- 1Department of Thyroid, Breast and Vascular Surgery, Xijing Hospital, Fourth Military Medical University, Xi’an, China
- 2Department of General Surgery, Eastern Theater Air Force Hospital of PLA, Nanjing, China
- 3Department of Urology, Xijing Hospital, Fourth Military Medical University, Xi’an, China
- 4Department of Geriatrics, Union Hospital of Tongji Medical College, Huazhong University of Science and Technology, Wuhan, China
In the tumor microenvironment, tumor-infiltrating immune cells (TIICs) are a key component. Different types of TIICs play distinct roles. CD8+ T cells and natural killer (NK) cells could secrete soluble factors to hinder tumor cell growth, whereas regulatory T cells (Tregs) and myeloid-derived suppressor cells (MDSCs) release inhibitory factors to promote tumor growth and progression. In the meantime, a growing body of evidence illustrates that the balance between pro- and anti-tumor responses of TIICs is associated with the prognosis in the tumor microenvironment. Therefore, in order to boost anti-tumor response and improve the clinical outcome of tumor patients, a variety of anti-tumor strategies for targeting TIICs based on their respective functions have been developed and obtained good treatment benefits, including mainly immune checkpoint blockade (ICB), adoptive cell therapies (ACT), chimeric antigen receptor (CAR) T cells, and various monoclonal antibodies. In recent years, the tumor-specific features of immune cells are further investigated by various methods, such as using single-cell RNA sequencing (scRNA-seq), and the results indicate that these cells have diverse phenotypes in different types of tumors and emerge inconsistent therapeutic responses. Hence, we concluded the recent advances in tumor-infiltrating immune cells, including functions, prognostic values, and various immunotherapy strategies for each immune cell in different tumors.
Introduction
Immunotherapies have become increasingly important for tumor patients, particularly those with advanced tumors (Tarantino et al., 2022). It is well known that using immune checkpoint blockades (ICBs) has yielded a beneficial effect in patients with advanced melanoma and lung cancer (Mehdizadeh et al., 2021); adoptive cell therapies (ACT) and chimeric antigen receptor (CAR)-T cells therapy have also improved the prognosis of patients with hematologic tumors (Martinez and Moon, 2019). However, immunotherapy resistance occurs in some tumors, and a possible explanation for this condition is the complication of the tumor microenvironment (TME) (Whiteside, 2012). TME, which is created by various cells and soluble molecules including immune cells and cytokines, exerts significant effects on tumor development and progression (Duhan and Smyth, 2021). In TME, the crosstalk of immune cells and tumor cells significantly controls tumor growth, namely, cancer immunoediting (Burnet, 1970). Cancer immunoediting involves three phases: elimination, equilibrium, and escape (Dunn et al., 2002; Wilczyński and Nowak, 2012). In the elimination phase, various effector cells and molecules destroy tumor cells and dampen tumor progression. For instance, dendritic cells (DCs) can present tumor antigens to T cells, and subsequently, T cells release perforin and granzyme to inhibit tumor cell growth or kill tumor cells through the Fas/FasL signal pathway. However, if immune cells can not eliminate tumor cells, cancer immunoediting might proceed into the equilibrium or escape phase. In the equilibrium phase, tumor cells could not be detectable and are deemed to be in a dormant status in the clinical. However, when the balance between tumor proliferation and apoptosis is disturbed by various signaling pathways, like the Wnt/β-catenin pathway, tumor cells start to proliferate dramatically and result in tumor metastasis, namely, the escape phase (Wilczyński and Nowak, 2012). In the escape phase, the anti-tumor immune response is weakened or suppressed via multiple mechanisms which mainly disturb the cancer immunity cycle (Wilczyński and Nowak, 2012; Wada et al., 2022). The cancer immunity cycle also consists of three phases: priming, migration, and effector. In the priming phase, the process of antigen-presenting is hampered by inhibitory signaling pathways, which impairs the activation of effector cells. In the migration phase, tumor cells release inhibitory molecules to restrain immune cell infiltration. In the effector phase, these mechanisms are even more complex. Immune cells infiltrating into the tumor sites perform diverse functions, thus, they influence tumor progression in various ways. The function of these immune cells will be discussed below (Wada et al., 2022). Importantly, immune checkpoints (ICs) are essential for tumor progression in every phase. Over the past decades, attention given to ICs has increasingly grown. The ICs can be produced by various cells, including immune cells and tumor cells infiltrating the TME. They could cause the dysfunction of effector cells and inhibit the apoptosis of tumor cells (Mehdizadeh et al., 2021; Munari et al., 2021). Apart from the immune cell components, cancer-associated fibroblasts (CAFs) and tumor endothelial cells (ECs) are associated with an aberrant vascular system that can transport nutrition to tumor cells and disturb the therapeutic delivery of T cells into the tumor sites (Nagarsheth et al., 2017; Lamplugh and Fan, 2021). It is well known that high demands for nutrients in tumor cells lead to the formation of abnormal vascular networks which promote tumor growth. Due to the intense competition for nutrients between tumor cells and immune cells, the nutrients and oxygen are insufficient in TME, causing a hypoxic and acidic status. Hypoxia-inducible factor 1-alpha (HIF1α) is a key factor in upregulating the level of vascular endothelial growth factor (VEGF) that arms the aberrant vasculature and fosters the epithelial-–mesenchymal transition (EMT) in the hypoxic microenvironment (Lamplugh and Fan, 2021). Under the hypoxic condition, tumor cells could escape immunosurveillance depending on activated HIF1α signaling which promotes CTL apoptosis. Besides, in TME, tumor cells and other immunosuppressive cells could express indoleamine 2,3-dioxygenase (IDO), which depletes tryptophan and results in the impairment of CD8+T cell cytotoxicity (Lamplugh and Fan, 2021). Other substances metabolized by tumor cells, including hyper glycolysis, lactate, and lipid, can impede the antigen-presenting process of DCs, recruit regulatory T cells (Tregs), and help tumor cells eventually escape from immune surveillance (Davis et al., 2015). Additionally, soluble factors also deliver signals to control tumor development. For example, upon the high levels of tumor-derived lactate, high-expressed PD-L1 on the surface of tumor cells, or IL-4, IL-10, and TGF-β are present in TME, tumor-associated macrophages (TAMs) would polarize into the M2 phenotype, which plays a pro-tumor role (Goossens et al., 2019; Petty et al., 2019). The presence of TGF-β in TME also stimulates TAMs to produce arginase-1 (Arg-1) and inhibit T cell immune response. Hereby, since the complex TME controls the benefits of immunotherapy, a comprehensive understanding of the complex components of tumor-infiltrating immune cells is required for tumor immunotherapy. In this review, we discussed the role of tumor-infiltrating immune cells in the process of tumor elimination in TME, as well as current immunotherapeutic strategies. In addition, we described the function and predictive value of tertiary lymphoid structures in TME.
Priming phase
Tumor antigens could be recognized by DCs, which present antigens to T cells and activate T cells. This process is a pivotal step in the priming phase (Eryn and Ott, 2021). Tumor antigens include tumor-specific antigens (TSAs) and tumor-associated antigens (TAAs). Tumor antigens include mutant and viral antigens. Genomic aberrations of tumor cells result in mutant antigens, which affects antigens recognition and presentation (Lu et al., 2014). Therefore, a comprehensive understanding of the antigen-presenting cells (APCs) is extremely critical.
Dendritic cells
DC subsets are specialized in antigen recognition and presentation and induce a tumor-specific immune response in patients. DC subsets are divided into conventional dendritic cells (cDCs), plasmacytoid DCs (pDCs), and monocyte-derived DCs (moDCs), according to different functions and phenotypes (Kvedaraite and Ginhoux, 2022). Notably, cDCs include are of two types: type 1 (cDC1s) and type 2 (cDC2s). cDC1s are critical for anti-tumor response and are associated with patient survival. cDC1 infiltration apparently improved prognosis in solid tumors, such as head and neck squamous cell carcinomas (HNSCC) , lung adenocarcinoma, melanoma, and triple-negative breast cancer (TNBC) (Bogunovic et al., 2009; Roberts et al., 2016; Barry et al., 2018; Böttcher et al., 2018). cDC1s express XC-chemokine receptor 1 (XCR1), which is used to make a distinction between cDC1 and other DC subsets (Villani et al., 2017). XCR1 expressed by cDC1s could bind to the CD8+T cell phenotype XC-chemokine ligand 1 (XCL1), which activates T cell function. XCL1 is also expressed by tumor cells, which boosts this process by activating T cells (Matsuo et al., 2018; Sánchez-Paulete et al., 2018; Ferris et al., 2020). CD103+ cDCs1 can prime CD8+ T cells and CD4+T cells by cross-presenting antigen (Cancel et al., 2019). CD40 expressed by cDCs1 binds to the CD40 ligand, which is produced by CD4+T cells, which activates CD8+T cells (Schoenberger et al., 1998). cDC1s also express CXC-chemokine ligand 9(CXCL9) and CXCL10 to activate CXCR3+ T and NK cells, recruit CD8+ T cells into the tumor sites, and foster the efficacy of anti-PD-1 or anti-TIM-3 therapy (de Mingo Pulido et al., 2018; Chow et al., 2019). Moreover, after the use of PD-1 blockade, CD8+T cells release IFN-γ, which promotes cDC1 to secrete IL-12 by using the non-canonical NFκB-dependent mechanism. In turn, IL-12 augments CD8+T cell functions (Stratikos et al., 2014; Garris et al., 2018). As a side note, the primary source of the CXCL9 and CXCL10 seems to be expressed by CD103+cDC1s in TME (Mikucki et al., 2015). Additionally, CCL5 and Fms-related tyrosine kinase 3 (FLT3) produced by NK cells or CCL4 produced by tumor cells could attract cDC1s into the tumor sites (Barry et al., 2018; Böttcher et al., 2018), but the activation of the WNT/ β-catenin signaling pathway and the accumulation of prostanoidprostaglandinE-2(PGE2) in TME could deduce the production of CCL4/CCL5, respectively (Spranger et al., 2017; Böttcher and Reis e Sousa, 2018; Ruiz de Galarreta et al., 2019).
cDC2s are specialized in priming CD4+T cells through MHC-II molecules and secreting IL-12 (Mittag et al., 1950; Segura et al., 2013; Jhunjhunwala et al., 2021). When Tregs are depleted, cDC2s could potently activate CD4+T cells to kill tumor cells and are associated with a favorable prognosis in HNSCC and melanoma (Binnewies et al., 2019). However, the functions of cDC2 in TME are less clear. The function of pDCs is complicated for controlling tumor progression. pDCs may promote tumor growth, foster angiogenesis, and promote metastasis in TME by triggering Tregs and releasing inducible co-stimulator (ICOS)-L, PD-L1, and IDO (Aspord et al., 2013). Some studies have shown that higher pDC frequencies are correlated with worse outcomes (Kvedaraite and Ginhoux, 2022). Conversely, pDCs also play the anti-tumor role by producing type I interferons (IFN-Is), which enhances the cytotoxicity of T cells and NK cells, or releasing Granzyme B that kills tumor cells directly. In TME, some inhibitory factors, like TGF-β, could also impair toll-like receptor (TLR)–induced IFN-α secretion by pDCs and promote tumor growth (Kvedaraite and Ginhoux, 2022). Notably, the pDC functions in cross-priming CD8+T cells remain currently unclear and need to be further dissected (Fu et al., 2022). At this juncture, it is well documented that moDCs are the inflammation subsets and produce various inflammatory cytokines to induce tumor growth (O'Keeffe et al., 2015). On the contrary, moDCs loading tumor antigens inhibit tumor progression by cross-presenting antigens, and this property has been considered as a therapeutic agent (Ma et al., 2013). However, the function of moDCs is still thoroughly unclear in tumor settings (Duhan and Smyth, 2021; O'Keeffe et al., 2015). Lastly, several factors in the tumor microenvironment have been implicated in the evolution of DCs into a tolerogenic phenotype, including TGF-β, IL- 10, and VEGF. This tolerogenic property of DCs might help tumor cells escape from immune surveillance, limit effector T cells functions, boost the production and expansion of Tregs, and even induce DC apoptosis (Mahnke et al., 2003; Chen et al., 2017; Castenmiller et al., 2021).
Furthermore, antigen presentation can also be influenced by tumor cells. During tumor development, tumor antigens can be lost or mutated, leading to the formation of neoantigens. Even with the assistance of HSP90, neoantigens are hidden by the tumor and result in the dysfunction of DCs (Jaeger et al., 2019). A study has shown that tumor antigen loss was associated with resistance to ICB in non-small small-cell lung cancer (NSCLC) (Anagnostou et al., 2017). Expression of the HLA-I complex is reduced by genetic alterations and the modulation of transcription, failing to recognize antigens (Jhunjhunwala et al., 2021). Cytokines also affect the expression of the HLA-I complex. For instance, the inhibition of IFN-γ signaling pathways decreases the level of the HLA-I complex and leads to resistance to anti-CTLA-4 therapy in melanoma (Gao et al., 2016). However, while the deficiency is tumor-specific, how does an immune response recognize antigens? This issue requires an in-depth research (Jhunjhunwala et al., 2021).
DC-based immunotherapies: Given the properties of DCs and tumor antigens, using the cDC1-based vaccine in mice tumor can enhance infiltration of T cells and halt tumor progression (Wculek et al., 2019). It was discovered that targeting XCR1 is crucial for the delivery of tumor antigen to cDC1 and, subsequently, CD8+T cell priming (de Mingo Pulido et al., 2021). The cDC2-based vaccine may also potently inhibit tumor growth and prolong the survival (Saito et al., 2022). Treatment with antibodies against the CD47-SIRPα axis could activate cDC2s, enhancing the cytotoxicity of CD8+ T cells (Saito et al., 2022). FLT3 is a key factor for the differentiation and maturation of cDCs; thus, FLT3 agonist, CDX-301 (FLT3L), has been developed (Kvedaraite and Ginhoux, 2022). A study has reported that FLT3L boosts the efficacy of DC-targeting vaccines in melanoma (Bhardwaj et al., 2020). Besides, some studies for other tumors are under the clinical trials (NCT04491084, NCT05029999, and NCT05010200). In recent years, pDCs-based treatment has been developed and has acquired benefits. For instance, using vaccination based on pDCs could enhance CD8+ T functions and improve the prognosis of patients (Tel et al., 2013; Westdorp et al., 2019) (NCT01863108). Additionally, the TLR7/TLR8 agonists used to activate pDCs are currently in preclinical models (Zhou et al., 2022) and are under clinical trials (NCT04588324, and NCT03906526). MoDCs-based vaccines have been generated, which loads tumor (neo)antigens for presentation to T cells. MoDCs-based vaccines can overcome the “silence” of DCs caused by neoantigens to restore and enhance the presentation functions of DCs, and improve the prognosis in melanoma patients (Carreno et al., 2015). It is well documented that using autologous monocyte-derived DC vaccination could facilitate the cytotoxicity of CD8+ T cells (Baek et al., 2015) (NCT02285413). Researchers have also shown autologous DC-based vaccines in which tumor antigens are loaded could also be considered as a potential therapeutic strategy through delivering the antigen presenting cells (Yewdall et al., 2010). Another study has shown that small interfering RNA (siRNA) reduces cDC1-immunosuppressive signals to delete PD-L1 and PD-L2 from moDCs (Hobo et al., 2010). A DCs-based vaccine combined with CTLA-4 inhibitor enhanced anti-tumor response (Ribas et al., 2009). Treatment with TLR9 agonists and anti-PD-1 was also associated with a high infiltration of DCs (Ribas et al., 2018). Furthermore, nanomaterials with autophagy regulation have been developed, which is important for DC function and facilitates its anti-tumor activity (Guan et al., 2022). Engineered exosomes to activate DCs have also been proposed and are considered as a promising method to develop (Huang et al., 2022a; Fu et al., 2022). For instance, HELA-Exos play an anti-tumor role by activating cDC1 and then enhancing the function of CD8+ T in breast cancer (Huang et al., 2022a). Despite the fact that DC vaccines have acquired good efficacy in mouse models and clinical trials, they still face huge challenges as a treatment strategy, as DC vaccines could not be appropriate for a wide range of cancers.
B cells
B cells could also take up antigens and process antigens by MHC class molecules to T cells (Avalos and Ploegh, 2014; Bruno et al., 2017). Extensive infiltration of B cells promotes tumor antigens to stimulate T cells potently and is associated with longer progression-free survival (PFS) and overall survival (OS) in NSCLC (Germain et al., 2014). B cells exert an important influence which activates CD4+T cells and induces CD4+T cell differentiation into follicular helper T (Tfh) cells (Hong et al., 2018). CD40L on activated T helper cells binds to CD40 on B cells to promote proliferation and development of B cells, and B cells and Tfh cells are involved in the formation of germinal centers (GCs) (He et al., 2013; Crotty, 2019). Intratumoral B cells could differentiate into plasma cells that express CD38, CD138, and CD79a. In high-grade serious ovarian cancer, the presence of high-level CXCL-13 +B cells, T cells, and PCs signified a better survival (Kroeger et al., 2016; Montfort et al., 2017; Moran et al., 2021). Intratumoral B cells switch isotypes and produce IgG or IgA antibodies, which is contradictory in influencing tumor growth (Lauss et al., 2021). Lastly, regulatory B (Breg) cells have been proposed in TME (Saze et al., 2013). Breg cells could produce TGF-β, IL-10, and IL-35, facilitate Treg polarization and help M2 macrophages and myeloid-derived suppressor cells (MDSCs), leading to disturbing tumor antigen presentation and promoting tumor proliferation. CD39 and CD73 on the surface of Breg cells could hydrolyze ATP to adenosine and suppress the tumor death in TME (Brossart, 2022; Flores-Borja and Blair, 2022). Therefore, the role of B cells is a double-edged sword (Fridman et al., 2020).
B-based immunotherapies
Some studies have proved that the fusion of antigen peptides loading on B cells can further enhance anti-tumor immune efficacy. The CD40/CD40L pathway is also critical to adoptive cell therapies with tumor antigen peptide-loaded B cells (Evans et al., 1950; Wennhold et al., 2017). Furthermore, ACT with CD40-activated B cells loaded with RNA encoding tumor antigen or DNA encoding tumor antigen inhibited the progression of melanoma and colorectal cancer (Gerloni et al., 2004; Colluru and McNeel, 2016). B-cell receptor (BCR) on the surface of B cells can directly process antigens and activate T cells. Thus, researchers exploited this trait to edit a specific BCR toward tumor antigens in vitro. The editing BCR strategies are attractive, but they have are yet to be applied to treat tumors (Page et al., 2021). Antibodies, targeting B cells, are mainly used to treat hematological malignancy, such as anti-CD19 and anti-CD20, which results in a conducive prognosis (NCT04160195), and currently, relevant trials are on the way.
Migration phase
Activated T cells primarily eliminate tumor cells in TME. Hence, activated T cells need to migrate from blood vessels to the microenvironment with the influence of various molecules and constructions. Vascular endothelial growth factor expressed by tumor cells can promote tumor angiogenesis and inhibit the migration of activated T cells (Nagarsheth et al., 2017). Adhesion molecules, including intercellular adhesion molecule-1 (ICAM-1) and vascular cell adhesion molecule-1 (VCAM-1), could help T cells to adhere to the vessel wall and migrate into TME (Lamplugh and Fan, 2021). Recently, ectopic lymphoid aggregation has been discovered in the tumor sites, which resembles secondary lymphoid organs (SLOs), termed tertiary lymphoid structures (TLSs) (Dieu-Nosjean et al., 2008). High endothelial venules (HEVs), one of the components of TLS, can facilitate the migration of immune cells into the tumor sites and accelerate tumor cell destruction (Sautès-Fridman et al., 2019). Lymphoid tissue-inducer cells (LTi), initiating SLOs formation, may enhance the expression of adhesion molecules like VCAM1 and ICAM1, and then stimulate HEV formation of TLS by expressing LTα1β2, which could combine with LTβ. However, it is still unclear whether LTi cells drive TLS formation (Jacquelot et al., 2021a; Schumacher and Thommen, 2022).
Consequently, promoting immune cell migration into the TME could be a usable strategy to enhance anti-tumor immunity. Several studies have demonstrated that the combination of anti-PD-L1 and antiangiogenic therapy can facilitate intratumoral HEV formation and augment the efficacy of immunotherapies (Allen et al., 2017; Johansson-Percival et al., 2017). In addition, LTβR agonistic antibodies, which binds LTα1β1 to induce HEVs, have been shown to boost the efficacy of anti-VEGFR2 and anti-PD-L1 combination therapy in a recalcitrant glioblastoma model (Allen et al., 2017; Schumacher and Thommen, 2022). Targeting LIGHT directly to tumor vasculature with vascular targeting peptides (VTP) induced HEVs in various tumors, improved response to ICB, and facilitated lymphocyte infiltration (Johansson-Percival et al., 2017; He et al., 2018; He et al., 2020). Intriguingly, this study has shown the depletion of Treg cells could drive HEV formation (Colbeck et al., 2017). Therapeutic induction of HEVs with ACT immunotherapy promotes lymphocyte trafficking and enhances anti-tumor response, which is a promising strategy (Lucas and Girard, 2021).
Effector phase
Activated T cells recognize tumor cancer antigens on tumor cells by T-cell receptor (TCR) and release effector molecules to eliminate tumor cells. In TME, immune cells and tumor cells secrete and express various molecules to regulate tumor progression and metastasis. Herein, we discussed how immune cells affected tumor progression.
T cells
According to their phenotypes, T cells are primarily classified into CD8+T cells and CD4+T cells. They play significant roles in tumor immunotherapy by releasing a variety of molecules to hamper tumor growth.
CD8+ T cells
When stimulated by tumor-specific antigen, CD8 + T cells can secrete perforin and granzyme which can directly kill tumor cells, or mediate the apoptosis of tumor cells by the Fas/FasL signaling pathway (Hamann et al., 1997). After the initial antigen stimulation is removed, CD8 + T cells can generate a series of memory subsets under physiological conditions. Memory T- cells are divided into four categories: T memory stem cell-like (TSCM) (Gattinoni et al., 2017), central memory T (TCM), effector memory T (TEM), and tissue-resident memory (TRM) (Sallusto et al., 1999; Schenkel and Masopust, 2014). TSCM cells mostly localize in the lymph nodes and have the capacity for self-renewal. TCM cells can express the lymph node homing molecules such as CCR7 and CD62L. TEM cells produce integrins and chemokine receptors and traffic them into various tissues (Masopust et al., 2001; Sallusto et al., 2004). TCM cells and TEM cells could trigger immune activity in different tissues, but TRM cells provide a more advanced immune response (Yang and Kallies, 2021). In a mouse model, the finding suggested that TRM cell deficiency resulted in uncontrolled tumor growth with no change in the number of CD8 effector cells. Researchers further found that their anti-tumor capacity enhanced from 40% to more than 80% by increasing the number of TRM cells in TME (Nizard et al., 2017). Consequently, TRM cells are focused on gradually.
CD8+ TRM cells
CD8+ TRM cells were initially defined in infected tissues such as the skin, lung, and intestine (Gebhardt et al., 2009; Masopust et al., 2010; Purwar et al., 2011). Gradually, CD8+ TRM cells were found in TME and were associated with the prognosis of tumor patients (Edwards et al., 2018; Savas et al., 2018; Abdeljaoued et al., 2022; Anadon et al., 2022; Jin et al., 2022; Smith, 2022). Different phenotypes are expressed by CD8+ TRM cells to destroy tumor cells effectively. First, CD103 is a characteristic marker for CD8+ TRM cells (Okla et al., 2021). CD103+ TRM-like cells possess a cytotoxic characteristic and secrete inflammatory cytokines such as GZMB, TNF-α, IL-2, and IFN-γ (Ganesan et al., 2017). They could also combine with E-cadherin on the surface of tumor cells to retain TRM in the tissue (Zhang and Bevan, 2013; Ganesan et al., 2017; Gauthier et al., 2017; Hoffmann and Schon, 2021). The expression of CD103 is highly heterogeneous. For instance, CD103 is essential in the skin, lung, and intestine (Gebhardt et al., 2009; Ganesan et al., 2017; Dumauthioz et al., 2018), but it is dispensable for the liver (Ghilas et al., 2020). CD103+ CD8+ TRM cells were associated with improved survival in cancer patients (Edwards et al., 2018; Savas et al., 2018; Hewavisenti et al., 2020; Shen et al., 2021; Huang et al., 2022b; Jin et al., 2022). For example, CD103+ CD8+ TRM cells infiltrating into TME were associated with a better adjuvant therapeutic benefit and were considered as an ideal prognostic biomarker in muscle-invasive bladder cancer. Second, CD8+ TRM cells are anchored in the tumor lesions by CD49a (VLA-1 ), which binds to collagen in the extracellular matrix (Roberts et al., 1999; Cheuk et al., 2017). When anti-VLA-1 antibodies were applied to treat patients with tumors, the number of TRM cells declined in TME (Sandoval et al., 2013). CD49a+CD8+ TRM cells produce IFN-γ to inhibit tumor progression in a melanoma mouse model, and alleviate inflammatory diseases (Cheuk et al., 2017; Le Floc’h et al., 2007; Murray et al., 2016). Moreover, CD49a also enhances the frequency of antigen encounters (Bromley et al., 2020). Third, CD69, a C-type lectin, effectively limits CD8+ TRM cell circulation by reducing the expression of sphingosine-1 phosphate receptor-1 (S1PR1), which facilitates the migration of TRM cells (Mackay et al., 1950; Bankovich et al., 2010; Skon et al., 2013). By the way, CD69 once was presumed as a marker of TRM cells, but CD69− TRM cells have also been reported (Steinert et al., 2015). CD8+ TRM cells also express chemokines like CXCR6, which promotes cell retention in the tumor sites and unleash effector functions in ovarian cancer (Muthuswamy et al., 2021a; Muthuswamy et al., 2021b). Interestingly, the level of TGF-β in TEM is required for the expression of CD103 and CD49a on the surface of CD8+ TRM cells in the lung, skin, and intestine (Zhang and Bevan, 2013; Boutet et al., 2016; Nath et al., 2019; Qiu et al., 2021a; Barros et al., 2022). TGF-β also inhibits the expression of S1PR1 through downregulating the transcription factor Krüppel-like factor 2 (KLF2) (Skon et al., 2013). Moreover, the heterogeneity of TRM cells depends on the regulation of TGF-β signaling. These findings suggested that TGF-β signaling might impact the production of TRM cells and the cytotoxicity of CD8+T cells (Mackay et al., 2013; Christo et al., 2021; Yang and Kallies, 2021). However, it is well known that TGF-β is a typical inhibitory cytokine to suppress the anti-tumor immune response. Thus, more research into the TGF-β signal pathway is required (Qiu et al., 2021b). In addition, CD8+ TRM cells express various immune checkpoint proteins, such as CTLA-4, PD-1, and PD-L1. These molecules are linked to CD8+T cell exhaustion (Gabriely et al., 2017; Philip and Schietinger, 2022). CD39 on the surface of CD8+ TRM cells also promotes tumor growth (Guo et al., 2022).
For heterogeneity of CD8+ TRM cells, researchers hypothesized several models of its differentiation, which included a separate lineage, self-maintenance, “one cell, one fate,”, and “one cell, multiple fates”. However, a plethora of studies have manifested that phenotypes of CD8+ TRM cells were specific to different tumor types, and CD8+ TRM cells were regarded as tissue-tailored (Amsen et al., 2018; Enamorado et al., 2018; Okla et al., 2021; Konjar et al., 2022). Furthermore, phenotypes of CD8+ TRM cells are inconsistent between lung cancers and healthy lung tissues (Marceaux et al., 2021). These findings have a significant impact on immunotherapy for various tumors. We also concluded the function of different phenotypes of CD8+ TRM cells (Table1). Of note, although TRM cells play a crucial role in autoimmune diseases and viral infections, they are still in infancy in human tumors.
The CD8+ TRM-based targeted therapies
According to the known functions of TRM cells, researchers have proposed some approaches to fortify the function of TRM cells and enhance anti-tumor response. First, the treatment with PD-1 inhibitors enhanced the capacity of CD8+ TRM cells in melanoma, lung cancer, and esophageal cancer (Edwards et al., 2018; Han et al., 2020; Abdeljaoued et al., 2022). Furthermore, in the preclinical melanoma model, using the combination of CD39 inhibitor and ICB made tumor growth retardation (Sade-Feldman et al., 2018). Recently, a bispecific CD28H/PD-L1 antibody has been developed, which could increase the number of TRM cells and enhance anti-tumor immunity (Ramaswamy et al., 2022). Second, vaccines have been designed to treat tumors. In a preclinical cervical cancer model, the HPV vaccine promoted CD103 expression on the surface of TRM cells and effectively prolonged the survival (Sandoval et al., 2013; Komdeur et al., 2017). By the same token, using STxB-E7 vaccination enhanced the number of TRM cells and delayed tumor growth in HNSCC (Mondini et al., 2015). After using Polypoly-ICLC-assisted tumor lysate vaccine to treat patients with low-grade gliomas, the drugs acquired a good efficacy and the number of CD8+ TRM cells increased in TME (NCT02549833). Treatment with cervicovaginal vaccination with HPV16 E7aa4362 peptide/CPG-1826 could induce the production of CD103+ CD8+ TRM cells, and; subsequently, the number of CD8+ T cells increased, resulting in suppressing tumor progression in the genital tract (Huang et al., 2022b). Vaccines were also applied to generate TRM cells in mouse models of various infections (Zens et al., 2016; Yang and Kallies, 2021; Zheng and Wakim, 2021). Researchers also attempted to utilize ACT to hinder tumor growth (Lim and June 2017). Using the adoptive transfer of expanded CXCR6+ TRM cells has acquired the benefits in gastrointestinal cancer (Abdeljaoued et al., 2022). Reprogramming DCs to induce CD103 expression of CD8+ TRM cells has acquired obvious efficacy in a preclinical model of breast cancer (Wu et al., 2014). In a melanoma mouse model, short-term depletion of CD11c+ cells not only facilitated TRM cell trafficking but also was favorable for long-term TRM cell maintenance (Vella et al., 2021). Of note, few clinical trials have been performed to dissect TRM cell functions in different tumors (Craig et al., 2020). In a nutshell, CD8+ TRM cells potentially serve as a critical role, but some challenges remain. For instance, what are the mechanisms by which TRM cells enhance anti-tumor immunity? Are the phenotypes consistent between normal tissues and tumor cells? Which phenotypes could define TRM? How are the TRM cells maintained and replenished in TME? Therefore, these problems will trigger intense research.
