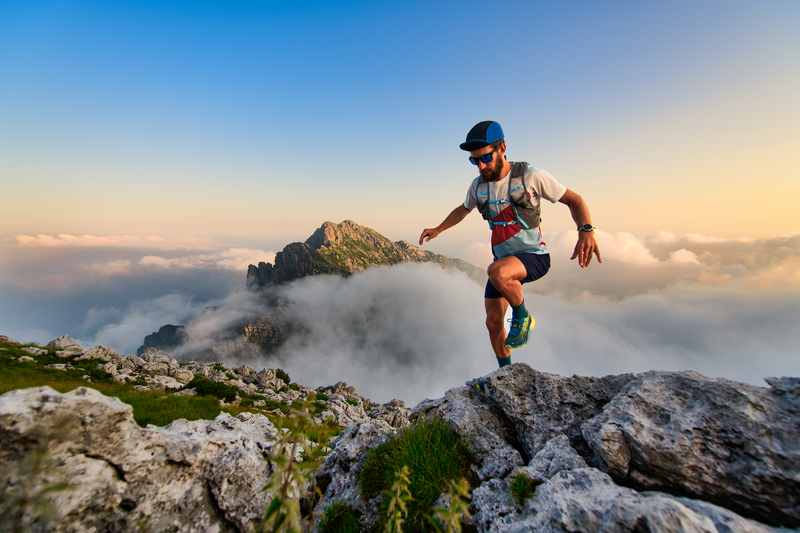
95% of researchers rate our articles as excellent or good
Learn more about the work of our research integrity team to safeguard the quality of each article we publish.
Find out more
REVIEW article
Front. Genet. , 30 August 2022
Sec. Stem Cell Research
Volume 13 - 2022 | https://doi.org/10.3389/fgene.2022.970699
This article is part of the Research Topic Genetic and Epigenetic Regulation of Stem Cells by the Immune System in Homeostasis, Regeneration, and Oncogenesis View all 6 articles
Mammalian target of rapamycin (mTOR) is a serine/threonine kinase involved in a variety of cellular functions, such as cell proliferation, metabolism, autophagy, survival and cytoskeletal organization. Furthermore, mTOR is made up of three multisubunit complexes, mTOR complex 1, mTOR complex 2, and putative mTOR complex 3. In recent years, increasing evidence has suggested that mTOR plays important roles in the differentiation and immune responses of mesenchymal stem cells (MSCs). In addition, mTOR is a vital regulator of pivotal cellular and physiological functions, such as cell metabolism, survival and ageing, where it has emerged as a novel therapeutic target for ageing-related diseases. Therefore, the mTOR signaling may develop a large impact on the treatment of ageing-related diseases with MSCs. In this review, we discuss prospects for future research in this field.
The mTOR protein plays an essential role in cell metabolism, promoting cell proliferation and survival through changes in energy and substance metabolism (Zhao et al., 2016; Karagianni et al., 2022). The mTOR protein is associated with the phosphoinositide 3-kinase (PI3K)/protein kinase B (Akt) signaling pathway, which is involved in hormone, growth factor and nutrient signal transduction (Holz et al., 2005; Murugan, 2019; Huang, 2020; Holz et al., 2021). Environmental signaling activates the mTOR pathway to regulate kinds of fundamental processes required for cell growth, metabolism, regeneration and ageing, among others (Murugan, 2019). mTOR is often dysregulated in human cancers, and somatic mutations that induce mTOR activation have recently been identified in several types of human cancers, suggesting that mTOR is a therapeutic target (Murugan, 2019; Huang, 2020; Zou et al., 2020). Over time, research on the mTOR signaling pathway has become a hot topic in various fields, such as metabolism, genomics, pharmacology and inhibitors. Studies have focused on not only animal models but also human-related diseases, such as cancers and neurodegenerative diseases. In recent years, the mTOR signaling pathway has been increasingly studied in stem cells, especially mesenchymal stem cells (MSCs).
The term “mesenchymal stem cell” was coined in the end of the 20th century, and the criteria for defining MSCs were issued by the International Society for Cellular Therapy in 2006 (Andrzejewska et al., 2019). This development was followed by numerous preclinical studies on the potential therapeutic properties of MSCs, such as immune regulation, nutritional support, the ability to spontaneously differentiate into connective tissue cells, and the ability to differentiate into most cell types under specific induction conditions (Andrzejewska et al., 2019). MSCs are widely used in regenerative medicine and oncology, in part because of the lack of conventional therapies for these demanding and expensive diseases. It has been speculated that MSCs are intermediate forms of subpopulations or pericytes, but there is still no convincing molecular evidence to confirm this hypothesis (Caplan, 2008; Blocki et al., 2013).
