- 1Department of Animal Science, Rivers State University, Port Harcourt, Rivers State, Nigeria
- 2Department of Animal Science, Federal University of Agriculture, Zuru, Kebbi State, Nigeria
- 3Department of Estate Management, Faculty of Environmental Sciences, Rivers State University, Port Harcourt, Nigeria
- 4Department of Animal and Environmental Biology, Rivers State University, Port Harcourt, Rivers State, Nigeria
- 5Insititute of Feed Research, Chinese Academy of Agricultural Sciences, Beijing, China
- 6Department of Animal Science, College of Agricultural Science, Landmark University, Omu-aran, Kwara State, Nigeria
- 7Department of Agriculture, Animal Science Programme, Alex-Ekwueme Federal University, Ikwo, Ebonyi, Nigeria
- 8Department of Medical Laboratory Science, Faculty of Science, Rivers State University, Port Harcourt, Rivers State, Nigeria
Sustenance of smallholder poultry production as an alternative source of food security and income is imperative in communities exposed to hydrocarbon pollution. Exposure to hydrocarbon pollutants causes disruption of homeostasis, thereby compromising the genetic potential of the birds. Oxidative stress-mediated dysfunction of the cellular membrane is a contributing factor in the mechanism of hydrocarbon toxicity. Epidemiological studies show that tolerance to hydrocarbon exposure may be caused by the activation of genes that control disease defense pathways like aryl hydrocarbon receptor (AhR) and nuclear factor erythroid 2p45-related factor 2 (Nrf2). Disparity in the mechanism and level of tolerance to hydrocarbon fragments among species may exist and may result in variations in gene expression within individuals of the same species upon exposure. Genomic variability is critical for adaptation and serves as a survival mechanism in response to environmental pollutants. Understanding the interplay of diverse genetic mechanisms in relation to environmental influences is important for exploiting the differences in various genetic variants. Protection against pollutant-induced physiological responses using dietary antioxidants can mitigate homeostasis disruptions. Such intervention may initiate epigenetic modulation relevant to gene expression of hydrocarbon tolerance, enhancing productivity, and possibly future development of hydrocarbon-tolerant breeds.
Introduction
Hydrocarbon pollution from oil spillage, natural gas flaring and organic pollutants is ubiquitous and of some fundamental health welfare concern in communities exposed to that pollution such as the Niger Delta region of Nigeria (Sam and Zabbey, 2018; Srivastava et al., 2019). The degradation of the environment by these pollutants destabilizes the ecosystem (Anejionu et al., 2015; Kponee et al., 2015; Croitoru et al., 2020), leading to the loss of biodiversity of organisms and low productivity of aquatic organisms, which serve as the major source of livelihood and animal protein supply to rural households (Izah, 2015; Osuagwu and Olaifa, 2018; Ansah et al., 2022). Diversification of income sources was recommended as a crucial strategy for achieving poverty reduction and economic stability in order to increase resilience and reduce the vulnerability of the rural poor to the effects of environmental deterioration (Yakubu, 2017). Poultry may be a good substitute due to its intrinsic qualities of having a short generational gap, high prolificacy, rapid turnover, and acceptance across all socio-cultural, economic, and religious strata (Oleforuh-Okoleh, 2010).
Due to their toxic effects, environmental stresses such as those caused by hydrocarbon pollutants may prevent the full expression of the genetic potential of these birds. The severity of hydrocarbon toxicity is dependent on the exposure route, type of chemical compound, dosage, and duration of exposure. Hydrocarbon tolerance is regulated by biological (metabolic, genetic, and signal transduction) pathways whose expression upon exposure may create species-specific tolerance variances (Miller et al., 2018). Interactions between different classes of pollutants, the generation of reactive oxygen species (ROS), and the onset of oxidative stress conditions are partly modulated by changes in the levels and functions of redox-sensitive signaling proteins and transcription factors (Regoli and Giuliani, 2014). Modulation of the biological pathways using enzymatic and non-enzymatic molecules that can prevent metabolic dysfunctions caused by oxidative stress becomes expedient (Figure 1). There is evidence of effective dietary interventions using exogenous antioxidants to regulate the impact of environmental perturbances on organisms and hence gene expression with regards to tolerance (Hennig et al., 2007; Hoffman et al., 2017; Andreescu et al., 2018; Ideraabdullah and Zeisel, 2018).
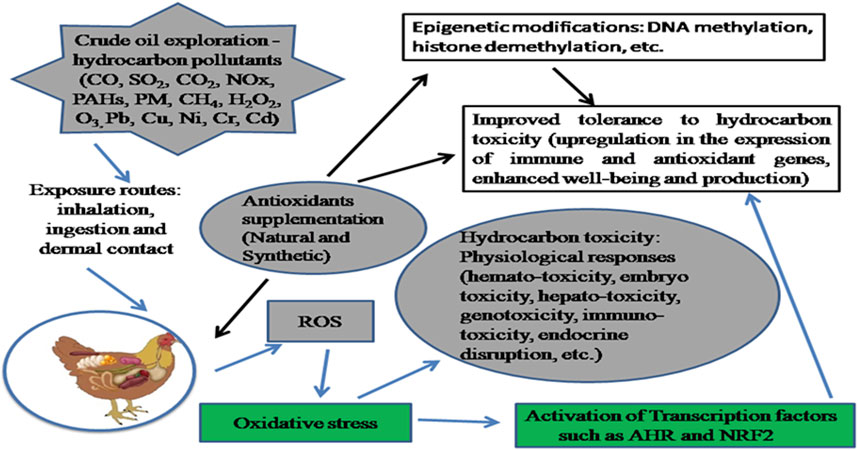
FIGURE 1. Consequence of hydrocarbon exposure and mitigation effect of antioxidant modulation. Crude oil exploration results in the release of hydrocarbon pollutants to the environment. Exposure to these pollutants via inhalation, dermal contact and/or ingestion results in interactions between different classes of pollutants, generation of reactive oxygen species and onset of oxidative stress conditions which are partly modulated by redox-sensitive signalling proteins and transcription factors. The mechanism of tolerance to hydrocarbon toxicity involves the activation of the aryl hydrocarbon receptor (AHR) and nuclear factor erythroid 2p45-related factor 2 (Nrf2) signalling pathways which regulates the expression of xenobiotic metabolism genes such as the cytochrome P450. To mitigate the impact of exposure on metabolic processes and enhance productivity, dietary interventions in regulating environmental perturbances could be beneficial.
Epidemiological studies indicate that oxidative stress modulates the organism’s epigenome and thus regulates gene expression (Malireddy et al., 2012; Hedman et al., 2016). Karchner et al. (2006) and Bianchini and Morrissey (2020) highlighted the fact that there are species-specific differences in the mechanism and level of tolerance to hydrocarbon fragments. This disparity could be observed between chickens raised in severely hydrocarbon-polluted environments and those raised in unaffected areas. The extent of vulnerability could be due to evolutionary capability being dependent on factors such as the genetic structure and strength of the selection pressure (Dutilleul et al., 2015). Modifications of the genetic structures, such as those associated with fecundity, survival, and morphology, are experiential (Nacci et al., 2002; Charmantier and Garant, 2005). Whitehead et al. (2017) asserted that the genetic architecture core to adaptive traits may interrelate to form the possibility of evolutionary rescue from pollution. Thus, genomic variability is critical for adaptation and serves as a survival mechanism in response to environmental pollutants (Oziolor and Matson, 2015).