CD4+ T cells
CD4+ T cells play a pivotal role in mediating adaptive immunity by various mechanisms. Over the past decades, extensive research suggested that CD4 +T cells could be mainly divided into T-helper 1 (Th1) cells, T-helper 2 (Th2) cells, T-helper 17 (Th17) cells, follicular helper T cells, and regulatory T cells. Th1 cells secrete IL-2 and IFN-γ. IL-2 promotes CD8+ T cell proliferation and activation, as well as the development of CD8+ memory cells (Kim et al., 2006; Williams et al., 2006). IFN-γ facilitates the process of antigen presentation (Dong, 2021). Th2 cells produce IL-4, IL-5, and IL-10 to exert their function. For example, after pathogens have been cleared, IL-10 inhibits innate immunity and function of Th1 cells, which could maintain host immune homeostasis (Couper et al., 1950). Th17 cells principally facilitate the death of extracellular bacteria and fungi (Luckheeram et al., 2012). Because of the complicated function of Tfh cells and Treg cells in anti-tumor immunity, thus, we mainly discussed the roles of Tfh cells and Treg cells.
Follicular helper T cells
Tfh cells, accumulated in the GCs of SLO and TLS, express a variety of phenotypes which are essential for the formation and maturation of the GCs (Asrir et al., 2017; Ribeiro et al., 2022; Schmidleithner and Feuerer, 2022) and improve the prognosis in breast cancer, colorectal cancer, and pancreatic ductal adenocarcinoma (PDAC) (Yamaguchi et al., 2020; Lin et al., 2021; Noël et al., 2021). IL-21 secreted by Tfh cells activates the STAT3 signaling pathway to induce the expression of transcription factor B cell lymphoma 6 (BCL6) and participate in the differentiation of Tfh cells (Nurieva et al., 2008; Linterman et al., 2010; Lüthje et al., 2012). IL-21 also plays a pivotal role in triggering CD8+T cell function and tumor regression in the lung adenocarcinoma model (Cui et al., 2021). BCL6 is the main transcription factor which upregulates the expression of CD28 and CXCR5, promotes the differentiation of Tfh cells through repressing Blimp1, and is important for GC to respond to tumor antigens (Nurieva et al., 2009; Yu et al., 2009; Ciucci et al., 2022). Of note, CD28 is required for the differentiation of Tfh cells. If CD28 was deficient in T cells or reduced by its inhibitor, the differentiation of Tfh cells was blocked. Tfh cells could express CTLA-4, which binds to CD80/CD86 and leads to the inhibition of CD28 (Hart and Laufer, 2022). Tfh cells are recruited into the B cell zone to form GC by expressing CXCR5, which combines with CXCL13 + B cells (Kim et al., 2001). CD40L on the surface of Tfh cells activates B cells and sustains the survival of GC B cells by binding CD40 (Vinuesa et al., 2016). Tfh cells also express ICOS. ICOS binding to its ligand ICOSL is essential for the survival of GC B cells and the maintenance of Tfh cell phenotypes by reducing the Kruppel-like factor 2 (Liu et al., 2015; Weber et al., 2015). In addition, other cytokines have different roles to affect Tfh functions. High-level IL-2 secreted by Th1 cells mediates the impairment of Tfh function through activating STAT5 signaling, whereas IL-6 secreted by DCs inversely prevents STAT5 from the combination of the IL-2rb locus (Hart and Laufer, 2022). Astoundingly, TGF-β in humans plays a protective role for Tfh cells, which activates STAT3 and STAT4 by interacting with IL-12 and IL-23, and silences genomic organizer SATB1 to aid Tfh cell differentiation (Kurata et al., 2021; Chaurio et al., 2022; Schmidleithner and Feuerer, 2022). However, it is a negative regulator in mice, and using TGF-β inhibitors reduces Tfh accumulation in the tumor sites (McCarron and Marie, 2014; Niogret et al., 2021). Although Tfh cells have been explored, it is deficient for the mechanism of Tfh differentiation and the function of GCs in various tumors.
Tfh-related immunotherapies
Recently, studies have found that the presence of Tfh cells is important for upregulating CD8-dependent anti-tumor immunity and improving the benefit of anti-PD-L1 therapy in tumors (Chen et al., 2021; Niogret et al., 2021). Immune checkpoint inhibitors also facilitated Tfh cells to activate B cells and further improved the anti-tumor response in specific breast models (Hollern et al., 2019). In addition, anti-CXCR5 CAR-T cells were applied to treat B cell Nonnon-Hodgkin’s lymphoma (B-NHLs), which eliminated B-NHL cells and lymphoma-supportive Tfh cells (Bunse et al., 2021). In a study, targeting Bcl6 – Blimp1 axis has been proposed to facilitate T cell differentiation, but the drug has not been generated (Ciucci et al., 2022). These data provide a treatment strategy for Tfh cells, but it is required to further investigation for the role of Tfh cells in human tumors.
Treg cells
Treg cells, another subset of CD4+ T cells, are responsible for immunosuppression and help tumor cells avoid immune surveillance. Tregs can be divided into three populations: naïve Tregs (FOXP3low, CD25low, and CD45RA+), eTregs (FOXP3high, CD25high, and CD45RA-), non-Tregs (FOXP3low, CD25low, and CD45RA-) based on themselves their phenotypes. The eTreg acts as a vigorous suppressor, whereas non-Tregs are immunostimulatory and secrete IFN-γ (Miyara et al., 2009). Emerging evidence indicated that eTregs resulted in a poor prognosis, but non-Tregs infiltration in colorectal cancer (CRC) was associated with a favorable outcome (Saito et al., 2016). Thus, a challenge was posed that distinguished the types of FOXP3+ Tregs in tumors (Kim et al., 2020). Further analysis found that the prognostic value of intratumoral Tregs in various tumors is inconsistent (Shan et al., 2022). In order to identify Tregs and dissect their functions, we must understand the phenotypes and cytokines expressed by Tregs. FOXP3 is a credible marker of Treg cells, and is essential for maintaining the function of Treg cells. It is reported that loss of FOXP3 expression could impair the stability of Tregs and transform Tregs into effector cells (Qu et al., 2022). It is intriguing that CD25 binding to IL-2 could activate STAT5 signaling and then induce expression of FOXP3 to inhibit CD8+ T cell response (Chinen et al., 2016). A study has also shown that CD45RA + Tregs play a suppressive role and are associated with an unfavorable prognosis in CRC (Saito et al., 2016). Cytokines secreted by Tregs, such as IL-10, IL-35, and TGF-β, are key factors in inhibiting the function of NK cells and effector T cells and promoting tumor progression (Qu et al., 2022). Increased IL-10 and IL-35 have been associated with worse outcomes in cancer patients (Zhao et al., 2015; Turnis et al., 2016). IL-35 also elicits the expression of inhibitory molecules on Teffs like TIM-3 and CTLA-4, which induces Teffs into the exhaustion status (Turnis et al., 2016; Sawant et al., 2019). IL-10 impairs CD8+ T cell function, and inhibits the expression of MHC II molecules and APCs activation (Wang et al., 2019). TGF-β is a crucial mediator for immunosuppression in the TME, which fosters the expression of FOXP3 on Tregs (Turnis et al., 2016; Colak and Ten Dijke, 2017), and induces the conversion of Th17 cells into Tregs, resulting in immune tolerance (Gagliani et al., 2015). Notably, Tregs could release GZMB and perforin to directly kill effector T cells and NK cells in TME (Cao et al., 2007). Furthermore, antigen-specific Tregs could disturb the combination of the effector T cells and cognate antigen by interacting with APC (Qu et al., 2022). Tregs also express CD39 and CD73, resulting in adenosine aggregation in TME (Allard et al., 2020). Additionally, CCR4 is the most studied receptor that can recruit Tregs into TME and promote tumor growth by binding to CCL22 or CCL17 (Gobert et al., 2009). Tregs express immune checkpoint molecules to bolster their function, such as TIM-3 and CTLA-4 (Dixon et al., 2021).
Treg-based targeted therapies
Based on these immunosuppressive mechanisms of Tregs, researchers have proposed numerous noteworthy therapeutic strategies. First, the depletion of Tregs via anti-CD25 mAb (daclizumab) and toxin conjugated anti-IL-2 (denileukin diftitox) induced tumor regression and prolonged disease-free survival (DFS) in tumors (Solomon et al., 2020; Nishikawa and Koyama, 2021). Despite the fact that anti-CD25 mAb could deplete Tregs in melanoma, it did not elicit an anti-tumor immune response (Luke et al., 2016). The anti-CD25 antibody, RG6292, designed to deplete Tregs without disturbing IL-2 signaling on effector T cells, has been applied in a mouse model (Solomon et al., 2020) and is currently being tested in human tumors (NCT04158583). Furthermore, immune checkpoint inhibitors (ICIs) like anti-CTLA-4 antibody or anti-TIGIT antibody combined with the blockade of CD25 potently resulted in the depletion of Tregs and enhanced anti-tumor responses in a mouse model (Arce Vargas et al., 2017). Near-infrared photoimmunotherapy (NIR) was also used to precisely deplete Tregs in TME (Sato et al., 2016). Second, It has been reported that using AZD8701, which targets FOXP3 on Tregs, reduces the number of FOXP3 expression in mouse models (Sinclair et al., 2019), and its clinical trial is ongoing (NCT04504669). Epigenetic modifiers have been designed to target genes that regulates FOXP3 expression on Tregs, leading to the depletion of Tregs. For instance, targeting Treg-specific demethylated region (TSDR) and histone deacetylation reduced FOXP3 expression on Tregs (Ma et al., 2018; Nagai et al., 2019). Third, CCR4 blockade may reduce the accumulation of Tregs in the tumor sites and improve therapeutic benefits in different types of cancers. Mogamulizumab, a defucosylated anti-CCR4 mAb, has been approved to treat patients with Sézary syndrome, a cutaneous T cell lymphoma. It has been tested for the clinical response in phase 1 clinical trials in various solid tumors (Shan et al., 2022). FLX475, another CCR4 inhibitor, is currently being evaluated alone or in combination with anti-PD-1 and anti-CTLA-4 for the treatment of advanced tumors (Shan et al., 2022). TNFR2-expressing Tregs play a potently immunosuppressive role in human tumors, so targeting TNFR2 has been generated such as APX601, which is tested and resulted in reducing Treg frequency and hindering Treg function in tumors (Hariyanto et al., 2022). Moreover, TGF-β receptor inhibitors have been investigated. TGF-β-R inhibitors (Galunisertib) suppress Treg function and control tumor growth (Holmgaard et al., 2018). The combination therapy of galunisertib and ICIs further reduced Treg numbers in a mouse melanoma model, and this approach is being investigated in human tumor (Ravi et al., 2018). Glycoprotein-A repetitions predominant (GARP) could facilitate the secretion of TGF-β and Treg function in preclinical models. Using the anti-GARP antibody, S1055a, could lead to the depletion of Tregs and activate effector T cells in preclinical models, and this drug is being investigated in a clinical trial (Shan et al., 2022). Besides, TGF-β-responsive CAR-T cells could prevent naïve T cells from differentiating into Tregs and promote anti-tumor immunity (Zhang et al., 1950). DC/4T1Adv-TGF-β-R fusion vaccine could inhibit tumor-derived TGF-β, which leads to the reduction of Tregs and favor anti-tumor immunity in the mouse model (Hou et al., 2018). In HPV positive cancers, a clinical trial, treatment with HPV vaccination alone or in combination with anti-PD-L1/TGF-β Trap (M7824), is underway (NCT04432597). Another clinical trial, using a TGF-β receptor ectodomain-IgG Fc fusion protein inhibitor of TGF-β in solid tumors, also is being investigated (NCT03834662). In brief, targeting T subsets is important for cancer immunotherapy. Despite enormous progress in the field, a further analysis needs to be conducted.
Tertiary lymphoid structures
Tertiary lymphoid structures which have been already mentioned are defined by an inner B-cell zone and an outer T-cell zone. B cells are indispensable for TLSs. Currently, activation of B cells in infections and autoimmune diseases has been studied, but little research has been performed in different cancers (Cogné et al., 2022). Naïve B cells could be activated through the interactions between BCR and tumor antigens, upon activated CD40 signaling (Cancro and Tomayko, 2021). In PDAC, immature B cells present in TLS only express IgD, and mature B cells express IgG and IgM (Andrew et al., 2021)). Likewise, in lung cancer, naive B cells express IgD, but mature B cells express IgD-CD38+ CD138+ (plasma cell) (Germain et al., 2014). These findings indicate B cell activation maybe undergo class-switch recombination (CSR). The activated induced deaminase (AID) expressed by B cells is required for CSR and could promote somatic hypermutation (SHM) (Dieu-Nosjean et al., 2016; Lehmann-Horn et al., 2016). Isotype class switching depends on different cytokines released by Tfh cells. For instance, upon the presence of IFN-γ in GC, IgG2a and IgG3 were expressed by B cells, but IgG2a and IgG3 were also converted to IgE mediated by IL-4 (Kinker et al., 2021). IgG and IgA antibodies secreted by plasma cells could recognize tumor antigens and control tumor cell growth. It is reported that high-level IgG antibody in vitro was correlated with a worse prognosis in breast cancer patients, but IgA antibody in vitro that reacts to tumor antigens is associated with TLS presence in TME (Garaud et al., 2018). In another study, a high-level IgG antibody is associated with a better immune response. Moreover, supernatants (SNs), including IgG and IgA antibodies, were used to evaluate the immune responses to 33 tumor antigens, and the results were different (Germain et al., 2014). Thus, the role of antibodies produced by GC B cells must be further explored. For the TLS formation, it is currently being explored, but researchers have demonstrated that the combination of 5-Aminoleuvulinic aminoleuvulinic acid-photodynamic therapy (ALA-PDT) and anti-PD-L1 mAb could promote the TLS formation and then enhance the clinical outcome in cutaneous squamous cell carcinoma (Zeng et al., 2022). Another study has also reported that TGFB1 mRNA expression was also associated with TLS formation in ccRCC (Takahara et al., 2022). However, the research has shown that tumor-associated sensory neurons are negatively correlated with mature tertiary lymphoid-like structures and HEVs (Vats et al., 2022).
Growing evidence showed that TLSs were associated with clinical outcomes of cancer patients (Schumacher and Thommen, 2022). Although TLSs were frequently correlated with a favorable prognosis in human tumors, but some studied have reported that TLSs were also linked to a negative correlation with clinical outcomes in hepatocellular carcinoma (HCC) and clear-cell renal carcinoma (ccRCC) (Finkin et al., 2015; Jacquelot et al., 2021a) or no impact on OS in melanoma and prostate cancer (Ladányi et al., 2014; García-Hernández et al., 2017). Moreover, the prognostic value of TLSs is inconsistent with the same tumor types, such as HCC and breast cancer (Liu et al., 2017; Calderaro et al., 2019). These inconsistencies might be explained by TLS heterogeneity, including TLS maturation state, location or detected phenotypes in tumors (Jacquelot et al., 2021a) (Figure 1). With respect to TLS location, the prognostic values differ from tumor types. The location and maturity of TLS contribute to the difference in HCC prognosis. Compared to TLS situated in stromal tumor, the intratumoral and peritumoral mature TLSs were associated with a favorable prognosis (Calderaro et al., 2019). Pancreatic cancer with intratumoral TLS signified a better prognostic value and exhibited a lower infiltration of immunosuppressive cells and higher infiltration of T and B cells compared to peritumoral TLS (Hiraoka et al., 2015). TLSs could also predict the prognosis of patients with tumor metastases. In melanoma and breast cancer, no representative phenotypes of TLS was observed in brain metastases (Cipponi et al., 2012; Lee et al., 2019). Besides, TLS density was related to primary tumor types in metastatic organs (Remark et al., 2013; Schweiger et al., 2016; Montfort et al., 2017; Lee et al., 2019). For example, TLS levels were found to be high in patients with lung metastases from colorectal and breast cancers. With regard to TLS maturation, TLS maturation were divided into three types: early, primary-, and secondary follicle–like TLS (Posch et al., 2018). The different degrees of maturation of TLS denoted inconsistent prognostic values in CRC, because early TLS without GCs had almost no impact on clinical outcome compared to mature TLS which signified a better outcome (Di Caro et al., 2014; Posch et al., 2018). In patients with lung squamous cell carcinoma, both early and primary TLSs did not affect patient survival, and only secondary TLSs exerted a favorable role in the prognosis (Siliņa et al., 2018). In preneoplastic hepatic lesions, immature TLSs did not effectively inhibit tumor cell growth (Meylan et al., 2020). Immature TLS without dendritic cell lysosome-associated membrane protein (DC-LAMP) exhibited a worse prognosis than existing TLS with DC-LAMP in NSCLC and ccRCC (Giraldo et al., 2015) 243). However, whether TLS is mature or not, its presence is associated with positive outcomes in oral squamous cell carcinoma (Li et al., 2020a). Remarkably, the most important factor should be the components of TLSs in various tumors. Tfh cells and B cells could express various chemokines to promote TLS formation. The presence of HEVs aids immune cells migration. These components have the potential to improve clinical outcomes. However, Tregs, the component of TLSs, play an immunosuppressive role and result in tumor growth (Martinet et al., 2012; Gu-Trantien et al., 2017; Ishigami et al., 2019). As a side note, a study has supposed that follicular Treg (Tfr) cells might be a key factor to reduce the number of CD8+T cells in adenocarcinoma (Wang et al., 2022). Moreover, it was reported that the plasma cells are crucial for the efficacy of ICB in the presence of TLS, but the molecular and cellular mechanisms for promoting plasma cells to response ICB are still unclear. Thus, the role of plasma cells in presence of TLS needs to be further explored (Teillaud and Dieu-Nosjean, 2022). TLS with high levels of M2 macrophages and CD4+THC cells (CD3+CD8−Bcl6− ) correlates with tumor progression and a higher recurrence rate in patients with CRC (Yamaguchi et al., 2020). In NSCLC, the subgroup with low-level DC-LAMP + DCs and high-level CD8+T cells reduced the likelihood of survival, suggesting the importance of DC-LAMP + DCs in TLS (Goc et al., 2014). Noteworthily, researchers have also reported that TRM could promote TLS maturation, and the number of TRM was more abundant in mature TLS in patients with lung adenocarcinoma. Furthermore, high-level TRM within TLS, especially CD103+ TRM, was associated with a better prognosis (Yang et al., 2022; Zhao et al., 2022). However, the components still need to be explored in the future. Some studies also proposed that the density of TLSs varied at different stages of the tumor. TLSs were less abundant in T3 and T4 stages compared to T1 and T2 stages of oral squamous cell carcinoma, but TLSs were more abundant in advanced stages (II-IV) than in stage I gastric cancer and high-grade breast cancer (Sautès-Fridman et al., 2019). Another study also reported that the number of TLS might be associated with the prognosis and could be considered as a target for treating patients with urachal carcinoma (Zhang et al., 2022a). Based on these conclusions, it is urgent to precisely understand the formation, components, and mechanism of TLS. Researchers have hypothesized the formation and maturation process of TLS in CRC and NSCLC, respectively, but there is a lack of evidence to support it (Meng et al., 2021). Hence, a comprehensive analysis of TLS is an area of immense interest.
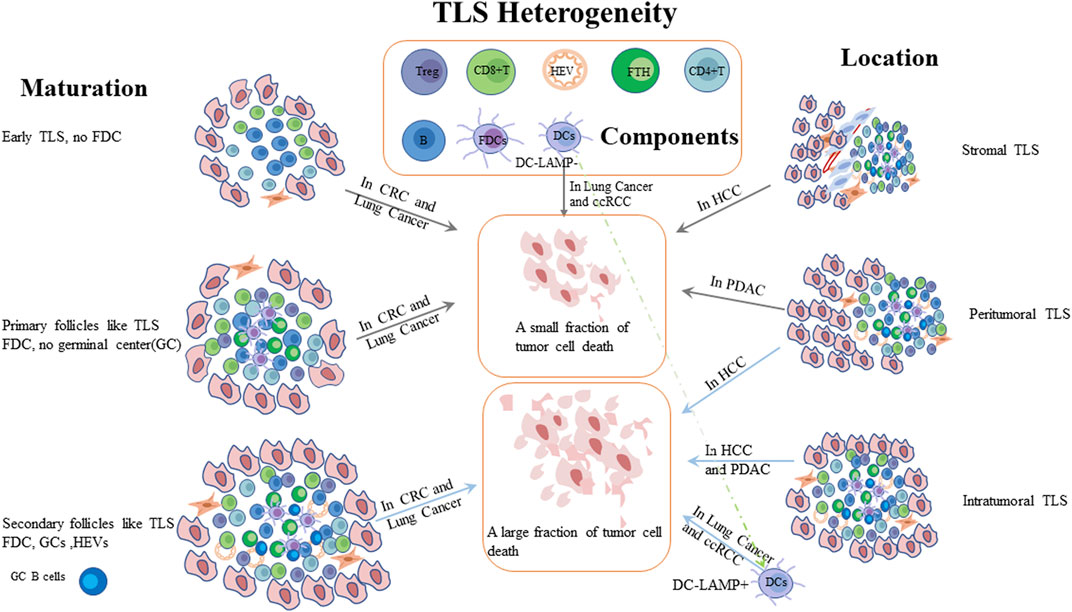
FIGURE 1. Patients with cancer have different prognosis due to TLS heterogeneity. Compared to stromal TLS, intratumoral or both intratumoral and peritumoral mature TLSs were associated with a better prognosis in different tumors. TLSs with GCs have been shown to kill tumor cells more effectively than immature TLS. PDAC: pancreatic ductal adenocarcinoma; HCC: hepatocellular carcinoma; CRC: colorectal cancer; ccRCC: clear cell renal cell carcinomas; DC-LAMP: Dendritic dendritic Cell cell Lysosomelysosome-–Associated associated Membrane membrane Proteinprotein; FDC: follicular dendritic cells; HEV: high endothelial venules.
Inducing or improving TLS function not only enhances anti-tumor responses, but also promotes the expansion of autoreactive T and B cells. First, the presence of intratumoral TLS has been regarded as a favorable marker of the responsiveness of ICB therapy in lung cancer, ccRCC, bladder cancer, urothelial carcinoma, melanoma and soft-tissue sarcoma (Groeneveld et al., 1990; Petitprez et al., 2020; van Dijk et al., 2020; Voabil et al., 2021). Accordingly, ICB increased the density of TLS or induced TLS formation in the tumor sites (Rita et al., 2020). Besides, ICB therapy combined with CXCL13 facilitated immune cell infiltration and TLS formation (Hsieh et al., 2022). Second, therapeutic vaccination also induced TLS formation in specific tumors. For instance, therapeutic vaccination targeting HPV16 and HPV18 induced TLS formation compared to non-vaccinated patients in high-grade cervical intraepithelial neoplasia (CIN2/3) (Maldonado et al., 2014). In PDAC, a specific vaccine, an irradiated, allogeneic granulocyte–macrophage colony-stimulating factor–secreting pancreatic tumor vaccine (GVAX), in combination with cyclophosphamide, was used to elicit TLS formation via suppressing the Treg pathway and activating the Th17 cell pathway. Lastly, the induction of HEV has already been elaborated (Lutz et al., 2014). To sum up, the role of TLS has been stated above. Thus, it is worthy of a comprehensive investigation of TLS, including the formation of TLS, the mechanisms of controlling tumor progression, and the interactions of TLS and immunotherapies, even the strategies for targeting TLS in TME.
Innate lymphoid cells
Innate lymphoid cells (ILCs) are an important part of the immune system to defend against tumor cells on the front line. ILCs are divided into five categories on the basis of cytokines and specific transcription factors, including natural killer (NK) cells, lymphoid tissue inducers, helper ILC1s, helper ILC2s, and helper ILC3s (Spits et al., 2013; Vivier et al., 2018). These cells, which lacks antigen-specific receptors, have different functions through secreting cytokines or activating specific signaling pathways.
Natural killer cells
NK cells have the potential to mediate anti-tumor immunity via directly or indirectly killing tumor cells. NK cells can be defined by the expression of CD16 and CD56 markers, but somatically rearranged antigen receptors like TCR is scarce (Myers and Miller, 2021; Stefania et al., 2021). Accordingly, NK cells are categorized into two subsets: CD56brightCD16- and CD56dimCD16+ NK cells. CD56bright NK cells not only release a variety of cytokines, but also interact with various molecules secreted by other immune cells (Fehniger et al., 1950; Cooper et al., 2001; Wagner et al., 2017a). CD56dimCD16+ NK cells rapidly mediate antibody-dependent cellular cytotoxicity (ADCC) through secreting granzyme and perforin (Bryceson et al., 2006; Stabile et al., 2015; Voskoboinik et al., 2015; Freud et al., 2017; Bald et al., 2019; Prager et al., 2019). With respect to the cytotoxicity of NK cells, the cytotoxicity receptors exert a powerful influence, including CD16 and the natural cytotoxicity receptor family, such as NKp30, NKp40, NKp44, and NKp46. CD16 is the strongest activating receptor and a trigger to ADCC without the assistance of other receptors (Bournazos et al., 2017). The natural cytotoxicity receptor family combined with tumor-associated ligands to remove malignant cells (Kruse et al., 2014; Barrow et al., 2019; Karagiannis and Kim, 2021). NKG2D is another important activating receptor, which recognizes MHC class I chain–related proteins sequence A (MICA) and MICB and then promotes the production of IFN-γ (Zompi et al., 2003; Raulet et al., 2013). NKG2D also interacts with transmembrane adaptor protein DAP10 to enhance the cell cytotoxicity (Sivori et al., 2021). Of note, soluble NKG2D ligands released by tumor cells have been reported to correlate with poor outcomes (Lanier, 2015; Ferrari de Andrade et al., 2018). Likewise, soluble NKp30 ligand from tumor cells promoted tumor progression and metastasis (Semeraro et al., 2015). On the surface of NK cells, inhibitory receptors also are expressed, which contains immunoreceptor tyrosine-based inhibitory motifs (ITIMs) (Myers and Miller, 2021). The inhibitory KIRs (iKIRs) recognize and bind to class I HLA molecules to hinder activating signals and impair NK cell functions (Guillerey et al., 2016; Chiossone et al., 2018). NKG2A/CD94 heterodimers combine with HLA-E molecules to impede their cytolytic activity and might assist tumor cells to evade immune surveillance. NKG2C/CD94 heterodimers, on the other hand, activate NK cells by binding to HLA-E, and their activation is dependent on NKG2A (Shifrin et al., 2014; Sivori et al., 2019; Myers and Miller, 2021). As a side note, the KIRs have both activating and inhibitory functions (Sivori et al., 2021). As mentioned previously, NK cells promote anti-tumor immunity through releasing IFN-γ, TNF-α, granzymes and perforins, but they could transdifferentiate into helper ILC1s (hILC1s ) under activated TGF-β signaling, resulting in impairing NK cell-mediated tumor control (Cortez et al., 2017; Gao et al., 2017; Cuff et al., 2019; Jacquelot et al., 2022). Besides, IL-15 signaling also triggers NK cells to convert into hILC1-like cells in head and neck cancer, but whether hILC1 cells can differentiate into NK cells is still unclear (Jacquelot et al., 2022). NK cell cytotoxicity was associated with clinical outcomes of cancer patients. Some studies have demonstrated an enhanced prognosis with tumor‐associated NK cells in CRC (Tartter et al., 1960), renal cancer (Eckl et al., 2012; Chiossone et al., 2018), melanoma (Messaoudene et al., 2016; Cursons et al., 2019), gastric cancer (Du and Wei, 2018), and HCC (Zhang et al., 2017a). However, NK cell infiltration exerts a negative influence on the prognosis in NSCLC (Platonova et al., 2011), breast cancer (Mamessier et al., 2011; Liu et al., 2021a), and renal cell carcinoma (Schleypen et al., 2003). These paradoxical observations are mainly based on the level expression of receptors or production of functional molecules.
Helper ILC1
Both hILC1s and NK cells express secrete IFN-γ, TNF-α, and transcription factor T-bet, but hILC1s do not depend on Eomes and have lower cytotoxicity (Bernink et al., 2013; Kim et al., 2021). Based on these features, NK cells and hILC1s mirror CD8+T cells and CD4+T, respectively (Gordon et al., 2012). In the context of cancer, hILC1s have both a tumoricidal function and an immunosuppressive function. When the presence of TGF-β in TME, hILC1s induced the development, growth and metastasis of tumors (Tumino et al., 2020). Although the hILC1s secrete IFN-γ to kill tumor cells (Castro et al., 2018; Verma et al., 2020), the IFN-γ can drive EMT leading to carcinogenesis (Wang et al., 2020a), and tumor cells escape (Zaidi and Merlino, 2011). When the function of hILC1s was impaired, TNF-α production decreased, resulting in a pro-tumor effect in patients with tumor (de Weerdt et al., 2016; Gao et al., 2017). Several studies have shown that the presence of hILC1s has a paradoxical prognosis in various tumors (Dadi et al., 2016; Salimi et al., 2018; Qi et al., 2021). Intriguingly, one study found that hILC1s predominantly expressed activating receptors in the early stage of CRC, but they converted to expressing inhibitory receptors in the advanced stage (Qi et al., 2021).