At present, human MSCs have been used in the clinical treatment of various diseases (Luzzani and Miriuka, 2017). In earlier studies, the efficacy of MSC therapy was primarily attributed to the ability of these cells to locally transplant and differentiate into multiple tissue types (Vizoso et al., 2017). However, with age, human brain function can also decline to cause certain senile neurodegenerative diseases, such as Alzheimer’s disease (AD) and Parkinson’s disease (PD) (Mezzaroba et al., 2019). The treatment, prevention and control of senile diseases are also major problems facing society. In this context, MSCs have been gradually used to prevent and treat senile diseases, and related studies have been performed. In this review, we summarize the latest advances in the rapidly evolving field of mTOR, discuss the composition of the mTOR signaling pathway and its related effects on MSCs, and provide a summary of the roles of the mTOR signaling pathway in MSC-mediated treatments of ageing-related diseases.
The mTOR protein belongs to the PI3K-related kinase family and is encoded by the mTOR gene, which is an evolutionarily conserved serine/threonine kinase (Xiang et al., 2011). The mTOR protein has a carboxy terminal sequence with strong homology to the catalytic domain of PI3K, and acts as a protein kinase (Brunn et al., 1997). As a central signal aggregator, mTOR can transmit and integrate various signals, such as those from growth factors, nutrients, and cellular energy metabolism, and can balance anabolic and catabolic states in a negatively regulated manner (Feng et al., 2005; Shams et al., 2021). Mammals express one mTOR protein that serves as a core and essential component of three multisubunit complexes, namely, mTOR complex 1 (mTORC1), mTOR complex 2 (mTORC2) and a putative mTOR complex 3 (mTORC3) (Zou et al., 2020; Butt et al., 2019; el Hage and Dormond, 2021; Harwood et al., 2018; Liu and Sabatini, 2020). The mTORC1 complex is composed mainly of mTOR, Raptor, mLST8, DEPTOR and PRAS40, and mTORC2 is composed mainly of mTOR, Rictor, mLST8, DEPTOR and mSIN1 (Ding et al., 2013) (Figure 1). Moreover, previous studies have shown that the PNT domain of ETV7 binds with the mTOR domain to form putative mTORC3, which does not possess key components of mTORC1/2 (Raptor, Rictor, mSIN1 and mSLT8); but its size is similar to that of mTORC2 (Thoreen et al., 2009; Luo et al., 2015; Harwood et al., 2018; Chiarini et al., 2019; Takahara et al., 2020) (Figure 1). mTORC1, as a signal integrator, balances protein synthesis and degradation to regulate cell growth by sensing nutrients and growth factors, while mTORC2 is involved in the regulation of cell survival and cytoskeletal organization by acting through protein kinase B (Akt) (Loewith et al., 2002; Kim et al., 2017a; Rion et al., 2019; Ciolczyk-Wierzbicka et al., 2020; Liu and Sabatini, 2020; Popova and Jucker, 2021). Whereas rapamycin is a potent inhibitor of mTORC1, mTORC2 is resistant to rapamycin (Ding et al., 2013) [(Julien and Roux, 2010), (Lund-Ricard et al., 2020)]. Putative mTORC3 is also resistant to rapamycin, which can assemble on the basis of ETV7 expression in various cancers and increase tumor incidence and penetrance (Harwood et al., 2018).
FIGURE 1. Domain structure of three mTOR complexes. PRAS40: A known Akt substrate is a 40 kDa proline-enriched protein; Raptor: Regulation related proteins of mTOR; FKBP-12: a prototype member of the immune affinity protein FKBP (FK506-binding protein) family capable of binding to the immunosuppressive drug FK506 (tacrolimus); mLST8: mammalian lethal with SEC13 protein eight; DEPTOR: it can interact with rictor; Protor: protein observed with rictor; Rictor: rapamycin-insensitive companion of mTOR; mSIN1: mammalian stress-activated protein kinase interacting protein; ETV7: ETS variant transcription factor 7.