Crucial to the successful establishment of smallholder poultry production in regions affected by hydrocarbon pollution is, therefore, an understanding of the impact of hydrocarbon pollutants on poultry with respect to gene-environment interaction as well as the mitigation effect of such an impact using exogenous antioxidants. This review highlights some physiological dysfunctions arising from exposure to hydrocarbon pollutants while noting some mechanisms of hydrocarbon toxicity and connecting dietary modulation to possible epigenetic changes towards improving tolerance to enhance productivity.
Hydrocarbon pollution and physiological responses of poultry
Pollutants (both primary and secondary) from crude oil exploration activities like gaseous emissions from incomplete fossil fuel combustion and petrochemical industries, particulate matter (PM), polycyclic aromatic hydrocarbons (PAH), volatile organic compounds (VOC), and heavy metals (Pb, Cu, Ni, Cr, and Cd) pose adverse health challenges not only to humans but to other organisms within the ecosystem (Table 1). The interference of free radicals and reactive oxygen species (ROS) on the function of the cellular membrane as well as the enzymatic systems could possibly explain the mechanism of hydrocarbon toxicity. Though free radicals in the body system are involved in normal cellular functions and are essential in reduction-oxidation reactions and other physiological responses, maintenance of the balance between oxidation and anti-oxidation is critical (Bouayed and Bohn, 2010). Exposure of populations to hydrocarbon pollutants results in an imbalance between free radicals and the cellular antioxidant defense system, which culminates in various mutagen-induced deleterious effects. Overproduction of ROS causes them to behave as molecular sharks whose function is to damage molecules of the cell membrane, mitochondria, and nucleic acids, leading to oxidative stress (Aher et al., 2011). In other words, active oxygen species serve as mediators of the pollutants induced physiological responses by acting as precursors to various types of diseases, thereby affecting production (Ekweozor et al., 2002), fitness, and survivability (Cohen et al., 2017).
The accumulation of petroleum hydrocarbons in different tissues of exposed organisms has been reported (Bursian et al., 2017; Alzahrani and Rajendran, 2019). Increased stress levels, elevated detoxification efforts, and impairment of reproductive fitness were reported in birds following inhalation of pollutants (Sanderfoot and Holloway, 2017). A varied degree of biochemical and cellular responses linked to protein catabolism, bile acid metabolism, glucose homeostasis, and lipid peroxidation was observed in birds found in oil spill regions (King et al., 2014; Bianchini and Morrissey, 2018). Certain diseases such as lipid pneumonia, pulmonary dysfunction, decreases in hemocrit values, and immune-toxic effects were diagnosed in exposed birds (Szaro, 1991; Leighton, 1993; Trust et al., 1994). Al-Badri et al. (2019) found early and progressive stages of apoptosis in the hepatocytes of ducks exposed to various sources of air pollution. Pollutants substantially impacted osmoregulatory mechanisms, resulting in a decline in kidney function in birds exposed to pollutants (Amakiri et al., 2009; Dean et al., 2017). In the human population, there is a correlation between maternal exposure to air pollution and an increased risk of impaired lung development in the progeny (Saha et al., 2018). Pre- and post-hatching examinations of fertile eggs of Larus marinus (Lewis and Malecki, 1984) exposed to petroleum revealed developmental abnormalities of the embryo, morbidity, and high mortality in various avian species (Albers, 1978; Albers, 2006; Dubansky et al., 2018). Ekweozor et al. (2002) noted that hens exposed to crude oil had diminished growth, egg production, egg quality, and hatchability. Kubale et al. (2018) and Jiang et al. (2019) reported incidences of cardiovascular diseases such as atherosclerosis, right ventricular failure, and developmental cardiotoxicity in poultry.
Genes’ function in hydrocarbon toxicity
Variations in single-nucleotide polymorphisms from xenobiotic response elements (like hydrocarbon pollutants) influence gene expression (Liu et al., 2018). Lodovici and Bigagli (2011) reported on the mechanisms and pathways by which this occurs. There is evidence that these pathways are stimulated by oxidants in a number of cell types and may be involved in the activation of transcription factors (Samet et al., 2002; Øvrevik et al., 2015). The resultant physiological dysfunctions (Låg et al., 2020) are evidenced by abnormal cell differentiation and transcriptional activation of xenobiotic-activated receptors such as the aryl hydrocarbon receptor (AHR), nuclear factor erythroid-derived 2 (Nrf2), and nuclear factor (NF)-kappa B-related genes (Shukla et al., 2000; Watzky et al., 2022). A redox balance in oxidant-antioxidant synthesis is critical to cell signaling mechanisms, which are crucial for regulation of different gene expressions, adaptation to stress, and ultimately homeostasis maintenance in the body (Malireddy et al., 2012; Hedman et al., 2016; Surai et al., 2019).
AhR-mediated signaling is known for its role as a sensor for environmental stimuli (Karchner et al., 2006; Bessede et al., 2014; Zhou, 2016); it regulates the expression of many genes in response to xenobiotics. It has been proposed as a signal transducer of hydrocarbon-induced oxidative stress and inflammation (Dietrich, 2016; Jang et al., 2019), involved in the regulation of biological responses to polycyclic aromatic hydrocarbons (Ma and Baldwin, 2000; Zhou, 2016). It also regulates angiogenesis, hematopoiesis, drug and lipid metabolism, cell motility, and immune modulation (Puga et al., 2002). The cellular mechanisms of the gene showed that when a ligand binds to AhR, it moves into the nucleus, where it forms heterodimers and triggers transcription by binding to xenobiotic response enzymes like cytochrome P450 monooxygenases, aldehyde dehydrogenases 3, glutathione-S-transferases, and NADPH/quinone oxidoreductases (Gomez et al., 2018). Reitzel et al. (2014) indicated that different populations of Atlantic killfish showed strong genetic structure at the AhR-related loci studied.
Furthermore, genes that protect the cells against damage from oxidative stress (cytoprotective genes) are induced by antioxidant response elements at the transcription level mediated by Nrf2 (Jaiswal, 2004). Expression of Nrf2 occurs mostly in tissues easily affected by external stimuli like the gastrointestinal tract, lungs, and skin, as well as those that function in detoxification (Speciale et al., 2011). It plays a key role in the regulation of antioxidant biomarkers such as superoxide dismutase, glutathione peroxidases, glutathione S-transferases, and catalase (Zazueta et al., 2022). Evidence from chromatin immune-precipitation indicates that Nrf2 regulates the expression of AhR by binding to an antioxidant response element region in the AhR promoter (Shin et al., 2007). For instance, Shukla et al. (2000) reported an increase in transcriptional activation of NF-keppa B-dependent (NF-κB) gene expression, which was inhibited in the presence of catalase in murine exposed to PM2.5 at non-cytotoxic concentrations. Inhalation and absorption of pollutants in different respiratory organ epithelial cells have been observed to induce oxidative stress (Liu et al., 2018), causing an increase in the translocation of some NF-κB to the nucleus and their increased binding to DNA, leading to the expression of genes associated with NF-κB such as interleukin 6 (IL-6) and tumor necrosis factor alpha (TNF-α) (Jiang et al., 2019). A study on genes associated with fat deposition in sanderling (Calidris alba) revealed that exposure to PAH downregulated the liver basic fatty acid binding protein 1 (Lbfabp) and hepatic lipase (Lipc) expression (Bianchini et al., 2021). There is evidence of inflammatory and immune diseases associated with respiratory disorders arising from the AhR-dependent disease pathway (Neavin et al., 2018).