Helper ILC2
The hILC2s could release various cytokines and express transcription factors, including IL-4, IL-5, IL-13, IL-33 receptor, GATA3, and RORα (Entwistle et al., 2019). IL-33 is a major activator of hILC2s by binding to the IL-33 receptor. Some studies have shown that a large number of hILC2s infiltrate and exert an anti-tumor effect in IL-33 enriched the tumor sites (Kim et al., 1950; Jacquelot et al., 2021b). For instance, IL-33 activated hILC2s, which released granulocyte-–macrophage colony-stimulating factor (GM-CSF) and eosinophils were attracted to the tumor location. These activities eradicated tumor cells in melanoma (Jacquelot et al., 2021b). However, IL-33 also promotes tumor development and angiogenesis by various mechanisms (Maggi et al., 2020). For instance, IL-33 could raise the number of CD4+FOXP3+Tregs to suppress immune activity. Accordingly, hILC2s have played both pro-tumor and anti-tumor roles. The hILC2–MDSC regulatory axis has been discovered in various tumors (Chevalier et al., 2017; Trabanelli et al., 2017; Maggi et al., 2020). The hILC2s secret IL-13 to activate MDSCs which could inhibit anti-tumor immunity, and MDSCs, in turn, produce IL-13 to enhance immunosuppressive activity further (Maggi et al., 2020). Besides, the anti-tumor function of hILC2s also has been reported in HCC (Xu et al., 2021a; Heinrich et al., 2022), CRC (Ercolano et al., 2021; Huang et al., 2021; Qi et al., 2021), pancreatic cancer (Moral et al., 2020), and melanoma (Wagner et al., 2020; Peng et al., 2021a; Jacquelot et al., 2021b).
Helper ILC3
The roles of hILC3s in cancer prognosis are controversial, which expresses IL-17, IL-22, IL-23 receptor, GM-CSF, and the RORγt (Penny et al., 2018; Meininger et al., 2020). In NSCLC, hILC3s produce IL-22, and TNF-α, recruit Teff cells, and promote TLS formation to prolong the survival (Carrega et al., 2015; Goc et al., 2016). In contrast, in breast cancer, IL-22 produced by hILC3s impelled tumor proliferation and metastasis (Irshad et al., 2017). In CRC, ILC3s produced IL-22 which activated STAT3 phosphorylation signaling to promote the development and invasion of tumor (Kirchberger et al., 2013). Additionally, IL-22 is important to maintain and repair the epithelial barrier (Goc et al., 2016; Mao et al., 2018). GM‐CSF produced by hILC3s could attract macrophages in the gut and induce the generation of FOXP3+Treg cells to counteract the immune response (Mortha et al., 2014). IL-17 released by hILC3 played a role in tumorigenesis of the liver with infection of Helicobacter hepaticus and CRC (Wang and Karin, 2015; Han et al., 2019). In human squamous cervical carcinoma and breast cancer, high-level IL-17 played a pro-tumor role (Punt et al., 2015; Irshad et al., 2017). IL-12 secreted by hILC3s inhibited tumor development in melanoma (Eisenring et al., 2010; Wu et al., 2020). In breast cancer, RORγt + hILC3s could also enhance the likelihood of lymph node metastasis (Irshad et al., 2017). Recently, a new subset of ILCs, regulatory ILCs, has been reported, which releases IL-10 following TGF-β signaling to play a tumor-promoting role (Wang et al., 2017; Bald et al., 2019; Wang et al., 2020b). High levels of IL-23 in TME binding to IL-23 receptors expressed by hILC3s were associated with gut tumorigenesis (Man, 2018; An et al., 2019). LTi cells are important components to assist the formation of Peyer’'s patches and lymphoid neogenesis and inhibit tumor growth (Tumino et al., 2020).
The interactions of hILCs
The phenotypes and functions of hILC subsets changed under different microenvironments. For example, hILC2s converted to hILC1s by expressing the receptors for IL-1β, IL-12, and IL-18, and further expressed hILC1 phenotypes, such as T-bet, IFN-γ. Additionally, under the presence of IL-4, hILC2s were reversed (Bald et al., 2019; Salvo et al., 2020). Under the influence of cytokines like IL-12, IL-23, and IL-1β, hILC3s exhibited the characteristics of hILC1s as well as cytotoxic activity against tumor growth in melanoma (Nussbaum et al., 2017; Cella et al., 2019). In pulmonary squamous cell carcinomas (SqCC), hILC3s derived from hILC1s conversion suppressed anti-tumor immunity and thus shortened patient survival (Koh et al., 2019). Besides, in the presence of TGF-β, hILC2s were converted into hILC3-like cells and hILC3s were converted into ILCregs (Koh et al., 2019). However, the conversion masochisms are still not a comprehensive explanation and are necessary to be explored.
NK-related therapies
With the advent of cancer immunotherapy, targeting innate lymphoid cells has been reported. First, targeting inhibitory and activated NK cell receptors have been developed. Anti-KIR2D antibody (Ab) (Lirilumab; IPH2102) or combined with ICBs has been used to treat patients with hematological malignancies (Benson et al., 2012; Benson et al., 2015; Yalniz et al., 2018). An anti-NKG2A mAb (omalizumab; IPH2201) has been applied in chronic lymphocytic leukemia (André et al., 2018; Kamiya et al., 2019) and could unleash the cytotoxicity of NK by combining with anti-PD-L1 mAb (André et al., 2018). Besides, anti-NKG2A mAb is being evaluated in a clinical trial by combining an anti-EGFR Ab (cetuximab) in advanced solid cancers (NCT02643550). However, a study has shown that NKG2A blockade could promote CD8+T cell functions, but were ineffective for NK cells in mouse tumor model with HPV16 induction (van Montfoort et al., 2018). Additionally, CAR-NK cells have been engineered to have a chimeric receptor (NKG2D), which improves their cytotoxic capacity against tumor cells (Chang et al., 2013; Parihar et al., 2019). Second, a novel approach, using pluripotent stem cells (iPSC) to elicit NK cells, has been designed. Treatment with iPSC-derived NK cells or combined with anti-PD-1 Ab made cancer cell growth arrest (Li et al., 2018; Cichocki et al., 2020). Third, CAR-NK cell-based therapeutic regimens are considered as a promising therapeutic method, and increasing evidence has been shown in the preclinical models. The therapeutic strategy using CAR-NK cells has been proven to improve the anti-tumor efficacy in preclinical models of CRC and acute myeloid leukemia (AML) (Hayes, 2021). HER-2-specific CAR-NK cells were injected into ovarian cancer mice also ameliorated NK cytotoxicity (Han et al., 2015). CXCR1-modified NK cells enhanced anti-tumor activity in ovarian cancer mice with peritoneal xenografts (Ng et al., 2020). CAR-NK cells with targeting EGFR increased anti-tumor efficacy in a mouse model of glioblastoma (Han et al., 2015). CAR-NK cells with targeting CD19 can the cytotoxic activity of NK cells in acute lymphoblastic leukemia (ALL) (Quintarelli et al., 2020). Noteworthily, Cytomegalovirus (CMV), the most potent stimulator of NK cells, has been adopted to treat pediatric ALL and it could prolong the survival (Sivori et al., 2021). DAP10, when added to the CAR-NK cells, has been reported to enhance NK cell cytotoxicity potently through facilitating and maintaining the expression of NKG2D (Morvan and Lanier, 2016). Additionally, it is intriguing that cytokines also are considered to add to the frame of CAR-NK cells. For example, IL-15 incorporated into the CAR construct enhanced NK cell cytotoxicity and eliminated tumor cells (Daher and Rezvani, 2021). Although CAR-NK cells have been designed to combat tumor cells, there are few relevant studies. In recent years, in order to find out beneficial approaches, researchers have registered relevant clinical trials (NCT03415100, NCT03940820, NCT03692637, NCT02839954, NCT03383978, and NCT03941457). Of note, two clinical trials have been withdrawn and suspended, respectively (NCT03579927, NCT01974479).
Moreover, because cytokines are important for ILCs, cytokine-based therapy could affect the functions of ILCs. Pre-activated NK cells ex vivo by several cytokines, primarily including IL-12, IL-15, and IL-18, could be endowed with memory-like features, termed cytokine-induced memory-like NK cells (CIML-NK), and then last to exert an anti-tumor function (Romee et al., 2016). At present, this strategy has been investigated for hematological malignancies (NCT01898793, NCT03068819, and NCT02782546). TGF-β is a potently immunosuppressive factor. A study has been conducted that deleting TGFβR2 from NK cells using CRISPR-Cas9 technology could suppress the function of TGF-β and maintain their cytotoxicity in AML. Therefore, NK cells have been engineered to express a non-functional TGFβR2-like receptor in order to inhibit the function of TGF-β (Daher and Rezvani, 2021). High doses of IL-2 have been applied to the clinical practice to treat a small part of patients with advanced tumors (Marabondo and Kaufman, 2017), but IL-2 could increase the number of Tregs (Ghiringhelli et al., 2005; Adotevi et al., 2018). Furthermore, researchers found utilizing IL-15 did not result in Tregs expansion in patients with neuroblastoma (Nguyen et al., 2019). Consequently, IL-15 which increases the number and function of NK cells, is considered as a therapeutic strategy. Therapy with IL‐15 superagonist, ALT-803, has been reported to boost anti-tumor activity of NK and T cells and prolong patient survival (Hosseini et al., 2020; Sivori et al., 2021). It is surprising that ALT-803 can attach to other molecular structures in order to generate a pleiotropic compound and obtain benefits (Sivori et al., 2021). Recently, treatment with IL-15 has been investigated and the results of several clinical trials have been published (NCT01572493, NCT03759184, NCT03905135, NCT04185220, and NCT02689453). Treatment with the combination of human IL-15 (rhIL-15) and monoclonal antibody, including alemtuzumab, obinutuzumab, avelumab, or mogamulizumab, has been reported to boost the cytotoxicity of NK cells and enhance the efficacy of these monoclonal antibodies in small population patients with advanced chronic lymphocytic leukemia (Dubois et al., 2021). However, these studies have found that systemic IL-15 (N-803) impacted the presence of infused NK cells in AML, although it improved the function of CD8+ T cells (Berrien-Elliott et al., 2022; Pende and Meazza, 2022). Therefore, N-803 is still being investigated in the clinical trials (NCT03050216 and NCT01898793). Another cytokine, IL-12, is of a similar anti-tumor function to IL-15. The injection of membrane-bound interleukin 21 (mbIL-21) after haploidentical HSCT of patients with leukemia reduced the risk of relapse (Ciurea et al., 2017). Additionally, the combination therapy of IL-15 and IL-21 was used in rhabdomyosarcoma to enhance anti-tumor response (Wagner et al., 2017b).
Lastly, novel polyfunctional antibodies, termed natural killer cell engagers (NKCEs), have been generated. NKCEs have been proposed to generate a more effective benefit against tumor cells (Davis et al., 2015). The CD16 x CD33 NK cell engager was the first bispecific killer engager (BiKE) which are used to treat patients with AML (Wiernik et al., 2013). Furthermore, new tri-specific killer cell engagers (TriKE) have been designed. Anti-CD16 x IL-15 x anti-CD33 TriKE played an anti-tumor role through eliciting NK cell functions in mouse models of tumors (Vallera et al., 2016; Vallera et al., 2020), and its efficacy was reported in a terminated clinical trial (NCT03214666). Anti-CD16 x anti- CD19 x IL-15 TriKE promoted NK cells to perform tumoricidal functions in chronic lymphoid leukemia (Felices et al., 2019). Similarly, another tri-specific NK cell (1615133TriKE) also could eliminate tumor cells by the mechanism of ADCC (JU et al., 2017). Besides, human EGFR3 x NKp30 NK cell engagers have been developed, which is modified based on the affinity of B7-H6. They induced NK cells to secret cytokines and eliminate tumor cells (Demaria et al., 2021). NKp46-NKCEs fused with a tumor antigen and an Fc fragment could kill tumor cells by the mechanism of ADCC (Demaria et al., 2021). Intriguingly, adaptive NK cells with potent ADCC capacity were able to not only ablate the immunosuppressive response of MDSCs and Tregs, but also amplify the efficacy of BiKE and TriKE (Sivori et al., 2021). The various NKCE strategies are promising therapeutic tactics and are necessary to be further explored.
The hILC-related therapies
At present, harnessing helper ILCs is relatively rare and mainly targets cytokines that influence the cytotoxicity of these cells. Treatment with IL-33 alone or the combination of IL-33 with PD-1 blockade boosted the cytotoxicity of hILC2s and anti-tumor activity in a mouse model of melanoma (Maggi et al., 2020; Jacquelot et al., 2021b). However, IL-33 may stimulate hILC2s to produce the immunosuppressive ectoenzyme CD73, thereby promoting tumor growth (Maggi et al., 2020). Targeting the hILC2–MDSC axis should be promising in APL. IL-13 is a key molecule in this axis. Targeting the IL-13 receptor on tumor cells has shown a good efficacy in glioma mouse model. Anti-IL-13R mAb was also used to treat patients with glioblastoma (Maggi et al., 2020). In a completed clinical trial, treatment with IL-4 PE38KDEL cytotoxin in patients with relapsed gliomas has shown to have a good prognosis (NCT00014677). The high levels of IL-4R also promote tumor growth, so targeting IL-4R has been designed. It is well known that using anti-IL-4R antibodies had a significant impact on a variety of tumors (Yang et al., 2012a; Seto et al., 2014). In patients with AML treated by allogeneic HSCT, IL‐22 secreted by ILC3s might forestall graft versus host disease (GVHD), and thus IL‐22 could be a feasible treatment option (Munneke et al., 2014). The function and plasticity of helper ILCs are important for tumor therapy. Thus, increasing research into helper ILCs should be conducted in the future.
Other tumor-infiltrating immune cells
Myeloid-derived suppressor cells
Myeloid-derived suppressor cells have been reported as inhibitors of anti-tumor immunity by antigen-specific and non-specific patterns (Serafini et al., 2006). Some researchers have shown that MDSCs are negatively associated with the prognosis of tumor patients (Serafini et al., 2006; Tian et al., 2019). MDSCs can be devided into two subtypes, monocytic MDSCs (M-MDSCs) and polymorphonuclear MDSCs (PMN-MDSCs). M-MDSCs could promote the maturation of DCs, differentiate into M2-TAM, produce nitric oxide (NO), Arg-1 which could deplete arginine, and secrete inhibitory cytokines including IL-10 and TGF-β (Wilczyński and Nowak, 2012; Tie et al., 2022a). PMN-MDSCs mainly induce antigen-specific T-cell tolerance and hinder T-cell migration by producing reactive oxygen species (ROS) (Gabrilovich, 2017; Li et al., 2020b). PMN-MDSCs also secrete some cytokines to facilitate angiogenesis in TME (12). Moreover, tumor cells could secrete various molecules to attract MDSCs into TME like GM-CSF and IL-6, in turn, MDSCs induce the mutations of tumor cells and express some proteins like SA100A8/9 to avoid immune surveillance (Sinha et al., 1950a; Bresnick et al., 2015; Li et al., 2020b). IL-6 also promotes MDSC accumulation and inhibits anti-tumor immunity by activating the JAK/STAT3 signaling pathway, which results in increased production of ROS, NO, and PD-L1 (Ostrand-Rosenberg and Fenselau, 1950; Weber et al., 2021). Other molecules such as CCR2 or CCR5 are important for the migration of MDSCs. MDSCs also induce the production of Tregs and Th17 cells (Sinha et al., 1950b; Messmer et al., 2015).
MDSC-based therapies
According to MDSC functions, targeting MDSCs has been developed. First, depletion of MDSCs has been carried out in mouse models. It is reported that tyrosine kinase inhibitors (TKIs) could deplete MDSCs. For instance, Sunitinib was used to eliminate MDSCs by interfering with VEGF and STAT3 signaling pathways in renal cell carcinoma (Peng et al., 2021b). Using ibrutinib could restrain the production MDSCs in melanoma (Stiff et al., 2016). Another novel therapeutic strategy has been proposed. Targeting S100A family proteins, “peptibodies” adjoined to antibody Fc fragments could selectively eliminate MDSCs (Qin et al., 2014). The TNF-related apoptosis-induced ligand (TRAIL) receptors are also considered as a target for the depletion of MDSCs (Condamine et al., 2014; Dominguez et al., 2017; Hartwig et al., 2017). Targeting TRAIL-R2, DS-8273a, is ongoing in advanced solid tumors and lymphoma (NCT02076451). Second, it is a practical strategy that MDSCs are blocked to migrate to the tumor sites. Targeting chemokines or its receptors which help MDSC migration could inhibit MDSC recruitment and trafficking. Targeting CCR5 secreted by MDSCs has been reported to reduce the number of tumor-infiltrating MDSCs, and improve the survival in melanoma and breast cancer (Velasco-Velázquez et al., 2012; Zhang et al., 2013; Blattner et al., 2018). Targeting CXCL13 or its receptor CXCR5 could decrease the accumulation of MDSCs in TME in preclinical models (Ding et al., 2015; Garg et al., 2017). CXCR2 antagonists have been reported to make MDSCs range from overt infiltration to subtle infiltration and T-cell infiltration increase in the tumor sites. Targeting CXCR2 also augments the therapeutic efficacy of PD-1 blockade (Highfill et al., 2014; Zhang et al., 2020). Additionally, CXCR2 antagonists (Reparixin and AZD5069) are currently in the clinical trial phase for locally advanced or metastatic breast cancer metastatic (NCT05212701) and castration-resistant prostate cancer (NCT03177187), respectively. Targeting CCL2–CCR2 axis has shown a good outcome in mouse models (Li et al., 2017; Xu et al., 2021b), but anti-CCL2 mAbs or CCR2 antagonists are mainly used to treat immune diseases in the clinical trials. Only a CCR2/CCR5 Dual Antagonist (BMS-813160) is being used to try to treat patients with locally advanced PDAC, which is in the clinical trial phase (NCT03767582). Targeting colony-stimulating factor 1 receptor (CSF1R) or its ligand CSF-1 could prevent myeloid cell differentiation into MDSC and impede tumor progression (Cannarile et al., 2017; Yeung et al., 2021). Other studies have demonstrated that CSF-1R blockades combined with CXCR2 antagonists, ICB or anti-VEGFR mAbs have better efficacy in tumor patients (Tie et al., 2022b). Third, the downregulation of immunosuppressive functions of MDSCs is a promising approach. The inhibition of COX-2/ PGE2 signaling could suppress MDSC functions, which leads to impairing the production of Arg-1 and ROS, improves CD8+ T cytotoxicity, and delays tumor growth (Eruslanov et al., 2010; Obermajer et al., 2012; Zelenay et al., 2015). In preclinical models of glioma, targeting COX2 combined with acetylsalicylic acid downregulated the levels of PGE2 and inhibited glioma progression (Fujita et al., 2011). Phosphodiesterase-5 (PDE-5) inhibitors could also impair the level of Arg-1 produced by MDSCs. Using PDE-5 inhibitors has been shown to boost the anti-tumor immune activity of T cells and NK cells, reduce the accumulation of MDSCs and Tregs, and promote cancer cell growth arrest in patients with HNSCC and metastatic melanoma (Weed et al., 2015; Hassel et al., 2017). The inhibition of the STAT3 pathway is another therapeutic strategy to impair the function of MDSCs. The STAT3 inhibitor, AZD9150, combined with ICB, has been utilized to treat patients with diffuse large B‐cell lymphoma in a clinical trial (NCT01563302). In localized and metastatic castration-resistant prostate cancer patients, treatment with TLR9-targeted STAT3 siRNA delivery to abrogate the immunosuppressive function of MDSCs diminished the enzymatic activity of Arg-1, inhibited STAT3 target gene and T cell function (Hossain et al., 2015). IL-6 inhibitors also impacted the STAT3 signaling by reducing STAT3 phosphorylation and the expression of STAT3 downstream anti-apoptotic genes in ovarian cancer (Guo et al., 2010a; Guo et al., 2010b). Lastly, another credible strategy is to reduce the production of MDSC populations. All-trans-retinoic acid (ATRA) binding to the retinoid receptor could induce the immature myeloid cell (IMC) population to differentiate into macrophages and dendritic cells, neutralize high ROS production and increase glutathione synthase (Liu et al., 2021b; Bi et al., 2022). ATRA administration or combined with other immunotherapies increased the number of T cells, enhanced dendritic cell functions, and downregulated the ROS generation in MDSCs, resulting in improving anti-tumor immunity (Li et al., 2014; Bauer et al., 2018; Tobin et al., 2018). The combination of ATRA and ipilimumab are more effective than using ipilimumab monotherapy alone in metastatic melanoma and cervical cancer patients (Tobin et al., 2018; Liang et al., 2022). ATRA administration is an extremely promising therapeutic option for restraining the immunosuppressive functions of MDSCs, thus, its application needs to be further explored in other tumors. Additionally, some studies have reported that histone deacetylase inhibitors (HDACs) also control the differentiation of MDSCs and inhibit MDSC functions in tumor mouse models (Orillion et al., 2017; Briere et al., 2018; Christmas et al., 2018). The low-dose HDACi trichostatin-A could impair the suppressive activity of MDSCs and prevent MDSCs from trafficking, but the off-target effects that is the upregulation of PD-L1 should be tackled in further research (Li et al., 2021; Adeshakin et al., 2022). Other approaches to impact the differentiation and function of MDSCs, such as promoting the expression of interferon regulatory factor (IRF)-8, and inhibiting casein kinase 2 (CK2) signaling, are promising strategies (Valanparambil et al., 2017; Hashimoto et al., 2018; De Cicco et al., 2020; Xia et al., 2020).
In summary, targeting MDSCs have been developed and acquired good outcomes in preclinical models. However, due to the MDSC heterogeneity in various tumor types, the drugs targeting MDSCs could not be applied broadly. Thus, further studies are indispensable in the different tumor types. Secondly, the plasticity of MDSCs has been mentioned previously. Thus, the factors which reshape the differentiation of MDSCs are essential for future treatment strategies. In addition, even if the depletion of MDSC and the inhibition of MDSC trafficking are favorable options, the complicated mechanisms to reduce the number of MDSCs have not been revealed, so complementary researches are cardinal to develop new options and improve the prognosis.
Tumor-associated macrophages
Macrophages have been traditionally divided into two types: inflammatory M1-macrophages (anti-tumoral phenotypes) and immunosuppressive M2-macrophages (pro-tumoral phenotypes) (Cassetta and Pollard, 2020; Christofides et al., 2022). Macrophages are recruited into the tumor sites and play different functions, termed tumor-associated macrophages. CSF1 is a key factor for the recruitment of macrophages and polarizes macrophages to express the M2 phenotype (De et al., 2016; Christofides et al., 2022). It is reported that the inhibition of CSF-1 could decrease the accumulation of TAM and transform the M2 phenotype into the M1 phenotype (Ramesh et al., 2019). CCL2 also attracts macrophages into the tumor sites and mediates macrophage polarization (Korbecki et al., 2020; Yang et al., 2020). TAMs have a dual function, pro-tumoral and anti-tumoral functions. TAMs promote tumor progression by following pathways. First, TAMs release various molecules to assist tumor cell proliferation and metastasis. Growth factors expressed by TAMs such as epidermal growth factor (EGF) aid tumor cell proliferation. NF-kB-mediated factors like IL-6 and CCL2 prevent tumor cell apoptosis (Xiang et al., 2021). TAMs also induce and activate the Wnt/β-catenin signaling, resulting in the proliferation of tumor progenitor cells in liver cancer (He and Tang, 2020). TAMs secrete CCL5 to activate the STAT3β-catenin pathway and favor the metastasis of tumor cells (Huang et al., 2020). The proangiogenic growth factors released by TAMs like VEGF, platelet-derived growth factor (PDGF) and fibroblast growth factor (FGF) also facilitate tumor cell migration (Kumari and Choi, 2022). Second, TAMs play an immunosuppressive role by expressing various small molecules. TMAs facilitate the ICs expression on tumor cells or produce IL-10, TGF-β, Arg-1, and IDO to impede T cell function (Arlauckas et al., 2018; Xiang et al., 2021; Kumari and Choi, 2022). Finally, TAMs also upregulate the level of inhibitory receptors to inhibit T cell and NKcell activity and recruit Tregs into TME (Yang et al., 2012b; Wu et al., 2019; Tie et al., 2022a). Conversely, TAMs could inhibit tumor progression by increasing their phagocytic capacity and enhancing the function of antigen presentation. TAMs also produce cytokines to activate Th1 and CD8+T cells and improve anti-tumor immunity (Moeini and Niedźwiedzka-Rystwej, 2021). Of note, the polarization of macrophages could be reshaped in the complex TME. For instance, activated mTOR signaling pathways facilitated the polarization of M2 macrophages (Mazzone et al., 2018; Chen et al., 2022).
The TAMs-based immunotherapies
Targeting TAMs mainly inhibits its pro-tumoral functions, and several studies have been conducted. First, depleting TAMs and arresting the recruitment of TAMs have been reported. Targeting the CSF-1/CSF-1R axis has been tested in preclinical models and is a significant therapeutic option to decrease the production and aggregation of TAMs. Inhibiting the CCL2/CCR2 axis is another reasonable treatment strategy for preventing TAMs from migrating into tumor sites. The efficacy of CSF-1 and CCL2 inhibitors has been comprehensively reviewed, therefore, we will not be covered here (reviewed in refs (Rasmussen and Etzerodt, 2021)). Second, inhibition of the immunosuppressive function of TAMs could boost anti-tumor immunity by polarizing M2 TAMs into anti-tumor phenotypes. The TLR7/8 influences the TAM polarization to skew toward M1 TAM, thus, its agonist, R848-Ad, has been used to treat tumors in the mouse models and then improves the anti-tumor activity (Rodell et al., 2018; Rodell et al., 2019). Upon a TLR agonist, targeted delivery of a long peptide antigen to TAMs via using a nano-sized hydrogel (nanogel) activated TAMs to promote tumor apoptosis and activate anti-tumor immune responses, including antigen-presenting activity and altering tumor immune responses from resistance to responsiveness (Muraoka et al., 2019; Tie et al., 2022a; Zhang et al., 2022b). Activated TLR3 ligands also shift the M2 phenotype to the M1 phenotype by upregulating the expression of MHC-II molecule (Vidyarthi et al., 2018). The PI3K/AKT pathway is responsible for the recruitment of M2-TAM, thus, PI3Kα inhibitors could impede tumor cell growth and invasion (Khan et al., 2013). PI3Kγ upregulates the immunosuppressive properties and downregulates the anti-tumor properties of TAMs (Vergadi et al., 1950; Zhang et al., 2017b; Kaneda et al., 2017), thus, targeting PI3Kγ would be necessary. Activation of the CD40 receptor on TAMs could convert TAMs into M1 macrophages (Wiehagen et al., 2017; Hoves et al., 2018). Using the combination of CD40 agonists and anti-CSF1R antibodies enhanced the cytotoxicity of T cells (Xiang et al., 2021). The inhibition of the NF-κB pathway by the siRNA pathway could transform TAM into M1 macrophages (Ortega et al., 2016). Other molecules also impact the polarization of TAMs, such as HDAC, and the microRNA processing enzyme DICER, because blocking HDAC or DICER could produce M1 phenotypes (Tie et al., 2022a; Tajaldini et al., 2022). Third, restoring phagocytic capacity is crucial for TAMs. The activated SIRPα–CD47 axis could limit the TAM phagocytic capacity for cancer cells. Therefore, targeting SIRPα has been proposed and tested in pancreatic cancer and breast cancer, where it strengthens the phagocytosis ability of macrophages and promotes tumor cell death (Jaiswal et al., 2009; Theocharides et al., 2012; Hu et al., 2020; Jia et al., 2021). Anti-CD47 antibodies have also been reported to strengthen the anti-tumor activity of macrophages (Brierley et al., 2019; Sikic et al., 2019; Jia et al., 2021), and some anti-CD47 mAbs in different tumors are in the clinical trials (NCT02953509, NCT04751383). Inhibition of leukocyte immunoglobulin-like receptor subfamily B (LILRB)- MHCI pathway axis could recover the phagocytic capacity of TAMs (Barkal et al., 2018). Finally, CAR expressed by TAMs has been reported to improve phagocytosis, transform TAMs to the M1 phenotype, and enhance anti-tumor immunity (Christofides et al., 2022). CAR for phagocytosis (CAR-P) could improve the phagocytic capacity of TAMs and hinder tumor cell growth in solid tumors (Morrissey et al., 2018; Kumari and Choi, 2022). CAR-macrophages (CAR-M) have also been developed, which inhibits tumor progression in a mouse model (Klichinsky et al., 2020; Villanueva, 2020; Sloas et al., 2021). Furthermore, a recent study proposed another tactic, anti-CCR7 CAR-M cells, which induces macrophages toward CCR7-positive cells and then deletes CCR7-positive cells by a series of activities (Kumari and Choi, 2022). At present, a novel method, targeting TAMs with nanomaterials, has been reported. The use of nanomaterials to inhibit tumor growth not only promotes anti-tumor immunity, but it also reduces the off-target effects and adverse events. The details of nanoimmunotherapies for TAMs have been concluded in this review (Kumari and Choi, 2022). Therefore, targeting nanoimmunotherapies is a promising option. Thus, more research is needed in the future.
In brief, although these therapeutic strategies have been successfully applied in mouse models and even in the clinical trials, targeting TAMs is still limited. At present, targeting all macrophage populations is less effective than targeting pro-tumor TAMs, so researchers want to further focus on targeting a specific macrophage population to reach maximal efficacy. Additionally, though the blockade of recruitment of TAMs is a potential option, it is a better strategy that converts TAMs from M2 to M1. Thus, further exploration needs to dissect the mechanisms of TAM polarization from top to bottom and develop novel treatment agents. Moreover, some inhibitory molecules have not been thoroughly discussed, including CD163, CD206, and TREM2. Thus, extensive investigations about targeting these molecules needs to be performed. Finally, the combination therapy of based-TAM and other ways like radiotherapy are also worthy of research.