Lipid synthesis is a key nutrient metabolic pathway that allows organisms to remain active even when energy is limited (Caron et al., 2015; Wang et al., 2021). Adipogenic differentiation of MSCs is a key developmental process associated with metabolic homeostasis and nutritional signal transduction (Fernandez-Veledo et al., 2013; Lee et al., 2016). In adipose cells, mTOR plays a central role in protein synthesis and adipose tissue morphogenesis (Xiang et al., 2011). Activated by anabolic signals, the kinase mTOR plays a primary role in controlling lipid biosynthesis and metabolism in response to nutrition, and a key role in the formation of complexes that both promote fat formation and inhibit fat decomposition and oxidation, ultimately leading to the accumulation of triglycerides (Caron et al., 2015). Early reports have revealed that mTOR has a lipogenic effect, and can promote adipogenesis in white adipocytes, brown adipocytes and muscle satellite cells (Vila-Bedmar et al., 2010). mTOR activity is critical in the first stage of the differentiation of brown adipocytes, and adenosine monophosphate-activated protein kinase (AMPK)-mTOR crossover is a mediator of this process (Vila-Bedmar et al., 2010). Insulin activates mTORC1 through the PI3K/AKT pathway to regulate adipogenesis, and mTOR inhibition has a negative regulation of adipocyte differentiation and insulin signaling (Kim and Chen, 2004; Yu et al., 2008; Zhang et al., 2009; Xiang et al., 2011).
In recent years, studies have shown that mTOR complexes play important roles in increasing de novo adipogenesis in liver and adipose tissue. mTORC1 has a positively regulation of sterol regulatory element binding protein (SREBP). mTORC1 promotes SREBP expression, maturation, and nuclear localization through an S6K1-dependent pathway or phosphorylates the phospholipid acid phosphatase lipin-1 and controls its nuclear translocation (Porstmann et al., 2008; Duvel et al., 2010; Chakrabarti and Kandror, 2015). In addition, adipogenesis independent of mTORC1 has also been shown to be controlled by mTORC2, which phosphorylates AKT, which targets ATP-citrate lyase as a distinct substrate, thereby driving brown adipogenesis and de novo lipogenesis (Calejman et al., 2020).
AMPK, containing a catalytic subunit (α) and two regulatory subunits (β and γ), is an upstream kinase of mTOR, and the tumor-suppressor protein liver kinase B1 (LKB1) can inhibit the mTORC1 signaling pathway by activating AMPK and TSC2 (Zhao et al., 2019; Sun, 2021) (Figure 2). AMPK activation stimulates pathways that lead to ATP production and that block the synthesis of ATP-consuming factors, such as lipids and cholesterol (Chen et al., 2019; Sun, 2021). In human adipocytes, TNF-α promotes basal glucose uptake and GLUT4 expression through AMPK activation dependent mechanisms (Fernandez-Veledo et al., 2013). Nevertheless, insulin-induced glucose uptake is blocked by AMPK activators, because AMPK stimulation may inhibit glucose transport in insulin-stimulated adipocytes and may inhibit triacylglycerol synthesis to conserve ATP (Fernandez-Veledo et al., 2013). Past studies have shown that once adipocytes differentiate, AMPK activation induces a reduction in the volume of adipocytes by decreasing the activity of enzymes related to triglyceride synthesis, glycerol phosphoryl transferase and acyl CoA (diacylglyceryl transferase) (Habinowski and Witters, 2001). In addition, other studies have suggested that AMPK may have a crucial effect on cell fate determination in human adipose-derived stem cells as a mediator of bone formation and adipogenesis (Fernandez-Veledo et al., 2013). Finally, the Notch signaling pathway has been reported to participate in the regulation of adipogenesis via the mTOR signaling pathway (Song et al., 2015).
MSCs differentiate into osteoblasts, which are involved in bone formation through the synthesis and sedimentation of mineralized extracellular matrix (Su et al., 2010). Mature osteoblasts eventually become osteoblasts and endoosteocytes or disappear due to apoptosis. Bones are important for mammalian survival, calcium and phosphorus metabolism, and energy homeostasis (Scharla, 2020). Osteoporosis and osteoarthritis are two chronic diseases that are associated with imbalances in bone resorption and formation, and mTOR modulation has been reported to be involved in symptom improvements in certain bone diseases [(Lund-Ricard et al., 2020)]. Researches revealed that mTOR regulates various cellular processes such as growth, proliferation, and differentiation [(Lund-Ricard et al., 2020), (Zhao et al., 2020a)]. In many species ranging from Drosophila to humans, the effect of mTOR signaling on regulatory processes has spurred extensive research (Sun and Liu, 2019). Early studies have suggested that mTOR regulates the function of osteoblasts, and that the mTOR/Raptor/S6K1 signaling pathway is essential for the proliferation and differentiation of osteoblasts [(Chen and Long, 2015), (Dai et al., 2017)]. Although the mTOR signaling pathway may influence osteoblast proliferation and differentiation, earlier studies disputed whether blocking the mTOR signaling pathway with rapamycin affects osteogenesis (Singha et al., 2008).
