There are thousands of different PFASs with fluorinated carbon chains of varying lengths that contain different functional groups (Buck et al., 2011). The high level of PFAS persistence, bioaccumulation, and use has contributed to their ubiquitous presence in the environment, especially in drinking water. Most PFASs are non-degradable or break down very slowly, allowing them to build up in organisms and the environment over time. Perfluorooctanoic acid (PFOA) and perfluorooctanesulfonic acid (PFOS) are two of the most widely used long-chain PFASs.
Human studies have found that some long-chain PFASs such as PFOS and PFOA tend to accumulate in the kidney, liver, and blood (Verreault et al., 2005), where they bind to serum albumin (Jones et al., 2003). PFAS concentration is determined using whole blood, plasma, or serum samples. Measurements in whole blood samples are approximately one-half of those detected in human plasma or serum (Ehresman et al., 2007). While PFOA has an average half-life of 3.8 years in serum, the half-life of PFOS is even longer (Olsen et al., 2007). Epidemiologic and animal-based research studies have linked PFAS exposure with cancer, impaired development, and detrimental effects on the immune system and reproductive function (Suh et al., 2011; McDonough et al., 2020; Grønnestad et al., 2021; Imir et al., 2021; Roth et al., 2021; Tarapore and Ouyang, 2021). Metabolic pathways, including fatty acid metabolism, are also affected (Sunderland et al., 2019).
Long-chain PFASs are recognized as environmental contaminants of serious concern due to their PBT characteristics (P: high persistence, B: bioaccumulation potential, T: toxicity). As a result, several long-chain PFASs, including PFOA and PFOS, have been gradually phased out in the US and Europe since 2000. They are replaced by various fluorinated alternatives, including the shorter-chain homologs of long-chain perfluoroalkyl acids and their precursors, as well as functionalized perfluoropolyethers (PFPEs) (Wang et al., 2013). GenX is the trade name for the ammonium salt of hexafluoropropylene oxide dimer acid (NH4-HFPO-DA), one of the most widely used fluorinated alternatives. This substance has been detected worldwide, including in the air and drinking water (Strynar et al., 2015; Sun et al., 2016; Gebbink et al., 2017), and persists for long periods in the environment (Wang et al., 2015). A recent study using electrooxidation found that GenX has a significantly slower rate of degradation than PFOA in water (Yang et al., 2022). Limited studies using rats and mice have shown that GenX has a shorter serum elimination half-life (Wang et al., 2013; Beekman et al., 2016). Recent animal experiments have found that GenX is also associated with reported adverse effects on neonatal development, lipid metabolism, and immune system function (Blake et al., 2020; Wen et al., 2020; Conley et al., 2021). In rat and human thyroid cell lines, GenX is shown to be more toxic than PFOA (Zhang et al., 2021). Acute toxicity, genotoxicity, and developmental toxicity have also been reported (Gordon, 2011; Gomis et al., 2018). The current study sought to complement rodent models by assessing the effects of PFAS exposure on a novel laboratory animal model, Monodelphis domestica.
The gray short-tailed opossum, Monodelphis domestica, is the best marsupial model for biomedical research (Fadem et al., 1982). This animal has been studied in a research laboratory environment for more than 40 years, has a well-assembled and well-annotated reference genome (Mikkelsen et al., 2007), and includes two validated inbred strains that enable control of its genetic background (Xiong et al., 2022). Marsupials (metatherian mammals) diverged from placentals (eutherian mammals) approximately 173–190 million years ago (Woodburne et al., 2003; van Rheede et al., 2006; Luo et al., 2011). The fundamental physiological and molecular processes of the opossum are conserved compared to eutherian mammals, making them an excellent alternative mammal model in which to identify highly conserved key genes and important functional pathways (Samollow, 2008). The current study performed RNA sequencing experiments in Monodelphis domestica to identify blood transcriptome changes that occurred in response to PFAS treatment. The effects of PFOA, one of the most widely used and most common long-chain PFAS in drinking water (Post et al., 2012), and GenX, one of the most popular PFOA/PFOS alternatives used in the industry, were assessed. The aim was to establish a blood cell culture system in the laboratory opossum to determine the effects of PFOA and GenX exposure on global gene expression, and to lay the foundation for further animal study of the impact of environmental pollutants on early embryonic development.
Materials and methods
Animal maintenance
The Monodelphis domestica used in this study was the LSD strain from a breeding colony established at Auburn University, with founder animals obtained from Dr. John VandeBerg’s laboratory at the University of Texas Rio Grande Valley. The animal maintenance, breeding, and operations followed the standard operating procedures (SOP) approved by the Auburn University Institutional Animal Care and Use Committee (AU-IACUC, approval numbers 2018-3,277 and 2021-3,883). The animals were individually caged under laboratory conditions at 23.5°C–26.5°C and 55%–60% humidity. A 14 h/10 h light/dark fluorescent lighting cycle was used. Dedicated commercial food and local municipal water were used as the daily supply. Daily care and veterinary services were provided by the Division of Laboratory Animal Health staff. A total of ten animals were used in the two experiments (Supplementary Table S1).
Blood sample collection and whole blood culture
Blood samples were obtained from anesthetized animals by cardiac puncture according to the IACUC-approved procedure for Monodelphis domestica. In brief, 1 mL sterile syringes (U-100, Becton, Dickinson and Company, NJ) were used for the blood draw, and samples were temporarily stored in a 2 mL vacutainer with lithium heparin (Becton, Dickinson and Company, NJ). In pilot Experiment 1, three biological replicates were included (A0036, A0039, and A0035_38). The blood samples from A0035 and A0038 were pooled to achieve a sufficient volume for culture experiments. The samples were diluted 1:2 and 1:4 with sterile RPMI-1640 culture medium complemented with 2 mM glutamine and antibiotics (100 U/mL penicillin and 100 μg/mL streptomycin), transferred to sterile 12-well culture plates with lids (USA Scientific, FL), and incubated at 37°C with 5% CO2. In Experiment 2, blood samples collected from two males (A0093 and A0094) were pooled to achieve a sufficient volume. Female blood samples were excluded from the analysis because insufficient amounts of blood were collected. The blood samples were diluted 1:2 with the same culture medium and cultured under the same conditions. Different exposure times were selected. In Experiment 1, 200 μl of the mixture was extracted at 0, 12, 24, 48, and 72 h after incubation (Figure 1A). In Experiment 2, 400 μl of blood culture was extracted at 0, 12, and 24 h after incubation. Three replicates were collected under each treatment condition at each time point. The samples were stored at −80°C before the extraction of DNA/RNA.
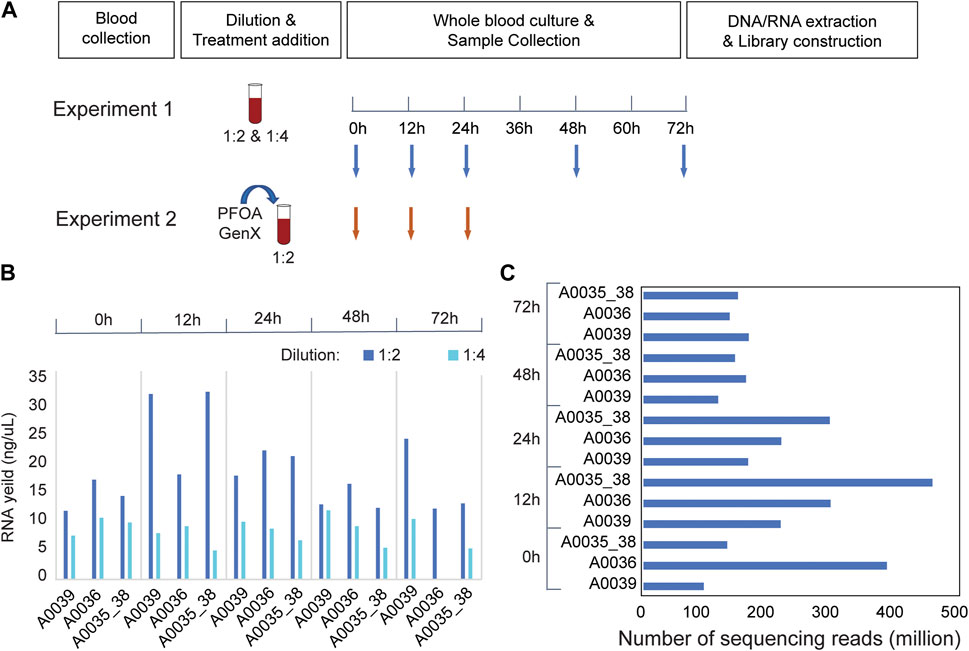
FIGURE 1. RNA-seq experiments in Monodelphis domestica whole blood culture samples without chemical disturbance. (A) Design of pilot experiments for protocol development (Experiment 1) and toxicity (Experiment 2). (B) RNA yield of Monodelphis domestica whole blood culture samples with different dilution factors and culture times. (C) RNA sequencing yield in Experiment 1.
PFOA and GenX treatments
PFOA and GenX were purchased from Sigma-Aldrich (Chemical Abstracts Service Registry numbers PFOA:335-67-1; GenX: 62037-80-3). Stock solutions were freshly prepared by dissolving the chemicals in molecular-grade dimethyl sulfoxide (DMSO) (>99.9%). The DMSO concentration was calculated and adjusted to achieve a final 0.1% volume percent (v/v) in all treatment and control groups. The desired concentrations in the final blood culture were achieved by adjusting the PFOA and GenX stock solutions. Two concentrations of each chemical were used in Experiment 2 (PFOA: 400 μM and 800 μM; GenX: 600 μM and 1,200 μM). DMSO of the same volume was added to the control group without treatment.