Antioxidants and hydrocarbon toxicity
Antioxidants are molecules that interact with reactive oxygen species to cease their oxidative reaction. They function by neutralizing free radicals (nitrogen, oxygen, and lipidic radicals) and protecting the body’s systems. Exposure to hydrocarbon pollutants induces oxidative stress, which stimulates injurious, inflammatory, adaptive, and reparative processes that overwhelm the antioxidant. The toxicity effect on the health and wellbeing of the organism arises from the level of genomic instabilities and alterations expressed as damage to biological molecules like proteins, lipids, and nucleic acids (Ren et al., 2017), resulting in oxidative stress, morbidity, mutation, and mortality. Oxidative stress is considered one of the critical pathways for the metabolism of hydrocarbon pollutant effects in the body (Tashakkor et al., 2011). The maintenance of the antioxidant status of exposed poultry is critical to halting cell damage and disrupting normal physiological processes. Antioxidants function in three ways to lessen the harm oxidative stress causes. These include enhancement of the expression of intracellular antioxidant enzymes, inhibition of the activity of ROS-generating enzymes, and direct reactions with the ROS (Lü et al., 2010; Poljšak and Fink, 2014).
The use of cellular antioxidants in prevention and protection against the metabolic dysfunctions can be achieved through enzymatic and non-enzymatic antioxidant defense systems (Bagyi et al., 2021) Consequently, dietary supplementation of exogenous antioxidants to compensate for the deficit in endogenous ones is imperative (Santos-Sánchez et al., 2019). Exogenous antioxidants rich in phenolic compounds have been proven to possess high antioxidant capacity owing to their redox properties, which enable them to adsorb and neutralize or minimize ROS, quench, and decompose peroxides (Osawa, 1994; Chen et al., 2020). Dietary antioxidants have been demonstrated to influence gene expression involving biochemical and pathological changes with respect to metabolic tissues, immune function, and disease risk factors induced by oxidative stress (Dincer and Yuksel, 2021; Dunisławska et al., 2022). The anti-oxidative effects of dietary antioxidants in a bid to compensate for the deficit in the endogenous system are achieved through various mechanisms and the interplay of signalling molecules, including the mediation of AhR, Nrf2, and CYP1A1 (Grishanova, 2022). Food polyphenolics showed AhR-based interactions at high concentrations (Amakura et al., 2008). Administration of a 6-shogaol-rich extract from ginger resulted in the induction of Nrf2 and Ho-1 regulated by mitogen-activated protein kinases (Bak et al., 2012). Numerous studies have shown potential benefits of antioxidant supplementation in the upregulation of transcription factor genes (Nrf2 antioxidant genes) and downregulation of inflammatory pathways triggered by oxidative stress (Table 2). Much as phytonutrients are capable of down- or up-modulating AhR signalling, the mechanism of their action on AhR and the Nrf2 system is not clear.
Dietary modulation of genes encoding xenobiotic metabolizing enzymes to speed up the onset of disease tolerance, like those associated with hydrocarbon toxicity, would be beneficial even in poultry. Proteomic and transcriptomic analysis identified nine candidate genes and two candidate proteins that improved antioxidant status in chickens given eucalyptus leaf polyphenol extract (Li et al., 2020). Broiler chickens that were fed nettle (Urtica dioica) had a significantly upregulated expression of some antioxidant genes (SOD1 and CAT), which obviously prevented pulmonary hypertension (Ahmadipour and Khajali, 2019). The expression of CAT and SOD genes in the heart and lung of chickens reared in cold and high-altitude environments was increased when birds were fed Securigera securidaca (Ahmadipour, 2018). Curcumin and ginger were found to induce a protective effect by upregulating the activities of antioxidative enzymes, thereby modulating oxidative stress (Lin et al., 2019; Gao et al., 2022). A reduction in inflammation from oxidative stress was seen in mice administered a polyherbal mix of ginger, Chinese date, and wood ear mushroom (Nakyam et al., 2022).
Gene-antioxidant modulation and hydrocarbon tolerance
During the selection process for economically important traits, genetic and environmental factors play a crucial role. Observed phenotypic characteristics, such as hydrocarbon tolerance in poultry, will be dependent on various mechanisms and pathways involving gene expressions pertinent to maintaining the integrity of cells in the face of pollutant-induced oxidative stress. Homeostatic misbalance due to oxidative stress during exposure to hydrocarbon pollutants may induce modifications in specific epigenetic markers. To this extent, the accessibility of genes to the cellular proteins that modulate gene transcription–how, when and where a gene is silenced or activated is required (Burton and Lillycrop, 2019).
Epigenetic changes are reversible mitotically stable modifications that do not necessarily change the DNA sequence but affect the way the sequence is transcribed (Ibeagha-Awemu and Ying, 2021; Dunislawska et al., 2022). There are therefore heritable alterations in gene expression that do not necessitate any change in the DNA sequence (Jirtle and Skinner, 2007; Guerrero-Bosagna and Skinner, 2012). Epigenetic processes influence the ability of an organism to adjust to prevailing environmental perturbance and include DNA methylation, histone acetylation, and non-coding ribonucleic acids (Ju et al., 2020; Corbett et al., 2021). These modifications are classical epigenetic mechanisms involved in packaging the chromatin structure, regulating DNA damage, and repressing gene expression, among other transcriptional activities (Bannister and Kouzarides, 2011; Hunt et al., 2013). The alterations that occur through DNA methylation inhibit gene expression by silencing the gene (Dhar et al., 2021); that due to histone acetylation can be in the form of post-transcriptional and post-transitional modifications (Wang and Ibeagha-Awemu, 2021); while the non-coding ribonucleic acid is essential for regulating cellular differentiation and organism development. Of the three mechanisms, DNA methylation causes differentiated cells to develop a more stable and long-lasting methylation pattern that regulates tissue-specific gene expression by recruiting proteins involved in gene repression or inhibition of the binding of transcription factor(s) to DNA (Moore et al., 2013; Dhar et al., 2021).
The use of dietary antioxidants has been shown to be an effective strategy involving epigenetic DNA methylation and cessation of oxidative stress. Nayak et al. (2016) emphasized the role of epigenetic mechanisms in stress regulation. Improving production, immune-competence, general health and wellbeing of poultry, through dietary intervention to assure tolerance level maintenance could involve epigenetic mechanisms. In addition, diets rich in folates, vitamins C, E, A, and D, as well as polyphenol metabolites found in phytobiotics, have been shown to suppress certain diseases via modulation of DNA methylation, histone modifications, and subsequent epigenetic regulation of gene expression (Malireddy et al., 2012). Ideraabdullah and Zeisel (2018) provide a straightforward illustration of how diet regulates genomic responses and potential physiological outcomes by establishing, recognizing, and responding to epigenetic markers. Hong and Gurjit (2012) assert that exposure to particulate matter can induce glutathione depletion in the methylation cycle, thereby promoting epigenetic changes, whereas Madrigano et al. (2012) established that PAHs form adducts inducing DNA methylation (hypo and hyper) of specific genes linked to physiological dysfunctions and contribute more to the occurrence and development of diseases (Cao et al., 2020). Hernández-Cruz et al. (2022) also reviewed cadium-induced epigenetic alterations and the use of antioxidant compounds to counteract Cd-induced epigenetic alterations.