Bone and dentin are derived from stem cells from apical papilla (SCAPs) that are postnatal MSCs with self-renewing abilities and differentiate into osteoblasts/odontoblasts, adipocytes and nerve cells. According to a recent study, inhibition of the PI3K-Akt-mTOR pathway promotes osteogenic/dentine differentiation in SCAPs in vitro and in vivo (Tanaka et al., 2018). Recent studies have shown that osteoblasts derived from vascular smooth muscle cells (VSMCs) and MSCs are modulated by autophagy to promote the transformation of calcification signals in vascular structures (Shanahan, 2013; Lee et al., 2014; Caffarelli et al., 2017; Zhou et al., 2021). With the involvement of autophagic proteins, AMPK activation powerfully links Akt/mTOR-associated autophagy to the osteogenic differentiation of MSCs (Zhou et al., 2021). MiR-100-5p and miR-143-3p are involved in regulating the mTOR signaling pathway and promoting osteogenesis (Cen et al., 2021). Moreover, research has shown that decreasing miR-141 in bone tissue alleviates the negative regulation of its target gene Dlx5, indirectly promoting DLX5-Msx2 dimer formation and Runx2 expression (Liu et al., 2016; Cen et al., 2021).
However, there is mounting evidence that the effect of mTOR-mediated autophagy is destructive in bone formation. Rapamycin can block osteoblast proliferation and differentiation in mic and rats (Isomoto et al., 2007; Singha et al., 2008). In contrast, baicalein can stimulate osteoblast differentiation by activating the mTORC1 signaling pathway (Li et al., 2015). PPARγ strongly inhibited Akt/mTOR/p70S6K activity, resulting in osteoblast differentiation and a reduction in the trabecular number (Shen et al., 2016). And epiregulin can promote osteoblast proliferation, and inhibit cell death induced by dexamethasone by activating the Akt/mTOR and Erk/MAPK (mitogen-activated protein kinase) signaling pathways (Fan et al., 2015; Shen et al., 2016).
Limb skeletal elements develop from cartilage templates in a process called chondrogenesis; during chondrogenesis, the aggregated mesenchymal cells undergo a highly ordered process of proliferation and maturation (Shimizu et al., 2007). Chondrogenesis is a key process in bone formation because endochondral ossification requires the formation of cartilage templates (Montero and Hurle, 2007). Osteoblasts and chondrocytes are the most useful cells in osteogenesis and chondrogenesis, respectively (Umezawa and Akutsu, 2008). Chondrogenesis is a rigorously regulated multistep process that includes recruitment/migration of mesenchymal cells, prechondrogenic coagulation of mesenchymal cells, transition to chondrogenic lineage and chondrogenic differentiation (Kang, 2008). There have been many reports on the regulation of the mTOR signaling pathway in chondrogenesis. For example, blebbisatin induces chondrogenesis by activating the PI3K/PDK1/mTOR/p70S6K pathway (Kim et al., 2017b); Akt activity is critical for chondrogenesis but is regulated by mTORC2. Mechanical stimulation combined with low-intensity pulsed ultrasound (LIPUS) promoted TGFβ1-induced chondrogenesis of bone marrow mesenchymal stem cells through the integrin-mTOR signaling pathway (Xia et al., 2017). The PI3K/Akt/mTOR pathway also plays an important role in the regulation of endometrial mesenchymal stromal cells (eMSCs) chondrogenesis, and fluoride can inhibit proliferation and promote autophagy through the PI3K/Akt/mTOR signaling pathway (Ma et al., 2021).