DNA/RNA extraction, RNA-seq library preparation, and Illumina sequencing
DNA/RNA extractions were conducted using Zymo Quick-DNA/RNA Miniprep Plus Kit (Zymo Research, CA) according to the manufacturer’s instructions. DNA/RNA quantity was measured using the Qubit Fluorometer 3.0 (Thermo Fisher Scientific, MA) with the Qubit RNA BR Assay Kit. RNA samples with >100 ng yield were selected for RNA-seq library preparation. RNA sequencing libraries were constructed using the NEBNext Ultra II Directional RNA Library Prep Kit for Illumina (New England Biolabs, MA) and NEBNext Poly(A) mRNA Magnetic Isolation Module (New England Biolabs, MA). The final concentrations and size distribution of the libraries were checked using the Qubit and HT DNA NGS 3K Assay on a LabChip GX Touch HT machine (Perkin Elmer, MA) (Supplementary Table S2). Libraries were sent for sequencing on an Illumina NovaSeq 6000 sequencing machine to generate 150-bp paired-end reads.
Analysis of the RNA-seq data
RNA-seq read quality was checked using FastQC v11.5 (Andrews, 2010). Trimmomatic v0.36 was used to remove Illumina adapter sequences and low-quality bases (Bolger et al., 2014). After trimming, 98.2% of the reads survived the quality control filtering in Experiment 1 and 96.4% in Experiment 2. The high-quality reads were mapped to the Monodelphis domestica reference genome assembly (momDom5, GCA_000002295.1) by Tophat (version 2.1.1) and STAR (version 2.7.5) (Trapnell et al., 2009; Langmead and Salzberg, 2012; Dobin et al., 2013).
Identification of differentially expressed genes (DEGs)
A total of 10 transcriptomes of the same sex (male) were used for analysis. Two control untreated samples, two PFOA-treated, and two GenX-treated samples were included 12 h after treatment. Two control untreated samples and two PFOA-treated samples were included 24 h after treatment. At 24 h, the GenX-treatment may suffer from a degradation issue (Liberatore et al., 2020), and was excluded from the analysis. Gene counts were summarized using BEDTools version 2.30.0 (Trapnell et al., 2009; Quinlan and Hall, 2010). Normalization of raw counts and identification of DEGs were performed using the TMM method associated with the edgeR package in R (version 4.1.2) (Robinson et al., 2010). Adjusted p-values were computed using the Benjamini–Hochberg method, with a False Discovery Rate (FDR) of <0.05. The cut-off for detecting significant DEGs was |log2(fold change)| >1 and FDR <0.05. Individual gene expression levels were quantified by Read Per Kilobase of transcript per Million mapped reads (RPKM). MA and volcano plots were generated in R.
Functional pathway analysis of the DEGs
Functional enrichment analysis was performed using Metascape with default parameters (Zhou et al., 2019), for upregulated and downregulated DEGs identified in the PFOA and GenX groups, respectively. Gene Ontology (GO) term analyses focusing on biological processes were performed at an adjusted p-value cut-off of 0.01.
Quantitative reverse-transcription PCR (qRT-PCR) validation of selected DEGs
Ten DEGs were selected for qRT-PCR validation, including three downregulated DEGs (Itgax, Alox15, and Plin2) and seven upregulated genes (Ptn, Aldh1a5, Wdr47, Cd36, Cd38, Fabp5, and Itgb3). The housekeeping gene, Gapdh, was selected as a reference gene for normalization based on relatively equal expression levels (as measured by RPKM) in the control and treated samples. Reverse transcription was performed using the LunaScript RT SuperMix Kit (New England Biolabs, MA). The input of the total RNA in each sample was 20 ng. A 20 μl reaction system was incubated for 2 min at 25°C, followed by 10 min at 55°C and 1 min at 95°C. QPCR primers were designed using Oligo 7.0 (Molecular Biology Insights Inc., CO) and synthesized at Eurofins Genomics (Supplementary Table S3). The UCSC In-Silico PCR tool was used to check the primer specificity in the Monodelphis domestica genome and transcriptome. QRT-PCR assays were performed with SYBR Green using the Luna Universal qPCR Master Mix kit (New England BioLabs, MA) in 96-well plates on a BioRad CFX Opus 96 thermocycler (Bio-Rad Laboratories, CA). The initial denaturing step was set at 95°C for 30 s, followed by 39 cycles of denaturation for 10 s at 95°C and extension for 30 s at primer-specific extension temperature. The melting curve was generated by heating from 65°C to 95°C in 0.5°C increments, with a 5 s dwell time. Two technical replicates were performed for each selected gene, and a t-test was used to measure differences in gene expression.
Results
A blood culture model of PFAS exposure in the laboratory opossum, Monodelphis domestica
To optimize the experimental conditions for blood culture in the laboratory opossum, a pilot experiment (Experiment 1) was performed using two different treatment dilutions in culture media (1:2 and 1:4). The blood mixtures were cultured for 72 h, and 200 μL samples were collected at 0, 12, 24, 36, and 72 h after culture (Figure 1A). At a 1:2 dilution, the total RNA yield was higher than 1:4, and the 12 and 24 h cultures had better RNA yield and quality than the 48 or 72 h cultures (Figure 1B). In the RNA-seq experiments, an average of 178.5 million mapped reads were obtained per sample, and a total of 11,349 expressed genes were detected in the blood transcriptome (Figure 1C). Transcriptome-wide expression correlations were high for the first 36 h after culture (Spearman’s rank correlation coefficient ρ = 0.9; Supplementary Figure S1A) after which they decreased significantly (ρ < 0.8; Supplementary Figure S1A). There were strong positive correlations between the two biological replicates (ρ > 0.95; Supplementary Figure S1B) at 12, 24, and 48 h after treatment that decreased at 72 h (Supplementary Figure S1B). Thus, up to 36 h of culture, extremely high correlations were observed between replicates and sufficient correlations were observed between time points.
Blood transcriptome analysis of Monodelphis domestica whole blood culture samples after PFOA/GenX treatment
Based on the results of pilot Experiment 1, PFOA and GenX treatments (Experiment 2) were performed in blood culture with samples collected at 12 and 24 h (Figure 1B). Two different concentrations of PFOA (400 μM and 800 μM) and GenX (600 μM and 1,200 μM) were used. Two biological replicates were included for RNA sequencing library preparation of the control, PFOA, and GenX treatment at 12 and 24 h, and the average insert size for the final libraries was 302 bp. Samples from the GenX group were excluded from the RNA-seq analysis at 24 h due to technical issues (see Methods). On average, 18.3 million mapped 150-bp reads were obtained per sample. A total of 10,940 and 11,470 expressed genes were detected in the PFOA and GenX treatment groups, respectively, which was similar to Experiment 1.
Identification of DEGs following exposure to PFOA and GenX
At a cut-off of FDR <0.05 and log2(fold change) > 1, 578 DEGs, including 422 upregulated and 156 downregulated genes, were detected in the PFOA treatment group (Figures 2A,B; Supplementary Data S1). Using the same cut-off values as those used in the PFOA group, 148 DEGs, including 125 upregulated and 23 downregulated, were detected in the GenX treatment group (Figures 2C,D and Supplementary Data S2). Twenty-two genes were significantly upregulated, and 10 genes were significantly downregulated in both treatment groups (Figure 4A), suggesting that similar molecular mechanisms were used. Interestingly, two DEGs, Plin2 and Lgals9-like, had opposing expression patterns (Figure 3B). While Plin2 was downregulated by 4.9-fold after PFOA treatment, its expression nearly quadrupled after GenX treatment. Lgals9-like had a 4.5-fold reduction in expression after PFOA exposure, while GenX treatment doubled its expression. Overall, however, most overlapping DEGs had concordant expression patterns following PFOA and GenX treatment, with minor differences between the two groups.