Future perspective
Evaluation of the genetic architecture and risk assessment of various chicken strains exposed to hydrocarbon contaminants is crucial for the development of intensive, commercial, and cost-effective smallholder poultry production in hydrocarbon-polluted communities. Studies on the regulation of target genes associated with environmental stress and immune-regulatory mechanisms related to hydrocarbon pollutants can provide a better understanding of how the phenotype plasticity of chickens exposed to hydrocarbon pollution can be utilized to increase productivity. Since the primary mechanism of hydrocarbon toxicity is oxidative stress, supplementing the diets of chickens exposed to pollutants with antioxidants is crucial, and the possibility of the supplement inducing epigenetic changes that promote hydrocarbon tolerance should be investigated. Based on the premise that expression patterns regulated by epigenetic processes, particularly DNA methylation, serve as a conduit for transmitting environmental information across generations via parental germ lines, such a change could be transgenerational (Weyrich et al., 2016). These studies may contribute to the identification of molecular markers for the creation of hydrocarbon-tolerant breeds.
Data availability statement
The original contributions presented in the study are included in the article/Supplementary Material, further inquiries can be directed to the corresponding authors.
Author contributions
Conceptualization of the study was done by VO-O. VO-O, ABS, AA, and OE were involved in literature search and writing the original draft. ABS, AJS, IK, and AA edited the manuscript. Substantial and intellectual contributions were made by VO-O, ABS, IK, BF, OO, UO, and OE. VO-O made major revisions to the manuscripts and prepared it for publication. All authors contributed to the article and approved the submitted version.
Funding
This work was funded by the Tertiary Education Trust Fund National Research Fund Intervention (TETF/ES/DR&D-CE/NRF2020/SETI/04/VOL.1).
Acknowledgments
We thank the Rivers State University who through the Faculty of Agriculture and Department of Animal Science provided the space for the work. We appreciate all research staff and students who participated in this work during the mind mapping sessions and field work.
Conflict of interest
The authors declare that the research was conducted in the absence of any commercial or financial relationships that could be construed as a potential conflict of interest.
Publisher’s note
All claims expressed in this article are solely those of the authors and do not necessarily represent those of their affiliated organizations, or those of the publisher, the editors and the reviewers. Any product that may be evaluated in this article, or claim that may be made by its manufacturer, is not guaranteed or endorsed by the publisher.
References
Abbas, I., Saint-Georges, F., Billet, S., Verdin, A., Mulliez, P., Shirali, P., et al. (2009). Air pollution particulate matter (PM2.5)-induced gene expression of volatile organic compound and/or polycyclic aromatic hydrocarbon-metabolizing enzymes in an in vitro coculture lung model. Vitro 23 (1), 37–46. doi:10.1016/j.tiv.2008.09.020
Aher, V. D., Wahi, A., Pawdey, A. M., and Sonawane, A. (2011). Antioxidants as immunomodulator: An expanding research avenue. Int. J. Curr. Pharm. Res. 3, 8–10.
Ahmadipour, B. (2018). Securigera securidaca seed medicinal herb supplementation of diets improves pulmonary hypertensive response in broiler chickens reared at high altitude. J. Anim. Physiol. Anim. Nutr. 102, 1601–1607. doi:10.1111/jpn.12981
Ahmadipour, B., and Khajali, F. (2019). Expression of antioxidant genes in broiler chickens fed nettle (Urtica dioica) and its link with pulmonary hypertension. Anim. Nutr. 5, 264–269. doi:10.1016/j.aninu.2019.04.004
Al-Badri, A., Bargooth, A. F., Al-jebori, J. G., and Zegyer, E. A. K. (2019). Identification of carbon nanotube particles in liver tissue and its effects on apoptosis of birds exposed to air pollution. Veterinary World 12 (9), 1372–1377. doi:10.14202/vetworld.2019.1372-1377
Albers, P. H. (2006). Birds and polycyclic aromatic hydrocarbons. Avian Poult. Biol. Rev. 17, 125–140. doi:10.3184/147020606783438740
Albers, P. H. (1978). The effects of petroleum of different stages of incubation in bird eggs. Bull. Environ. Contam. Toxicol. 19, 624–630. doi:10.1007/BF01685849
Alzahrani, A. M., and Rajendran, P. (2019). “Petroleum hydrocarbon and living organisms,” in Hydrocarbon pollution and its effect on the environment Editors M. Ince, and O. K. Ince (London, UK: IntechOpen). doi:10.5772/intechopen.86948
Amakiri, A. O., Monsi, A., Owen, O. J., and Ngodigha, E. M. (2009). Enzyme serology of broiler chickens exposed to crude petroleum flame and fumes. Toxicol. Environ. Chem. 91, 345–348. doi:10.1080/02772240802131577
Amakura, Y., Tsutsumi, T., Sasaki, K., Nakamura, M., Yoshida, T., and Maitani, Y. (2008). Influence of food polyphenols on aryl hydrocarbon receptor-signaling pathway estimated by in vitro bioassay. Phytochemistry 69 (18), 3117–3130. doi:10.1016/j.phytochem.2007.07.022
Andreescu, N., Puiu, M., and Niculescu, M. (2018). Effects of dietary nutrients on epigenetic changes in cancer. Methods Mol. Biol. 1856, 121–139. doi:10.1007/978-1-4939-8751-1_7
Anejionu, O. C. D., Ahiarammunnah, P. N., and Nri-ezedi, C. J. (2015). Hydrocarbon pollution in the Niger Delta: Geographies of impacts and appraisal of lapses in extant legal framework. Resour. Policy 45, 65–77. doi:10.1016/j.resourpol.2015.03.012
Ansah, C. E., Abu, I. O., Kleemann, J., Mahmoud, M. I., and Thiel, M. (2022). Environmental contamination of a biodiversity hotspot—Action needed for nature conservation in the Niger Delta, Nigeria. Sustainability 14, 14256. doi:10.3390/su142114256
Aryaeian, N., Shahram, F., Mahmoudi, M., Tavakoli, H., Yousefi, B., Arablou, T., et al. (2019). The effect of ginger supplementation on some immunity and inflammation intermediate genes expression in patients with active Rheumatoid Arthritis. Gene 698, 179–185. doi:10.1016/j.gene.2019.01.048