Conclusion and perspectives
With the advancement of immunotherapies, it is well revealed that tumor-infiltrating immune cells play increasingly important roles and interact with the efficacy of targeting these immune cells. Therefore, the dissection of these cell properties in TME is indispensable for improving the clinical response of immunotherapies. In this review, we have dissected the characteristics of mainly tumor-infiltrating immune cells, including their phenotypes, their recruitment, their activation, and immune-based therapies (Figure 2). The interactions with these cells in TME are complicated due to the presence of anti-tumor and pro-tumor activities, which is associated with the clinical outcome of cancer patients. In addition, these cells also form a special structure which impacts the clinical outcome of tumor patients, but it remains in its infancy for TLS properties. Correspondingly, according to the characteristics of these immune cells, various immunotherapy approaches have been developed and are successful in preclinical tumor models and human tumors, including targeting cytokines and chemokines, targeting various phenotypes, and CAR-T. Recently, nanoimmunotherapies have been generated and acquired a better efficacy, which provides a novel approach to target tumor cells and is worthy of emulation. However, due to the conspicuously complex TME, the clinical response of some strategies is limited, even ineffective and resistant. Consequently, extensive complementary researches on tumor-infiltrating immune cells are necessary to overcome these shortcomings and further develop curative tactics for a conducive prognosis.
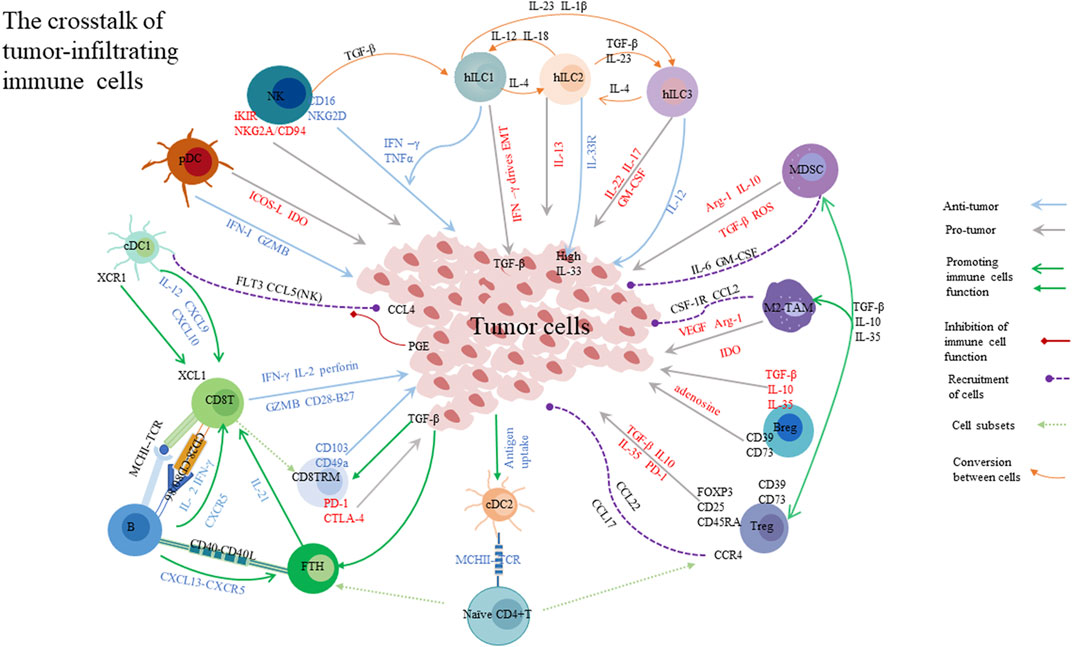
FIGURE 2. Tumor-infiltrating immune cells are important. Different cells play different roles. CD8+ T cell, CD8 TRM, and NK could kill tumor cells. Bregs, Tregs, MDSCs, and M2-TAM promote tumor cell growth. Tumor cells also secrete various molecules to disturb immune cell function. These molecules can convert cell phenotype and change their function, like NK cells. Specially, TGF-β derived from tumor cells could promote the function of CD8 TRM and Tfh cells. The crosstalk of these immune cells are is important for their function. Inhibitory cells secrete various immunosuppressive molecules to impair the cytotoxicity of effector cells. CD8 TRM: CD8 tissue resident memory; DC: dendritic cell; cDCs: conventional dendritic cells; pDCs: plasmacytoid DCs; Tfh: T follicular cell; NK: natural killer; hILC: helper innate lymphoid cells; Bregs: regulatory B cells; Tregs: regulatory T cells; MDSC: myeloid-derived suppressor cell; M2-TAM: M2 macrophages; EMT: epithelial mesenchymal transition.
Data availability statement
The original contributions presented in the study are included in the article/Supplementary Material, further inquiries can be directed to the corresponding authors.
Author contributions
Concept and design: LR, HN, and WY. Drafting of the manuscript: WY, CZ, LR, and FP. Critical revision of the manuscript: GL, JC, ZB, LL, HG, and WY. Obtained funding: LR. Administrative, technical, or material support: WY, HN, and WZ. Supervision: LR and HN. All authors contributed to the article and approved the submitted version.
Funding
This study was financially supported by the National Natural Science Foundation of China (No.81902677).
Conflict of interest
The authors declare that the research was conducted in the absence of any commercial or financial relationships that could be construed as a potential conflict of interest.
Publisher’s note
All claims expressed in this article are solely those of the authors and do not necessarily represent those of their affiliated organizations, or those of the publisher, the editors, and the reviewers. Any product that may be evaluated in this article, or claim that may be made by its manufacturer, is not guaranteed or endorsed by the publisher.
References
Abdeljaoued, S., Arfa, S., Kroemer, M., Ben Khelil, M., Vienot, A., Heyd, B., et al. (2022). Tissue-resident memory T cells in gastrointestinal cancer immunology and immunotherapy: Ready for prime time? J. Immunother. Cancer 10 (4), e003472. doi:10.1136/jitc-2021-003472
Adeshakin, A. O., Adeshakin, F. O., Yan, D., and Wan, X. (2022). Regulating histone deacetylase signaling pathways of myeloid-derived suppressor cells enhanced T cell-based immunotherapy. Front. Immunol. 13, 781660. doi:10.3389/fimmu.2022.781660
Adotevi, O., Godet, Y., Galaine, J., Lakkis, Z., Idirene, I., Certoux, J. M., et al. (2018). In situ delivery of allogeneic natural killer cell (NK) combined with cetuximab in liver metastases of gastrointestinal carcinoma: A phase I clinical trial. Oncoimmunology 7 (5), e1424673. doi:10.1080/2162402X.2018.1424673
Allard, B., Allard, D., Buisseret, L., and Stagg, J. (2020). The adenosine pathway in immuno-oncology. Nat. Rev. Clin. Oncol. 17 (10), 611–629. doi:10.1038/s41571-020-0382-2
Allen, E., Jabouille, A., Rivera, L. B., Lodewijckx, I., Missiaen, R., Steri, V., et al. (2017). Combined antiangiogenic and anti-PD-L1 therapy stimulates tumor immunity through HEV formation. Sci. Transl. Med. 9 (385), eaak9679. doi:10.1126/scitranslmed.aak9679
Amsen, D., van Gisbergen, K., Hombrink, P., and van Lier, R. A. W. (2018). Tissue-resident memory T cells at the center of immunity to solid tumors. Nat. Immunol. 19 (6), 538–546. doi:10.1038/s41590-018-0114-2
An, Z., Flores-Borja, F., Irshad, S., Deng, J., and Ng, T. (2019). Pleiotropic role and bidirectional immunomodulation of innate lymphoid cells in cancer. Front. Immunol. 10, 3111. doi:10.3389/fimmu.2019.03111
Anadon, C. M., Yu, X., Hänggi, K., Biswas, S., Chaurio, R. A., Martin, A., et al. (2022). Ovarian cancer immunogenicity is governed by a narrow subset of progenitor tissue-resident memory T cells. Cancer Cell. 40 (5), 545–557. e13. doi:10.1016/j.ccell.2022.03.008
Anagnostou, V., Smith, K. N., Forde, P. M., Niknafs, N., Bhattacharya, R., White, J., et al. (2017). Evolution of neoantigen landscape during immune checkpoint blockade in non-small cell lung cancer. Cancer Discov. 7 (3), 264–276. doi:10.1158/2159-8290.CD-16-0828
André, P., Denis, C., Soulas, C., Bourbon-Caillet, C., Lopez, J., Arnoux, T., et al. (2018). Anti-NKG2A mAb is a checkpoint inhibitor that promotes anti-tumor immunity by unleashing both T and NK cells. Cell. 175 (7), 1731–1743. e13. doi:10.1016/j.cell.2018.10.014
Andrew, J. G., Rajamanickam, V., Bui, C., Bernard, B., Pucilowska, J., Ballesteros-Merino, C., et al. (2021). Germinal center reactions in tertiary lymphoid structures associate with neoantigen burden, humoral immunity and long-term survivorship in pancreatic cancer. Oncoimmunology 10 (1), 1900635. doi:10.1080/2162402X.2021.1900635
Arce Vargas, F., Furness, A. J. S., Solomon, I., Joshi, K., Mekkaoui, L., Lesko, M. H., et al. (2017). Fc-optimized anti-CD25 depletes tumor-infiltrating regulatory T cells and synergizes with PD-1 blockade to eradicate established tumors. Immunity 46 (4), 577–586. doi:10.1016/j.immuni.2017.03.013
Arlauckas, S. P., Garren, S. B., Garris, C. S., Kohler, R. H., Oh, J., Pittet, M. J., et al. (2018). Arg1 expression defines immunosuppressive subsets of tumor-associated macrophages. Theranostics 8 (21), 5842–5854. doi:10.7150/thno.26888
Aspord, C., Leccia, M. T., Charles, J., and Plumas, J. (2013). Plasmacytoid dendritic cells support melanoma progression by promoting Th2 and regulatory immunity through OX40L and ICOSL. Cancer Immunol. Res. 1 (6), 402–415. doi:10.1158/2326-6066.CIR-13-0114-T
Asrir, A., Aloulou, M., Gador, M., Pérals, C., and Fazilleau, N. (2017). Interconnected subsets of memory follicular helper T cells have different effector functions. Nat. Commun. 8 (1), 847. doi:10.1038/s41467-017-00843-7
Avalos, A. M., and Ploegh, H. L. (2014). Early BCR events and antigen capture, processing, and loading on MHC class II on B cells. Front. Immunol. 5, 92. doi:10.3389/fimmu.2014.00092
Baek, S., Kim, Y. M., Kim, S. B., Kim, C. S., Kwon, S. W., Kim, Y., et al. (2015). Therapeutic DC vaccination with IL-2 as a consolidation therapy for ovarian cancer patients: A phase I/II trial. Cell. Mol. Immunol. 12 (1), 87–95. doi:10.1038/cmi.2014.40
Bald, T., Wagner, M., Gao, Y., Koyasu, S., and Smyth, M. J. (2019). Hide and seek: Plasticity of innate lymphoid cells in cancer. Semin. Immunol. 41, 101273. doi:10.1016/j.smim.2019.04.001
Bankovich, A. J., Shiow, L. R., and Cyster, J. G. (2010). CD69 suppresses sphingosine 1-phosophate receptor-1 (S1P1) function through interaction with membrane helix 4. J. Biol. Chem. 285 (29), 22328–22337. doi:10.1074/jbc.M110.123299
Barkal, A. A., Weiskopf, K., Kao, K. S., Gordon, S. R., Rosental, B., Yiu, Y. Y., et al. (2018). Engagement of MHC class I by the inhibitory receptor LILRB1 suppresses macrophages and is a target of cancer immunotherapy. Nat. Immunol. 19 (1), 76–84. doi:10.1038/s41590-017-0004-z
Barros, L., Ferreira, C., and Veldhoen, M. (2022). The fellowship of regulatory and tissue-resident memory cells. Mucosal Immunol. 15 (1), 64–73. doi:10.1038/s41385-021-00456-w
Barrow, A. D., Martin, C. J., and Colonna, M. (2019). The natural cytotoxicity receptors in health and disease. Front. Immunol. 10, 909. doi:10.3389/fimmu.2019.00909
Barry, K. C., Hsu, J., Broz, M. L., Cueto, F. J., Binnewies, M., Combes, A. J., et al. (2018). A natural killer-dendritic cell axis defines checkpoint therapy-responsive tumor microenvironments. Nat. Med. 24 (8), 1178–1191. doi:10.1038/s41591-018-0085-8
Bauer, R., Udonta, F., Wroblewski, M., Ben-Batalla, I., Santos, I. M., Taverna, F., et al. (2018). Blockade of myeloid-derived suppressor cell expansion with all-trans retinoic acid increases the efficacy of antiangiogenic therapy. Cancer Res. 78 (12), 3220–3232. doi:10.1158/0008-5472.CAN-17-3415
Benson, D. M., Cohen, A. D., Jagannath, S., Munshi, N. C., Spitzer, G., Hofmeister, C. C., et al. (2015). A phase I trial of the anti-KIR antibody IPH2101 and lenalidomide in patients with relapsed/refractory multiple myeloma. Clin. Cancer Res. 21 (18), 4055–4061. doi:10.1158/1078-0432.CCR-15-0304
Benson, D. M., Hofmeister, C. C., Padmanabhan, S., Suvannasankha, A., Jagannath, S., Abonour, R., et al. (2012). A phase 1 trial of the anti-KIR antibody IPH2101 in patients with relapsed/refractory multiple myeloma. Blood 120 (22), 4324–4333. doi:10.1182/blood-2012-06-438028
Bernink, J. H., Peters, C. P., Munneke, M., te Velde, A. A., Meijer, S. L., Weijer, K., et al. (2013). Human type 1 innate lymphoid cells accumulate in inflamed mucosal tissues. Nat. Immunol. 14 (3), 221–229. doi:10.1038/ni.2534
Berrien-Elliott, M. M., Becker-Hapak, M., Cashen, A. F., Jacobs, M., Wong, P., Foster, M., et al. (2022). Systemic IL-15 promotes allogeneic cell rejection in patients treated with natural killer cell adoptive therapy. Blood 139 (8), 1177–1183. doi:10.1182/blood.2021011532
Bhardwaj, N., Friedlander, P. A., Pavlick, A. C., Ernstoff, M. S., Gastman, B. R., Hanks, B. A., et al. (2020). Flt3 ligand augments immune responses to anti-DEC-205-NY-ESO-1 vaccine through expansion of dendritic cell subsets. Nat. Cancer 1 (12), 1204–1217. doi:10.1038/s43018-020-00143-y
Bi, G., Liang, J., Bian, Y., Shan, G., Besskaya, V., Wang, Q., et al. (2022). The immunomodulatory role of all-trans retinoic acid in tumor microenvironment. Clin. Exp. Med. doi:10.1007/s10238-022-00860-x
Binnewies, M., Mujal, A. M., Pollack, J. L., Combes, A. J., Hardison, E. A., Barry, K. C., et al. (2019). Unleashing type-2 dendritic cells to drive protective antitumor CD4(+) T cell immunity. Cell. 177 (3), 556–571. e16. doi:10.1016/j.cell.2019.02.005
Blattner, C., Fleming, V., Weber, R., Himmelhan, B., Altevogt, P., Gebhardt, C., et al. (2018). CCR5(+) myeloid-derived suppressor cells are enriched and activated in melanoma lesions. Cancer Res. 78 (1), 157–167. doi:10.1158/0008-5472.CAN-17-0348
Bogunovic, D., O'Neill, D. W., Belitskaya-Levy, I., Vacic, V., Yu, Y. L., Adams, S., et al. (2009). Immune profile and mitotic index of metastatic melanoma lesions enhance clinical staging in predicting patient survival. Proc. Natl. Acad. Sci. U. S. A. 106 (48), 20429–20434. doi:10.1073/pnas.0905139106
Böttcher, J. P., Bonavita, E., Chakravarty, P., Blees, H., Cabeza-Cabrerizo, M., Sammicheli, S., et al. (2018). NK cells stimulate recruitment of cDC1 into the tumor microenvironment promoting cancer immune control. Cell. 172 (5), 1022–1037. e14. doi:10.1016/j.cell.2018.01.004
Böttcher, J. P., and Reis e Sousa, C. (2018). The role of type 1 conventional dendritic cells in cancer immunity. Trends Cancer 4 (11), 784–792. doi:10.1016/j.trecan.2018.09.001
Bournazos, S., Wang, T. T., Dahan, R., Maamary, J., and Ravetch, J. V. (2017). Signaling by antibodies: Recent progress. Annu. Rev. Immunol. 35, 285–311. doi:10.1146/annurev-immunol-051116-052433
Boutet, M., Gauthier, L., Leclerc, M., Gros, G., de Montpreville, V., Théret, N., et al. (2016). TGFβ signaling intersects with CD103 integrin signaling to promote T-lymphocyte accumulation and antitumor activity in the lung tumor microenvironment. Cancer Res. 76 (7), 1757–1769. doi:10.1158/0008-5472.CAN-15-1545
Bresnick, A. R., Weber, D. J., and Zimmer, D. B. (2015). S100 proteins in cancer. Nat. Rev. Cancer 15 (2), 96–109. doi:10.1038/nrc3893
Briere, D., Sudhakar, N., Woods, D. M., Hallin, J., Engstrom, L. D., Aranda, R., et al. (2018). The class I/IV HDAC inhibitor mocetinostat increases tumor antigen presentation, decreases immune suppressive cell types and augments checkpoint inhibitor therapy. Cancer Immunol. Immunother. 67 (3), 381–392. doi:10.1007/s00262-017-2091-y
Brierley, C. K., Staves, J., Roberts, C., Johnson, H., Vyas, P., Goodnough, L. T., et al. (2019). The effects of monoclonal anti-CD47 on RBCs, compatibility testing, and transfusion requirements in refractory acute myeloid leukemia. Transfusion 59 (7), 2248–2254. doi:10.1111/trf.15397
Bromley, S. K., Akbaba, H., Mani, V., Mora-Buch, R., Chasse, A. Y., Sama, A., et al. (2020). CD49a regulates cutaneous resident memory CD8(+) T cell persistence and response. Cell. Rep. 32 (9), 108085. doi:10.1016/j.celrep.2020.108085
Brossart, P. (2022). Editorial: Versatile roles for B cells in tumor immunity. Front. Immunol. 13, 951933. doi:10.3389/fimmu.2022.951933
Bruno, T. C., Ebner, P. J., Moore, B. L., Squalls, O. G., Waugh, K. A., Eruslanov, E. B., et al. (2017). Antigen-presenting intratumoral B cells affect CD4(+) TIL phenotypes in non-small cell lung cancer patients. Cancer Immunol. Res. 5 (10), 898–907. doi:10.1158/2326-6066.CIR-17-0075
Bryceson, Y. T., March, M. E., Ljunggren, H. G., and Long, E. O. (2006). Activation, coactivation, and costimulation of resting human natural killer cells. Immunol. Rev. 214, 73–91. doi:10.1111/j.1600-065X.2006.00457.x
Bunse, M., Pfeilschifter, J., Bluhm, J., Zschummel, M., Joedicke, J. J., Wirges, A., et al. (2021). CXCR5 CAR-T cells simultaneously target B cell non-Hodgkin's lymphoma and tumor-supportive follicular T helper cells. Nat. Commun. 12 (1), 240. doi:10.1038/s41467-020-20488-3
Burnet, F. M. (1970). The concept of immunological surveillance. Prog. Exp. Tumor Res. 13, 1–27. doi:10.1159/000386035
Calderaro, J., Petitprez, F., Becht, E., Laurent, A., Hirsch, T. Z., Rousseau, B., et al. (2019). Intratumoral tertiary lymphoid structures are associated with a low risk of early recurrence of hepatocellular carcinoma. J. Hepatol. 70 (1), 58–65. doi:10.1016/j.jhep.2018.09.003
Cancel, J. C., Crozat, K., Dalod, M., and Mattiuz, R. (2019). Are conventional type 1 dendritic cells critical for protective antitumor immunity and how? Front. Immunol. 10, 9. doi:10.3389/fimmu.2019.00009
Cancro, M. P., and Tomayko, M. M. (2021). Memory B cells and plasma cells: The differentiative continuum of humoral immunity. Immunol. Rev. 303 (1), 72–82. doi:10.1111/imr.13016
Cannarile, M. A., Weisser, M., Jacob, W., Jegg, A. M., Ries, C. H., and Rüttinger, D. (2017). Colony-stimulating factor 1 receptor (CSF1R) inhibitors in cancer therapy. J. Immunother. Cancer 5 (1), 53. doi:10.1186/s40425-017-0257-y
Cao, X., Cai, S. F., Fehniger, T. A., Song, J., Collins, L. I., Piwnica-Worms, D. R., et al. (2007). Granzyme B and perforin are important for regulatory T cell-mediated suppression of tumor clearance. Immunity 27 (4), 635–646. doi:10.1016/j.immuni.2007.08.014
Carrega, P., Loiacono, F., Di Carlo, E., Scaramuccia, A., Mora, M., Conte, R., et al. (2015). NCR(+)ILC3 concentrate in human lung cancer and associate with intratumoral lymphoid structures. Nat. Commun. 6, 8280. doi:10.1038/ncomms9280
Carreno, B. M., Magrini, V., Becker-Hapak, M., Kaabinejadian, S., Hundal, J., Petti, A. A., et al. (2015). Cancer immunotherapy. A dendritic cell vaccine increases the breadth and diversity of melanoma neoantigen-specific T cells. Sci. (New York, NY) 348 (6236), 803–808. doi:10.1126/science.aaa3828
Cassetta, L., and Pollard, J. W. (2020). Tumor-associated macrophages. Curr. Biol. 30 (6), R246-R248–r8. doi:10.1016/j.cub.2020.01.031
Castenmiller, C., Keumatio-Doungtsop, B. C., van Ree, R., de Jong, E. C., and van Kooyk, Y. (2021). Tolerogenic immunotherapy: Targeting DC surface receptors to induce antigen-specific tolerance. Front. Immunol. 12, 643240. doi:10.3389/fimmu.2021.643240
Castro, F., Cardoso, A. P., Gonçalves, R. M., Serre, K., and Oliveira, M. J. (2018). Interferon-Gamma at the crossroads of tumor immune surveillance or evasion. Front. Immunol. 9, 847. doi:10.3389/fimmu.2018.00847
Cella, M., Gamini, R., Sécca, C., Collins, P. L., Zhao, S., Peng, V., et al. (2019). Subsets of ILC3-ILC1-like cells generate a diversity spectrum of innate lymphoid cells in human mucosal tissues. Nat. Immunol. 20 (8), 980–991. doi:10.1038/s41590-019-0425-y
Chang, Y. H., Connolly, J., Shimasaki, N., Mimura, K., Kono, K., and Campana, D. (2013). A chimeric receptor with NKG2D specificity enhances natural killer cell activation and killing of tumor cells. Cancer Res. 73 (6), 1777–1786. doi:10.1158/0008-5472.CAN-12-3558
Chaurio, R. A., Anadon, C. M., Lee Costich, T., Payne, K. K., Biswas, S., Harro, C. M., et al. (2022). TGF-β-mediated silencing of genomic organizer SATB1 promotes Tfh cell differentiation and formation of intratumoral tertiary lymphoid structures. Immunity 55 (1), 115–128.e9. e9. doi:10.1016/j.immuni.2021.12.007
Chen, H., Huang, S., Niu, P., Zhu, Y., Zhou, J., Jiang, L., et al. (2022). Cardamonin suppresses pro-tumor function of macrophages by decreasing M2 polarization on ovarian cancer cells via mTOR inhibition. Mol. Ther. Oncolytics 26, 175–188. doi:10.1016/j.omto.2022.06.009
Chen, L., Hasni, M. S., Jondal, M., and Yakimchuk, K. (2017). Modification of anti-tumor immunity by tolerogenic dendritic cells. Autoimmunity 50 (6), 370–376. doi:10.1080/08916934.2017.1344837
Chen, Y., You, S., Li, J., Zhang, Y., Kokaraki, G., Epstein, E., et al. (2021). Follicular helper T-cell-based classification of endometrial cancer promotes precise checkpoint immunotherapy and provides prognostic stratification. Front. Immunol. 12, 788959. doi:10.3389/fimmu.2021.788959
Cheuk, S., Schlums, H., Gallais Sérézal, I., Martini, E., Chiang, S. C., Marquardt, N., et al. (2017). CD49a expression defines tissue-resident CD8(+) T cells poised for cytotoxic function in human skin. Immunity 46 (2), 287–300. doi:10.1016/j.immuni.2017.01.009
Chevalier, M. F., Trabanelli, S., Racle, J., Salomé, B., Cesson, V., Gharbi, D., et al. (2017). ILC2-modulated T cell-to-MDSC balance is associated with bladder cancer recurrence. J. Clin. Investig. 127 (8), 2916–2929. doi:10.1172/JCI89717
Chinen, T., Kannan, A. K., Levine, A. G., Fan, X., Klein, U., Zheng, Y., et al. (2016). An essential role for the IL-2 receptor in T(reg) cell function. Nat. Immunol. 17 (11), 1322–1333. doi:10.1038/ni.3540
Chiossone, L., Dumas, P. Y., Vienne, M., and Vivier, E. (2018). Natural killer cells and other innate lymphoid cells in cancer. Nat. Rev. Immunol. 18 (11), 671–688. doi:10.1038/s41577-018-0061-z
Chow, M. T., Ozga, A. J., Servis, R. L., Frederick, D. T., Lo, J. A., Fisher, D. E., et al. (2019). Intratumoral activity of the CXCR3 chemokine system is required for the efficacy of anti-PD-1 therapy. Immunity 50 (6), 1498–1512. e5. doi:10.1016/j.immuni.2019.04.010
Christmas, B. J., Rafie, C. I., Hopkins, A. C., Scott, B. A., Ma, H. S., Cruz, K. A., et al. (2018). Entinostat converts immune-resistant breast and pancreatic cancers into checkpoint-responsive tumors by reprogramming tumor-infiltrating MDSCs. Cancer Immunol. Res. 6 (12), 1561–1577. doi:10.1158/2326-6066.CIR-18-0070
Christo, S. N., Evrard, M., Park, S. L., Gandolfo, L. C., Burn, T. N., Fonseca, R., et al. (2021). Discrete tissue microenvironments instruct diversity in resident memory T cell function and plasticity. Nat. Immunol. 22 (9), 1140–1151. doi:10.1038/s41590-021-01004-1
Christofides, A., Strauss, L., Yeo, A., Cao, C., Charest, A., and Boussiotis, V. A. (2022). The complex role of tumor-infiltrating macrophages. Nat. Immunol. 23 (8), 1148–1156. doi:10.1038/s41590-022-01267-2
Cichocki, F., Bjordahl, R., Gaidarova, S., Mahmood, S., Abujarour, R., Wang, H., et al. (2020). iPSC-derived NK cells maintain high cytotoxicity and enhance in vivo tumor control in concert with T cells and anti-PD-1 therapy. Sci. Transl. Med. 12 (568), eaaz5618. doi:10.1126/scitranslmed.aaz5618