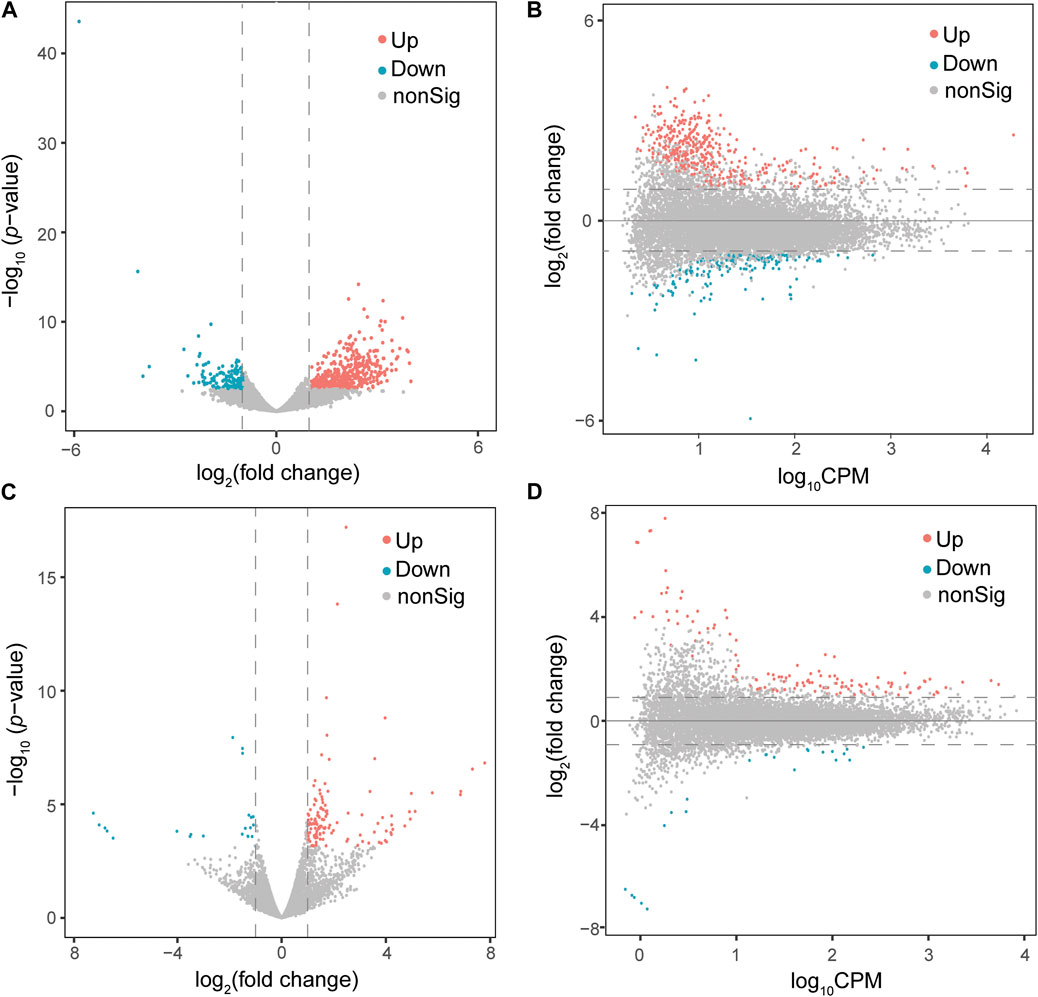
FIGURE 2. Differential gene expression analysis of male Monodelphis domestica blood culture samples from the PFOA/GenX treatment and control groups. (A, C) Volcano plot demonstrating differentially expressed genes (DEGs) in the PFOA (A) and GenX (C) treatment groups compared to the control group. Red dots represent significantly upregulated genes and blue dots represent significantly downregulated genes (FDR <0.05 and |log2FoldChange| >1). Grey dots represent non-significant genes. (B, D) MA plot demonstrating the DEGs in the PFOA (B) and GenX (D) treatment groups compared to the control group. The x-axis is log10CPM (log10 Counts per Million). The y-axis is log2 (Fold Change). The horizontal line indicates |log2FoldChange| = 1. Red dots indicate genes with significantly increased expression in the PFOA treatment group compared to the control group, and blue dots indicate genes with significantly decreased expression (FDR <0.05 and |log2FoldChange| >1).
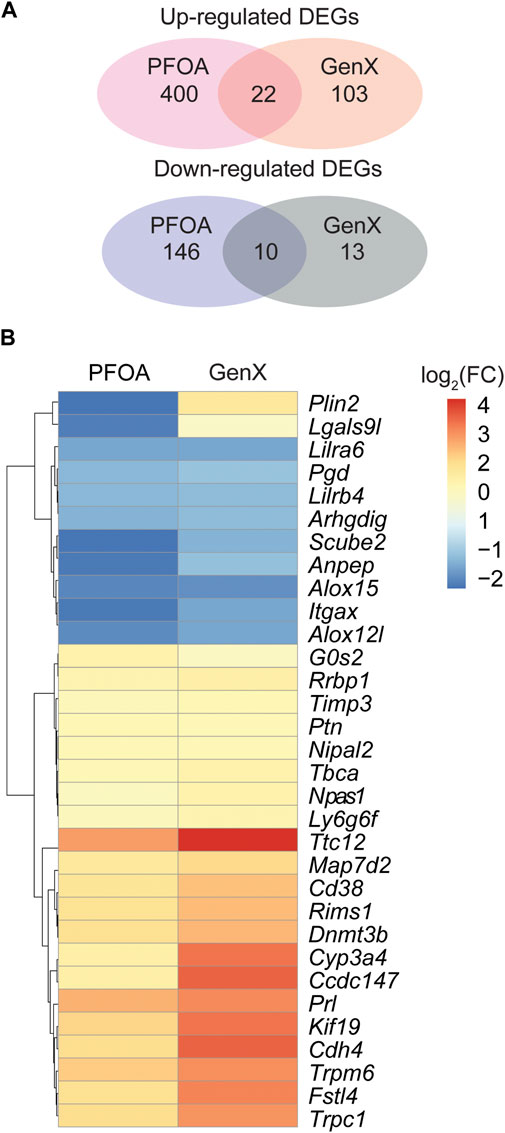
FIGURE 3. Differentially expressed genes in the PFOA and GenX-treated groups. (A) Venn diagram showing the overlap of upregulated and downregulated DEGs among the two treatment groups. (B) Hierarchical clustering of 32 overlapped DEGs identified between the PFOA and GenX treatment groups. Red represents upregulation, and blue indicates downregulation.
qRT-PCR validation of the DEGs
To confirm the DEGs identified in RNA-seq data, ten genes were selected for validation using qRT-PCR (see Methods). The opposing expression patterns of Plin2 observed following treatment with PFOA and GenX was confirmed by qRT-PCR (p < 0.01, t-test; Figure 4A). Meanwhile, Ptn was upregulated and Itgax was downregulated in both the PFOA and GenX treatment groups (Figures 4B,C). The remaining genes selected for testing (Wdr47, Aldh1a5, Alox15, Cd36, Cd38, Fabp5, and Itgb3) were also confirmed by qRT-PCR (Figures 4D–G; Supplementary Figure S2). The validation results were consistent with the RNA-seq results.
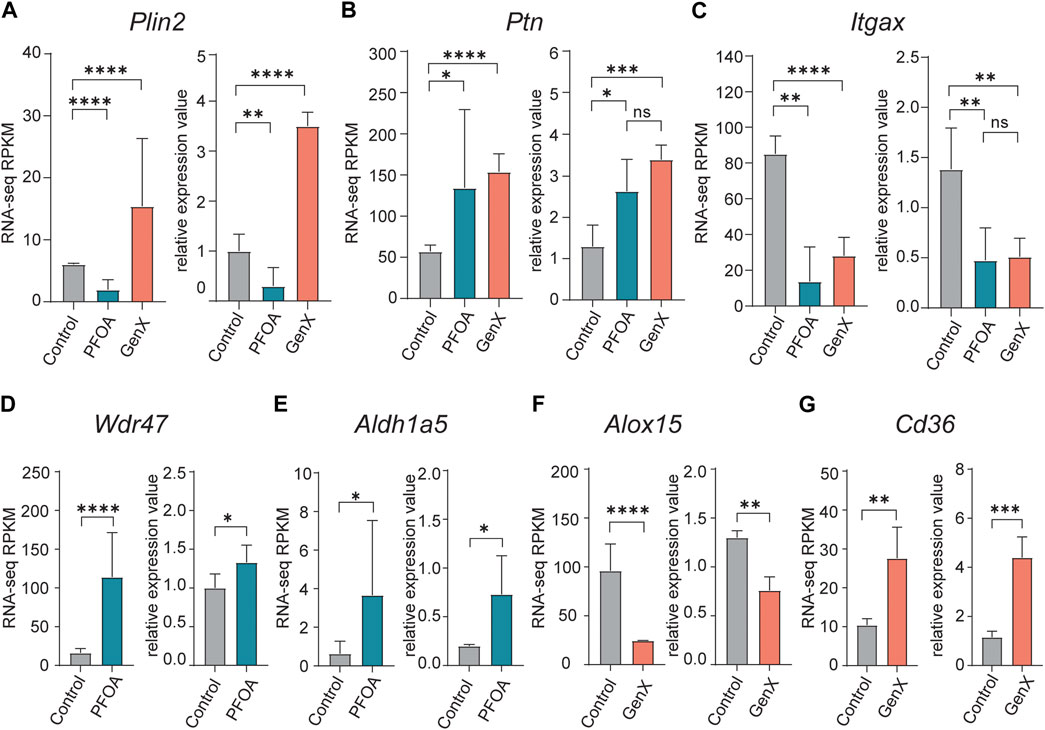
FIGURE 4. Quantitative reverse transcription PCR validation of upregulated and downregulated DEGs identified in the PFOA and GenX treatment groups. (A–G) Bar plots of qRT-PCR relative quantification and RNA-seq RPKM (Reads Per Kilobase of transcript per Million mapped reads) values for selected DEGs. (A) Plin2, (B) Ptn, (C) Itgax, (D) Wdr47, (E) Aldh1a5, (F) Alox15, (G) Cd36. For the RNA-seq RPKM level, significance was determined by q-values in edgeR (*, q < 0.05; **, q < 0.01; ****, q < 0.0001). For qRT-PCR validation experiments, significance was determined using an unpaired t-test (*p < 0.05; **, p < 0.01; ***, p < 0.001; ****, p < 0.0001).
Biological pathways altered by PFOA/GenX treatment
In response to PFOA treatment, five of the top ten significantly upregulated GO terms (p < 10−6) were involved in developmental processes, including cell morphogenesis (GO: 0000902), tissue morphogenesis (GO: 0048729), sensory organ development (GO: 0007423), renal system development (GO: 0072001), and heart development (0007507) (Figure 5A). The GO interaction network illustrated that tissue morphogenesis was tightly associated with other developmental processes, such as other organ development and embryonic morphogenesis (Figure 5B), suggesting that development-related functional terms were affected. Microtubule-based movement (GO: 0007018) was the most significant GO term, and the actin filament-based process (GO: 0030029) was also enriched, indicating a potential upregulation of intracellular transport. Pathways that were downregulated after PFOA exposure included metabolic and immune system processes (p < 10−4; Figure 5C). The regulation of cysteine-type endopeptidase activity involved in apoptotic signaling (GO: 2001267) ranked highest among the downregulated GO terms (Figure 5C).