Bagyi, J., Sripada, V., Aidone, A. M., Lin, H. Y., Ruder, E. H., and Crawford, D. R. (2021). Dietary rational targeting of redox-regulated genes. Free Radic. Biol. Med. 173, 19–28. doi:10.1016/j.freeradbiomed.2021.07.021
Bak, M. J., Ok, S., Jun, M., and Jeong, W. S. (2012). 6-shogaol-rich extract from ginger up-regulates the antioxidant defense systems in cells and mice. Molecules 17 (7), 8037–8055. doi:10.3390/molecules17078037
Bannister, A. J., and Kouzarides, T. (2011). Regulation of Chromatin by histone modifications. Cell Res. 21, 381–395. doi:10.1038/cr.2011.22
Bessede, A., Gargaro, M., Pallotta, M. T., Matino,Servillo, D. G., Brunacci, C., Bicciato, S., et al. (2014). Aryl hydrocarbon receptor control of a disease tolerance defence pathway. Nature 511 (7508), 184–190. doi:10.1038/nature13323
Bianchini, K., Crump, D., Farht, A., and Morrissey, C. A. (2021). Polycyclic aromatic hydrocarbons alter the hepatic expression of genes involved in sanderling (Calidris alba) pre-migratory fueling. Environ. Toxicol. Chem. 40 (7), 1981–1989. doi:10.1002/etc.5056
Bianchini, K., and Morrissey, C. A. (2018). Polycyclic aromatic hydrocarbon exposure impairs pre-migratory fuelling in captively-dosed sanderling (Calidris alba). Ecotoxicol. Environ. Saf. 161, 383–391. doi:10.1016/j.ecoenv.2018.05.036
Bianchini, K., and Morrissey, C. A. (2020). Species traits predict the aryl hydrocarbon receptor 1 (AHR1) subtypes responsible for dioxin sensitivity in birds. Sci. Rep. 10, 11706. doi:10.1038/s41598.020.68497-y
Bouayed, J., and Bohn, T. (2010). Exogenous antioxidants - double-edged swords in cellular redox state: Health beneficial effects at physiologic doses versus deleterious effects at high doses. Oxidative Med. Cell. Longev. 3, 228–237. doi:10.4161/oxim.3.4.12858
Bursian, S. J., Alexander, C. R., Cacela, D., Cunningham, F. L., Dean, K. M., Dorr, B. S., et al. (2017). Reprint of: Overview of avian toxicity studies for the deepwater horizon natural resource damage assessment. Ecotoxicol. Environ. Saf. 146, 4–10. doi:10.1016/j.ecoenv.2017.05.014
Burton, M. A., and Lillycrop, K. A. (2019). Nutritional modulation of the epigenome and its implication for future health. Proc. Nutr. Soc. 78 (3), 305–312. doi:10.1017/S0029665119000016
Cao, L., Zhou, Y., Tan, A., Shi, T., Zhu, C., Xiao, L., et al. (2020). Oxidative damage mediates the association between polycyclic aromatic hydrocarbon exposure and lung function. Environ. Health 19, 75. doi:10.1186/s12940-020-00621-x
Charmantier, A., and Garant, D. (2005). Environmental quality and evolutionary potential: Lessons from wild populations. Proc. R. Soc. B 272, 1415–1425. doi:10.1098/rspb.2005.3117
Chen, Y., Chen, Y., Zhang, H., and Wang, T. (2020). Pterostilbene as a protective antioxidant attenuates diquat-induced liver injury and oxidative stress in 21-day-old broiler chickens. Poult. Sci. 99 (6), 3158–3167. doi:10.1016/j.psj.2020.01.021
Chi, X., Zhang, Y., Ma, X., Lu, M., Li, Z., Xu, W., et al. (2020). Antioxidative stress of oral administration of tea extract granule in chickens. Poult. Sci. 4, 1956. doi:10.1016/j.psj.2019.11.063
Cohen, A. J., Brauer, M., Burnett, R., Anderson, H. R., Frostad, J., Estep, K., et al. (2017). Estimates and 25-year trends of the global burden of disease attributable to ambient air pollution: An analysis of data from the global burden of diseases study 2015. Lancet 389 (10082), 1907–1918. doi:10.1016/S0140-6736(17)30505-6
Corbett, R. J., Luttman, A. M., Wurtz, K. E., Siegford, J. M., Raney, N. E., Ford, L. M., et al. (2021). Weaning induces stress-dependent DNA methylation and transcriptional changes in piglet PBMCs. Front. Genet. 12, 633564. doi:10.3389/fgene.2021.633564
Croitoru, L., Miranda, J. J., Khattabi, A., and Lee, J. J. (2020). World Bank. Washington, DC: World Bank.The cost of coastal zone degradation in Nigeria: Cross river, Delta and lagos states
Das, D. N., and Ravi, N. (2022). Influences of polycyclic aromatic hydrocarbon on the epigenome toxicity and its applicability in human health risk assessment. Environ. Res. 213, 113677. doi:10.1016/j.envres.2022.113677
Dean, K. M., Cacela, D., Carney, M. W., Cunningham, F. L., Ellis, C., Gerson, A. R., et al. (2017). Testing of an oral dosing technique for double-crested cormorants, Phalacocorax auritus, laughing gulls, Leucophaeus atricilla, homing pigeons, Columba livia, and Western sandpipers, Calidris mauri, with artificially weather MC252 oil. Ecotoxicol. Environ. Saf. 146, 11–18. doi:10.1016/J.ECOENV.2017.07.003
Dhar, G. A., Saha, S., Mitra, P., and Nag Chaudhuri, R. (2021). DNA methylation and regulation of gene expression: Guardian of our health. Nucl. 64, 259–270. doi:10.1007/s13237-021-00367-y
Dincer, Y., and Yuksel, S. (2021). Antiobesity effects of phytochemicals from an epigenetic perspective. Nutrition 84, 111119. doi:10.1016/j.nut.2020.111119
Dietrich, C. (2016). Antioxidant functions of the aryl hydrocarbon receptor. Stem Cells Int. 2016, 1–10. doi:10.1155/2016/7943495
Dormans, J. A. M. A., van BreeL., , Boere, A. J. F., Marra, M., and Rombout, P. J. A. (1999). Interspecies differences in time course of pulmonary toxicity following repeated exposure to ozone. Inhal. Toxicol. 11 (4), 309–329. doi:10.1080/089583799197113
Dubansky, B., Verbeck, G., Mach, P., and Burggren, W. (2018). Methodology for exposing avian embryos to quantified levels of airborne aromatic compounds associated with crude oil spills. Environ. Toxicol. Pharmacol. 58, 163–169. doi:10.1016/j.etap.2018.01.005
Dunislawska, A., Pietrzak, E., Kadawarage, R. W., Beldowska, A., and Siwek, M. (2022). Pre-hatching and post-hatching environmental factors related to epigenetic mechanisms in poultry. J. Anim. Sci. 100, skab370. doi:10.1093/jas/skab370
Dutilleul, M., Goussen, B., Bonzom, J-M., Galas, S., and Réale, D. (2015). Pollution breaks down the genetic architecture of life history traits in Caenorhabditis elegans. PLOS ONE 10, e0116214. doi:10.1371/journal.pone.0116214
Ekweozor, I. K. E., Granville, A. W., Nkanga, E. E., and Ogbalu, O. K. (2002). The effects of crude oil contaminated feeds on the yield and quality of eggs of poultry birds (Gallus domesticus). J. Agric. Tropics Subtropics 103 (1), 89–97.