Cipponi, A., Mercier, M., Seremet, T., Baurain, J. F., Théate, I., van den Oord, J., et al. (2012). Neogenesis of lymphoid structures and antibody responses occur in human melanoma metastases. Cancer Res. 72 (16), 3997–4007. doi:10.1158/0008-5472.CAN-12-1377
Ciucci, T., Vacchio, M. S., Chen, T., Nie, J., Chopp, L. B., McGavern, D. B., et al. (2022). Dependence on Bcl6 and Blimp1 drive distinct differentiation of murine memory and follicular helper CD4+ T cells. J. Exp. Med. 219 (1), e20202343. doi:10.1084/jem.20202343
Ciurea, S. O., Schafer, J. R., Bassett, R., Denman, C. J., Cao, K., Willis, D., et al. (2017). Phase 1 clinical trial using mbIL21 ex vivo-expanded donor-derived NK cells after haploidentical transplantation. Blood 130 (16), 1857–1868. doi:10.1182/blood-2017-05-785659
Cogné, M., Fest, T., and Aoufouchi, S. (2022). Editorial: Germinal centers in lymphoid and non-lymphoid tissues: Adaptive and evolving structures. Front. Immunol. 13, 880733. doi:10.3389/fimmu.2022.880733
Colak, S., and Ten Dijke, P. (2017). Targeting TGF-β signaling in cancer. Trends Cancer 3 (1), 56–71. doi:10.1016/j.trecan.2016.11.008
Colbeck, E. J., Jones, E., Hindley, J. P., Smart, K., Schulz, R., Browne, M., et al. (2017). Treg depletion licenses T cell-driven HEV neogenesis and promotes tumor destruction. Cancer Immunol. Res. 5 (11), 1005–1015. doi:10.1158/2326-6066.CIR-17-0131
Colluru, V. T., and McNeel, D. G. (2016). B lymphocytes as direct antigen-presenting cells for anti-tumor DNA vaccines. Oncotarget 7 (42), 67901–67918. doi:10.18632/oncotarget.12178
Condamine, T., Kumar, V., Ramachandran, I. R., Youn, J. I., Celis, E., Finnberg, N., et al. (2014). ER stress regulates myeloid-derived suppressor cell fate through TRAIL-R-mediated apoptosis. J. Clin. Investig. 124 (6), 2626–2639. doi:10.1172/JCI74056
Cooper, M. A., Fehniger, T. A., Ponnappan, A., Mehta, V., Wewers, M. D., and Caligiuri, M. A. (2001). Interleukin-1beta costimulates interferon-gamma production by human natural killer cells. Eur. J. Immunol. 31 (3), 792–801. doi:10.1002/1521-4141(200103)31:3<792:aid-immu792>3.0.co;2-u
Cortez, V. S., Ulland, T. K., Cervantes-Barragan, L., Bando, J. K., Robinette, M. L., Wang, Q., et al. (2017). SMAD4 impedes the conversion of NK cells into ILC1-like cells by curtailing non-canonical TGF-β signaling. Nat. Immunol. 18 (9), 995–1003. doi:10.1038/ni.3809
Couper, K. N., Blount, D. G., and Riley, E. M. (1950). IL-10: The master regulator of immunity to infection. J. Immunol. 180 (9), 5771–5777. doi:10.4049/jimmunol.180.9.5771
Craig, D. J., Creeden, J. F., Einloth, K. R., Gillman, C. E., Stanbery, L., Hamouda, D., et al. (2020). Resident memory T cells and their effect on cancer. Vaccines 8 (4), E562. doi:10.3390/vaccines8040562
Crotty, S. T. (2019). T follicular helper cell biology: A decade of discovery and diseases. Immunity 50 (5), 1132–1148. doi:10.1016/j.immuni.2019.04.011
Cuff, A. O., Sillito, F., Dertschnig, S., Hall, A., Luong, T. V., Chakraverty, R., et al. (2019). The obese liver environment mediates conversion of NK cells to a less cytotoxic ILC1-like phenotype. Front. Immunol. 10, 2180. doi:10.3389/fimmu.2019.02180
Cui, C., Wang, J., Fagerberg, E., Chen, P. M., Connolly, K. A., Damo, M., et al. (2021). Neoantigen-driven B cell and CD4 T follicular helper cell collaboration promotes anti-tumor CD8 T cell responses. Cell. 184 (25), 6101–6118. e13. doi:10.1016/j.cell.2021.11.007
Cursons, J., Souza-Fonseca-Guimaraes, F., Foroutan, M., Anderson, A., Hollande, F., Hediyeh-Zadeh, S., et al. (2019). A gene signature predicting natural killer cell infiltration and improved survival in melanoma patients. Cancer Immunol. Res. 7 (7), 1162–1174. doi:10.1158/2326-6066.CIR-18-0500
Dadi, S., Chhangawala, S., Whitlock, B. M., Franklin, R. A., Luo, C. T., Oh, S. A., et al. (2016). Cancer immunosurveillance by tissue-resident innate lymphoid cells and innate-like T cells. Cell. 164 (3), 365–377. doi:10.1016/j.cell.2016.01.002
Daher, M., and Rezvani, K. (2021). Outlook for new CAR-based therapies with a focus on CAR NK cells: What lies beyond CAR-engineered T cells in the race against cancer. Cancer Discov. 11 (1), 45–58. doi:10.1158/2159-8290.CD-20-0556
Davis, Z. B., Cooley, S. A., Cichocki, F., Felices, M., Wangen, R., Luo, X., et al. (2015). Adaptive natural killer cell and killer cell immunoglobulin-like receptor-expressing T cell responses are induced by Cytomegalovirus and are associated with protection against Cytomegalovirus reactivation after allogeneic donor hematopoietic cell transplantation. Biol. Blood Marrow Transpl. 21 (9), 1653–1662. doi:10.1016/j.bbmt.2015.05.025
De Cicco, P., Ercolano, G., and Ianaro, A. (2020). The new era of cancer immunotherapy: Targeting myeloid-derived suppressor cells to overcome immune evasion. Front. Immunol. 11, 1680. doi:10.3389/fimmu.2020.01680
De, I., Steffen, M. D., Clark, P. A., Patros, C. J., Sokn, E., Bishop, S. M., et al. (2016). CSF1 overexpression promotes high-grade glioma formation without impacting the polarization status of glioma-associated microglia and macrophages. Cancer Res. 76 (9), 2552–2560. doi:10.1158/0008-5472.CAN-15-2386
de Mingo Pulido, Á., Gardner, A., Hiebler, S., Soliman, H., Rugo, H. S., Krummel, M. F., et al. (2018). TIM-3 regulates CD103(+) dendritic cell function and response to chemotherapy in breast cancer. Cancer Cell. 33 (1), 60–74. e6. doi:10.1016/j.ccell.2017.11.019
de Mingo Pulido, Á., Hänggi, K., Celias, D. P., Gardner, A., Li, J., Batista-Bittencourt, B., et al. (2021). The inhibitory receptor TIM-3 limits activation of the cGAS-STING pathway in intratumoral dendritic cells by suppressing extracellular DNA uptake. Immunity 54 (6), 1154–1167.e7. e7. doi:10.1016/j.immuni.2021.04.019
de Weerdt, I., van Hoeven, V., Munneke, J. M., Endstra, S., Hofland, T., Hazenberg, M. D., et al. (2016). Innate lymphoid cells are expanded and functionally altered in chronic lymphocytic leukemia. Haematologica 101 (11), e461–e464. doi:10.3324/haematol.2016.144725
Demaria, O., Gauthier, L., Debroas, G., and Vivier, E. (2021). Natural killer cell engagers in cancer immunotherapy: Next generation of immuno-oncology treatments. Eur. J. Immunol. 51 (8), 1934–1942. doi:10.1002/eji.202048953
Di Caro, G., Bergomas, F., Grizzi, F., Doni, A., Bianchi, P., Malesci, A., et al. (2014). Occurrence of tertiary lymphoid tissue is associated with T-cell infiltration and predicts better prognosis in early-stage colorectal cancers. Clin. Cancer Res. 20 (8), 2147–2158. doi:10.1158/1078-0432.CCR-13-2590
Dieu-Nosjean, M. C., Antoine, M., Danel, C., Heudes, D., Wislez, M., Poulot, V., et al. (2008). Long-term survival for patients with non-small-cell lung cancer with intratumoral lymphoid structures. J. Clin. Oncol. 26 (27), 4410–4417. doi:10.1200/JCO.2007.15.0284
Dieu-Nosjean, M. C., Giraldo, N. A., Kaplon, H., Germain, C., Fridman, W. H., and Sautès-Fridman, C. (2016). Tertiary lymphoid structures, drivers of the anti-tumor responses in human cancers. Immunol. Rev. 271 (1), 260–275. doi:10.1111/imr.12405
Ding, Y., Shen, J., Zhang, G., Chen, X., Wu, J., and Chen, W. (2015). CD40 controls CXCR5-induced recruitment of myeloid-derived suppressor cells to gastric cancer. Oncotarget 6 (36), 38901–38911. doi:10.18632/oncotarget.5644
Dixon, M. L., Leavenworth, J. D., and Leavenworth, J. W. (2021). Lineage reprogramming of effector regulatory T cells in cancer. Front. Immunol. 12, 717421. doi:10.3389/fimmu.2021.717421
Dominguez, G. A., Condamine, T., Mony, S., Hashimoto, A., Wang, F., Liu, Q., et al. (2017). Selective targeting of myeloid-derived suppressor cells in cancer patients using DS-8273a, an agonistic TRAIL-R2 antibody. Clin. Cancer Res. 23 (12), 2942–2950. doi:10.1158/1078-0432.CCR-16-1784
Dong, C. (2021). Cytokine regulation and function in T cells. Annu. Rev. Immunol. 39, 51–76. doi:10.1146/annurev-immunol-061020-053702
Du, Y., and Wei, Y. (2018). Therapeutic potential of natural killer cells in gastric cancer. Front. Immunol. 9, 3095. doi:10.3389/fimmu.2018.03095
Dubois, S. P., Miljkovic, M. D., Fleisher, T. A., Pittaluga, S., Hsu-Albert, J., Bryant, B. R., et al. (2021). Short-course IL-15 given as a continuous infusion led to a massive expansion of effective NK cells: Implications for combination therapy with antitumor antibodies. J. Immunother. Cancer 9 (4), e002193. doi:10.1136/jitc-2020-002193
Duhan, V., and Smyth, M. J. (2021). Innate myeloid cells in the tumor microenvironment. Curr. Opin. Immunol. 69, 18–28. doi:10.1016/j.coi.2021.01.001
Dumauthioz, N., Labiano, S., and Romero, P. (2018). Tumor resident memory T cells: New players in immune surveillance and therapy. Front. Immunol. 9, 2076. doi:10.3389/fimmu.2018.02076
Dunn, G. P., Bruce, A. T., Ikeda, H., Old, L. J., and Schreiber, R. D. (2002). Cancer immunoediting: From immunosurveillance to tumor escape. Nat. Immunol. 3 (11), 991–998. doi:10.1038/ni1102-991
Eckl, J., Buchner, A., Prinz, P. U., Riesenberg, R., Siegert, S. I., Kammerer, R., et al. (2012). Transcript signature predicts tissue NK cell content and defines renal cell carcinoma subgroups independent of TNM staging. J. Mol. Med. 90 (1), 55–66. doi:10.1007/s00109-011-0806-7
Edwards, J., Wilmott, J. S., Madore, J., Gide, T. N., Quek, C., Tasker, A., et al. (2018). CD103(+) tumor-resident CD8(+) T cells are associated with improved survival in immunotherapy-naïve melanoma patients and expand significantly during anti-PD-1 treatment. Clin. Cancer Res. 24 (13), 3036–3045. doi:10.1158/1078-0432.CCR-17-2257
Eisenring, M., vom Berg, J., Kristiansen, G., Saller, E., and Becher, B. (2010). IL-12 initiates tumor rejection via lymphoid tissue-inducer cells bearing the natural cytotoxicity receptor NKp46. Nat. Immunol. 11 (11), 1030–1038. doi:10.1038/ni.1947
Enamorado, M., Khouili, S. C., Iborra, S., and Sancho, D. (2018). Genealogy, dendritic cell priming, and differentiation of tissue-resident memory CD8(+) T cells. Front. Immunol. 9, 1751. doi:10.3389/fimmu.2018.01751
Entwistle, L. J., Gregory, L. G., Oliver, R. A., Branchett, W. J., Puttur, F., and Lloyd, C. M. (2019). Pulmonary group 2 innate lymphoid cell phenotype is context specific: Determining the effect of strain, location, and stimuli. Front. Immunol. 10, 3114. doi:10.3389/fimmu.2019.03114
Ercolano, G., Gomez-Cadena, A., Dumauthioz, N., Vanoni, G., Kreutzfeldt, M., Wyss, T., et al. (2021). PPARɣ drives IL-33-dependent ILC2 pro-tumoral functions. Nat. Commun. 12 (1), 2538. doi:10.1038/s41467-021-22764-2
Eruslanov, E., Daurkin, I., Ortiz, J., Vieweg, J., and Kusmartsev, S. (2010). Pivotal Advance: Tumor-mediated induction of myeloid-derived suppressor cells and M2-polarized macrophages by altering intracellular PGE₂ catabolism in myeloid cells. J. Leukoc. Biol. 88 (5), 839–848. doi:10.1189/jlb.1209821
Eryn, B., and Ott, P. (2021). Advances in the development of personalized neoantigen-based therapeutic cancer vaccines. Nat. Rev. Clin. Oncol. 18, 215–229. doi:10.1038/s41571-020-00460-2
Evans, D. E., Munks, M. W., Purkerson, J. M., and Parker, D. C. (1950)., 164. Baltimore, Md, 688–697. doi:10.4049/jimmunol.164.2.688Resting B lymphocytes as APC for naive T lymphocytes: Dependence on CD40 ligand/CD40J. Immunol.2
Fehniger, T. A., Shah, M. H., Turner, M. J., VanDeusen, J. B., Whitman, S. P., Cooper, M. A., et al. (1950). Differential cytokine and chemokine gene expression by human NK cells following activation with IL-18 or IL-15 in combination with IL-12: Implications for the innate immune response. J. Immunol. 162 (8), 4511–4520.
Felices, M., Kodal, B., Hinderlie, P., Kaminski, M. F., Cooley, S., Weisdorf, D. J., et al. (2019). Novel CD19-targeted TriKE restores NK cell function and proliferative capacity in CLL. Blood Adv. 3 (6), 897–907. doi:10.1182/bloodadvances.2018029371
Ferrari de Andrade, L., Tay, R. E., Pan, D., Luoma, A. M., Ito, Y., Badrinath, S., et al. (2018). Antibody-mediated inhibition of MICA and MICB shedding promotes NK cell-driven tumor immunity. Sci. (New York, NY) 359 (6383), 1537–1542. doi:10.1126/science.aao0505
Ferris, S. T., Durai, V., Wu, R., Theisen, D. J., Ward, J. P., Bern, M. D., et al. (2020). cDC1 prime and are licensed by CD4(+) T cells to induce anti-tumour immunity. Nature 584 (7822), 624–629. doi:10.1038/s41586-020-2611-3
Finkin, S., Yuan, D., Stein, I., Taniguchi, K., Weber, A., Unger, K., et al. (2015). Ectopic lymphoid structures function as microniches for tumor progenitor cells in hepatocellular carcinoma. Nat. Immunol. 16 (12), 1235–1244. doi:10.1038/ni.3290
Flores-Borja, F., and Blair, P. (2022). Mechanisms of induction of regulatory B cells in the tumour microenvironment and their contribution to immunosuppression and pro-tumour responses. Clin. Exp. Immunol. 209 (1), 33–45. doi:10.1093/cei/uxac029
Freud, A. G., Mundy-Bosse, B. L., Yu, J., and Caligiuri, M. A. (2017). The broad spectrum of human natural killer cell diversity. Immunity 47 (5), 820–833. doi:10.1016/j.immuni.2017.10.008
Fridman, W., Petitprez, F., Meylan, M., Chen, T., Cheng-ming, S., Roumenina, L., et al. (2020). B cells and cancer: To B or not to B? J. Exp. Med., 218. doi:10.1084/jem.20200851
Fu, C., Zhou, L., Mi, Q. S., and Jiang, A. (2022). Plasmacytoid dendritic cells and cancer immunotherapy. Cells 11 (2), 222. doi:10.3390/cells11020222
Fujita, M., Kohanbash, G., Fellows-Mayle, W., Hamilton, R. L., Komohara, Y., Decker, S. A., et al. (2011). COX-2 blockade suppresses gliomagenesis by inhibiting myeloid-derived suppressor cells. Cancer Res. 71 (7), 2664–2674. doi:10.1158/0008-5472.CAN-10-3055
Gabriely, G., da Cunha, A. P., Rezende, R. M., Kenyon, B., Madi, A., Vandeventer, T., et al. (2017). Targeting latency-associated peptide promotes antitumor immunity. Sci. Immunol. 2 (11), eaaj1738. doi:10.1126/sciimmunol.aaj1738
Gabrilovich, D. I. (2017). Myeloid-derived suppressor cells. Cancer Immunol. Res. 5 (1), 3–8. doi:10.1158/2326-6066.CIR-16-0297
Gagliani, N., Amezcua Vesely, M. C., Iseppon, A., Brockmann, L., Xu, H., Palm, N. W., et al. (2015). Th17 cells transdifferentiate into regulatory T cells during resolution of inflammation. Nature 523 (7559), 221–225. doi:10.1038/nature14452
Ganesan, A. P., Clarke, J., Wood, O., Garrido-Martin, E. M., Chee, S. J., Mellows, T., et al. (2017). Tissue-resident memory features are linked to the magnitude of cytotoxic T cell responses in human lung cancer. Nat. Immunol. 18 (8), 940–950. doi:10.1038/ni.3775
Gao, J., Shi, L. Z., Zhao, H., Chen, J., Xiong, L., He, Q., et al. (2016). Loss of IFN-γ pathway genes in tumor cells as a mechanism of resistance to anti-CTLA-4 therapy. Cell. 167 (2), 397–404. e9. doi:10.1016/j.cell.2016.08.069
Gao, Y., Souza-Fonseca-Guimaraes, F., Bald, T., Ng, S. S., Young, A., Ngiow, S. F., et al. (2017). Tumor immunoevasion by the conversion of effector NK cells into type 1 innate lymphoid cells. Nat. Immunol. 18 (9), 1004–1015. doi:10.1038/ni.3800
Garaud, S., Zayakin, P., Buisseret, L., Rulle, U., Silina, K., de Wind, A., et al. (2018). Antigen specificity and clinical significance of IgG and IgA autoantibodies produced in situ by tumor-infiltrating B cells in breast cancer. Front. Immunol. 9, 2660. doi:10.3389/fimmu.2018.02660
García-Hernández, M. L., Uribe-Uribe, N. O., Espinosa-González, R., Kast, W. M., Khader, S. A., and Rangel-Moreno, J. (2017). A unique cellular and molecular microenvironment is present in tertiary lymphoid organs of patients with spontaneous prostate cancer regression. Front. Immunol. 8, 563. doi:10.3389/fimmu.2017.00563
Garg, R., Blando, J. M., Perez, C. J., Abba, M. C., Benavides, F., and Kazanietz, M. G. (2017). Protein kinase C epsilon cooperates with PTEN loss for prostate tumorigenesis through the CXCL13-CXCR5 pathway. Cell. Rep. 19 (2), 375–388. doi:10.1016/j.celrep.2017.03.042
Garris, C. S., Arlauckas, S. P., Kohler, R. H., Trefny, M. P., Garren, S., Piot, C., et al. (2018). Successful anti-PD-1 cancer immunotherapy requires T cell-dendritic cell crosstalk involving the cytokines IFN-γ and IL-12. Immunity 49 (6), 1148–1161. e7. doi:10.1016/j.immuni.2018.09.024
Gattinoni, L., Speiser, D. E., Lichterfeld, M., and Bonini, C. (2017). T memory stem cells in health and disease. Nat. Med. 23 (1), 18–27. doi:10.1038/nm.4241
Gauthier, L., Corgnac, S., Boutet, M., Gros, G., Validire, P., Bismuth, G., et al. (2017). Paxillin binding to the cytoplasmic domain of CD103 promotes cell adhesion and effector functions for CD8(+) resident memory T cells in tumors. Cancer Res. 77 (24), 7072–7082. doi:10.1158/0008-5472.CAN-17-1487
Gebhardt, T., Wakim, L. M., Eidsmo, L., Reading, P. C., Heath, W. R., and Carbone, F. R. (2009). Memory T cells in nonlymphoid tissue that provide enhanced local immunity during infection with herpes simplex virus. Nat. Immunol. 10 (5), 524–530. doi:10.1038/ni.1718
Gerloni, M., Rizzi, M., Castiglioni, P., and Zanetti, M. (2004). T cell immunity using transgenic B lymphocytes. Proc. Natl. Acad. Sci. U. S. A. 101 (11), 3892–3897. doi:10.1073/pnas.0400138101
Germain, C., Gnjatic, S., Tamzalit, F., Knockaert, S., Remark, R., Goc, J., et al. (2014). Presence of B cells in tertiary lymphoid structures is associated with a protective immunity in patients with lung cancer. Am. J. Respir. Crit. Care Med. 189 (7), 832–844. doi:10.1164/rccm.201309-1611OC
Ghilas, S., Valencia-Hernandez, A. M., Enders, M. H., Heath, W. R., and Fernandez-Ruiz, D. (2020). Resident memory T cells and their role within the liver. Int. J. Mol. Sci. 21 (22), E8565. doi:10.3390/ijms21228565
Ghiringhelli, F., Ménard, C., Terme, M., Flament, C., Taieb, J., Chaput, N., et al. (2005). CD4+CD25+ regulatory T cells inhibit natural killer cell functions in a transforming growth factor-beta-dependent manner. J. Exp. Med. 202 (8), 1075–1085. doi:10.1084/jem.20051511
Giraldo, N. A., Becht, E., Pagès, F., Skliris, G., Verkarre, V., Vano, Y., et al. (2015). Orchestration and prognostic significance of immune checkpoints in the microenvironment of primary and metastatic renal cell cancer. Clin. Cancer Res. 21 (13), 3031–3040. doi:10.1158/1078-0432.CCR-14-2926
Gobert, M., Treilleux, I., Bendriss-Vermare, N., Bachelot, T., Goddard-Leon, S., Arfi, V., et al. (2009). Regulatory T cells recruited through CCL22/CCR4 are selectively activated in lymphoid infiltrates surrounding primary breast tumors and lead to an adverse clinical outcome. Cancer Res. 69 (5), 2000–2009. doi:10.1158/0008-5472.CAN-08-2360
Goc, J., Germain, C., Vo-Bourgais, T. K., Lupo, A., Klein, C., Knockaert, S., et al. (2014). Dendritic cells in tumor-associated tertiary lymphoid structures signal a Th1 cytotoxic immune contexture and license the positive prognostic value of infiltrating CD8+ T cells. Cancer Res. 74 (3), 705–715. doi:10.1158/0008-5472.CAN-13-1342
Goc, J., Hepworth, M. R., and Sonnenberg, G. F. (2016). Group 3 innate lymphoid cells: Regulating host-commensal bacteria interactions in inflammation and cancer. Int. Immunol. 28 (1), 43–52. doi:10.1093/intimm/dxv056
Goossens, P., Rodriguez-Vita, J., Etzerodt, A., Masse, M., Rastoin, O., Gouirand, V., et al. (2019). Membrane cholesterol efflux drives tumor-associated macrophage reprogramming and tumor progression. Cell. Metab. 29 (6), 1376–1389. e4. doi:10.1016/j.cmet.2019.02.016
Gordon, S. M., Chaix, J., Rupp, L. J., Wu, J., Madera, S., Sun, J. C., et al. (2012). The transcription factors T-bet and Eomes control key checkpoints of natural killer cell maturation. Immunity 36 (1), 55–67. doi:10.1016/j.immuni.2011.11.016
Groeneveld, C. S., Fontugne, J., Cabel, L., Bernard-Pierrot, I., Radvanyi, F., Allory, Y., et al. (1990). Tertiary lymphoid structures marker CXCL13 is associated with better survival for patients with advanced-stage bladder cancer treated with immunotherapy, 148. Oxford, England: European journal of cancer, 181–189.
Gu-Trantien, C., Migliori, E., Buisseret, L., de Wind, A., Brohée, S., Garaud, S., et al. (2017). CXCL13-producing TFH cells link immune suppression and adaptive memory in human breast cancer. JCI insight 2 (11), 91487. doi:10.1172/jci.insight.91487
Guan, Y. H., Wang, N., Deng, Z. W., Chen, X. G., and Liu, Y. (2022). Exploiting autophagy-regulative nanomaterials for activation of dendritic cells enables reinforced cancer immunotherapy. Biomaterials 282, 121434. doi:10.1016/j.biomaterials.2022.121434
Guillerey, C., Huntington, N. D., and Smyth, M. J. (2016). Targeting natural killer cells in cancer immunotherapy. Nat. Immunol. 17 (9), 1025–1036. doi:10.1038/ni.3518
Guo, S., Han, F., and Zhu, W. (2022). CD39 - a bright target for cancer immunotherapy. Biomed. Pharmacother. = Biomedecine Pharmacother. 151, 113066. doi:10.1016/j.biopha.2022.113066
Guo, Y., Nemeth, J., O'Brien, C., Susa, M., Liu, X., Zhang, Z., et al. (2010). Effects of siltuximab on the IL-6-induced signaling pathway in ovarian cancer. Clin. Cancer Res. 16 (23), 5759–5769. doi:10.1158/1078-0432.CCR-10-1095
Guo, Y. Q., Lu, P., Duan, Z. F., and Zhang, Z. (2010). Effects of siltuximab on the interleukin-6/Stat3 signaling pathway in ovarian cancer. Zhonghua fu chan ke za zhi 45 (11), 854–859.