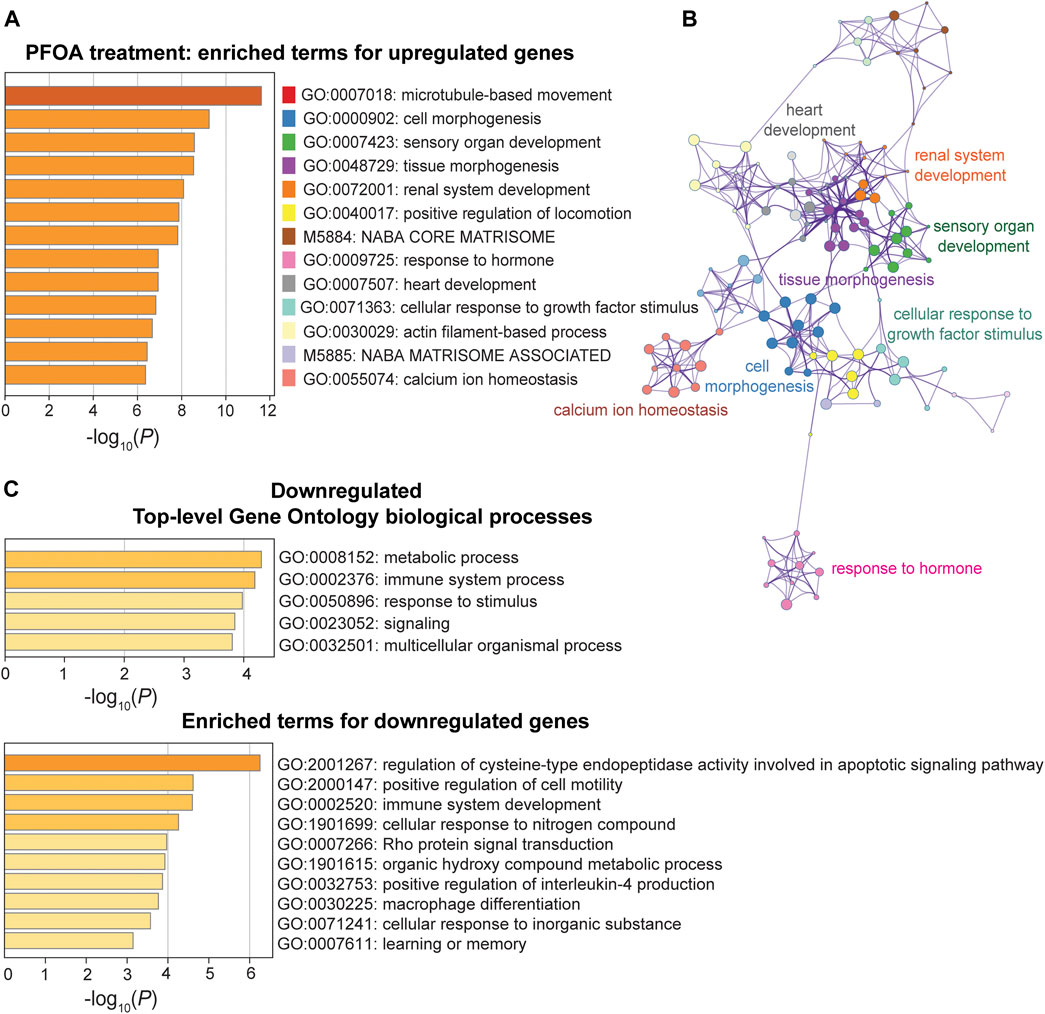
FIGURE 5. Pathway enrichment analysis of DEGs between the PFOA treatment and control groups. (A, C) Enriched functional categories for upregulated and downregulated genes in the PFOA treatment and control groups. Enrichment scores are measured by −log10(p-value). (B) The network plot of enriched terms for upregulated genes. GO terms were represented by the same color dots as in (A).
In the GenX treatment group, upregulated genes were enriched for GO terms involved in blood coagulation (GO:0007596), fatty acid transport (GO:0015908), and negative regulation of peptidase activity (GO:0010466) (p < 10−5; Figure 6A). One upregulated gene, Cd36, was involved in all three of the most significant pathways (Figure 4G). Three additional DEGs, Fabp5, Fabp4, and Plin2, were also involved in fatty acid transport. The DEGs, Pros1, Cd9, Cd36, and Itgb3, were involved in blood coagulation, a pathway that is closely linked to inflammation (Verhamme and Hoylaerts, 2009). Despite the limited number of downregulated DEGs in the GenX group (N = 22), the inflammatory response (GO:0006954), integrin-mediated signaling (GO:0007229), and wound healing (GO:0042060) pathways were significantly enriched (Figure 6B). Of the GenX downregulated genes, the lipoxygenases, Alox15 and Alox12, in addition to Lilrb4, Fn1, and Adra2a, were involved in inflammatory response pathways. Integrin-mediated signaling genes, including integrin’s two subunits, Itgax and Itgam, were also downregulated.
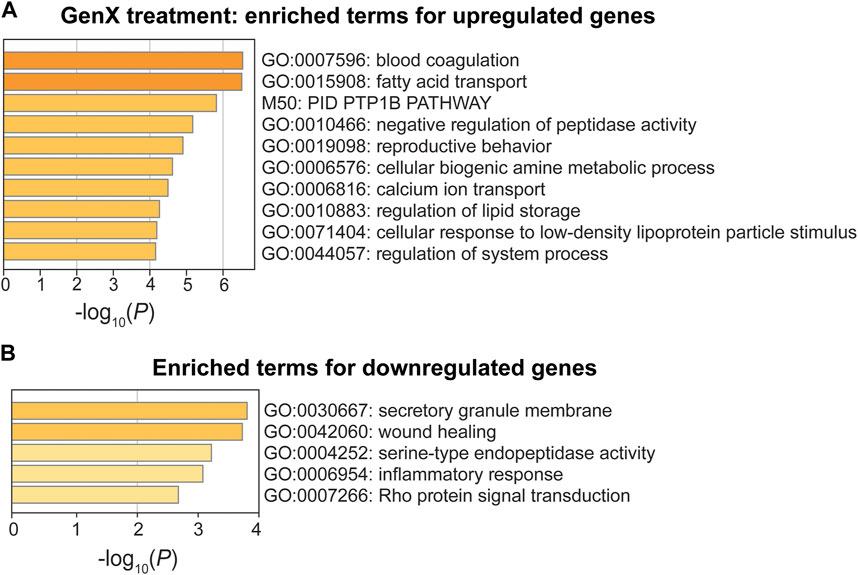
FIGURE 6. Pathway enrichment analysis of DEGs between the GenX treatment and control groups. (A, B) Enriched functional categories for upregulated genes and downregulated genes in the GenX treatment group compared to the control group. Enrichment scores are measured by −log10(p-value).
Discussion
RNA-seq transcriptome analysis of PFAS exposure in a marsupial model
The laboratory opossum is a unique marsupial model for investigating the effects of environmental compounds given the availability of the reference genome and inbred laboratory strains, the ability to annotate genes, and the development of an established breeding protocol. To our knowledge, this is the first in vitro study that investigates the effect of PFAS chemicals on whole blood from the laboratory marsupial, Monodelphis domestica, providing important preliminary data for in vivo research. This study identified significant changes in the expression of hundreds of genes in whole blood culture following exposure to PFOA and GenX, serving as proof of principle for further in vivo toxicological studies.
PFOA exposure altered the expression of genes involved in developmental processes
PFASs are present in both human serum and in the cord blood and milk of pregnant women (Monroy et al., 2008; Sundström et al., 2011), and can be transmitted from the mother to embryos across the placenta and to infants through breast milk (Gyllenhammar et al., 2018; Blake and Fenton, 2020). Serum PFAS levels in children generally exceed those of their mothers (Fromme et al., 2010). Prenatal exposure can affect fetal growth and may cause various adverse health issues, including a disruption in fetal hormone levels, depressed immune functionality, and increased susceptibility to liver injury (Pennings et al., 2016; Nian et al., 2020; Stratakis N et al., 2020), leading to a reduction in fetal growth and birth weight (Johnson et al., 2014; Verner et al., 2015; Li et al., 2017; Starling et al., 2017; Xu et al., 2019). Possible molecular mechanisms include altered maternal metabolic and hormonal profiles, as well as epigenetic modifications of developing embryos and fetuses (Kobayashi et al., 2017; Blake et al., 2020). The current study found that several genes associated with developmental processes were altered by PFOA exposure, including cell/tissue morphogenesis and organ/system development. However, it is important to note that gene expression in whole blood cultures is not a direct measurement of developmental processes. Further investigation of gene expression patterns at early embryonic development stages is required to assess the effect of PFOA on fetal development.
Fatty acid transport functions are enriched in upregulated genes following GenX treatment
Lipid transport, metabolism, and liver function are some of the most susceptible targets to PFAS treatment (Guruge et al., 2006; Fragki et al., 2021). Human epidemiological studies have shown that PFOA and PFOS are positively associated with blood cholesterol levels (Nelson et al., 2010; Eriksen et al., 2013), and animal studies have identified impaired fatty acid homeostasis and transport in rodent models (Curran et al., 2008; Wan et al., 2012).