Ferrari, L., Carugno, M., and Bollati, V. (2019). Particulate matter exposure shapes DNA methylation through the lifespan. Clin. epigenetics 11, 129–214. doi:10.1186/s13148-019-0726-x
Gao, Z., Ren, Y., Liu, B., Ma, R., Li, F., Li, D., et al. (2022). Ginger constituents ameliorated B(α)P-induced toxicity via modulation of antioxidants and xenobiotic-metabolising enzymes in mice. Int. Food Res. J. 29 (2), 433–445. doi:10.47836/ifrj.29.2.20
Gomez, A., Bindesbøll, C., Satheesh, S. V., Grimaldi, G., Hutin, D., MacPherson, L., et al. (2018). Characterization of TCDD-inducible poly-ADP-ribose polymerase (TIPARP/ARTD14) catalytic activity. Biochem. J. 475 (23), 3827–3846. doi:10.1042/BCJ20180347
Grishanova, A., Perepechaeva, M. L. Y., and Perepechaeva, M. L. (2022) Aryl hydrocarbon receptor in oxidative stress as a double agent and its biological and therapeutic significance. Int. J. Mol. Sci. 123, 6719. doi:10.3390/ijms23126719
Guerrero-Bosagna, C., and Skinner, M. K. (2012). Environmentally induced epigenetic transgenerational inheritance of phenotype and disease. Mol. Cell Endocrinol. 354 (1-2), 3–8. doi:10.1016/j.mce.2011.10.004
Hennig, B., Ettinger, A. S., Jandacek, R. J., Koo, S., McClain, C., Seifried, H., et al. (2007). Using nutrition for intervention and prevention against environmental chemical toxicity and associated diseases. Environ. Health Perspect. 115 (4), 493–495. doi:10.1289/ehp.9549
Hedman, Å. K., Zilmer, M., Sundström, J., Lind, L., and Ingelsson, E. (2016). DNA methylation patterns associated with oxidative stress in an ageing population. BMC Med. Genomics 9, 72. doi:10.1186/s12920-016-0235-0
Hernández-Cruz, E. Y., Arancibia-Hernández, Y. L., Loyola-Mondragón, D. Y., and Pedraza- Chaverri, J. (2022). Oxidative stress and its role in cd-induced epigenetic modifications: Use of antioxidants as a possible preventive strategy. Oxygen 2, 177–212. doi:10.3390/oxygen2020015
Hoffman, J. B., Petriello, M. C., and Hennig, B. (2017). Impact of nutrition on pollutant toxicity: An update with new insights into epigenetic regulation. Rev. Environ. Health 32 (1-2), 65–72. doi:10.1515/reveh-2016-0041
Hong, J., and Gurjit, K. K. H. (2012). Genetic and epigenetic influence on the response to environmental particulate matter. J. Allergy Clin. Immunol. 129 (1), 33–41. doi:10.1016/j.jaci.2011.11.008
Hunt, C. R., Ramnarain, D., Horikoshi, N., Iyengar, P., Pandita, R. K., Shay, J. W., et al. (2013). Histone modifications and DNA double-strand break repair after exposure to ionizing radiations. Radiat. Res. 179, 383–392. doi:10.1667/RR3308.2
Ibeagha-Awemu, E., and Ying, Y. (2021). Consequence of egigenetic processes on animal health and productivity: Is additional level of regulation of relevance? Anim. Front. 11 (6), 7–18. doi:10.1093/af/vfab057
Ideraabdullah, F. Y., and Zeisel, S. H. (2018). Dietary modulation of the epigenome. Physiol. Rev. 98 (2), 667–695. doi:10.1152/physrev.00010.2017
Izah, S. C. (2015). Ecosystem of the Niger Delta region of Nigeria: Potentials and threats. Biodivers. Int. J. 2 (4), 338–345. doi:10.15406/bij.2018.02.00084
Jaiswal, A. K. (2004). Nrf2 signaling in coordinated activation of antioxidant gene expression. Free RadicBiol Med. 36 (10), 1199–1207. doi:10.1016/j.freeradbiomed.2004.02.074
Jang, H. S., Lee, J. E., Myung, C. H., Park, J. I., Jo, C. S., and Hwang, S. (2019). Particulate matter-induced aryl hydrocarbon receptor regulates autophagy in keratinocytes. Biomol. Ther. Seoul. 27 (6), 570–576. doi:10.4062/biomolther.2019.025
Jiang, X., Xu, F., Qiu, X., Shi, X., Pardo, M., Shang, Y., et al. (2019). Hydrophobic organic components of ambient fine particulate matter (pm2.5) associated with inflammatory cellular response. Environ. Sci. Technol. 53 (17), 10479–10486. doi:10.1021/acs.est.9b02902
Jirtle, R. L., and Skinner, M. K. (2007). Environmental epigenomics and disease susceptibility. Nat. Rev. Genet. 8, 253–262. doi:10.1038/nrg2045
Ju, Z., Jiang, Q., Wang, J., Wang, X., Yang, C., Sun, Y., et al. (2020). Genome-wide methylation and transcriptome of blood neutrophils reveal the roles of DNA methylation in affecting transcription of protein-coding genes and miRNAs in E. coli-infected mastitis cows. BMC Genomics 21, 102. doi:10.1186/s12864-020-6526-z
Karchner, S. I., Franks, D. G., Kennedy, S. W., and Hahn, M. E. (2006). The molecular basis for differential dioxin sensitivity in birds: Role of the aryl hydrocarbon receptor. Proc. Natl. Acad. Sci. U. S. A. 103, 6252–6257. doi:10.1073/pnas.0509950103
King, L. E., de Solla, S. R., Small, J. M., Sverko, E., and Quinn, J. S. (2014). Microsatellite DNA mutations in double-crested cormorants (Phalacrocorax auritus) associated with exposure to PAH-containing industrial air pollution. Environ. Sci. Technol. 48 (19), 11637–11645. doi:10.1021/es502720a
King, M. D., Elliott, J. E., and Williams, T. D. (2021). Effects of petroleum exposure on birds: A review. Sci. Total Environ. 755 (1), 142834,. doi:10.1016/j.scitotenv.2020.142834
Kponee, K. Z., Chiger, A., Kakulu, I. I., Vorhees, D., and Heiger-Bernays, W. (2015). Petroleum contaminated water and health symptoms: A cross-sectional pilot study in rural Nigerian community. Environ. Health 14 (1), 1–8.
Kubale, V., Merry, K., Miller, G., Diaz, M. R., and Rutland, C. S. (2018). “Avian cardiovascular disease characteristics, causes and genomics. In,” in Application of genetics and genomics in poultry science (London, UK: IntechOpen). doi:10.5772/intechopen.78005
Låg, M., Øvrevik, J., Refsnes, M., and Holme, J. A. (2020). Potential role of polycyclic aromatic hydrocarbons in air pollution-induced non-malignant respiratory diseases. Respir. Res. 21 (1), 299. doi:10.1186/s12931-020-01563-1
Latif, I. K., Karim, A. J., Zuki, A. B., Zamri-Saad, M., Niu, J. P., and Noordin, M. M. (2010). Pulmonary modulation of benzo[a]pyrene-induced hemato- and hepatotoxicity in broilers. Poult. Sci. 89 (7), 1379–1388. doi:10.3382/ps.2009-00622
Leighton, F. A. (1993). The toxicity of petroleum oils to birds. Environ. Rev. 1, 92–103. doi:10.1139/a93-008
Lewis, S. J., and Malecki, R. A. (1984). Effects of egg oiling on larid productivity and population dynamics. Auk 101, 584–592. doi:10.1093/auk/101.3.584