Hamann, D., Baars, P. A., Rep, M. H., Hooibrink, B., Kerkhof-Garde, S. R., Klein, M. R., et al. (1997). Phenotypic and functional separation of memory and effector human CD8+ T cells. J. Exp. Med. 186 (9), 1407–1418. doi:10.1084/jem.186.9.1407
Han, J., Chu, J., Keung Chan, W., Zhang, J., Wang, Y., Cohen, J. B., et al. (2015). CAR-engineered NK cells targeting wild-type EGFR and EGFRvIII enhance killing of glioblastoma and patient-derived glioblastoma stem cells. Sci. Rep. 5, 11483. doi:10.1038/srep11483
Han, L., Gao, Q. L., Zhou, X. M., Shi, C., Chen, G. Y., Song, Y. P., et al. (2020). Characterization of CD103+ CD8+ tissue-resident T cells in esophageal squamous cell carcinoma: May be tumor reactive and resurrected by anti-PD-1 blockade. Cancer Immunol. Immunother. 69 (8), 1493–1504. doi:10.1007/s00262-020-02562-3
Han, X., Huang, T., and Han, J. (2019). Cytokines derived from innate lymphoid cells assist Helicobacter hepaticus to aggravate hepatocellular tumorigenesis in viral transgenic mice. Gut Pathog. 11, 23. doi:10.1186/s13099-019-0302-0
Hariyanto, A. D., Permata, T. B. M., and Gondhowiardjo, S. A. (2022). Role of CD4(+)CD25(+)FOXP3(+) T(Reg) cells on tumor immunity. Immunol. Med. 45 (2), 94–107. doi:10.1080/25785826.2021.1975228
Hart, A. P., and Laufer, T. M. (2022). A review of signaling and transcriptional control in T follicular helper cell differentiation. J. Leukoc. Biol. 111 (1), 173–195. doi:10.1002/JLB.1RI0121-066R
Hartwig, T., Montinaro, A., von Karstedt, S., Sevko, A., Surinova, S., Chakravarthy, A., et al. (2017). The TRAIL-induced cancer secretome promotes a tumor-supportive immune microenvironment via CCR2. Mol. Cell. 65 (4), 730–742. e5. doi:10.1016/j.molcel.2017.01.021
Hashimoto, A., Gao, C., Mastio, J., Kossenkov, A., Abrams, S. I., Purandare, A. V., et al. (2018). Inhibition of casein kinase 2 disrupts differentiation of myeloid cells in cancer and enhances the efficacy of immunotherapy in mice. Cancer Res. 78 (19), 5644–5655. doi:10.1158/0008-5472.CAN-18-1229
Hassel, J. C., Jiang, H., Bender, C., Winkler, J., Sevko, A., Shevchenko, I., et al. (2017). Tadalafil has biologic activity in human melanoma. Results of a pilot trial with Tadalafil in patients with metastatic Melanoma (TaMe). Oncoimmunology 6 (9), e1326440. doi:10.1080/2162402X.2017.1326440
Hayes, C. (2021). Cellular immunotherapies for cancer. Ir. J. Med. Sci. 190 (1), 41–57. doi:10.1007/s11845-020-02264-w
He, B., Jabouille, A., Steri, V., Johansson-Percival, A., Michael, I. P., Kotamraju, V. R., et al. (2018). Vascular targeting of LIGHT normalizes blood vessels in primary brain cancer and induces intratumoural high endothelial venules. J. Pathol. 245 (2), 209–221. doi:10.1002/path.5080
He, B., Johansson-Percival, A., Backhouse, J., Li, J., Lee, G. Y. F., Hamzah, J., et al. (2020). Remodeling of metastatic vasculature reduces lung colonization and sensitizes overt metastases to immunotherapy. Cell. Rep. 30 (3), 714–724. e5. doi:10.1016/j.celrep.2019.12.013
He, J., Tsai, L. M., Leong, Y. A., Hu, X., Ma, C. S., Chevalier, N., et al. (2013). Circulating precursor CCR7(lo)PD-1(hi) CXCR5⁺ CD4⁺ T cells indicate Tfh cell activity and promote antibody responses upon antigen reexposure. Immunity 39 (4), 770–781. doi:10.1016/j.immuni.2013.09.007
He, S., and Tang, S. (2020). WNT/β-catenin signaling in the development of liver cancers. Biomed. Pharmacother. = Biomedecine Pharmacother. 132, 110851. doi:10.1016/j.biopha.2020.110851
Heinrich, B., Gertz, E. M., Schäffer, A. A., Craig, A., Ruf, B., Subramanyam, V., et al. (2022). The tumour microenvironment shapes innate lymphoid cells in patients with hepatocellular carcinoma. Gut 71 (6), 1161–1175. doi:10.1136/gutjnl-2021-325288
Hewavisenti, R., Ferguson, A., Wang, K., Jones, D., Gebhardt, T., Edwards, J., et al. (2020). CD103+ tumor-resident CD8+ T cell numbers underlie improved patient survival in oropharyngeal squamous cell carcinoma. J. Immunother. Cancer 8 (1), e000452. doi:10.1136/jitc-2019-000452
Highfill, S. L., Cui, Y., Giles, A. J., Smith, J. P., Zhang, H., Morse, E., et al. (2014). Disruption of CXCR2-mediated MDSC tumor trafficking enhances anti-PD1 efficacy. Sci. Transl. Med. 6 (237), 237ra67. doi:10.1126/scitranslmed.3007974
Hiraoka, N., Ino, Y., Yamazaki-Itoh, R., Kanai, Y., Kosuge, T., and Shimada, K. (2015). Intratumoral tertiary lymphoid organ is a favourable prognosticator in patients with pancreatic cancer. Br. J. Cancer 112 (11), 1782–1790. doi:10.1038/bjc.2015.145
Hobo, W., Maas, F., Adisty, N., de Witte, T., Schaap, N., van der Voort, R., et al. (2010). siRNA silencing of PD-L1 and PD-L2 on dendritic cells augments expansion and function of minor histocompatibility antigen-specific CD8+ T cells. Blood 116 (22), 4501–4511. doi:10.1182/blood-2010-04-278739
Hoffmann, J. C., and Schon, M. P. (2021). Integrin αe(cd103)β7 in epithelial cancer. Cancers 13 (24), 6211. doi:10.3390/cancers13246211
Hollern, D. P., Xu, N., Thennavan, A., Glodowski, C., Garcia-Recio, S., Mott, K. R., et al. (2019). B cells and T follicular helper cells mediate response to checkpoint inhibitors in high mutation burden mouse models of breast cancer. Cell. 179 (5), 1191–1206. e21. doi:10.1016/j.cell.2019.10.028
Holmgaard, R. B., Schaer, D. A., Li, Y., Castaneda, S. P., Murphy, M. Y., Xu, X., et al. (2018). Targeting the TGFβ pathway with galunisertib, a TGFβRI small molecule inhibitor, promotes anti-tumor immunity leading to durable, complete responses, as monotherapy and in combination with checkpoint blockade. J. Immunother. Cancer 6 (1), 47. doi:10.1186/s40425-018-0356-4
Hong, S., Zhang, Z., Liu, H., Tian, M., Zhu, X., Zhang, Z., et al. (2018). B cells are the dominant antigen-presenting cells that activate naive CD4(+) T cells upon immunization with a virus-derived nanoparticle antigen. Immunity 49 (4), 695–708. e4. doi:10.1016/j.immuni.2018.08.012
Hossain, D. M., Pal, S. K., Moreira, D., Duttagupta, P., Zhang, Q., Won, H., et al. (2015). TLR9-Targeted STAT3 silencing abrogates immunosuppressive activity of myeloid-derived suppressor cells from prostate cancer patients. Clin. Cancer Res. 21 (16), 3771–3782. doi:10.1158/1078-0432.CCR-14-3145
Hosseini, S., Nadia, S., Narges, S., Hemmatzadeh, M., Marofi, F., Navid, S., et al. (2020). Progression or suppression: Two sides of the innate lymphoid cells in cancer. J. Cell. Biochem. 121, 2739–2755. doi:10.1002/jcb.29503
Hou, A. J., Chang, Z. L., Lorenzini, M. H., Zah, E., and Chen, Y. Y. (2018). TGF-β-responsive CAR-T cells promote anti-tumor immune function. Bioeng. Transl. Med. 3 (2), 75–86. doi:10.1002/btm2.10097
Hoves, S., Ooi, C. H., Wolter, C., Sade, H., Bissinger, S., Schmittnaegel, M., et al. (2018). Rapid activation of tumor-associated macrophages boosts preexisting tumor immunity. J. Exp. Med. 215 (3), 859–876. doi:10.1084/jem.20171440
Hsieh, C. H., Jian, C. Z., Lin, L. I., Low, G. S., Ou, P. Y., Hsu, C., et al. (2022). Potential role of CXCL13/CXCR5 signaling in immune checkpoint inhibitor treatment in cancer. Cancers 14 (2), 294. doi:10.3390/cancers14020294
Hu, J., Xiao, Q., Dong, M., Guo, D., Wu, X., and Wang, B. (2020). Glioblastoma immunotherapy targeting the innate immune checkpoint CD47-sirpα Axis. Front. Immunol. 11, 593219. doi:10.3389/fimmu.2020.593219
Huang, L., Rong, Y., Tang, X., Yi, K., Qi, P., Hou, J., et al. (2022). Engineered exosomes as an in situ DC-primed vaccine to boost antitumor immunity in breast cancer. Mol. Cancer 21 (1), 45. doi:10.1186/s12943-022-01515-x
Huang, Q., Jacquelot, N., Preaudet, A., Hediyeh-Zadeh, S., Souza-Fonseca-Guimaraes, F., McKenzie, A. N. J., et al. (2021). Type 2 innate lymphoid cells protect against colorectal cancer progression and predict improved patient survival. Cancers 13 (3), 559. doi:10.3390/cancers13030559
Huang, R., Wang, S., Wang, N., Zheng, Y., Zhou, J., Yang, B., et al. (2020). CCL5 derived from tumor-associated macrophages promotes prostate cancer stem cells and metastasis via activating β-catenin/STAT3 signaling. Cell. Death Dis. 11 (4), 234. doi:10.1038/s41419-020-2435-y
Huang, Y., Zhou, L., Zhang, H., Zhang, L., Xi, X., and Sun, Y. (2022). BMDCs induce the generation of the CD103(+)CD8(+) tissue-resident memory T cell subtype, which amplifies local tumor control in the genital tract. Cell. Immunol. 374, 104502. doi:10.1016/j.cellimm.2022.104502
Irshad, S., Flores-Borja, F., Lawler, K., Monypenny, J., Evans, R., Male, V., et al. (2017). RORγt(+) innate lymphoid cells promote lymph node metastasis of breast cancers. Cancer Res. 77 (5), 1083–1096. doi:10.1158/0008-5472.CAN-16-0598
Ishigami, E., Sakakibara, M., Sakakibara, J., Masuda, T., Fujimoto, H., Hayama, S., et al. (2019). Coexistence of regulatory B cells and regulatory T cells in tumor-infiltrating lymphocyte aggregates is a prognostic factor in patients with breast cancer. Breast cancer (Tokyo, Jpn. 26 (2), 180–189. doi:10.1007/s12282-018-0910-4
Jacquelot, N. J., Tellier, J., Nutt, Sl, and Belz, G. T. (2021). Tertiary lymphoid structures and B lymphocytes in cancer prognosis and response to immunotherapies. Oncoimmunology 10 (1), 1900508. doi:10.1080/2162402X.2021.1900508
Jacquelot, N., Seillet, C., É, V., and Gabrielle, B. (2022). Innate lymphoid cells and cancer. Nat. Immunol. 23, 371–379. doi:10.1038/s41590-022-01127-z
Jacquelot, N., Seillet, C., Wang, M., Pizzolla, A., Liao, Y., Hediyeh-Zadeh, S., et al. (2021). Blockade of the co-inhibitory molecule PD-1 unleashes ILC2-dependent antitumor immunity in melanoma. Nat. Immunol. 22 (7), 851–864. doi:10.1038/s41590-021-00943-z
Jaeger, A. M., Stopfer, L., Lee, S., Gaglia, G., Sandel, D., Santagata, S., et al. (2019). Rebalancing protein homeostasis enhances tumor antigen presentation. Clin. Cancer Res. 25 (21), 6392–6405. doi:10.1158/1078-0432.CCR-19-0596
Jaiswal, S., Jamieson, C. H., Pang, W. W., Park, C. Y., Chao, M. P., Majeti, R., et al. (2009). CD47 is upregulated on circulating hematopoietic stem cells and leukemia cells to avoid phagocytosis. Cell. 138 (2), 271–285. doi:10.1016/j.cell.2009.05.046
Jhunjhunwala, S., Hammer, C., and Delamarre, L. (2021). Antigen presentation in cancer: Insights into tumour immunogenicity and immune evasion. Nat. Rev. Cancer 21 (5), 298–312. doi:10.1038/s41568-021-00339-z
Jia, X., Yan, B., Tian, X., Liu, Q., Jin, J., Shi, J., et al. (2021). CD47/SIRPα pathway mediates cancer immune escape and immunotherapy. Int. J. Biol. Sci. 17 (13), 3281–3287. doi:10.7150/ijbs.60782
Jin, K., Yu, Y., Zeng, H., Liu, Z., You, R., Zhang, H., et al. (2022). CD103(+)CD8(+) tissue-resident memory T cell infiltration predicts clinical outcome and adjuvant therapeutic benefit in muscle-invasive bladder cancer. Br. J. Cancer 126 (11), 1581–1588. doi:10.1038/s41416-022-01725-6
Johansson-Percival, A., He, B., Li, Z. J., Kjellén, A., Russell, K., Li, J., et al. (2017). De novo induction of intratumoral lymphoid structures and vessel normalization enhances immunotherapy in resistant tumors. Nat. Immunol. 18 (11), 1207–1217. doi:10.1038/ni.3836
Ju, Schmohl, Felices, M., Oh, F., Lenvik, A. J., Lebeau, A. M., Panyam, J., et al. (2017). Engineering of anti-cd133 trispecific molecule capable of inducing NK expansion and driving antibody-dependent cell-mediated cytotoxicity. Cancer Res. Treat. 49 (4), 1140–1152. doi:10.4143/crt.2016.491
Kamiya, T., Seow, S. V., Wong, D., Robinson, M., and Campana, D. (2019). Blocking expression of inhibitory receptor NKG2A overcomes tumor resistance to NK cells. J. Clin. Investig. 129 (5), 2094–2106. doi:10.1172/JCI123955
Kaneda, M. M., Messer, K. S., Ralainirina, N., Li, H., Leem, C. J., Gorjestani, S., et al. (2017). Corrigendum: PI3Kγ is a molecular switch that controls immune suppression. Nature 542 (7639), 124. doi:10.1038/nature21026
Karagiannis, P., and Kim, S. I. (2021). iPSC-derived natural killer cells for cancer immunotherapy. Mol. Cells 44 (8), 541–548. doi:10.14348/molcells.2021.0078
Khan, M. W., Keshavarzian, A., Gounaris, E., Melson, J. E., Cheon, E. C., Blatner, N. R., et al. (2013). PI3K/AKT signaling is essential for communication between tissue-infiltrating mast cells, macrophages, and epithelial cells in colitis-induced cancer. Clin. Cancer Res. 19 (9), 2342–2354. doi:10.1158/1078-0432.CCR-12-2623
Kim, C. H., Rott, L. S., Clark-Lewis, I., Campbell, D. J., Wu, L., and Butcher, E. C. (2001). Subspecialization of CXCR5+ T cells: B helper activity is focused in a germinal center-localized subset of CXCR5+ T cells. J. Exp. Med. 193 (12), 1373–1381. doi:10.1084/jem.193.12.1373
Kim, H. P., Imbert, J., and Leonard, W. J. (2006). Both integrated and differential regulation of components of the IL-2/IL-2 receptor system. Cytokine Growth Factor Rev. 17 (5), 349–366. doi:10.1016/j.cytogfr.2006.07.003
Kim, J., Kim, W., Moon, U. J., Kim, H. J., Choi, H. J., Sin, J. I., et al. (1950). Intratumorally establishing type 2 innate lymphoid cells blocks tumor growth. J. Immunol. 196 (5), 2410–2423. doi:10.4049/jimmunol.1501730
Kim, J., Ryu, S., and Kim, H. Y. (2021). Innate lymphoid cells in tissue homeostasis and disease pathogenesis. Mol. Cells 44 (5), 301–309. doi:10.14348/molcells.2021.0053
Kim, J. H., Kim, B. S., and Lee, S. K. (2020). Regulatory T cells in tumor microenvironment and approach for anticancer immunotherapy. Immune Netw. 20 (1), e4. doi:10.4110/in.2020.20.e4
Kinker, G. S., Vitiello, G. A. F., Ferreira, W. A. S., Chaves, A. S., Cordeiro de Lima, V. C., and Medina, T. D. S. (2021). B cell orchestration of anti-tumor immune responses: A matter of cell localization and communication. Front. Cell. Dev. Biol. 9, 678127. doi:10.3389/fcell.2021.678127
Kirchberger, S., Royston, D. J., Boulard, O., Thornton, E., Franchini, F., Szabady, R. L., et al. (2013). Innate lymphoid cells sustain colon cancer through production of interleukin-22 in a mouse model. J. Exp. Med. 210 (5), 917–931. doi:10.1084/jem.20122308
Klichinsky, M., Ruella, M., Shestova, O., Lu, X. M., Best, A., Zeeman, M., et al. (2020). Human chimeric antigen receptor macrophages for cancer immunotherapy. Nat. Biotechnol. 38 (8), 947–953. doi:10.1038/s41587-020-0462-y
Koh, J., Kim, H. Y., Lee, Y., Park, I. K., Kang, C. H., Kim, Y. T., et al. (2019). IL23-Producing human lung cancer cells promote tumor growth via conversion of innate lymphoid cell 1 (ILC1) into ILC3. Clin. Cancer Res. 25 (13), 4026–4037. doi:10.1158/1078-0432.CCR-18-3458
Komdeur, F. L., Prins, T. M., van de Wall, S., Plat, A., Wisman, G. B. A., Hollema, H., et al. (2017). CD103+ tumor-infiltrating lymphocytes are tumor-reactive intraepithelial CD8+ T cells associated with prognostic benefit and therapy response in cervical cancer. Oncoimmunology 6 (9), e1338230. doi:10.1080/2162402X.2017.1338230
Konjar, Š., Ficht, X., Iannacone, M., and Veldhoen, M. (2022). Heterogeneity of tissue resident memory T cells. Immunol. Lett. 245, 1–7. doi:10.1016/j.imlet.2022.02.009
Korbecki, J., Kojder, K., Simińska, D., Bohatyrewicz, R., Gutowska, I., Chlubek, D., et al. (2020). CC chemokines in a tumor: A review of pro-cancer and anti-cancer properties of the ligands of receptors CCR1, CCR2, CCR3, and CCR4. Int. J. Mol. Sci. 21 (21), E8412. doi:10.3390/ijms21218412
Kroeger, D. R., Milne, K., and Nelson, B. H. (2016). Tumor-infiltrating plasma cells are associated with tertiary lymphoid structures, cytolytic T-cell responses, and superior prognosis in ovarian cancer. Clin. Cancer Res. 22 (12), 3005–3015. doi:10.1158/1078-0432.CCR-15-2762
Kruse, P. H., Matta, J., Ugolini, S., and Vivier, E. (2014). Natural cytotoxicity receptors and their ligands. Immunol. Cell. Biol. 92 (3), 221–229. doi:10.1038/icb.2013.98
Kumari, N., and Choi, S. H. (2022). Tumor-associated macrophages in cancer: Recent advancements in cancer nanoimmunotherapies. J. Exp. Clin. Cancer Res. 41 (1), 68. doi:10.1186/s13046-022-02272-x
Kurata, I., Matsumoto, I., and Sumida, T. (2021). T follicular helper cell subsets: A potential key player in autoimmunity. Immunol. Med. 44 (1), 1–9. doi:10.1080/25785826.2020.1776079
Kvedaraite, E., and Ginhoux, F. (2022). Human dendritic cells in cancer. Sci. Immunol. 7 (70), eabm9409. doi:10.1126/sciimmunol.abm9409
Ladányi, A., Sebestyén, T., Mohos, A., Liszkay, G., Somlai, B., Tóth, E., et al. (2014). Ectopic lymphoid structures in primary cutaneous melanoma. Pathol. Oncol. Res. 20 (4), 981–985. doi:10.1007/s12253-014-9784-8
Lamplugh, Z., and Fan, Y. (2021). Vascular microenvironment, tumor immunity and immunotherapy. Front. Immunol. 12, 811485. doi:10.3389/fimmu.2021.811485
Lanier, L. L. (2015). NKG2D receptor and its ligands in host defense. Cancer Immunol. Res. 3 (6), 575–582. doi:10.1158/2326-6066.CIR-15-0098
Lauss, M., Donia, M., Svane, I. M., and Jönsson, G. (2021). B cells and tertiary lymphoid structures: Friends or foes in cancer immunotherapy? Clinical cancer research. Journal of the American Association for Cancer Research.
Le Floc'h, A., Jalil, A., Vergnon, I., Le Maux Chansac, B., Lazar, V., Bismuth, G., et al. (2007). Alpha E beta 7 integrin interaction with E-cadherin promotes antitumor CTL activity by triggering lytic granule polarization and exocytosis. J. Exp. Med. 204 (3), 559–570. doi:10.1084/jem.20061524
Lee, M., Heo, S. H., Song, I. H., Rajayi, H., Park, H. S., Park, I. A., et al. (2019). Presence of tertiary lymphoid structures determines the level of tumor-infiltrating lymphocytes in primary breast cancer and metastasis. Mod. Pathol. 32 (1), 70–80. doi:10.1038/s41379-018-0113-8
Lehmann-Horn, K., Wang, S. Z., Sagan, S. A., Zamvil, S. S., and von Büdingen, H. C. (2016). B cell repertoire expansion occurs in meningeal ectopic lymphoid tissue. JCI insight 1 (20), e87234. doi:10.1172/jci.insight.87234
Li, B. H., Garstka, M. A., and Li, Z. F. (2020). Chemokines and their receptors promoting the recruitment of myeloid-derived suppressor cells into the tumor. Mol. Immunol. 117, 201–215. doi:10.1016/j.molimm.2019.11.014
Li, Q., Liu, X., Wang, D., Wang, Y., Lu, H., Wen, S., et al. (2020). Prognostic value of tertiary lymphoid structure and tumour infiltrating lymphocytes in oral squamous cell carcinoma. Int. J. Oral Sci. 12 (1), 24. doi:10.1038/s41368-020-00092-3
Li, X., Su, X., Liu, R., Pan, Y., Fang, J., Cao, L., et al. (2021). HDAC inhibition potentiates anti-tumor activity of macrophages and enhances anti-PD-L1-mediated tumor suppression. Oncogene 40 (10), 1836–1850. doi:10.1038/s41388-020-01636-x
Li, X., Yao, W., Yuan, Y., Chen, P., Li, B., Li, J., et al. (2017). Targeting of tumour-infiltrating macrophages via CCL2/CCR2 signalling as a therapeutic strategy against hepatocellular carcinoma. Gut 66 (1), 157–167. doi:10.1136/gutjnl-2015-310514
Li, Y., Hermanson, D. L., Moriarity, B. S., and Kaufman, D. S. (2018). Human iPSC-derived natural killer cells engineered with chimeric antigen receptors enhance anti-tumor activity. Cell. stem Cell. 23 (2), 181–192. e5. doi:10.1016/j.stem.2018.06.002
Li, Y., Wongsiriroj, N., and Blaner, W. S. (2014). The multifaceted nature of retinoid transport and metabolism. Hepatobiliary Surg. Nutr. 3 (3), 126–139. doi:10.3978/j.issn.2304-3881.2014.05.04
Liang, Y., Wang, W., Zhu, X., Yu, M., and Zhou, C. (2022). Inhibition of myeloid-derived suppressive cell function with all-trans retinoic acid enhanced anti-PD-L1 efficacy in cervical cancer. Sci. Rep. 12 (1), 9619. doi:10.1038/s41598-022-13855-1
Lim, W. A., and June, C. H. (2017). The principles of engineering immune cells to treat cancer. Cell. 168 (4), 724–740. doi:10.1016/j.cell.2017.01.016
Lin, X., Ye, L., Wang, X., Liao, Z., Dong, J., Yang, Y., et al. (2021). Follicular helper T cells remodel the immune microenvironment of pancreatic cancer via secreting CXCL13 and IL-21. Cancers 13 (15), 3678. doi:10.3390/cancers13153678
Linterman, M. A., Beaton, L., Yu, D., Ramiscal, R. R., Srivastava, M., Hogan, J. J., et al. (2010). IL-21 acts directly on B cells to regulate Bcl-6 expression and germinal center responses. J. Exp. Med. 207 (2), 353–363. doi:10.1084/jem.20091738
Liu, D., Xu, H., Shih, C., Wan, Z., Ma, X., Ma, W., et al. (2015). T-B-cell entanglement and ICOSL-driven feed-forward regulation of germinal centre reaction. Nature 517 (7533), 214–218. doi:10.1038/nature13803
Liu, S., Galat, V., Galat, Y., Lee, Y. K. A., Wainwright, D., and Wu, J. (2021). NK cell-based cancer immunotherapy: From basic biology to clinical development. J. Hematol. Oncol. 14 (1), 7. doi:10.1186/s13045-020-01014-w
Liu, T., Yang, F., Xie, J., Chen, J., Gao, W., Bai, X., et al. (2021). All-trans-retinoic acid restores CD4+ T cell response after sepsis by inhibiting the expansion and activation of myeloid-derived suppressor cells. Mol. Immunol. 136, 8–15. doi:10.1016/j.molimm.2021.04.025
Liu, X., Tsang, J. Y. S., Hlaing, T., Hu, J., Ni, Y. B., Chan, S. K., et al. (2017). Distinct tertiary lymphoid structure associations and their prognostic relevance in HER2 positive and negative breast cancers. Oncologist 22 (11), 1316–1324. doi:10.1634/theoncologist.2017-0029
Lu, Y. C., Yao, X., Crystal, J. S., Li, Y. F., El-Gamil, M., Gross, C., et al. (2014). Efficient identification of mutated cancer antigens recognized by T cells associated with durable tumor regressions. Clin. Cancer Res. 20 (13), 3401–3410. doi:10.1158/1078-0432.CCR-14-0433
Lucas, B., and Girard, J. (2021). High endothelial venules (HEVs) in immunity, inflammation and cancer. Angiogenesis 24, 719–753. doi:10.1007/s10456-021-09792-8
Luckheeram, R. V., Zhou, R., Verma, A. D., and Xia, B. (2012). CD4⁺T cells: Differentiation and functions. Clin. Dev. Immunol. 2012, 925135. doi:10.1155/2012/925135
Luke, J. J., Zha, Y., Matijevich, K., and Gajewski, T. F. (2016). Single dose denileukin diftitox does not enhance vaccine-induced T cell responses or effectively deplete Tregs in advanced melanoma: Immune monitoring and clinical results of a randomized phase II trial. J. Immunother. Cancer 4, 35. doi:10.1186/s40425-016-0140-2
Lüthje, K., Kallies, A., Shimohakamada, Y., Belz, G. T., Light, A., Tarlinton, D. M., et al. (2012). The development and fate of follicular helper T cells defined by an IL-21 reporter mouse. Nat. Immunol. 13 (5), 491–498. doi:10.1038/ni.2261
Lutz, E. R., Wu, A. A., Bigelow, E., Sharma, R., Mo, G., Soares, K., et al. (2014). Immunotherapy converts nonimmunogenic pancreatic tumors into immunogenic foci of immune regulation. Cancer Immunol. Res. 2 (7), 616–631. doi:10.1158/2326-6066.CIR-14-0027
Ma, H., Gao, W., Sun, X., and Wang, W. (2018). STAT5 and TET2 cooperate to regulate FOXP3-TSDR demethylation in CD4(+) T cells of patients with colorectal cancer. J. Immunol. Res. 2018, 6985031. doi:10.1155/2018/6985031
Ma, Y., Adjemian, S., Mattarollo, S. R., Yamazaki, T., Aymeric, L., Yang, H., et al. (2013). Anticancer chemotherapy-induced intratumoral recruitment and differentiation of antigen-presenting cells. Immunity 38 (4), 729–741. doi:10.1016/j.immuni.2013.03.003
Mackay, L. K., Braun, A., Macleod, B. L., Collins, N., Tebartz, C., Bedoui, S., et al. (1950)., 194. Baltimore, Md, 2059–2063. doi:10.4049/jimmunol.1402256Cutting edge: CD69 interference with sphingosine-1-phosphate receptor function regulates peripheral T cell retentionJ. Immunol.5
Mackay, L. K., Rahimpour, A., Ma, J. Z., Collins, N., Stock, A. T., Hafon, M. L., et al. (2013). The developmental pathway for CD103(+)CD8+ tissue-resident memory T cells of skin. Nat. Immunol. 14 (12), 1294–1301. doi:10.1038/ni.2744
Maggi, E., Veneziani, I., Moretta, L., Cosmi, L., and Annunziato, F. (2020). Group 2 innate lymphoid cells: A double-edged sword in cancer? Cancers 12, E3452. doi:10.3390/cancers12113452
Mahnke, K., Knop, J., and Enk, A. H. (2003). Induction of tolerogenic DCs: 'you are what you eat. Trends Immunol. 24 (12), 646–651. doi:10.1016/j.it.2003.09.012
Maldonado, L., Teague, J. E., Morrow, M. P., Jotova, I., Wu, T. C., Wang, C., et al. (2014). Intramuscular therapeutic vaccination targeting HPV16 induces T cell responses that localize in mucosal lesions. Sci. Transl. Med. 6 (221), 221ra13. doi:10.1126/scitranslmed.3007323
Mamessier, E., Sylvain, A., Thibult, M. L., Houvenaeghel, G., Jacquemier, J., Castellano, R., et al. (2011). Human breast cancer cells enhance self tolerance by promoting evasion from NK cell antitumor immunity. J. Clin. Investig. 121 (9), 3609–3622. doi:10.1172/JCI45816
Man, S. M. (2018). Inflammasomes in the gastrointestinal tract: Infection, cancer and gut microbiota homeostasis. Nat. Rev. Gastroenterol. Hepatol. 15 (12), 721–737. doi:10.1038/s41575-018-0054-1
Mao, K., Baptista, A. P., Tamoutounour, S., Zhuang, L., Bouladoux, N., Martins, A. J., et al. (2018). Innate and adaptive lymphocytes sequentially shape the gut microbiota and lipid metabolism. Nature 554 (7691), 255–259. doi:10.1038/nature25437
Marabondo, S., and Kaufman, H. L. (2017). High-dose interleukin-2 (IL-2) for the treatment of melanoma: Safety considerations and future directions. Expert Opin. Drug Saf. 16 (12), 1347–1357. doi:10.1080/14740338.2017.1382472
Marceaux, C., Weeden, C. E., Gordon, C. L., and Asselin-Labat, M. L. (2021). Holding our breath: The promise of tissue-resident memory T cells in lung cancer. Transl. Lung Cancer Res. 10 (6), 2819–2829. doi:10.21037/tlcr-20-819
Martinet, L., Garrido, I., and Girard, J. P. (2012). Tumor high endothelial venules (HEVs) predict lymphocyte infiltration and favorable prognosis in breast cancer. Oncoimmunology 1 (5), 789–790. doi:10.4161/onci.19787
Martinez, M., and Moon, E. K. (2019). CAR T cells for solid tumors: New strategies for finding, infiltrating, and surviving in the tumor microenvironment. Front. Immunol. 10, 128. doi:10.3389/fimmu.2019.00128
Masopust, D., Choo, D., Vezys, V., Wherry, E. J., Duraiswamy, J., Akondy, R., et al. (2010). Dynamic T cell migration program provides resident memory within intestinal epithelium. J. Exp. Med. 207 (3), 553–564. doi:10.1084/jem.20090858
Masopust, D., Vezys, V., Marzo, A. L., and Lefrançois, L. (2001). Preferential localization of effector memory cells in nonlymphoid tissue. Sci. (New York, NY) 291 (5512), 2413–2417. doi:10.1126/science.1058867
Matsuo, K., Kitahata, K., Kawabata, F., Kamei, M., Hara, Y., Takamura, S., et al. (2018). A highly active form of XCL1/lymphotactin functions as an effective adjuvant to recruit cross-presenting dendritic cells for induction of effector and memory CD8(+) T cells. Front. Immunol. 9, 2775. doi:10.3389/fimmu.2018.02775
Mazzone, M., Menga, A., and Castegna, A. (2018). Metabolism and TAM functions-it takes two to tango. FEBS J. 285 (4), 700–716. doi:10.1111/febs.14295
McCarron, M. J., and Marie, J. C. (2014). TGF-β prevents T follicular helper cell accumulation and B cell autoreactivity. J. Clin. Investig. 124 (10), 4375–4386. doi:10.1172/JCI76179
Mehdizadeh, S., Bayatipoor, H., Pashangzadeh, S., Jafarpour, R., Shojaei, Z., and Motallebnezhad, M. (2021). Immune checkpoints and cancer development: Therapeutic implications and future directions. Pathol. Res. Pract. 223, 153485. doi:10.1016/j.prp.2021.153485
Meininger, I., Carrasco, A., Rao, A., Soini, T., Kokkinou, E., and Mjösberg, J. (2020). Tissue-specific features of innate lymphoid cells. Trends Immunol. 41 (10), 902–917. doi:10.1016/j.it.2020.08.009
Meng, Q., Ying, J., and Pan, L. (2021). Tertiary lymphoid structure and B-cell-related pathways: A potential target in tumor immunotherapy. Oncol. Lett. 22.
Messaoudene, M., Fregni, G., Enot, D., Jacquelot, N., Neves, E., Germaud, N., et al. (2016). NKp30 isoforms and NKp46 transcripts in metastatic melanoma patients: Unique NKp30 pattern in rare melanoma patients with favorable evolution. Oncoimmunology 5 (12), e1154251. doi:10.1080/2162402X.2016.1154251
Messmer, M. N., Netherby, C. S., Banik, D., and Abrams, S. I. (2015). Tumor-induced myeloid dysfunction and its implications for cancer immunotherapy. Cancer Immunol. Immunother. 64 (1), 1–13. doi:10.1007/s00262-014-1639-3
Meylan, M., Petitprez, F., Lacroix, L., Di Tommaso, L., Roncalli, M., Bougoüin, A., et al. (2020). Early hepatic lesions display immature tertiary lymphoid structures and show elevated expression of immune inhibitory and immunosuppressive molecules. Clin. Cancer Res. 26 (16), 4381–4389. doi:10.1158/1078-0432.CCR-19-2929
Mikucki, M. E., Fisher, D. T., Matsuzaki, J., Skitzki, J. J., Gaulin, N. B., Muhitch, J. B., et al. (2015). Non-redundant requirement for CXCR3 signalling during tumoricidal T-cell trafficking across tumour vascular checkpoints. Nat. Commun. 6, 7458. doi:10.1038/ncomms8458
Mittag, D., Proietto, A. I., Loudovaris, T., Mannering, S. I., Vremec, D., Shortman, K., et al. (1950). Human dendritic cell subsets from spleen and blood are similar in phenotype and function but modified by donor health status. J. Immunol. 186 (11), 6207–6217. doi:10.4049/jimmunol.1002632
Miyara, M., Yoshioka, Y., Kitoh, A., Shima, T., Wing, K., Niwa, A., et al. (2009). Functional delineation and differentiation dynamics of human CD4+ T cells expressing the FoxP3 transcription factor. Immunity 30 (6), 899–911. doi:10.1016/j.immuni.2009.03.019
Moeini, P., and Niedźwiedzka-Rystwej, P. (2021). Tumor-associated macrophages: Combination of therapies, the approach to improve cancer treatment. Int. J. Mol. Sci. 22 (13), 7239. doi:10.3390/ijms22137239
Mondini, M., Nizard, M., Tran, T., Mauge, L., Loi, M., Clémenson, C., et al. (2015). Synergy of radiotherapy and a cancer vaccine for the treatment of HPV-associated head and neck cancer. Mol. Cancer Ther. 14 (6), 1336–1345. doi:10.1158/1535-7163.MCT-14-1015
Montfort, A., Pearce, O., Maniati, E., Vincent, B. G., Bixby, L., Böhm, S., et al. (2017). A strong B-cell response is part of the immune landscape in human high-grade serous ovarian metastases. Clin. Cancer Res. 23 (1), 250–262. doi:10.1158/1078-0432.CCR-16-0081
Moral, J. A., Leung, J., Rojas, L. A., Ruan, J., Zhao, J., Sethna, Z., et al. (2020). ILC2s amplify PD-1 blockade by activating tissue-specific cancer immunity. Nature 579 (7797), 130–135. doi:10.1038/s41586-020-2015-4
Moran, Y., Jiaqi, L., Guodong, Z., Yiying, W., Mengdi, H., Qing, X., et al. (2021). CXCL13 shapes immunoactive tumor microenvironment and enhances the efficacy of PD-1 checkpoint blockade in high-grade serous ovarian cancer. J. Immunother. Cancer 9, e001136. doi:10.1136/jitc-2020-001136
Morrissey, M. A., Williamson, A. P., Steinbach, A. M., Roberts, E. W., Kern, N., Headley, M. B., et al. (2018). Chimeric antigen receptors that trigger phagocytosis. eLife 7, e36688. doi:10.7554/eLife.36688
Mortha, A., Chudnovskiy, A., Hashimoto, D., Bogunovic, M., Spencer, S. P., Belkaid, Y., et al. (2014). Microbiota-dependent crosstalk between macrophages and ILC3 promotes intestinal homeostasis. Sci. (New York, NY) 343 (6178), 1249288. doi:10.1126/science.1249288
Morvan, M. G., and Lanier, L. L. (2016). NK cells and cancer: You can teach innate cells new tricks. Nat. Rev. Cancer 16 (1), 7–19. doi:10.1038/nrc.2015.5
Munari, E., Mariotti, F. R., Quatrini, L., Bertoglio, P., Tumino, N., Vacca, P., et al. (2021). PD-1/PD-L1 in cancer: Pathophysiological, diagnostic and therapeutic aspects. Int. J. Mol. Sci. 22 (10), 5123. doi:10.3390/ijms22105123
Munneke, J. M., Björklund, A. T., Mjösberg, J. M., Garming-Legert, K., Bernink, J. H., Blom, B., et al. (2014). Activated innate lymphoid cells are associated with a reduced susceptibility to graft-versus-host disease. Blood 124 (5), 812–821. doi:10.1182/blood-2013-11-536888
Muraoka, D., Seo, N., Hayashi, T., Tahara, Y., Fujii, K., Tawara, I., et al. (2019). Antigen delivery targeted to tumor-associated macrophages overcomes tumor immune resistance. J. Clin. Investig. 129 (3), 1278–1294. doi:10.1172/JCI97642
Murray, T., Fuertes Marraco, S. A., Baumgaertner, P., Bordry, N., Cagnon, L., Donda, A., et al. (2016). Very late antigen-1 marks functional tumor-resident CD8 T cells and correlates with survival of melanoma patients. Front. Immunol. 7, 573. doi:10.3389/fimmu.2016.00573
Muthuswamy, R., McGray, A. J. R., Battaglia, S., He, W., Miliotto, A., Eppolito, C., et al. (2021). CXCR6 governs the retention of tissue-resident memory T cells to promote enhanced surveillance and control of ovarian cancer. bioRxiv.