Previous studies in zebrafish embryos have confirmed that GenX and PFOA both cause a similar level of hepatotoxicity and lipid metabolism dysfunction (Gebreab et al., 2020). The upregulation of fatty acid transport and lipid storage pathways found in GenX-treated laboratory opossums is in accordance with previous findings. This study validated the major genes, including Fabp5, Cd36, and Plin2, that are regulated during fatty acid transport. CD36, or fatty acid translocase, is a membrane glycoprotein that helps to transport long-chain fatty acids through the adipocyte membrane and is the predominant protein responsible for facilitating fatty acid uptake (Hajri and Abumrad, 2002; Glatz and Luiken, 2018). Fatty acid binding proteins (FABPs) bind to CD26 to form a physical complex (Spitsberg et al., 1995), so they are often co-expressed and co-regulated in various tissues (Coburn et al., 2001). GenX treatment is associated with increased expression of both genes. Similar findings were reported in human and rodent liver cell lines (Wu et al., 2017; Sheng et al., 2018). Enhanced expression of CD36 and L-FABP were also observed in GenX-exposed chicken liver tissues, and this was abolished by peroxisome proliferator-activated receptor alpha (PPARα) silencing (Xu et al., 2021). PLIN2 (perilipins) is a ubiquitously expressed lipid droplet protein associated with lipid storage (Brasaemle et al., 1997). This protein protects lipid droplets from lipolysis in the liver, and its downregulation depletes hepatic triglyceride through enhanced autophagy in mouse MEF cells (Tsai et al., 2017). The current studies showed that Plin2 was upregulated in response to GenX treatment, enriching the lipid storage pathway.
A potential limitation of this study is the use of DMSO to prepare the GenX treatment. While several studies have used DMSO as a solvent for GenX (Jabeen et al., 2020; Wen et al., 2020; Hvizdak et al., 2023), recent findings have shown that GenX (HFPO-DA) is degraded to Fluoroether E-1 within 2 h of exposure to >99.5% HPLC-grade DMSO (Liberatore et al., 2020). While GenX was newly prepared prior to use and was diluted immediately to 0.1% in cell culture media, GenX may not remain effective for the full 12-h treatment time. Thus, changes in gene expression observed in the blood transcriptomes may have occurred in response to the degradation product, Fluoroether E-1. EtOH or DI water may be more appropriate solvents for future GenX studies.
Inflammatory responses and immune pathways are enriched in both PFOA and GenX treatment groups
PFAS, especially PFOA and PFOS, are associated with inflammation, cytokine expression, and adaptive and innate immune responses (Sunderland et al., 2019). There is mounting evidence demonstrating that PFAS have an immunotoxic effect on rodents, birds, and reptiles (DeWitt et al., 2009; DeWitt et al., 2012; Woodlief et al., 2021). PFOA and PFOS inhibit T-cell-dependent IgM antibody responses (TDAR) in mice, strongly indicating that immune function has been suppressed (DeWitt et al., 2009; Zheng et al., 2011). A thorough review of 25 epidemiological studies in humans illustrates that PFAS have an immunotoxic effect, reducing the production of antibodies and immune markers (Sunderland et al., 2019). A transcriptomic study in human cord blood also showed that prenatal exposure to PFAS can impair immune functionality in early childhood (Pennings et al., 2016).
The current study showed significantly decreased expression levels of Lgals9, Zfp36l1, Cd40lg, and other genes associated with immunity and inflammation following PFOA treatment. Galectin-9, encoded by Lgals9, belongs to the tandem-repeat subfamily of galectins and is highly expressed in the liver, where it has various effects on innate and adaptive immune responses (Golden-Mason and Rosen, 2017). This protein binds T cell immunoglobulin and mucin domain-containing molecule 3 (TIM-3), which are important immune regulators (Zhu et al., 2005). Zfp36l1 helps to maintain the marginal-zone B cell compartment (Newman et al., 2017) and Cd40lg plays an important role in T cell-dependent immune responses and binds to CD40 on B cells (Miga et al., 2000; Zhou et al., 2009).
GenX treatment increased the expression of Pros1, Cd9, and Cd36, positive regulators of inflammation. Anticoagulant protein S (PROS1) negatively regulates the blood coagulation cascade (Castoldi and Hackeng, 2008) and serves as a ligand for tyrosine kinase (TAM) receptors, which play a fundamental role in regulating inflammatory responses (Lemke, 2013). PROS1 is also upregulated in macrophages when inflammation resolves (Lumbroso et al., 2018). CD9 is a platelet antigen (Mangin et al., 2009), which associates with CD36 on the macrophage surface, leading to the formation of foam cells (Huang et al., 2011). The most significantly downregulated DEG in the inflammatory response pathway, Alox15, encodes a lipoxygenase, which acts to suppress inflammation (Tian et al., 2017). Alox15 expression and metabolite levels were reduced by both PFOA and GenX in an in vitro study of human trophoblast migration (Szilagyi et al., 2020).
The current study found that the expression of several DEGs was similarly impacted by PFOA and GenX treatment, suggesting potential shared mechanisms. While downregulated genes, including Scube2, Anpep, and Itgax, showed more profound repression in response to PFOA than GenX, upregulated genes, including Ttc12, Cdh4, and Fstl4, were more significantly expressed in response to GenX treatment, suggesting that the two chemicals induced different levels of gene expression.
Working toward an established in vivo model of fetal toxicology using Monodelphis domestica
This study broadened the spectrum of current research on the effects of PFOA and GenX treatment on gene expression in whole blood culture from the marsupial model, Monodelphis domestica. The findings that PFAS exposure can lead to significant alterations in developmental processes, fatty acids transport/storage, and immune and inflammatory pathways are consistent with previous rodent and human studies, reinforcing existing knowledge of the susceptible pathways and potential harm induced by PFAS exposure. Monodelphis domestica has several advantages over existing eutherian models in developmental toxicology research. It is an ideal model for evaluating the effects of environmental toxic compounds exposure on early embryonic development because the young are born in a very immature state on embryonic day 13.5, equivalent to a 5.5-week human embryo or an 11.5-day mouse fetus (Mate et al., 1994). Thus, this model provides a unique opportunity to access and examine early embryos without sacrificing the mother or disturbing the developing littermates. The lungs are not well developed when laboratory opossums are born, so much of the respiratory function occurs via the skin. Other major organs, including the brain and digestive and neuronal systems, are still developing (Saunders et al., 1989; Leo and Brunjes, 1999; Modepalli et al., 2018). The extra-uterine embryonic and fetal stages can facilitate time series studies using embryos/pups from the same litter. Another advantage is that laboratory opossums reach sexual maturity at 6 months of age, providing more resolution over laboratory mouse models. This in vitro study provided preliminary results that will inform future in vivo studies in developing embryos that focus on major organs (such as the lung, liver, brain, and kidney). This will help to elucidate the impact of gestational PFOA/GenX exposure via the mother on embryonic stages throughout early development.
Data availability statement
The datasets presented in this study can be found in online repositories. The names of the repository/repositories and accession number(s) can be found below: https://www.ncbi.nlm.nih.gov/, GSE208237.
Ethics statement
The animal study was reviewed and approved by Auburn University Institutional Animal Care and Use Committee.
Author contributions
XW and WC contributed to the conception and design of the study. WC, XW, BS, MP, TK, JS, and PR worked on animal maintenance and sample collection. JV provided the founder animals and advice on colony establishment and management. WC performed the treatment and RNAseq experiments with advice from KH, BS, and XW. WC and XW performed the data analysis. WC and XW wrote the first draft of the manuscript. All authors contributed to manuscript editing and revision, and approved the submitted version.
Funding
This study is supported by an Auburn University College of Veterinary Medicine Animal Health and Disease Research award, and a laboratory start-up fund from Auburn University College of Veterinary Medicine. XW is supported by a USDA National Institute of Food and Agriculture, Hatch project ALA05-2-18041, an Alabama Agriculture Experiment Station (AAES) Agriculture Research Enhancement, Exploration, and Development (AgR-SEED) award, and a National Science Foundation (1928770). WC is supported by the Auburn University Presidential Graduate Research Fellowship, College of Veterinary Medicine Dean’s Fellowship, and by the Alabama EPSCoR Graduate Research Scholars Program.
Acknowledgments
We thank Auburn University Easley Cluster for the computational support of this work.
Conflict of interest
The authors declare that the research was conducted in the absence of any commercial or financial relationships that could be construed as a potential conflict of interest.
Publisher’s note
All claims expressed in this article are solely those of the authors and do not necessarily represent those of their affiliated organizations, or those of the publisher, the editors and the reviewers. Any product that may be evaluated in this article, or claim that may be made by its manufacturer, is not guaranteed or endorsed by the publisher.
Supplementary material
The Supplementary Material for this article can be found online at: https://www.frontiersin.org/articles/10.3389/fgene.2023.1073461/full#supplementary-material
References
Andrews, S. (2010). “FastQC: A quality control tool for high throughput sequence data,” in Babraham bioinformatics (Cambridge, United Kingdom: Babraham Institute).
Beekman, M., Zweers, P., Muller, A., De Vries, W., Janssen, P., and Zeilmaker, M. (2016). Evaluation of substances used in the GenX technology by Chemours. Dordrecht: RIVM letter report.