Li, M., Ghulam Nabi, G., Yanfeng Sun, Y., Wang, Y., Wang, L., Jiang, C., et al. (2021). The effect of air pollution on immunological, antioxidative and hematological parameters, and body condition of Eurasian tree sparrows. Ecotoxicol. Environ. Saf. 208, 111755. doi:10.1016/j.ecoenv.2020.111755
Li, S., Zhi, L., Liu, Y., Shen, J., Liu, L., Yao, J., et al. (2016). Effect of in ovo feeding of folic acid on the folate metabolism, immune function and epigenetic modification of immune effector molecules of broiler. Br. J. Nutr. 115 (3), 411–421. doi:10.1017/S0007114515004511
Li, W., He, Z. Q., Zhang, X. Y., Chen, Y. J., Zuo, J. J., and Cao, Y. (2020). Proteome and transcriptome analysis of the antioxidant mechanism in chicken regulated by eucalyptus leaf polyphenols extract. Oxid. Med. Cell Longev. 2020, 1384907. doi:10.1155/2020/1384907
Li, W., Zhang, X., He, Z., Chen, Y., Li, Z., Meng, T., et al. (2020). In vitro and in vivo antioxidant activity of eucalyptus leaf polyphenols extract and its effect on chicken meat quality and cecum microbiota. Food Res. Int. 136, 109302. doi:10.1016/j.foodres.2020.109302
Lin, X., Bai, D., Wei, Z., Zhang, Y., Huang, Y., Deng, H., et al. (2019). Curcumin attenuates oxidative stress in RAW264.7 cells by increasing the activity of antioxidant enzymes and activating theNrf2-Keap1 pathway. PLoS One 14, e0216711. doi:10.1371/journal.pone.0216711
Liu, D., Qin, S., Ray, B., Kalari, K. R., Wang, L., and Weinshilboum, R. M. (2018). Single nucleotide polymorphisms (SNPs) distant from xenobiotic response elements can modulate aryl hydrocarbon receptor function: SNP-dependent CYP1A1 induction. Drug Metab. Dispos. 46 (9), 1372–1381. doi:10.1124/dmd.118.082164
Llacuna, S., Gorriz, A., Riera, M., and Nadal, J. (1996). Effects of air pollution on hematological parameters in passerine birds. Arch. Environ. ContamToxicol 31 (1), 148–152. doi:10.1007/BF00203919
Lodovici, M., and Bigagli, E. (2011). Oxidative stress and air pollution exposure. J. Toxicol. 2011, 487074. doi:10.1155/2011/487074
Lü, J. M., Lin, P. H., Yao, Q., and Chen, C. (2010). Chemical and molecular mechanisms of antioxidants: Experimental approaches and model systems. J. Cell Mol. Med. 4, 840–860. doi:10.1111/j.1582-4934.2009.00897.x
Lv, Z., Fan, H., Song, B., Li, G., Liu, D., and Guo, Y. (2019). Supplementing genistein for breeder hens alters the fatty acid metabolism and growth performance of offsprings by epigenetic modification. Oxidative Med. Cell. Longev. 2019, 9214209. doi:10.1155/2019/9214209
Ma, Q., and Baldwin, K. T. (2000). 2,3,7,8-Tetrachlorodibenzo-p-doxin-induced degradation of aryl hydrocarbon receptor (AhR) by the ubiquitin-proteasome pathway: Role of the transcription activation and DNA binding of AhR. J. Biol. Chem. 275 (12), 8432–8438. doi:10.1074/jbc.275.12.8432
Madrigano, J., Baccarelli, A., Mittleman, M. A., Sparrow, D., Spiro, A., Vokonas, P. S., et al. (2012). Air pollution and DNA methylation: Interaction by psychological factors in the VA normative aging study. Am. J. Epidemiol.1 176 (3), 224–232. doi:10.1093/aje/kwr523
Malireddy, S., Kotha, S. R., Secor, J. D., Gurney, T. O., Abbott, J. L., Maulik, G., et al. (2012). Phytochemical antioxidants modulate mammalian cellular epigenome: Implications in health and disease. Antioxidants& redox Signal. 17 (2), 327–339. doi:10.1089/ars.2012.4600
Miller, J., Clark, B., Champlin, D., Nacci, D., and Whitehead, A. (2018). Genetic architecture of pollution resistance in multiple populations of killifish. Society of environmental toxicology and chemistry north America (SETAC-NA) 39th annual meeting. Sacramento: California.
Min, Y. N., Niu, Z. Y., Sun, T. T., Wang, Z. P., Jiao, P. X., Zi, B. B., et al. (2018). Vitamin E and vitamin C supplementation improves antioxidant status and immune function in oxidative-stressed breeder roosters by up-regulating expression of GSH-Px gene. Poult. Sci. 97 (4), 1238–1244. doi:10.3382/ps/pex417
Mohamed, O. I., El-Nahas, A. F., El-Sayed, Y. S., and Ashry, K. M. (2016). Ginger extract modulates Pb-induced hepatic oxidative stress and expression of antioxidant gene transcripts in rat liver. Pharm. Biol. 54 (7), 1164–1172. doi:10.3109/13880209.2015.1057651
Mondal, N. K., Saha, H., Mukherjee, B., Tyagi, N., and Ray, M. R. (2018). Inflammation, oxidative stress, and higher expression levels of Nrf2 and NQO1 proteins in the airways of women chronically exposed to biomass fuel smoke. Mol. Cell Biochem. 447, 63–76. doi:10.1007/s11010-018-3293-0
Moore, L. D., Le, T., and Fan, G. (2013). DNA methylation and its basic function. Neuropsychopharmacology 38 (1), 23–38. doi:10.1038/npp.2012.112
Mountzouris, K. C., Paraskeuas, V. V., and Fegeros, K. (2020). Priming of intestinal cytoprotective genes and antioxidant capacity by dietary phytogenic inclusion in broilers. Anim. Nutr. 6 (3), 305–312. doi:10.1016/j.aninu.2020.04.003
Nacci, D. E., Champlin, D., Coiro, L., McKinney, R., and Jayaraman, S. (2002). Predicting the occurrence of genetic adaptation to dioxin like compounds in populations of the estuarine fish (Fundulus heteroclitus). Environ. Toxicol. Chem. 21 (7), 1525–1532. doi:10.1897/1551-5028(2002)021<1525:ptooga>2.0.co;2
Nakyam, T., Wattanathorn, J., and Thukham-mee, W. (2022). Neuroprotective effect of polyherbal recipe containing ginger, Chinese date and wood ear mushroom against ischemic stroke with metabolic syndrome condition via epigenetic modification of inflammation and oxidative stress. BioMed Res. Int. 2022, 1–14. doi:10.1155/2022/8940303
Nayak, N., Bhanja, S. K., Ahmad, S. F., Mehra, M., Goel, A., Sahu, A. R., et al. (2016). Role of epigenetic modifications in improving the thermo-tolerance, growth and immuno-competence in poultry: Current status and future applications. Indian J. Poult. Sci. 51 (1), 1–9. doi:10.5958/0974-8180.2016.00015.5
Neavin, D. R., Liu, D., Ray, B., and Weinshilboum, R. M. (2018). The role of the aryl hydrocarbon receptor (AHR) in immune and inflammatory diseases. Int. J. Mol. Sci. 19 (12), 3851. doi:10.3390/ijms19123851
Oleforuh-Okoleh, V. U. (2010). Improvement in egg production traits in the light local chicken ecotype using a selection index. PhD thesis submitted to Department of Animal Science. Nsukka: University of Nigeria.
Osawa, T. (1994). Novel natural antioxidants for utilization in food and biological systems: In Postharvest biochemistry of plant food-materials in the tropics eds (Tokyo: Japan Scientific Societies Press), 241–251.