Muthuswamy, R., McGray, A. R., Battaglia, S., He, W., Miliotto, A., Eppolito, C., et al. (2021). CXCR6 by increasing retention of memory CD8(+) T cells in the ovarian tumor microenvironment promotes immunosurveillance and control of ovarian cancer. J. Immunother. Cancer 9 (10), e003329. doi:10.1136/jitc-2021-003329
Myers, J. A., and Miller, J. S. (2021). Exploring the NK cell platform for cancer immunotherapy. Nat. Rev. Clin. Oncol. 18 (2), 85–100. doi:10.1038/s41571-020-0426-7
Nagai, Y., Lam, L., Greene, M. I., and Zhang, H. T. (2019). FOXP3 and its cofactors as targets of immunotherapies. Engineering 5 (1), 115–121. doi:10.1016/j.eng.2019.01.001
Nagarsheth, N., Wicha, M. S., and Zou, W. (2017). Chemokines in the cancer microenvironment and their relevance in cancer immunotherapy. Nat. Rev. Immunol. 17 (9), 559–572. doi:10.1038/nri.2017.49
Nath, A. P., Braun, A., Ritchie, S. C., Carbone, F. R., Mackay, L. K., Gebhardt, T., et al. (2019). Comparative analysis reveals a role for TGF-β in shaping the residency-related transcriptional signature in tissue-resident memory CD8+ T cells. PloS one 14 (2), e0210495. doi:10.1371/journal.pone.0210495
Ng, Y. Y., Tay, J. C. K., and Wang, S. (2020). CXCR1 expression to improve anti-cancer efficacy of intravenously injected CAR-NK cells in mice with peritoneal xenografts. Mol. Ther. Oncolytics 16, 75–85. doi:10.1016/j.omto.2019.12.006
Nguyen, R., Moustaki, A., Norrie, J. L., Brown, S., Akers, W. J., Shirinifard, A., et al. (2019). Interleukin-15 enhances anti-GD2 antibody-mediated cytotoxicity in an orthotopic PDX model of neuroblastoma. Clin. Cancer Res. 25 (24), 7554–7564. doi:10.1158/1078-0432.CCR-19-1045
Niogret, J., Berger, H., Rebe, C., Mary, R., Ballot, E., Truntzer, C., et al. (2021). Follicular helper-T cells restore CD8(+)-dependent antitumor immunity and anti-PD-L1/PD-1 efficacy. J. Immunother. Cancer 9 (6), e002157. doi:10.1136/jitc-2020-002157
Nishikawa, H., and Koyama, S. (2021). Mechanisms of regulatory T cell infiltration in tumors: Implications for innovative immune precision therapies. J. Immunother. Cancer 9 (7), e002591. doi:10.1136/jitc-2021-002591
Nizard, M., Roussel, H., Diniz, M. O., Karaki, S., Tran, T., Voron, T., et al. (2017). Induction of resident memory T cells enhances the efficacy of cancer vaccine. Nat. Commun. 8, 15221. doi:10.1038/ncomms15221
Noël, G., Fontsa, M. L., Garaud, S., Silva, P. D., Wind, Ad, Eynden, G. G. Vd, et al. (2021). Functional Th1-oriented T follicular helper cells that infiltrate human breast cancer promote effective adaptive immunity. J. Clin. Investig. 131, e139905. doi:10.1172/JCI139905
Nurieva, R. I., Chung, Y., Hwang, D., Yang, X. O., Kang, H. S., Ma, L., et al. (2008). Generation of T follicular helper cells is mediated by interleukin-21 but independent of T helper 1, 2, or 17 cell lineages. Immunity 29 (1), 138–149. doi:10.1016/j.immuni.2008.05.009
Nurieva, R. I., Chung, Y., Martinez, G. J., Yang, X. O., Tanaka, S., Matskevitch, T. D., et al. (2009). Bcl6 mediates the development of T follicular helper cells. Sci. (New York, NY) 325 (5943), 1001–1005. doi:10.1126/science.1176676
Nussbaum, K., Burkhard, S. H., Ohs, I., Mair, F., Klose, C. S. N., Arnold, S. J., et al. (2017). Tissue microenvironment dictates the fate and tumor-suppressive function of type 3 ILCs. J. Exp. Med. 214 (8), 2331–2347. doi:10.1084/jem.20162031
O'Keeffe, M., Mok, W. H., and Radford, K. J. (2015). Human dendritic cell subsets and function in health and disease. Cell. Mol. Life Sci. 72 (22), 4309–4325. doi:10.1007/s00018-015-2005-0
Obermajer, N., Wong, J. L., Edwards, R. P., Odunsi, K., Moysich, K., and Kalinski, P. (2012). PGE(2)-driven induction and maintenance of cancer-associated myeloid-derived suppressor cells. Immunol. Investig. 41 (6-7), 635–657. doi:10.3109/08820139.2012.695417
Okla, K., Farber, D. L., and Zou, W. (2021). Tissue-resident memory T cells in tumor immunity and immunotherapy. J. Exp. Med. 218 (4), e20201605. doi:10.1084/jem.20201605
Orillion, A., Hashimoto, A., Damayanti, N., Shen, L., Adelaiye-Ogala, R., Arisa, S., et al. (2017). Entinostat neutralizes myeloid-derived suppressor cells and enhances the antitumor effect of PD-1 inhibition in murine models of lung and renal cell carcinoma. Clin. Cancer Res. 23 (17), 5187–5201. doi:10.1158/1078-0432.CCR-17-0741
Ortega, R. A., Barham, W., Sharman, K., Tikhomirov, O., Giorgio, T. D., and Yull, F. E. (2016). Manipulating the NF-κB pathway in macrophages using mannosylated, siRNA-delivering nanoparticles can induce immunostimulatory and tumor cytotoxic functions. Int. J. Nanomedicine 11, 2163–2177. doi:10.2147/IJN.S93483
Ostrand-Rosenberg, S., and Fenselau, C. (1950). Myeloid-derived suppressor cells: Immune-suppressive cells that impair antitumor immunity and are sculpted by their environment. J. Immunol. 200. Baltimore, Md, 422–431. doi:10.4049/jimmunol.1701019
Page, A., Hubert, J., Fusil, F., and Cosset, F. L. (2021). Exploiting B cell transfer for cancer therapy: Engineered B cells to eradicate tumors. Int. J. Mol. Sci. 22 (18), 9991. doi:10.3390/ijms22189991
Parihar, R., Rivas, C., Huynh, M., Omer, B., Lapteva, N., Metelitsa, L. S., et al. (2019). NK cells expressing a chimeric activating receptor eliminate MDSCs and rescue impaired CAR-T cell activity against solid tumors. Cancer Immunol. Res. 7 (3), 363–375. doi:10.1158/2326-6066.CIR-18-0572
Pende, D., and Meazza, R. (2022). N-803: A double-edged sword in haplo-NK therapy. Blood 139 (8), 1122–1124. doi:10.1182/blood.2021014789
Peng, L., Sun, W., Wei, F., Chen, L., and Wen, W. (2021). Interleukin-33 modulates immune responses in cutaneous melanoma in a context-specific way. Aging 13 (5), 6740–6751. doi:10.18632/aging.202531
Peng, Y., Dong, S., Song, Y., Hou, D., Wang, L., Li, B., et al. (2021). Key sunitinib-related biomarkers for renal cell carcinoma. Cancer Med. 10 (19), 6917–6930. doi:10.1002/cam4.4206
Penny, H. A., Hodge, S. H., and Hepworth, M. R. (2018). Orchestration of intestinal homeostasis and tolerance by group 3 innate lymphoid cells. Semin. Immunopathol. 40 (4), 357–370. doi:10.1007/s00281-018-0687-8
Petitprez, F., de Reyniès, A., Keung, E. Z., Chen, T. W., Sun, C. M., Calderaro, J., et al. (2020). B cells are associated with survival and immunotherapy response in sarcoma. Nature 577 (7791), 556–560. doi:10.1038/s41586-019-1906-8
Petty, A. J., Li, A., Wang, X., Dai, R., Heyman, B., Hsu, D., et al. (2019). Hedgehog signaling promotes tumor-associated macrophage polarization to suppress intratumoral CD8+ T cell recruitment. J. Clin. Investig. 129 (12), 5151–5162. doi:10.1172/JCI128644
Philip, M., and Schietinger, A. (2022). CD8(+) T cell differentiation and dysfunction in cancer. Nat. Rev. Immunol. 22 (4), 209–223. doi:10.1038/s41577-021-00574-3
Platonova, S., Cherfils-Vicini, J., Damotte, D., Crozet, L., Vieillard, V., Validire, P., et al. (2011). Profound coordinated alterations of intratumoral NK cell phenotype and function in lung carcinoma. Cancer Res. 71 (16), 5412–5422. doi:10.1158/0008-5472.CAN-10-4179
Posch, F., Silina, K., Leibl, S., Mündlein, A., Moch, H., Siebenhüner, A., et al. (2018). Maturation of tertiary lymphoid structures and recurrence of stage II and III colorectal cancer. Oncoimmunology 7 (2), e1378844. doi:10.1080/2162402X.2017.1378844
Prager, I., Liesche, C., van Ooijen, H., Urlaub, D., Verron, Q., Sandström, N., et al. (2019). NK cells switch from granzyme B to death receptor-mediated cytotoxicity during serial killing. J. Exp. Med. 216 (9), 2113–2127. doi:10.1084/jem.20181454
Punt, S., Fleuren, G. J., Kritikou, E., Lubberts, E., Trimbos, J. B., Jordanova, E. S., et al. (2015). Angels and demons: Th17 cells represent a beneficial response, while neutrophil IL-17 is associated with poor prognosis in squamous cervical cancer. Oncoimmunology 4 (1), e984539. doi:10.4161/2162402X.2014.984539
Purwar, R., Campbell, J., Murphy, G., Richards, W. G., Clark, R. A., and Kupper, T. S. (2011). Resident memory T cells (T(RM)) are abundant in human lung: Diversity, function, and antigen specificity. PloS one 6 (1), e16245. doi:10.1371/journal.pone.0016245
Qi, J., Crinier, A., Escalière, B., Ye, Y., Wang, Z., Zhang, T., et al. (2021). Single-cell transcriptomic landscape reveals tumor specific innate lymphoid cells associated with colorectal cancer progression. Cell. Rep. Med. 2 (8), 100353. doi:10.1016/j.xcrm.2021.100353
Qin, H., Lerman, B., Sakamaki, I., Wei, G., Cha, S. C., Rao, S. S., et al. (2014). Generation of a new therapeutic peptide that depletes myeloid-derived suppressor cells in tumor-bearing mice. Nat. Med. 20 (6), 676–681. doi:10.1038/nm.3560
Qiu, Z., Chu, T. H., and Sheridan, B. S. (2021). TGF-β: Many paths to CD103(+) CD8 T cell residency. Cells 10 (5), 989. doi:10.3390/cells10050989
Qiu, Z., Chu, T. H., and Sheridan, B. S. (2021). TGF-β: Many paths to CD103+ CD8 T cell residency. Cells 10 (5), 989. doi:10.3390/cells10050989
Qu, G., Chen, J., Li, Y., Yuan, Y., Liang, R., and Li, B. (2022). Current status and perspectives of regulatory T cell-based therapy. J. Genet. genomics = Yi chuan xue bao 49 (7), 599–611. doi:10.1016/j.jgg.2022.05.005
Quintarelli, C., Sivori, S., Caruso, S., Carlomagno, S., Falco, M., Boffa, I., et al. (2020). Efficacy of third-party chimeric antigen receptor modified peripheral blood natural killer cells for adoptive cell therapy of B-cell precursor acute lymphoblastic leukemia. Leukemia 34 (4), 1102–1115. doi:10.1038/s41375-019-0613-7
Ramaswamy, M., Kim, T., Jones, D. C., Ghadially, H., Mahmoud, T. I., Garcia, A., et al. (2022). Immunomodulation of T- and NK-cell responses by a bispecific antibody targeting CD28 homolog and PD-L1. Cancer Immunol. Res. 10 (2), 200–214. doi:10.1158/2326-6066.CIR-21-0218
Ramesh, A., Kumar, S., Nandi, D., and Kulkarni, A. (2019). CSF1R- and SHP2-inhibitor-loaded nanoparticles enhance cytotoxic activity and phagocytosis in tumor-associated macrophages. Adv. Mat. 31 (51), e1904364. doi:10.1002/adma.201904364
Rasmussen, R. K., and Etzerodt, A. (2021). Therapeutic targeting of tumor-associated macrophages. Adv. Pharmacol. 91, 185–211. doi:10.1016/bs.apha.2021.03.002
Raulet, D. H., Gasser, S., Gowen, B. G., Deng, W., and Jung, H. (2013). Regulation of ligands for the NKG2D activating receptor. Annu. Rev. Immunol. 31, 413–441. doi:10.1146/annurev-immunol-032712-095951
Ravi, R., Noonan, K. A., Pham, V., Bedi, R., Zhavoronkov, A., Ozerov, I. V., et al. (2018). Bifunctional immune checkpoint-targeted antibody-ligand traps that simultaneously disable TGFβ enhance the efficacy of cancer immunotherapy. Nat. Commun. 9 (1), 741. doi:10.1038/s41467-017-02696-6
Remark, R., Alifano, M., Cremer, I., Lupo, A., Dieu-Nosjean, M. C., Riquet, M., et al. (2013). Characteristics and clinical impacts of the immune environments in colorectal and renal cell carcinoma lung metastases: Influence of tumor origin. Clin. Cancer Res. 19 (15), 4079–4091. doi:10.1158/1078-0432.CCR-12-3847
Ribas, A., Comin-Anduix, B., Chmielowski, B., Jalil, J., de la Rocha, P., McCannel, T. A., et al. (2009). Dendritic cell vaccination combined with CTLA4 blockade in patients with metastatic melanoma. Clin. Cancer Res. 15 (19), 6267–6276. doi:10.1158/1078-0432.CCR-09-1254
Ribas, A., Medina, T., Kummar, S., Amin, A., Kalbasi, A., Drabick, J. J., et al. (2018). SD-101 in combination with pembrolizumab in advanced melanoma: Results of a phase ib, multicenter study. Cancer Discov. 8 (10), 1250–1257. doi:10.1158/2159-8290.CD-18-0280
Ribeiro, F., Perucha, E., and Graca, L. (2022). T follicular cells: The regulators of germinal center homeostasis. Immunol. Lett. 244, 1–11. doi:10.1016/j.imlet.2022.02.008
Rita, C., Lauss, M., Adriana, S., Donia, M., Mathilde Skaarup, L., Shamik, M., et al. (2020). Tertiary lymphoid structures improve immunotherapy and survival in melanoma. Nature 577, 561–565. doi:10.1038/s41586-019-1914-8
Roberts, A. I., Brolin, R. E., and Ebert, E. C. (1999). Integrin alpha1beta1 (VLA-1) mediates adhesion of activated intraepithelial lymphocytes to collagen. Immunology 97 (4), 679–685. doi:10.1046/j.1365-2567.1999.00812.x
Roberts, E. W., Broz, M. L., Binnewies, M., Headley, M. B., Nelson, A. E., Wolf, D. M., et al. (2016). Critical role for cd103(+)/cd141(+) dendritic cells bearing CCR7 for tumor antigen trafficking and priming of T cell immunity in melanoma. Cancer Cell. 30 (2), 324–336. doi:10.1016/j.ccell.2016.06.003
Rodell, C. B., Ahmed, M. S., Garris, C. S., Pittet, M. J., and Weissleder, R. (2019). Development of adamantane-conjugated TLR7/8 agonists for supramolecular delivery and cancer immunotherapy. Theranostics 9 (26), 8426–8436. doi:10.7150/thno.35434
Rodell, C. B., Arlauckas, S. P., Cuccarese, M. F., Garris, C. S., Li, R., Ahmed, M. S., et al. (2018). TLR7/8-agonist-loaded nanoparticles promote the polarization of tumour-associated macrophages to enhance cancer immunotherapy. Nat. Biomed. Eng. 2 (8), 578–588. doi:10.1038/s41551-018-0236-8
Romee, R., Rosario, M., Berrien-Elliott, M. M., Wagner, J. A., Jewell, B. A., Schappe, T., et al. (2016). Cytokine-induced memory-like natural killer cells exhibit enhanced responses against myeloid leukemia. Sci. Transl. Med. 8 (357), 357ra123. 357ra123. doi:10.1126/scitranslmed.aaf2341
Ruiz de Galarreta, M., Bresnahan, E., Molina-Sánchez, P., Lindblad, K. E., Maier, B., Sia, D., et al. (2019). β-Catenin activation promotes immune escape and resistance to anti-PD-1 therapy in hepatocellular carcinoma. Cancer Discov. 9 (8), 1124–1141. doi:10.1158/2159-8290.CD-19-0074
Sade-Feldman, M., Yizhak, K., Bjorgaard, S. L., Ray, J. P., de Boer, C. G., Jenkins, R. W., et al. (2018). Defining T cell states associated with response to checkpoint immunotherapy in melanoma. Cell. 175 (4), 998–1013. e20. doi:10.1016/j.cell.2018.10.038
Saito, T., Nishikawa, H., Wada, H., Nagano, Y., Sugiyama, D., Atarashi, K., et al. (2016). Two FOXP3(+)CD4(+) T cell subpopulations distinctly control the prognosis of colorectal cancers. Nat. Med. 22 (6), 679–684. doi:10.1038/nm.4086
Saito, Y., Komori, S., Kotani, T., Murata, Y., and Matozaki, T. (2022). The role of type-2 conventional dendritic cells in the regulation of tumor immunity. Cancers 14 (8), 1976. doi:10.3390/cancers14081976
Salimi, M., Wang, R., Yao, X., Li, X., Wang, X., Hu, Y., et al. (2018). Activated innate lymphoid cell populations accumulate in human tumour tissues. BMC cancer 18 (1), 341. doi:10.1186/s12885-018-4262-4
Sallusto, F., Geginat, J., and Lanzavecchia, A. (2004). Central memory and effector memory T cell subsets: Function, generation, and maintenance. Annu. Rev. Immunol. 22, 745–763. doi:10.1146/annurev.immunol.22.012703.104702
Sallusto, F., Lenig, D., Förster, R., Lipp, M., and Lanzavecchia, A. (1999). Two subsets of memory T lymphocytes with distinct homing potentials and effector functions. Nature 401 (6754), 708–712. doi:10.1038/44385
Salvo, C. D., Kristine-Ann, G. B., and Pizarro, T. (2020). Cytokine-mediated regulation of innate lymphoid cell plasticity in gut mucosal immunity. Front. Immunol. 11, 585319. doi:10.3389/fimmu.2020.585319
Sánchez-Paulete, A. R., Teijeira, Á., Quetglas, J. I., Rodríguez-Ruiz, M. E., Sánchez-Arráez, Á., Labiano, S., et al. (2018). Intratumoral immunotherapy with XCL1 and sFlt3L encoded in recombinant semliki forest virus-derived vectors fosters dendritic cell-mediated T-cell cross-priming. Cancer Res. 78 (23), 6643–6654. doi:10.1158/0008-5472.CAN-18-0933
Sandoval, F., Terme, M., Nizard, M., Badoual, C., Bureau, M. F., Freyburger, L., et al. (2013). Mucosal imprinting of vaccine-induced CD8+T cells is crucial to inhibit the growth of mucosal tumors. Sci. Transl. Med. 5 (172), 172ra20. doi:10.1126/scitranslmed.3004888
Sato, K., Sato, N., Xu, B., Nakamura, Y., Nagaya, T., Choyke, P. L., et al. (2016). Spatially selective depletion of tumor-associated regulatory T cells with near-infrared photoimmunotherapy. Sci. Transl. Med. 8 (352), 352ra110. doi:10.1126/scitranslmed.aaf6843
Sautès-Fridman, C., Petitprez, F., Calderaro, J., and Fridman, W. H. (2019). Tertiary lymphoid structures in the era of cancer immunotherapy. Nat. Rev. Cancer 19 (6), 307–325. doi:10.1038/s41568-019-0144-6
Savas, P., Virassamy, B., Ye, C., Salim, A., Mintoff, C. P., Caramia, F., et al. (2018). Single-cell profiling of breast cancer T cells reveals a tissue-resident memory subset associated with improved prognosis. Nat. Med. 24 (7), 986–993. doi:10.1038/s41591-018-0078-7
Sawant, D. V., Yano, H., Chikina, M., Zhang, Q., Liao, M., Liu, C., et al. (2019). Adaptive plasticity of IL-10(+) and IL-35(+) T(reg) cells cooperatively promotes tumor T cell exhaustion. Nat. Immunol. 20 (6), 724–735. doi:10.1038/s41590-019-0346-9
Saze, Z., Schuler, P. J., Hong, C. S., Cheng, D., Jackson, E. K., and Whiteside, T. L. (2013). Adenosine production by human B cells and B cell-mediated suppression of activated T cells. Blood 122 (1), 9–18. doi:10.1182/blood-2013-02-482406
Schenkel, J. M., and Masopust, D. (2014). Tissue-resident memory T cells. Immunity 41 (6), 886–897. doi:10.1016/j.immuni.2014.12.007
Schleypen, J. S., Von Geldern, M., Weiss, E. H., Kotzias, N., Rohrmann, K., Schendel, D. J., et al. (2003). Renal cell carcinoma-infiltrating natural killer cells express differential repertoires of activating and inhibitory receptors and are inhibited by specific HLA class I allotypes. Int. J. Cancer 106 (6), 905–912. doi:10.1002/ijc.11321
Schmidleithner, L., and Feuerer, M. (2022). Tfh cells induce intratumoral tertiary lymphoid structures. Trends Immunol. 43 (4), 274–276. doi:10.1016/j.it.2022.02.010
Schoenberger, S. P., Toes, R. E., van der Voort, E. I., Offringa, R., and Melief, C. J. (1998). T-cell help for cytotoxic T lymphocytes is mediated by CD40-CD40L interactions. Nature 393 (6684), 480–483. doi:10.1038/31002
Schumacher, T. N., and Thommen, D. S. (2022). Tertiary lymphoid structures in cancer. Sci. (New York, NY) 375 (6576), eabf9419. doi:10.1126/science.abf9419
Schweiger, T., Berghoff, A. S., Glogner, C., Glueck, O., Rajky, O., Traxler, D., et al. (2016). Tumor-infiltrating lymphocyte subsets and tertiary lymphoid structures in pulmonary metastases from colorectal cancer. Clin. Exp. Metastasis 33 (7), 727–739. doi:10.1007/s10585-016-9813-y
Segura, E., Touzot, M., Bohineust, A., Cappuccio, A., Chiocchia, G., Hosmalin, A., et al. (2013). Human inflammatory dendritic cells induce Th17 cell differentiation. Immunity 38 (2), 336–348. doi:10.1016/j.immuni.2012.10.018
Semeraro, M., Rusakiewicz, S., Minard-Colin, V., Delahaye, N. F., Enot, D., Vély, F., et al. (2015). Clinical impact of the NKp30/B7-H6 axis in high-risk neuroblastoma patients. Sci. Transl. Med. 7 (283), 283ra55. doi:10.1126/scitranslmed.aaa2327
Serafini, P., Borrello, I., and Bronte, V. (2006). Myeloid suppressor cells in cancer: Recruitment, phenotype, properties, and mechanisms of immune suppression. Semin. Cancer Biol. 16 (1), 53–65. doi:10.1016/j.semcancer.2005.07.005
Seto, K., Shoda, J., Horibe, T., Warabi, E., Kohno, M., Yanagawa, T., et al. (2014). Targeting interleukin-4 receptor alpha by hybrid Peptide for novel biliary tract cancer therapy. Int. J. Hepatol. 2014, 584650. doi:10.1155/2014/584650
Shan, F., Somasundaram, A., Bruno, T. C., Workman, C. J., and Vignali, D. A. A. (2022). Therapeutic targeting of regulatory T cells in cancer. Trends Cancer. doi:10.1016/j.trecan.2022.06.008
Shen, Y., Li, X. L., Li, Y. X., Shan, Z. G., Zhao, Y. L., Cheng, P., et al. (2021). Distribution, phenotype, functional and clinical relevance of CD8(+)CD103(+) tissue-resident memory T cells in human gastric cancer. Cancer Immunol. Immunother. 71 (7), 1645–1654. doi:10.1007/s00262-021-03105-0
Shifrin, N., Raulet, D. H., and Ardolino, M. (2014). NK cell self tolerance, responsiveness and missing self recognition. Semin. Immunol. 26 (2), 138–144. doi:10.1016/j.smim.2014.02.007
Sikic, B. I., Lakhani, N., Patnaik, A., Shah, S. A., Chandana, S. R., Rasco, D., et al. (2019). First-in-Human, first-in-class phase I trial of the anti-CD47 antibody Hu5F9-G4 in patients with advanced cancers. J. Clin. Oncol. 37 (12), 946–953. doi:10.1200/JCO.18.02018
Siliņa, K., Soltermann, A., Attar, F. M., Casanova, R., Uckeley, Z. M., Thut, H., et al. (2018). Germinal centers determine the prognostic relevance of tertiary lymphoid structures and are impaired by corticosteroids in lung squamous cell carcinoma. Cancer Res. 78 (5), 1308–1320. doi:10.1158/0008-5472.CAN-17-1987
Sinclair, C., Revenko, A. S., Johnson, R. B., Peter, A., and Macleod, A. R. (Editors) (2019). “Abstract 2713: Discovery and characterization of AZD8701, a high affinity antisense oligonucleotide targeting FOXP3 to relieve immunosuppression in cancer,” in Proceedings: AACR annual meeting(Atlanta, GA: IEEE).