Blake, B. E., Cope, H. A., Hall, S. M., Keys, R. D., Mahler, B. W., McCord, J., et al. (2020). Evaluation of maternal, embryo, and placental effects in CD-1 mice following gestational exposure to perfluorooctanoic acid (PFOA) or hexafluoropropylene oxide dimer acid (HFPO-da or GenX). Environ. Health Perspect. 128, 27006. doi:10.1289/EHP6233
Blake, B. E., and Fenton, S. E. (2020). Early life exposure to per- and polyfluoroalkyl substances (PFAS) and latent health outcomes: A review including the placenta as a target tissue and possible driver of peri- and postnatal effects. Toxicology 443, 152565. doi:10.1016/j.tox.2020.152565
Bolger, A. M., Lohse, M., and Usadel, B. (2014). Trimmomatic: A flexible trimmer for Illumina sequence data. Bioinformatics 30, 2114–2120. doi:10.1093/bioinformatics/btu170
Brasaemle, D. L., Barber, T., Wolins, N. E., Serrero, G., BlanchetteMackie, E. J., and Londos, C. (1997). Adipose differentiation-related protein is an ubiquitously expressed lipid storage droplet-associated protein. J. Lipid Res. 38, 2249–2263. doi:10.1016/s0022-2275(20)34939-7
Buck, R. C., Franklin, J., Berger, U., Conder, J. M., Cousins, I. T., de Voogt, P., et al. (2011). Perfluoroalkyl and polyfluoroalkyl substances in the environment: Terminology, classification, and origins. Integr. Environ. Assess. Manag. 7, 513–541. doi:10.1002/ieam.258
Castoldi, E., and Hackeng, T. M. (2008). Regulation of coagulation by protein S. Curr. Opin. Hematol. 15, 529–536. doi:10.1097/MOH.0b013e328309ec97
Coburn, C. T., Hajri, T., Ibrahimi, A., and Abumrad, N. A. (2001). Role of CD36 in membrane transport and utilization of long-chain fatty acids by different tissues. J. Mol. Neurosci. 16, 117–121. doi:10.1385/JMN:16:2-3:117
Conley, J. M., Lambright, C. S., Evans, N., McCord, J., Strynar, M. J., Hill, D., et al. (2021). Hexafluoropropylene oxide-dimer acid (HFPO-DA or GenX) alters maternal and fetal glucose and lipid metabolism and produces neonatal mortality, low birthweight, and hepatomegaly in the Sprague-Dawley rat. Environ. Int. 146, 106204. doi:10.1016/j.envint.2020.106204
Curran, I., Hierlihy, S. L., Liston, V., Pantazopoulos, P., Nunnikhoven, A., Tittlemier, S., et al. (2008). Altered fatty acid homeostasis and related toxicologic sequelae in rats exposed to dietary potassium perfluorooctanesulfonate (PFOS). J. Toxicol. Environ. Health A 71, 1526–1541. doi:10.1080/15287390802361763
DeWitt, J. C., Peden-Adams, M. M., Keller, J. M., and Germolec, D. R. (2012). Immunotoxicity of perfluorinated compounds: Recent developments. Toxicol. Pathol. 40, 300–311. doi:10.1177/0192623311428473
DeWitt, J. C., Shnyra, A., Badr, M. Z., Loveless, S. E., Hoban, D., Frame, S. R., et al. (2009). Immunotoxicity of perfluorooctanoic acid and perfluorooctane sulfonate and the role of peroxisome proliferator-activated receptor alpha. Crit. Rev. Toxicol. 39, 76–94. doi:10.1080/10408440802209804
Dobin, A., Davis, C. A., Schlesinger, F., Drenkow, J., Zaleski, C., Jha, S., et al. (2013). Star: Ultrafast universal RNA-seq aligner. Bioinformatics 29, 15–21. doi:10.1093/bioinformatics/bts635
Ehresman, D. J., Froehlich, J. W., Olsen, G. W., Chang, S. C., and Butenhoff, J. L. (2007). Comparison of human whole blood, plasma, and serum matrices for the determination of perfluorooctanesulfonate (PFOS), perfluorooctanoate (PFOA), and other fluorochemicals. Environ. Res. 103, 176–184. doi:10.1016/j.envres.2006.06.008
Eriksen, K. T., Raaschou-Nielsen, O., McLaughlin, J. K., Lipworth, L., Tjonneland, A., Overvad, K., et al. (2013). Association between plasma PFOA and PFOS levels and total cholesterol in a middle-aged Danish population. Plos One 8, e56969. doi:10.1371/journal.pone.0056969
Fadem, B. H., Trupin, G. L., Maliniak, E., VandeBerg, J. L., and Hayssen, V. (1982). Care and breeding of the gray, short-tailed opossum (Monodelphis domestica). Lab. Anim. Sci. 32, 405–409.
Fragki, S., Dirven, H., Fletcher, T., Grasl-Kraupp, B., Bjerve Gützkow, K., Hoogenboom, R., et al. (2021). Systemic PFOS and PFOA exposure and disturbed lipid homeostasis in humans: What do we know and what not? Crit. Rev. Toxicol. 51, 141–164. doi:10.1080/10408444.2021.1888073
Fromme, H., Mosch, C., Morovitz, M., Alba-Alejandre, I., Boehmer, S., Kiranoglu, M., et al. (2010). Pre- and postnatal exposure to perfluorinated compounds (PFCs). Environ. Sci. Technol. 44, 7123–7129. doi:10.1021/es101184f
Gebbink, W. A., van Asseldonk, L., and van Leeuwen, S. P. J. (2017). Presence of emerging per- and polyfluoroalkyl substances (PFASs) in river and drinking water near a fluorochemical production plant in The Netherlands. Environ. Sci. Technol. 51, 11057–11065. doi:10.1021/acs.est.7b02488
Gebreab, K. Y., Eeza, M. N. H., Bai, T., Zuberi, Z., Matysik, J., O'Shea, K. E., et al. (2020). Comparative toxicometabolomics of perfluorooctanoic acid (PFOA) and next-generation perfluoroalkyl substances. Environ. Pollut. 265, 114928. doi:10.1016/j.envpol.2020.114928
Giesy, J. P., and Kannan, K. (2002). Perfluorochemical surfactants in the environment. Perfluorochem. surfactants Environ. 36, 146A–152A. doi:10.1021/es022253t
Glatz, J. F. C., and Luiken, J. (2018). Dynamic role of the transmembrane glycoprotein CD36 (SR-B2) in cellular fatty acid uptake and utilization. J. Lipid Res. 59, 1084–1093. doi:10.1194/jlr.R082933
Golden-Mason, L., and Rosen, H. R. (2017). Galectin-9: Diverse roles in hepatic immune homeostasis and inflammation. Hepatology 66, 271–279. doi:10.1002/hep.29106
Gomis, M. I., Vestergren, R., Borg, D., and Cousins, I. T. (2018). Comparing the toxic potency in vivo of long-chain perfluoroalkyl acids and fluorinated alternatives. Environ. Int. 113, 1–9. doi:10.1016/j.envint.2018.01.011
Gordon, S. C. (2011). Toxicological evaluation of ammonium 4, 8-dioxa-3H-perfluorononanoate, a new emulsifier to replace ammonium perfluorooctanoate in fluoropolymer manufacturing. Regul. Toxicol. Pharmacol. 59, 64–80. doi:10.1016/j.yrtph.2010.09.008
Grønnestad, R., Johanson, S. M., Müller, M. H. B., Schlenk, D., Tanabe, P., Krøkje, Å., et al. (2021). Effects of an environmentally relevant PFAS mixture on dopamine and steroid hormone levels in exposed mice. Toxicol. Appl. Pharmacol. 428, 115670. doi:10.1016/j.taap.2021.115670
Guruge, K. S., Yeung, L. W., Yamanaka, N., Miyazaki, S., Lam, P. K., Giesy, J. P., et al. (2006). Gene expression profiles in rat liver treated with perfluorooctanoic acid (PFOA). Toxicol. Sci. 89, 93–107. doi:10.1093/toxsci/kfj011
Gyllenhammar, I., Benskin, J. P., Sandblom, O., Berger, U., Ahrens, L., Lignell, S., et al. (2018). Perfluoroalkyl acids (PFAAs) in serum from 2-4-month-old infants: Influence of maternal serum concentration, gestational age, breast-feeding, and contaminated drinking water. Environ. Sci. Technol. 52, 7101–7110. doi:10.1021/acs.est.8b00770
Hajri, T., and Abumrad, N. A. (2002). Fatty acid transport across membranes: Relevance to nutrition and metabolic pathology. Annu. Rev. Nutr. 22, 383–415. doi:10.1146/annurev.nutr.22.020402.130846
Huang, W., Febbraio, M., and Silverstein, R. L. (2011). CD9 tetraspanin interacts with CD36 on the surface of macrophages: A possible regulatory influence on uptake of oxidized low density lipoprotein. PLoS One 6, e29092. doi:10.1371/journal.pone.0029092
Hvizdak, M., Kandel, S. E., Work, H. M., Gracey, E. G., McCullough, R. L., and Lampe, J. N. (2023). Per- and polyfluoroalkyl substances (PFAS) inhibit cytochrome P450 CYP3A7 through direct coordination to the heme iron and water displacement. J. Inorg. Biochem. 240, 112120. doi:10.1016/j.jinorgbio.2023.112120
Imir, O. B., Kaminsky, A. Z., Zuo, Q. Y., Liu, Y. J., Singh, R., Spinella, M. J., et al. (2021). Per- and polyfluoroalkyl substance exposure combined with high-fat diet supports prostate cancer progression. Nutrients 13, 3902. doi:10.3390/nu13113902
Jabeen, M., Fayyaz, M., and Irudayaraj, J. (2020). Epigenetic modifications, and alterations in cell cycle and apoptosis pathway in A549 lung carcinoma cell line upon exposure to perfluoroalkyl substances. Toxics 8, 112. doi:10.3390/toxics8040112
Johnson, P. I., Sutton, P., Atchley, D. S., Koustas, E., Lam, J., Sen, S., et al. (2014). The navigation guide-evidence-based medicine meets environmental health: Systematic review of human evidence for PFOA effects on fetal growth. Environ. Health Perspect. 122, 1028–1039. doi:10.1289/ehp.1307893