Osuagwu, E. S., and Olaifa, E. (2018). Effects of oil spills on fish production in the Niger Delta. PLoS One. 13, e0205114. doi:10.1371/journal.pone.0205114
Øvrevik, J., Refsnes, M., Låg, M., Holme, J. A., and Schwarze, P. E. (2015). Activation of proinflammatory responses in cells of the airway mucosa by particulate matter: Oxidant- and non-oxidant-mediated triggering mechanisms. Biomolecules.2 5 (3), 1399–1440. doi:10.3390/biom5031399
Oziolor, E. M., and Matson, C. W. (2015). “Evolutionary toxicology: Population adaptation in response to anthropogenic pollution,” in Extremophile fishes Editors R. Riesch, M. Tobler, and M. Plath (Cham: Springer). doi:10.1007/978-3-319-13362-1_11
Poljšak, B., and Fink, R. (2014). The protective role of antioxidants in the defence against ROS/RNS-mediated environmental pollution. Oxidative Med. Cell. Longev. 2014, 671539. doi:10.1155/2014/671539
Puga, A., Xia, Y., and Elferink, C. (2002). Role of the aryl hydrocarbon receptor in cell cycle regulation. Chemico-Biological Interact. 141 (1–2), 117–130. doi:10.1016/S0009-2797(02)00069-8
Regoli, F., and Giuliani, M. E. (2014). Oxidative pathways of chemical toxicity and oxidative Stress biomarkers in marine organisms. Environ. Res. 93, 106–117. doi:10.1016/j.marenvres.2013.07.006
Reitzel, A. M., Karchner, S. I., Franks, D. A., Evans, B. R., Nacci, D., Champlin, D., et al. (2014). Genetic variation at aryl hydrocarbon receptor (AHR) loci in populations of Atlantic killifish (Fundulus heteroclitus) inhabiting polluted and reference habitats. Evol. Biol. 14, 6. doi:10.1186/1471-2148-14-6
Ren, N., Atyah, M., Chen, W. Y., and Zhou, C. H. (2017). The various aspects of genetic and epigenetic toxicology: Testing methods and clinical applications. J. Transl. Med. 15 (1), 110. doi:10.1186/s12967-017-1218-4
Rombout, P. J. A., Dormans, J. A. M. A., van Bree, L., and Marra, M. (1991). Structural and biochemical effects in lungs of Japanese quail following a 1-week exposure to ozone. Environ. Res. 54, 39–51. doi:10.1016/s0013-9351(05)80193-8
Saha, P., Johny, E., Dangi, A., Shinde, S., Brake, S., Eapen, M. S., et al. (2018). Impact of maternal air pollution exposure on children's lung health: An Indian perspective. Toxics 6 (4), 68. doi:10.3390/toxics6040068
Sam, K., and Zabbey, N. (2018). Contaminated land and wetland remediation in Nigeria: Opportunities for sustainable livelihood creation. Sci. Total Environ. 63, 1560–1573. doi:10.1016/j.scitotenv.2018.05.266
Samet, J. M., Silbajoris, R., Huang, T., and Jaspers, I. (2002). Transcription factor activation following exposure of an intact lung preparation to metallic particulate matter. Environ. Health Perspect. 110 (10), 985–990. doi:10.1289/ehp.02110985
Sanderfoot, O. V., and Holloway, T. (2017). Air pollution impacts on avian species via inhalation exposure and associated outcomes. Environ. Res. Lett. 12 (2017), 083002. doi:10.1088/1748-9326/aa8051
Santos-Sánchez, N. F., Salas-Coronado, R., Villanueva-Cañongo, C., and Hernández-Carlos, B. (2019). Antioxidant compounds and their antioxidant mechanism. London, UK: IntechOpen.
Shin, S., Wakabayashi, N., Misra, V., Biswal, S., Lee, G. H., Agoston, E. S., et al. (2007). NRF2 modulates aryl hydrocarbon receptor signaling: Influence on adipogenesis. Mol. Cell Biol. 27 (20), 7188–7197. doi:10.1128/MCB.00915-07
Shukla, A., Timblin, C., BeruBe, K., Gordon, T., McKinney, W., Driscoll, K., et al. (2000). Inhaled particulate matter causes expression of nuclear factor (NF)-kappaB-related genes and oxidant-dependent NF-kappaB activation in vitro. Am. JRespir Cell Mol. Biol. 23 (2), 182–187. doi:10.1165/ajrcmb.23.2.4035
Speciale, A., Chirafisi, J., Saija, A., and Cimino, F. (2011). Nutritional antioxidants and adaptive cell responses: An update. Curr. Mol. Med. 11 (9), 770–789. doi:10.2174/156652411798062395
Srivastava, M., Srivastava, A., Yadav, A., and Rawat, V. (2019). Source and control of hydrocarbon pollution. Hydrocarbon Pollut. its Eff. Environ. 2019. doi:10.5772/intechopen.86487
Surai, P. F., Kochish, I. I., Fisinin, V. I., and Kidd, M. T. (2019). Antioxidant defence systems and oxidative stress in poultry biology: An Update. Antioxidants 8, 235. doi:10.3390/antiox8070235
Tashakkor, A. Y., Chow, K. S., and Carlsten, C. (2011). Modification by antioxidant supplementation of changes in human lung function associated with air pollutant exposure: A systematic review. BMC Public Health 2011 (11), 532. doi:10.1186/1471-2458-11-532
Trust, K. A., Fairbrother, A., and Hooper, M. J. (1994). Effects of 7,12-dimethylbenz[a]anthracene on immune function and mixed-function oxygenase activity in the European starling. Environ. Toxicol. Chem. 13, 821–830. doi:10.1002/etc.5620130518
Wang, M., and Ibeagha-Awemu, E. M. (2021). Impacts of epigenetic processes on the health and productivity of livestock. Front. Genet. 11, 613636. doi:10.3389/fgene.2020.613636
Watzky, M., Huard, S., Juricek, L., Dario, J., Chauvet, C., Coumoul, X. A., et al. (2022). Hexokinase 2 is a transcriptional target and a positive modulator of AHR signalling. Nucleic Acids Res. 50 (10), 5545–5564. doi:10.1093/nar/gkac360
Weyrich, A., Lenz, D., Jeschek, M., Chung, T. H., Rübensam, K., Göritz, F., et al. (2016). Paternal intergenerational epigenetic response to heat exposure in male wild Guinea pigs. Mol. Ecol. 25, 1729–1740. doi:10.1111/mec.13494
Whitehead, A., Clark, B. W., Reid, N. M., Hahn, M. E., and Nacci, D. (2017). When evolution is the solution to pollution: Key principles, and lessons from rapid repeated adaptation of killifish (Fundulus heteroclitus) populations. Evol. Appl. 10 (8), 762–783. doi:10.1111/eva.12470
Yakubu, O. H. (2017). Addressing environmental health problems in Ogoniland through implementation of United Nations environment program recommendations: Environmental management strategies. Environments 4 (2), 28. doi:10.3390/environments4020028
Zazueta, C., Jimenez-Uribe, A. P., Pedraza-Chaverri, J., and Buelna-Chontal, M. (2022). Genetic variations on redox control in cardiometabolic diseases: The role of Nrf2. Antioxidants 507. doi:10.3390/antiox11030507
Zhou, L. (2016). AHR function in lymphocytes: Emerging concepts. Trends Immunol. 37 (1), 17–31. doi:10.1016/j.it.2015.11.007
Keywords: antioxidants, epigenetic modulation, gene expression, hydrocarbon pollutants, oxidative stress, toxicity
Citation: Oleforuh-Okoleh VU, Sikiru AB, Kakulu II, Fakae BB, Obianwuna UE, Shoyombo AJ, Adeolu AI, Ollor OA and Emeka OC (2023) Improving hydrocarbon toxicity tolerance in poultry: role of genes and antioxidants. Front. Genet. 14:1060138. doi: 10.3389/fgene.2023.1060138
Received: 02 October 2022; Accepted: 23 May 2023;
Published: 14 June 2023.
Edited by:
Thiruvenkadan Aranganoor Kannan, Tamil Nadu Veterinary and Animal Sciences University, IndiaReviewed by:
Vincent Coustham, Université de Pau et des Pays de l’Adour, E2S UPPA, INRAE, NUMEA, FranceCopyright © 2023 Oleforuh-Okoleh, Sikiru, Kakulu, Fakae, Obianwuna, Shoyombo, Adeolu, Ollor and Emeka. This is an open-access article distributed under the terms of the Creative Commons Attribution License (CC BY). The use, distribution or reproduction in other forums is permitted, provided the original author(s) and the copyright owner(s) are credited and that the original publication in this journal is cited, in accordance with accepted academic practice. No use, distribution or reproduction is permitted which does not comply with these terms.
*Correspondence: Vivian U. Oleforuh-Okoleh, dml2aWFuLm9sZWZvcnVoLW9rb2xlaEB1c3QuZWR1Lm5n; Ayoola J. Shoyombo, c2hveW9tYm8uYXlvb2xhQGxtdS5lZHUubmc=
†These authors have contributed equally to this work