Sinha, P., Clements, V. K., Bunt, S. K., Albelda, S. M., and Ostrand-Rosenberg, S. (1950). Cross-talk between myeloid-derived suppressor cells and macrophages subverts tumor immunity toward a type 2 response. J. Immunol. 179 (2), 977–983. doi:10.4049/jimmunol.179.2.977
Sinha, P., Okoro, C., Foell, D., Freeze, H. H., Ostrand-Rosenberg, S., and Srikrishna, G. (1950)., 181. Baltimore, Md, 4666–4675. doi:10.4049/jimmunol.181.7.4666Proinflammatory S100 proteins regulate the accumulation of myeloid-derived suppressor cellsJ. Immunol.7
Sivori, S., Pende, D., Quatrini, L., Pietra, G., Della Chiesa, M., Vacca, P., et al. (2021). NK cells and ILCs in tumor immunotherapy. Mol. Asp. Med. 80, 100870. doi:10.1016/j.mam.2020.100870
Sivori, S., Vacca, P., Del Zotto, G., Munari, E., Mingari, M. C., and Moretta, L. (2019). Human NK cells: Surface receptors, inhibitory checkpoints, and translational applications. Cell. Mol. Immunol. 16 (5), 430–441. doi:10.1038/s41423-019-0206-4
Skon, C. N., Lee, J. Y., Anderson, K. G., Masopust, D., Hogquist, K. A., and Jameson, S. C. (2013). Transcriptional downregulation of S1pr1 is required for the establishment of resident memory CD8+ T cells. Nat. Immunol. 14 (12), 1285–1293. doi:10.1038/ni.2745
Sloas, C., Gill, S., and Klichinsky, M. (2021). Engineered CAR-macrophages as adoptive immunotherapies for solid tumors. Front. Immunol. 12, 783305. doi:10.3389/fimmu.2021.783305
Smith, K. N. (2022). Big fish in small ponds: Narrow repertoires of stem-like tissue-resident T cells yield great power in anti-tumor immunity. Immunity 55 (6), 979–981. doi:10.1016/j.immuni.2022.05.017
Solomon, I., Amann, M., Goubier, A., Arce Vargas, F., Zervas, D., Qing, C., et al. (2020). CD25-T(reg)-depleting antibodies preserving IL-2 signaling on effector T cells enhance effector activation and antitumor immunity. Nat. Cancer 1 (12), 1153–1166. doi:10.1038/s43018-020-00133-0
Spits, H., Artis, D., Colonna, M., Diefenbach, A., Di Santo, J. P., Eberl, G., et al. (2013). Innate lymphoid cells--a proposal for uniform nomenclature. Nat. Rev. Immunol. 13 (2), 145–149. doi:10.1038/nri3365
Spranger, S., Dai, D., Horton, B., and Gajewski, T. F. (2017). Tumor-residing Batf3 dendritic cells are required for effector T cell trafficking and adoptive T cell therapy. Cancer Cell. 31 (5), 711–723. e4. doi:10.1016/j.ccell.2017.04.003
Stabile, H., Nisti, P., Pagliara, D., Locatelli, F., Santoni, A., and Gismondi, A. (2015). Response to comment on Multifunctional human CD56low CD16low NK cells are the prominent subset in bone marrow of both pediatric healthy donors and leukemic patients. Haematologica 100 (8), e332–3. doi:10.3324/haematol.2015.130831
Stefania, R., Laura, C., Raveane, A., and Bertolini, F. (2021). The dual role of innate lymphoid and natural killer cells in cancer. From phenotype to single-cell transcriptomics, functions and clinical uses. Cancers 13, 5042. doi:10.3390/cancers13205042
Steinert, E. M., Schenkel, J. M., Fraser, K. A., Beura, L. K., Manlove, L. S., Igyártó, B. Z., et al. (2015). Quantifying memory CD8 T cells reveals regionalization of immunosurveillance. Cell. 161 (4), 737–749. doi:10.1016/j.cell.2015.03.031
Stiff, A., Trikha, P., Wesolowski, R., Kendra, K., Hsu, V., Uppati, S., et al. (2016). Myeloid-derived suppressor cells express bruton's tyrosine kinase and can Be depleted in tumor-bearing hosts by ibrutinib treatment. Cancer Res. 76 (8), 2125–2136. doi:10.1158/0008-5472.CAN-15-1490
Stratikos, E., Stamogiannos, A., Zervoudi, E., and Fruci, D. (2014). A role for naturally occurring alleles of endoplasmic reticulum aminopeptidases in tumor immunity and cancer pre-disposition. Front. Oncol. 4, 363. doi:10.3389/fonc.2014.00363
Tajaldini, M., Saeedi, M., Amiriani, T., Amiriani, A. H., Sedighi, S., Mohammad Zadeh, F., et al. (2022). Cancer-associated fibroblasts (CAFs) and tumor-associated macrophages (TAMs); where do they stand in tumorigenesis and how they can change the face of cancer therapy? Eur. J. Pharmacol. 928, 175087. doi:10.1016/j.ejphar.2022.175087
Takahara, T., Tsuyuki, T., Satou, A., Wada, E., Sakurai, K., Ueda, R., et al. (2022). TGFB1 mRNA expression is associated with poor prognosis and specific features of inflammation in ccRCC. Virchows Arch. 480 (3), 635–643. doi:10.1007/s00428-021-03256-6
Tarantino, P., Barroso-Sousa, R., Garrido-Castro, A. C., McAllister, S. S., Guerriero, J. L., Mittendorf, E., et al. (2022). Understanding resistance to immune checkpoint inhibitors in advanced breast cancer. Expert Rev. Anticancer Ther. 22 (2), 141–153. doi:10.1080/14737140.2022.2020650
Tartter, P. I., Steinberg, B., Barron, D. M., and Martinelli, G. (1960). The prognostic significance of natural killer cytotoxicity in patients with colorectal cancer. Arch. Surg. 122 (11), 1264–1268. doi:10.1001/archsurg.1987.01400230050009
Teillaud, J. L., and Dieu-Nosjean, M. C. (2022). Intratumoral plasma cells: More than a predictive marker of response to anti-PD-L1 treatment in lung cancer? Cancer Cell. 40 (3), 240–243. doi:10.1016/j.ccell.2022.02.008
Tel, J., Aarntzen, E. H., Baba, T., Schreibelt, G., Schulte, B. M., Benitez-Ribas, D., et al. (2013). Natural human plasmacytoid dendritic cells induce antigen-specific T-cell responses in melanoma patients. Cancer Res. 73 (3), 1063–1075. doi:10.1158/0008-5472.CAN-12-2583
Theocharides, A. P., Jin, L., Cheng, P. Y., Prasolava, T. K., Malko, A. V., Ho, J. M., et al. (2012). Disruption of SIRPα signaling in macrophages eliminates human acute myeloid leukemia stem cells in xenografts. J. Exp. Med. 209 (10), 1883–1899. doi:10.1084/jem.20120502
Tian, X., Shen, H., Li, Z., Wang, T., and Wang, S. (2019). Tumor-derived exosomes, myeloid-derived suppressor cells, and tumor microenvironment. J. Hematol. Oncol. 12 (1), 84. doi:10.1186/s13045-019-0772-z
Tie, Y., Fan, T., Yu-quan, W., and Xia-Wei, W. (2022). Immunosuppressive cells in cancer: Mechanisms and potential therapeutic targets.
Tie, Y., Tang, F., Wei, Y. Q., and Wei, X. W. (2022). Immunosuppressive cells in cancer: Mechanisms and potential therapeutic targets. J. Hematol. Oncol. 15 (1), 61. doi:10.1186/s13045-022-01282-8
Tobin, R. P., Jordan, K. R., Robinson, W. A., Davis, D., Borges, V. F., Gonzalez, R., et al. (2018). Targeting myeloid-derived suppressor cells using all-trans retinoic acid in melanoma patients treated with Ipilimumab. Int. Immunopharmacol. 63, 282–291. doi:10.1016/j.intimp.2018.08.007
Trabanelli, S., Chevalier, M. F., Martinez-Usatorre, A., Gomez-Cadena, A., Salomé, B., Lecciso, M., et al. (2017). Tumour-derived PGD2 and NKp30-B7H6 engagement drives an immunosuppressive ILC2-MDSC axis. Nat. Commun. 8 (1), 593. doi:10.1038/s41467-017-00678-2
Tumino, N., Vacca, P., Quatrini, L., Munari, E., Moretta, F., Pelosi, A., et al. (2020). Helper innate lymphoid cells in human tumors: A double-edged sword? Front. Immunol. 10, 3140. doi:10.3389/fimmu.2019.03140
Turnis, M. E., Sawant, D. V., Szymczak-Workman, A. L., Andrews, L. P., Delgoffe, G. M., Yano, H., et al. (2016). Interleukin-35 limits anti-tumor immunity. Immunity 44 (2), 316–329. doi:10.1016/j.immuni.2016.01.013
Valanparambil, R. M., Tam, M., Gros, P. P., Auger, J. P., Segura, M., Gros, P., et al. (2017). IRF-8 regulates expansion of myeloid-derived suppressor cells and Foxp3+ regulatory T cells and modulates Th2 immune responses to gastrointestinal nematode infection. PLoS Pathog. 13 (10), e1006647. doi:10.1371/journal.ppat.1006647
Vallera, D. A., Felices, M., McElmurry, R., McCullar, V., Zhou, X., Ju, Schmohl, et al. (2016). IL15 trispecific killer engagers (TriKE) make natural killer cells specific to CD33+ targets while also inducing persistence, in vivo expansion, and enhanced function. Clin. Cancer Res. 22 (14), 3440–3450. doi:10.1158/1078-0432.CCR-15-2710
Vallera, D. A., Ferrone, S., Kodal, B., Hinderlie, P., Bendzick, L., Ettestad, B., et al. (2020). NK-Cell-Mediated targeting of various solid tumors using a B7-H3 tri-specific killer engager in vitro and in vivo. Cancers 12 (9), E2659. doi:10.3390/cancers12092659
van Dijk, N., Gil-Jimenez, A., Silina, K., Hendricksen, K., Smit, L. A., de Feijter, J. M., et al. (2020). Preoperative ipilimumab plus nivolumab in locoregionally advanced urothelial cancer: The NABUCCO trial. Nat. Med. 26 (12), 1839–1844. doi:10.1038/s41591-020-1085-z
van Montfoort, N., Borst, L., Korrer, M. J., Sluijter, M., Marijt, K. A., Santegoets, S. J., et al. (2018). NKG2A blockade potentiates CD8 T cell immunity induced by cancer vaccines. Cell. 175 (7), 1744–1755. e15. doi:10.1016/j.cell.2018.10.028
Vats, K., Kruglov, O., Sahoo, B., Soman, V., Zhang, J., Shurin, G. V., et al. (2022). Sensory nerves impede the formation of tertiary lymphoid structures and development of protective anti-melanoma immune responses. Cancer immunology research.
Velasco-Velázquez, M., Jiao, X., De La Fuente, M., Pestell, T. G., Ertel, A., Lisanti, M. P., et al. (2012). CCR5 antagonist blocks metastasis of basal breast cancer cells. Cancer Res. 72 (15), 3839–3850. doi:10.1158/0008-5472.CAN-11-3917
Vella, J. L., Molodtsov, A., Angeles, C. V., Branchini, B. R., Turk, M. J., and Huang, Y. H. (2021). Dendritic cells maintain anti-tumor immunity by positioning CD8 skin-resident memory T cells. Life Sci. Alliance 4 (10), e202101056. doi:10.26508/lsa.202101056
Vergadi, E., Ieronymaki, E., Lyroni, K., Vaporidi, K., and Tsatsanis, C. (1950). Akt signaling pathway in macrophage activation and M1/M2 polarization. J. Immunol. 198, 1006–1014. doi:10.4049/jimmunol.16015153
Verma, R., Er, J. Z., Pu, R. W., Sheik Mohamed, J., Soo, R. A., Muthiah, H. M., et al. (2020). Eomes expression defines group 1 innate lymphoid cells during metastasis in human and mouse. Front. Immunol. 11, 1190. doi:10.3389/fimmu.2020.01190
Vidyarthi, A., Khan, N., Agnihotri, T., Negi, S., Das, D. K., Aqdas, M., et al. (2018). TLR-3 stimulation skews M2 macrophages to M1 through IFN-αβ signaling and restricts tumor progression. Front. Immunol. 9, 1650. doi:10.3389/fimmu.2018.01650
Villani, A. C., Satija, R., Reynolds, G., Sarkizova, S., Shekhar, K., Fletcher, J., et al. (2017)., 356. New York, NY), eaah4573. doi:10.1126/science.aah4573Single-cell RNA-seq reveals new types of human blood dendritic cells, monocytes, and progenitorsScience6335
Villanueva, M. T. (2020). Macrophages get a CAR. Nat. Rev. Immunol. 20 (5), 273. doi:10.1038/s41577-020-0302-9
Vinuesa, C. G., Linterman, M. A., Yu, D., and MacLennan, I. C. (2016). Follicular helper T cells. Annu. Rev. Immunol. 34, 335–368. doi:10.1146/annurev-immunol-041015-055605
Vivier, E., Artis, D., Colonna, M., Diefenbach, A., Di Santo, J. P., Eberl, G., et al. (2018). Innate lymphoid cells: 10 Years on. Cell. 174 (5), 1054–1066. doi:10.1016/j.cell.2018.07.017
Voabil, P., de Bruijn, M., Roelofsen, L. M., Hendriks, S. H., Brokamp, S., van den Braber, M., et al. (2021). An ex vivo tumor fragment platform to dissect response to PD-1 blockade in cancer. Nat. Med. 27 (7), 1250–1261. doi:10.1038/s41591-021-01398-3
Voskoboinik, I., Whisstock, J. C., and Trapani, J. A. (2015). Perforin and granzymes: Function, dysfunction and human pathology. Nat. Rev. Immunol. 15 (6), 388–400. doi:10.1038/nri3839
Wada, S., Kobayashi, S., and Tsunoda, T. (2022). Future prospects for cancer immunotherapy - strategies for ineffective cancers. Hum. Vaccin. Immunother. 18 (1), 2031699. doi:10.1080/21645515.2022.2031699
Wagner, J., Pfannenstiel, V., Waldmann, A., Bergs, J. W. J., Brill, B., Huenecke, S., et al. (2017). A two-phase expansion protocol combining interleukin (IL)-15 and IL-21 improves natural killer cell proliferation and cytotoxicity against rhabdomyosarcoma. Front. Immunol. 8, 676. doi:10.3389/fimmu.2017.00676
Wagner, J. A., Rosario, M., Romee, R., Berrien-Elliott, M. M., Schneider, S. E., Leong, J. W., et al. (2017). CD56bright NK cells exhibit potent antitumor responses following IL-15 priming. J. Clin. Investig. 127 (11), 4042–4058. doi:10.1172/JCI90387
Wagner, M., Ealey, K. N., Tetsu, H., Kiniwa, T., Motomura, Y., Moro, K., et al. (2020). Tumor-derived lactic acid contributes to the paucity of intratumoral ILC2s. Cell. Rep. 30 (8), 2743–2757. e5. doi:10.1016/j.celrep.2020.01.103
Wang, J., Jiang, D., Zheng, X., Li, W., Zhao, T., Wang, D., et al. (2022). Tertiary lymphoid structure and decreased CD8(+) T cell infiltration in minimally invasive adenocarcinoma. iScience 25 (3), 103883. doi:10.1016/j.isci.2022.103883
Wang, K., and Karin, M. (2015). The IL-23 to IL-17 cascade inflammation-related cancers. Clin. Exp. Rheumatol. 33 (4), S87–S90.
Wang, S., Qu, Y., Xia, P., Chen, Y., Zhu, X., Zhang, J., et al. (2020). Transdifferentiation of tumor infiltrating innate lymphoid cells during progression of colorectal cancer. Cell. Res. 30 (7), 610–622. doi:10.1038/s41422-020-0312-y
Wang, S., Wu, P., Chen, Y., and Chai, Y. (2020). Ambiguous roles and potential therapeutic strategies of innate lymphoid cells in different types of tumor. Oncol. Lett. 20 (2), 1513–1525. doi:10.3892/ol.2020.11736
Wang, S., Xia, P., Chen, Y., Qu, Y., Xiong, Z., Ye, B., et al. (2017). Regulatory innate lymphoid cells control innate intestinal inflammation. Cell. 171 (1), 201–216. e18. doi:10.1016/j.cell.2017.07.027
Wang, X., Wong, K., Ouyang, W., and Rutz, S. (2019). Targeting IL-10 family cytokines for the treatment of human diseases. Cold Spring Harb. Perspect. Biol. 11 (2), a028548. doi:10.1101/cshperspect.a028548
Wculek, S. K., Amores-Iniesta, J., Conde-Garrosa, R., Khouili, S. C., Melero, I., and Sancho, D. (2019). Effective cancer immunotherapy by natural mouse conventional type-1 dendritic cells bearing dead tumor antigen. J. Immunother. Cancer 7 (1), 100. doi:10.1186/s40425-019-0565-5
Weber, J. P., Fuhrmann, F., Feist, R. K., Lahmann, A., Al Baz, M. S., Gentz, L. J., et al. (2015). ICOS maintains the T follicular helper cell phenotype by down-regulating Krüppel-like factor 2. J. Exp. Med. 212 (2), 217–233. doi:10.1084/jem.20141432
Weber, R., Groth, C., Lasser, S., Arkhypov, I., Petrova, V., Altevogt, P., et al. (2021). IL-6 as a major regulator of MDSC activity and possible target for cancer immunotherapy. Cell. Immunol. 359, 104254. doi:10.1016/j.cellimm.2020.104254
Weed, D. T., Vella, J. L., Reis, I. M., De la Fuente, A. C., Gomez, C., Sargi, Z., et al. (2015). Tadalafil reduces myeloid-derived suppressor cells and regulatory T cells and promotes tumor immunity in patients with head and neck squamous cell carcinoma. Clin. Cancer Res. 21 (1), 39–48. doi:10.1158/1078-0432.CCR-14-1711
Wennhold, K., Weber, T. M., Klein-Gonzalez, N., Thelen, M., Garcia-Marquez, M., Chakupurakal, G., et al. (2017). CD40-activated B cells induce anti-tumor immunity in vivo. Oncotarget 8 (17), 27740–27753. doi:10.18632/oncotarget.7720
Westdorp, H., Creemers, J. H. A., van Oort, I. M., Schreibelt, G., Gorris, M. A. J., Mehra, N., et al. (2019). Blood-derived dendritic cell vaccinations induce immune responses that correlate with clinical outcome in patients with chemo-naive castration-resistant prostate cancer. J. Immunother. Cancer 7 (1), 302. doi:10.1186/s40425-019-0787-6
Whiteside, T. L. (2012). Tumor-infiltrating lymphocytes and their role in solid tumor progression. Exp. Suppl. 113, 89–106. doi:10.1007/978-3-030-91311-3_3
Wiehagen, K. R., Girgis, N. M., Yamada, D. H., Smith, A. A., Chan, S. R., Grewal, I. S., et al. (2017). Combination of CD40 agonism and CSF-1R blockade reconditions tumor-associated macrophages and drives potent antitumor immunity. Cancer Immunol. Res. 5 (12), 1109–1121. doi:10.1158/2326-6066.CIR-17-0258
Wiernik, A., Foley, B., Zhang, B., Verneris, M. R., Warlick, E., Gleason, M. K., et al. (2013). Targeting natural killer cells to acute myeloid leukemia in vitro with a CD16 x 33 bispecific killer cell engager and ADAM17 inhibition. Clin. Cancer Res. 19 (14), 3844–3855. doi:10.1158/1078-0432.CCR-13-0505
Wilczyński, J. R., and Nowak, M. (2012). Cancer immunoediting: Elimination, equilibrium, and immune escape in solid tumors. Exp. Suppl. 113, 1–57. doi:10.1007/978-3-030-91311-3_1
Williams, M. A., Tyznik, A. J., and Bevan, M. J. (2006). Interleukin-2 signals during priming are required for secondary expansion of CD8+ memory T cells. Nature 441 (7095), 890–893. doi:10.1038/nature04790
Wu, Q., Zhou, W., Yin, S., Zhou, Y., Chen, T., Qian, J., et al. (2019). Blocking triggering receptor expressed on myeloid cells-1-positive tumor-associated macrophages induced by hypoxia reverses immunosuppression and anti-programmed cell death ligand 1 resistance in liver cancer. Hepatol. Baltim. Md) 70 (1), 198–214. doi:10.1002/hep.30593
Wu, S. Y., Fu, T., Jiang, Y. Z., and Shao, Z. M. (2020). Natural killer cells in cancer biology and therapy. Mol. Cancer 19 (1), 120. doi:10.1186/s12943-020-01238-x
Wu, T. C., Xu, K., Banchereau, R., Marches, F., Yu, C. I., Martinek, J., et al. (2014). Reprogramming tumor-infiltrating dendritic cells for CD103+ CD8+ mucosal T-cell differentiation and breast cancer rejection. Cancer Immunol. Res. 2 (5), 487–500. doi:10.1158/2326-6066.CIR-13-0217
Xia, X., Wang, W., Yin, K., and Wang, S. (2020). Interferon regulatory factor 8 governs myeloid cell development. Cytokine Growth Factor Rev. 55, 48–57. doi:10.1016/j.cytogfr.2020.03.003
Xiang, X., Wang, J., Lu, D., and Xu, X. (2021). Targeting tumor-associated macrophages to synergize tumor immunotherapy. Signal Transduct. Target. Ther. 6 (1), 75. doi:10.1038/s41392-021-00484-9
Xu, M., Wang, Y., Xia, R., Wei, Y., and Wei, X. (2021). Role of the CCL2-CCR2 signalling axis in cancer: Mechanisms and therapeutic targeting. Cell. Prolif. 54 (10), e13115. doi:10.1111/cpr.13115
Xu, X., Ye, L., Zhang, Q., Shen, H., Li, S., Zhang, X., et al. (2021). Group-2 innate lymphoid cells promote HCC progression through CXCL2-neutrophil-induced immunosuppression. Hepatol. Baltim. Md) 74 (5), 2526–2543. doi:10.1002/hep.31855
Yalniz, F. F., Daver, N., Rezvani, K., Kornblau, S., Ohanian, M., Borthakur, G., et al. (2018). A pilot trial of lirilumab with or without azacitidine for patients with myelodysplastic syndrome. Clin. Lymphoma Myeloma Leuk. 18 (10), 658–663. doi:10.1016/j.clml.2018.06.011
Yamaguchi, K., Ito, M., Ohmura, H., Hanamura, F., Nakano, M., Tsuchihashi, K., et al. (2020). Helper T cell-dominant tertiary lymphoid structures are associated with disease relapse of advanced colorectal cancer. Oncoimmunology 9 (1), 1724763. doi:10.1080/2162402X.2020.1724763
Yang, H., Zhang, Q., Xu, M., Wang, L., Chen, X., Feng, Y., et al. (2020). CCL2-CCR2 axis recruits tumor associated macrophages to induce immune evasion through PD-1 signaling in esophageal carcinogenesis. Mol. Cancer 19 (1), 41. doi:10.1186/s12943-020-01165-x
Yang, J., Zhang, J. X., Wang, H., Wang, G. L., Hu, Q. G., and Zheng, Q. C. (2012). Hepatocellular carcinoma and macrophage interaction induced tumor immunosuppression via Treg requires TLR4 signaling. World J. Gastroenterol. 18 (23), 2938–2947. doi:10.3748/wjg.v18.i23.2938
Yang, K., and Kallies, A. (2021). Tissue-specific differentiation of CD8(+) resident memory T cells. Trends Immunol. 42 (10), 876–890. doi:10.1016/j.it.2021.08.002
Yang, L., He, Y. T., Dong, S., Wei, X. W., Chen, Z. H., Zhang, B., et al. (2022). Single-cell transcriptome analysis revealed a suppressive tumor immune microenvironment in EGFR mutant lung adenocarcinoma. J. Immunother. Cancer 10 (2), e003534. doi:10.1136/jitc-2021-003534
Yang, L., Horibe, T., Kohno, M., Haramoto, M., Ohara, K., Puri, R. K., et al. (2012). Targeting interleukin-4 receptor α with hybrid peptide for effective cancer therapy. Mol. Cancer Ther. 11 (1), 235–243. doi:10.1158/1535-7163.MCT-11-0363
Yeung, J., Yaghoobi, V., Miyagishima, D., Vesely, M. D., Zhang, T., Badri, T., et al. (2021). Targeting the CSF1/CSF1R axis is a potential treatment strategy for malignant meningiomas. Neuro. Oncol. 23 (11), 1922–1935. doi:10.1093/neuonc/noab075
Yewdall, A. W., Drutman, S. B., Jinwala, F., Bahjat, K. S., and Bhardwaj, N. (2010). CD8+ T cell priming by dendritic cell vaccines requires antigen transfer to endogenous antigen presenting cells. PloS one 5 (6), e11144. doi:10.1371/journal.pone.0011144
Yu, D., Rao, S., Tsai, L. M., Lee, S. K., He, Y., Sutcliffe, E. L., et al. (2009). The transcriptional repressor Bcl-6 directs T follicular helper cell lineage commitment. Immunity 31 (3), 457–468. doi:10.1016/j.immuni.2009.07.002
Zaidi, M. R., and Merlino, G. (2011). The two faces of interferon-γ in cancer. Clin. Cancer Res. 17 (19), 6118–6124. doi:10.1158/1078-0432.CCR-11-0482
Zelenay, S., van der Veen, A. G., Böttcher, J. P., Snelgrove, K. J., Rogers, N., Acton, S. E., et al. (2015). Cyclooxygenase-dependent tumor growth through evasion of immunity. Cell. 162 (6), 1257–1270. doi:10.1016/j.cell.2015.08.015
Zeng, Q., Yang, J., Ji, J., Wang, P., Zhang, L., Yan, G., et al. (2022). PD-L1 blockade potentiates the antitumor effects of ALA-PDT and optimizes the tumor microenvironment in cutaneous squamous cell carcinoma. Oncoimmunology 11 (1), 2061396. doi:10.1080/2162402X.2022.2061396
Zens, K. D., Chen, J. K., and Farber, D. L. (2016). Vaccine-generated lung tissue-resident memory T cells provide heterosubtypic protection to influenza infection. JCI insight 1 (10), 85832. doi:10.1172/jci.insight.85832
Zhang, M., Berndt, B. E., Chen, J. J., and Kao, J. Y. (1950). Expression of a soluble TGF-beta receptor by tumor cells enhances dendritic cell/tumor fusion vaccine efficacy. J. Immunol. 181 (5), 3690–3697. doi:10.4049/jimmunol.181.5.3690
Zhang, M., Huang, L., Ding, G., Huang, H., Cao, G., Sun, X., et al. (2020). Interferon gamma inhibits CXCL8-CXCR2 axis mediated tumor-associated macrophages tumor trafficking and enhances anti-PD1 efficacy in pancreatic cancer. J. Immunother. Cancer 8 (1), e000308. doi:10.1136/jitc-2019-000308
Zhang, N., and Bevan, M. J. (2013). Transforming growth factor-β signaling controls the formation and maintenance of gut-resident memory T cells by regulating migration and retention. Immunity 39 (4), 687–696. doi:10.1016/j.immuni.2013.08.019
Zhang, Q. F., Yin, W. W., Xia, Y., Yi, Y. Y., He, Q. F., Wang, X., et al. (2017). Liver-infiltrating CD11b(-)CD27(-) NK subsets account for NK-cell dysfunction in patients with hepatocellular carcinoma and are associated with tumor progression. Cell. Mol. Immunol. 14 (10), 819–829. doi:10.1038/cmi.2016.28
Zhang, X., Wang, S., Nie, R. C., Qu, C., Chen, J., Yang, Y., et al. (2022). Immune microenvironment characteristics of urachal carcinoma and its implications for prognosis and immunotherapy. Cancers 14 (3), 615. doi:10.3390/cancers14030615
Zhang, X., Zeng, Y., Qu, Q., Zhu, J., Liu, Z., Ning, W., et al. (2017). PD-L1 induced by IFN-γ from tumor-associated macrophages via the JAK/STAT3 and PI3K/AKT signaling pathways promoted progression of lung cancer. Int. J. Clin. Oncol. 22 (6), 1026–1033. doi:10.1007/s10147-017-1161-7
Zhang, Y., Feng, Z., Liu, J., Li, H., Su, Q., Zhang, J., et al. (2022). Polarization of tumor-associated macrophages by TLR7/8 conjugated radiosensitive peptide hydrogel for overcoming tumor radioresistance. Bioact. Mat. 16, 359–371. doi:10.1016/j.bioactmat.2021.12.033
Zhang, Y., Lv, D., Kim, H. J., Kurt, R. A., Bu, W., Li, Y., et al. (2013). A novel role of hematopoietic CCL5 in promoting triple-negative mammary tumor progression by regulating generation of myeloid-derived suppressor cells. Cell. Res. 23 (3), 394–408. doi:10.1038/cr.2012.178
Zhao, H., Wang, H., Zhao, Y., Sun, Q., and Ren, X. (2022). Tumor-resident T cells, associated with tertiary lymphoid structure maturity, improve survival in patients with stage III lung adenocarcinoma. Front. Immunol. 13, 877689. doi:10.3389/fimmu.2022.877689
Zhao, S., Wu, D., Wu, P., Wang, Z., and Huang, J. (2015). Serum IL-10 predicts worse outcome in cancer patients: A meta-analysis. PloS one 10 (10), e0139598. doi:10.1371/journal.pone.0139598
Zheng, M. Z. M., and Wakim, L. M. (2021). Tissue resident memory T cells in the respiratory tract. Mucosal Immunol. 15, 379–388. doi:10.1038/s41385-021-00461-z
Zhou, J., Xu, Y., Wang, G., Mei, T., Yang, H., and Liu, Y. (2022). The TLR7/8 agonist R848 optimizes host and tumor immunity to improve therapeutic efficacy in murine lung cancer. Int. J. Oncol. 61 (1), 81. doi:10.3892/ijo.2022.5371
Keywords: tertiary lymphoid structures, antigen presentations, immunotherapy, tumor-infiltrating immune cells, tumor microenvironment
Citation: Yaping W, Zhe W, Zhuling C, Ruolei L, Pengyu F, Lili G, Cheng J, Bo Z, Liuyin L, Guangdong H, Yaoling W, Niuniu H and Rui L (2022) The soldiers needed to be awakened: Tumor-infiltrating immune cells. Front. Genet. 13:988703. doi: 10.3389/fgene.2022.988703
Received: 07 July 2022; Accepted: 29 August 2022;
Published: 29 September 2022.
Edited by:
Chuyan Wu, Nanjing Medical University, ChinaReviewed by:
Mario Ernesto Cruz-Munoz, Universidad Autónoma del Estado de Morelos, MexicoGuorong Yan, Tongji University, China
Copyright © 2022 Yaping, Zhe, Zhuling, Ruolei, Pengyu, Lili, Cheng, Bo, Liuyin, Guangdong, Yaoling, Niuniu and Rui. This is an open-access article distributed under the terms of the Creative Commons Attribution License (CC BY). The use, distribution or reproduction in other forums is permitted, provided the original author(s) and the copyright owner(s) are credited and that the original publication in this journal is cited, in accordance with accepted academic practice. No use, distribution or reproduction is permitted which does not comply with these terms.
*Correspondence: Hou Niuniu, aG91X25lb25pdUAxNjMuY29t; Ling Rui, bGluZ3J1aTAxMDVAMTYzLmNvbQ==
†These authors have contributed equally to this work