Jones, P. D., Hu, W., De Coen, W., Newsted, J. L., and Giesy, J. P. (2003). Binding of perfluorinated fatty acids to serum proteins. Environ. Toxicol. Chem. 22, 2639–2649. doi:10.1897/02-553
Kobayashi, S., Azumi, K., Goudarzi, H., Araki, A., Miyashita, C., Kobayashi, S., et al. (2017). Effects of prenatal perfluoroalkyl acid exposure on cord blood IGF2/H19 methylation and ponderal index: The Hokkaido Study. J. Expo. Sci. Environ. Epidemiol. 27, 251–259. doi:10.1038/jes.2016.50
Langmead, B., and Salzberg, S. L. (2012). Fast gapped-read alignment with Bowtie 2. Nat. Methods 9, 357–359. doi:10.1038/nmeth.1923
Lemke, G. (2013). Biology of the TAM receptors. Cold Spring Harb. Perspect. Biol. 5, a009076. doi:10.1101/cshperspect.a009076
Leo, J. M. C., and Brunjes, P. C. (1999). Developmental analysis of the peripheral olfactory organ of the opossum Monodelphis domestica. Dev. Brain Res. 114, 43–48. doi:10.1016/s0165-3806(99)00017-6
Li, M., Zeng, X. W., Qian, Z. M., Vaughn, M. G., Sauve, S., Paul, G., et al. (2017). Isomers of perfluorooctanesulfonate (PFOS) in cord serum and birth outcomes in China: Guangzhou Birth Cohort Study. Environ. Int. 102, 1–8. doi:10.1016/j.envint.2017.03.006
Liberatore, H. K., Jackson, S. R., Strynar, M. J., and McCord, J. P. (2020). Solvent suitability for HFPO-da ("GenX" parent acid) in toxicological studies. Environ. Sci. Technol. Lett. 7, 477–481. doi:10.1021/acs.estlett.0c00323
Lumbroso, D., Soboh, S., Maimon, A., Schif-Zuck, S., Ariel, A., and Burstyn-Cohen, T. (2018). Macrophage-derived protein S facilitates apoptotic polymorphonuclear cell clearance by resolution phase macrophages and supports their reprogramming. Front. Immunol. 9, 358. doi:10.3389/fimmu.2018.00358
Luo, Z. X., Yuan, C. X., Meng, Q. J., and Ji, Q. (2011). A Jurassic eutherian mammal and divergence of marsupials and placentals. Nature 476, 442–445. doi:10.1038/nature10291
Mangin, P. H., Kleitz, L., Boucheix, C., Gachet, C., and Lanza, F. (2009). CD9 negatively regulates integrin alphaIIbbeta3 activation and could thus prevent excessive platelet recruitment at sites of vascular injury. J. Thromb. Haemost. 7, 900–902. doi:10.1111/j.1538-7836.2009.03322.x
Mate, K. E., Robinson, E. S., VandeBerg, J. L., and Pedersen, R. A. (1994). Timetable of in vivo embryonic development in the grey short-tailed opossum (Monodelphis domestica). Mol. Reprod. Dev. 39, 365–374. doi:10.1002/mrd.1080390404
McDonough, C. A., Ward, C., Hu, Q., Vance, S., Higgins, C. P., and DeWitt, J. C. (2020). Immunotoxicity of an electrochemically fluorinated aqueous film-forming foam. Toxicol. Sci. 178, 104–114. doi:10.1093/toxsci/kfaa138
Miga, A., Masters, S., Gonzalez, M., and Noelle, R. J. (2000). The role of CD40-CD154 interactions in the regulation of cell mediated immunity. Immunol. Invest. 29, 111–114. doi:10.3109/08820130009062292
Mikkelsen, T. S., Wakefield, M. J., Aken, B., Amemiya, C. T., Chang, J. L., Duke, S., et al. (2007). Genome of the marsupial Monodelphis domestica reveals innovation in non-coding sequences. Nature 447, 167–177. doi:10.1038/nature05805
Modepalli, V., Kumar, A., Sharp, J. A., Saunders, N. R., Nicholas, K. R., and Lefevre, C. (2018). Gene expression profiling of postnatal lung development in the marsupial gray short-tailed opossum (Monodelphis domestica) highlights conserved developmental pathways and specific characteristics during lung organogenesis. BMC Genomics 19, 732. doi:10.1186/s12864-018-5102-2
Monroy, R., Morrison, K., Teo, K., Atkinson, S., Kubwabo, C., Stewart, B., et al. (2008). Serum levels of perfluoroalkyl compounds in human maternal and umbilical cord blood samples. Environ. Res. 108, 56–62. doi:10.1016/j.envres.2008.06.001
Nelson, J. W., Hatch, E. E., and Webster, T. F. (2010). Exposure to polyfluoroalkyl chemicals and cholesterol, body weight, and insulin resistance in the general US population. Environ. Health Perspect. 118, 197–202. doi:10.1289/ehp.0901165
Newman, R., Ahlfors, H., Saveliev, A., Galloway, A., Hodson, D. J., Williams, R., et al. (2017). Maintenance of the marginal-zone B cell compartment specifically requires the RNA-binding protein ZFP36L1. Nat. Immunol. 18, 683–693. doi:10.1038/ni.3724
Nian, M., Luo, K., Luo, F., Aimuzi, R., Huo, X., Chen, Q., et al. (2020). Association between prenatal exposure to PFAS and fetal sex hormones: Are the short-chain PFAS safer? Environ. Sci. Technol. 54, 8291–8299. doi:10.1021/acs.est.0c02444
Olsen, G. W., Burris, J. M., Ehresman, D. J., Froehlich, J. W., Seacat, A. M., Butenhoff, J. L., et al. (2007). Half-life of serum elimination of perfluorooctanesulfonate,perfluorohexanesulfonate, and perfluorooctanoate in retired fluorochemical production workers. Environ. Health Perspect. 115, 1298–1305. doi:10.1289/ehp.10009
Pennings, J. L. A., Jennen, D. G. J., Nygaard, U. C., Namork, E., Haug, L. S., van Loveren, H., et al. (2016). Cord blood gene expression supports that prenatal exposure to perfluoroalkyl substances causes depressed immune functionality in early childhood. J. Immunotoxicol. 13, 173–180. doi:10.3109/1547691X.2015.1029147
Post, G. B., Cohn, P. D., and Cooper, K. R. (2012). Perfluorooctanoic acid (PFOA), an emerging drinking water contaminant: A critical review of recent literature. Environ. Res. 116, 93–117. doi:10.1016/j.envres.2012.03.007
Quinlan, A. R., and Hall, I. M. (2010). BEDTools: A flexible suite of utilities for comparing genomic features. Bioinformatics 26, 841–842. doi:10.1093/bioinformatics/btq033
Robinson, M. D., McCarthy, D. J., and Smyth, G. K. (2010). edgeR: a Bioconductor package for differential expression analysis of digital gene expression data. Bioinformatics 26, 139–140. doi:10.1093/bioinformatics/btp616
Roth, K., Yang, Z., Agarwal, M., Liu, W., Peng, Z., Long, Z., et al. (2021). Exposure to a mixture of legacy, alternative, and replacement per- and polyfluoroalkyl substances (PFAS) results in sex-dependent modulation of cholesterol metabolism and liver injury. Environ. Int. 157, 106843. doi:10.1016/j.envint.2021.106843
Samollow, P. B. (2008). The opossum genome: Insights and opportunities from an alternative mammal. Genome Res. 18, 1199–1215. doi:10.1101/gr.065326.107
Saunders, N. R., Adam, E., Reader, M., and Mollgard, K. (1989). Monodelphis domestica (grey short-tailed opossum): An accessible model for studies of early neocortical development. Anat. Embryol. Berl. 180, 227–236. doi:10.1007/BF00315881
Sheng, N., Cui, R. N., Wang, J. H., Guo, Y., Wang, J. S., and Dai, J. Y. (2018). Cytotoxicity of novel fluorinated alternatives to long-chain perfluoroalkyl substances to human liver cell line and their binding capacity to human liver fatty acid binding protein. Archives Toxicol. 92, 359–369. doi:10.1007/s00204-017-2055-1
Spitsberg, V. L., Matitashvili, E., and Gorewit, R. C. (1995). Association and coexpression of fatty-acid-binding protein and glycoprotein CD36 in the bovine mammary gland. Eur. J. Biochem. 230, 872–878. doi:10.1111/j.1432-1033.1995.tb20630.x
Starling, A. P., Adgate, J. L., Hamman, R. F., Kechris, K., Calafat, A. M., Ye, X. Y., et al. (2017). Perfluoroalkyl substances during pregnancy and offspring weight and adiposity at birth: Examining mediation by maternal fasting glucose in the healthy start study. Environ. Health Perspect. 125, 067016. doi:10.1289/EHP641
Stratakis N, D. V. C., Jin, R., Margetaki, K., Valvi, D., Siskos, A. P., Maitre, L., et al. (2020). Prenatal exposure to perfluoroalkyl substances associated with increased susceptibility to liver injury in children. Hepatology 72, 1758–1770. doi:10.1002/hep.31483
Strynar, M., Dagnino, S., McMahen, R., Liang, S., Lindstrom, A., Andersen, E., et al. (2015). Identification of novel perfluoroalkyl ether carboxylic acids (PFECAs) and sulfonic acids (PFESAs) in natural waters using accurate mass time-of-flight mass spectrometry (TOFMS). Environ. Sci. Technol. 49, 11622–11630. doi:10.1021/acs.est.5b01215
Suh, C. H., Cho, N. K., Lee, C. K., Lee, C. H., Kim, D. H., Kim, J. H., et al. (2011). Perfluorooctanoic acid-induced inhibition of placental prolactin-family hormone and fetal growth retardation in mice. Mol. Cell Endocrinol. 337, 7–15. doi:10.1016/j.mce.2011.01.009
Sun, M., Arevalo, E., Strynar, M., Lindstrom, A., Richardson, M., Kearns, B., et al. (2016). Legacy and emerging perfluoroalkyl substances are important drinking water contaminants in the cape fear river watershed of North Carolina. Environ. Sci. Technol. Lett. 3, 415–419. doi:10.1021/acs.estlett.6b00398
Sunderland, E. M., Hu, X. C., Dassuncao, C., Tokranov, A. K., Wagner, C. C., and Allen, J. G. (2019). A review of the pathways of human exposure to poly- and perfluoroalkyl substances (PFASs) and present understanding of health effects. J. Expo. Sci. Environ. Epidemiol. 29, 131–147. doi:10.1038/s41370-018-0094-1
Sundström, M., Ehresman, D. J., Bignert, A., Butenhoff, J. L., Olsen, G. W., Chang, S. C., et al. (2011). A temporal trend study (1972-2008) of perfluorooctanesulfonate, perfluorohexanesulfonate, and perfluorooctanoate in pooled human milk samples from Stockholm, Sweden. Environ. Int. 37, 178–183. doi:10.1016/j.envint.2010.08.014
Szilagyi, J. T., Freedman, A. N., Kepper, S. L., Keshava, A. M., Bangma, J. T., and Fry, R. C. (2020). Per- and polyfluoroalkyl substances differentially inhibit placental trophoblast migration and invasion in vitro. Toxicol. Sci. 175, 210–219. doi:10.1093/toxsci/kfaa043
Tarapore, P., and Ouyang, B. (2021). Perfluoroalkyl chemicals and male reproductive health: Do PFOA and PFOS increase risk for male infertility? Int. J. Environ. Res. Public Health 18, 3794. doi:10.3390/ijerph18073794
Tian, R., Zuo, X. S., Jaoude, J., Mao, F., Colby, J., and Shureiqi, I. (2017). ALOX15 as a suppressor of inflammation and cancer: Lost in the link. Prostagl. Other Lipid Mediat. 132, 77–83. doi:10.1016/j.prostaglandins.2017.01.002
Trapnell, C., Pachter, L., and Salzberg, S. L. (2009). TopHat: Discovering splice junctions with RNA-seq. Bioinformatics 25, 1105–1111. doi:10.1093/bioinformatics/btp120
Tsai, T. H., Chen, E., Li, L., Saha, P., Lee, H. J., Huang, L. S., et al. (2017). The constitutive lipid droplet protein PLIN2 regulates autophagy in liver. Autophagy 13, 1130–1144. doi:10.1080/15548627.2017.1319544
van Rheede, T., Bastiaans, T., Boone, D. N., Hedges, S. B., de Jong, W. W., and Madsen, O. (2006). The platypus is in its place: Nuclear genes and indels confirm the sister group relation of monotremes and therians. Mol. Biol. Evol. 23, 587–597. doi:10.1093/molbev/msj064
Verhamme, P., and Hoylaerts, M. F. (2009). Hemostasis and inflammation: Two of a kind? Thromb. J. 7, 15. doi:10.1186/1477-9560-7-15
Verner, M. A., Loccisano, A. E., Morken, N. H., Yoon, M., Wu, H., McDougall, R., et al. (2015). Associations of perfluoroalkyl substances (PFAS) with lower birth weight: An evaluation of potential confounding by glomerular filtration rate using a physiologically based pharmacokinetic model (PBPK). Environ. Health Perspect. 123, 1317–1324. doi:10.1289/ehp.1408837
Verreault, J., Houde, M., Gabrielsen, G. W., Berger, U., Haukas, M., Letcher, R. J., et al. (2005). Perfluorinated alkyl substances in plasma, liver, brain, and eggs of glaucous gulls (Larus hyperboreus) from the Norwegian arctic. Environ. Sci. Technol. 39, 7439–7445. doi:10.1021/es051097y
Wan, H. T., Zhao, Y. G., Wei, X., Hui, K. Y., Giesy, J. P., and Wong, C. K. (2012). PFOS-induced hepatic steatosis, the mechanistic actions on beta-oxidation and lipid transport. Biochim. Biophys. Acta 1820, 1092–1101. doi:10.1016/j.bbagen.2012.03.010
Wang, Z., Cousins, I. T., Scheringer, M., and Hungerbuehler, K. (2015). Hazard assessment of fluorinated alternatives to long-chain perfluoroalkyl acids (PFAAs) and their precursors: Status quo, ongoing challenges and possible solutions. Environ. Int. 75, 172–179. doi:10.1016/j.envint.2014.11.013
Wang, Z. Y., Cousins, I. T., Scheringer, M., and Hungerbuhler, K. (2013). Fluorinated alternatives to long-chain perfluoroalkyl carboxylic acids (PFCAs), perfluoroalkane sulfonic acids (PFSAs) and their potential precursors. Environ. Int. 60, 242–248. doi:10.1016/j.envint.2013.08.021
Wen, Y., Mirji, N., and Irudayaraj, J. (2020). Epigenetic toxicity of PFOA and GenX in HepG2 cells and their role in lipid metabolism. Toxicol. Vitro 65, 104797. doi:10.1016/j.tiv.2020.104797
Woodburne, M. O., Rich, T. H., and Springer, M. S. (2003). The evolution of tribospheny and the antiquity of mammalian clades. Mol. Phylogenet Evol. 28, 360–385. doi:10.1016/s1055-7903(03)00113-1
Woodlief, T., Vance, S., Hu, Q., and DeWitt, J. (2021). Immunotoxicity of per- and polyfluoroalkyl substances: Insights into short-chain PFAS exposure. Toxics 9, 100. doi:10.3390/toxics9050100
Wu, X., Liang, M., Yang, Z., Su, M., and Yang, B. (2017). Effect of acute exposure to PFOA on mouse liver cells in vivo and in vitro. Environ. Sci. Pollut. Res. Int. 24, 24201–24206. doi:10.1007/s11356-017-0072-5
Xiong, X., Samollow, P. B., Cao, W., Metz, R., Zhang, C., Leandro, A. C., et al. (2022). Genetic and genomic architecture in eight strains of the laboratory opossum Monodelphis domestica. G3 (Bethesda) 12, jkab389. doi:10.1093/g3journal/jkab389
Xu, C., Yin, S., Liu, Y., Chen, F., Zhong, Z., Li, F., et al. (2019). Prenatal exposure to chlorinated polyfluoroalkyl ether sulfonic acids and perfluoroalkyl acids: Potential role of maternal determinants and associations with birth outcomes. J. Hazard Mater 380, 120867. doi:10.1016/j.jhazmat.2019.120867
Xu, X., Ni, H., Guo, Y., Lin, Y., Ji, J., Jin, C., et al. (2021). Hexafluoropropylene oxide dimer acid (HFPO-DA) induced developmental cardiotoxicity and hepatotoxicity in hatchling chickens: Roles of peroxisome proliferator activated receptor alpha. Environ. Pollut. 290, 118112. doi:10.1016/j.envpol.2021.118112
Yang, L. H., Yang, W. J., Lv, S. H., Zhu, T. T., Adeel Sharif, H. M., Yang, C., et al. (2022). Is HFPO-da (GenX) a suitable substitute for PFOA? A comprehensive degradation comparison of PFOA and GenX via electrooxidation. Environ. Res. 204, 111995. doi:10.1016/j.envres.2021.111995
Zhang, S., Chen, K., Li, W., Chai, Y., Zhu, J., Chu, B., et al. (2021). Varied thyroid disrupting effects of perfluorooctanoic acid (PFOA) and its novel alternatives hexafluoropropylene-oxide-dimer-acid (GenX) and ammonium 4,8-dioxa-3H-perfluorononanoate (ADONA) in vitro. Environ. Int. 156, 106745. doi:10.1016/j.envint.2021.106745
Zheng, L., Dong, G. H., Zhang, Y. H., Liang, Z. F., Jin, Y. H., and He, Q. C. (2011). Type 1 and Type 2 cytokines imbalance in adult male C57BL/6 mice following a 7-day oral exposure to perfluorooctanesulfonate (PFOS). J. Immunotoxicol. 8, 30–38. doi:10.3109/1547691X.2010.537287
Zhou, Y., Yuan, J., Pan, Y., Fei, Y., Qiu, X., Hu, N., et al. (2009). T cell CD40LG gene expression and the production of IgG by autologous B cells in systemic lupus erythematosus. Clin. Immunol. 132, 362–370. doi:10.1016/j.clim.2009.05.011
Zhou, Y., Zhou, B., Pache, L., Chang, M., Khodabakhshi, A. H., Tanaseichuk, O., et al. (2019). Metascape provides a biologist-oriented resource for the analysis of systems-level datasets. Nat. Commun. 10, 1523. doi:10.1038/s41467-019-09234-6
Keywords: per-and polyfluoroalkyl substances (PFAS), laboratory opossum, long-chain fatty acid transport, inflammatory response, developmental processes, toxicology
Citation: Cao W, Horzmann K, Schemera B, Petrofski M, Kendall T, Spooner J, Rynders PE, VandeBerg JL and Wang X (2023) Blood transcriptome responses to PFOA and GenX treatment in the marsupial biomedical model Monodelphis domestica. Front. Genet. 14:1073461. doi: 10.3389/fgene.2023.1073461
Received: 18 October 2022; Accepted: 01 February 2023;
Published: 15 February 2023.
Edited by:
J. Chris Corton, United States Environmental Protection Agency, United StatesReviewed by:
Kevin Gerrish, National Institute of Environmental Health Sciences (NIH), United StatesGyanendra Singh, National Institute of Occupational Health (ICMR), India
Copyright © 2023 Cao, Horzmann, Schemera, Petrofski, Kendall, Spooner, Rynders, VandeBerg and Wang. This is an open-access article distributed under the terms of the Creative Commons Attribution License (CC BY). The use, distribution or reproduction in other forums is permitted, provided the original author(s) and the copyright owner(s) are credited and that the original publication in this journal is cited, in accordance with accepted academic practice. No use, distribution or reproduction is permitted which does not comply with these terms.
*Correspondence: Xu Wang, eHp3MDA3MEBhdWJ1cm4uZWR1
†ORCID: Xu Wang, orcid.org/0000-0002-7594-5004