Osteoclasts are terminal multinucleated cells that are regulated by nuclear factor-activated T cell C1 (NFATc1) and are responsible for bone absorption (Tong et al., 2020). Enhanced osteoclast formation is an important pathological feature of several age-related bone diseases (Zhang et al., 2005). Osteoclasts participate in bone resorption; bone destruction in rheumatoid arthritis (RA) is caused by osteoclasts and multinucleated cells in the mononuclear/macrophage lineage (Kim and Chen, 2004). Furthermore, bone remodeling is usually a dynamic process regulated by both bone resorbing osteoclasts and bone forming osteoblasts (Wu et al., 2022). Calmodulin-dependent kinase II (CaMKII) regulates osteoclast formation, and the increase in intracellular calcium concentration is the basic process that mediates osteoclast formation (Kim and Chen, 2004; Kang et al., 2020). An imbalance between osteoblasts and bone resorbing osteoclasts is at the heart of many bone diseases (Smink et al., 2009). Exogenous hydrogen sulphide (H2S) can promote osteoclast formation by activating the PI3K/AKT/mTOR pathway to down-regulate autophagy (Ma et al., 2020). Similarly, activation of the mTOR signaling is a pivotal player in osteoclast formation induced by Pasteurella multocida toxin (PMT) (Kloos et al., 2015). The activation of mTOR in reponse to overloaded orthopedic force can facilitate the osteoblastic differentiation of MSCs (Tian et al., 2021a). In contrast, inhibition of AMPK/mTOR/ULK1 signaling can suppress the formation of osteoclasts by reducing autophagy in glucose-mediated osteoclasts (Cai et al., 2018).
Myogenesis is a highly regulated multi-step process that refers to the transformation of progenitor cells into multi-nucleated and functional myofibers (Knight and Kothary, 2011). Myogenesis generally occurs in embryonic development or in response to adult muscle damage (Das et al., 2020). In response to adult muscle damage, MSCs differentiate into myoblasts and contribute to muscle tissue homeostasis and regeneration (Shan et al., 2021). Injectable MSCs that at the injured site can differentiate into myogenic cells and further form muscle fibers, are used for the treatment of skeletal muscle injury (Pumberger et al., 2016; Shan et al., 2021). A recent study demonstrated that a ROS-scavenging hydrogel with MSCs facilitates myogenesis to repair injured skeletal muscle, and the gel can enhance MSC proliferation and myogenesis through the PI3K/Akt/mTOR signaling pathway (Shan et al., 2021). Furthermore, mTOR can regulate myogenesis by integrating nutrient availability (Zhang et al., 2016). Abundant evidence has demonstrated that mTOR, as a nutrient sensor, plays a key role in skeletal muscle development (Laplante and Sabatini, 2012). In mice model, deficiency of mTOR alters a series of metabolic statuses of muscles, such as increased basal glucose uptake, impaired redox homeostasis and changed mitochondrial regulation, and then reduces muscle dystrophin content, thereby causing premature death (Risson et al., 2009). Moreover, mTOR can regulate muscle-specific micro-RNAs. For example, mTOR controls miR-1 by affecting the stability of MyoD protein in differentiating myoblasts or regenerating skeletal muscle (Sun et al., 2010). miR-133 and miR-206 are also targets of MyoD and are sensitive to or inhibited by rapamycin during myoblast differentiation (Liu et al., 2007; Williams et al., 2009; Sun et al., 2010). In a rat injury model, the coaction of miR-1, miR-133 and miR-206 mimics can facilitate myogenic differentiation by activating myogenic markers (myoD1, Pax7 and myogenin) (Nakasa et al., 2010). Additionally, mTOR directly affects the expression of miR-17–92 and miR-125b to control skeletal myogenesis (Zhang et al., 2016).
MSCs possess numerous regenerative and immunomodulatory properties (Bottcher et al., 2016). The immunomodulatory properties of MSCs were recognized to play important roles in vitro and in vivo [(Bartholomew et al., 2002), (Refaie et al., 2021)]. MSCs exert immunoregulatory effects on various immune cells in a cell-cell contact or paracrine manner, which in turn affects the migration, proliferation and differentiation of MSCs [(Chen et al., 2022), (Zhao et al., 2020b)]. MSCs produce many different immunomodulators that regulate the immune function of autologous and allogeneic immune cells, including both innate and acquired immune cells (including T and B cells) (Li et al., 2022). In fact, studies have shown that MSCs affect the metabolic phenotype of activated T cells [(Bottcher et al., 2016), (Pollizzi and Powell, 2014)]. mTOR signaling is a key regulator of glycolysis that increases rapidly to activate normal T cells, and MSC-mediated interference of mTOR signaling is consistent with the T-cell-mediated inhibition of MSCs (Peter et al., 2010). It is now well accepted that targeting mTOR exerts both immunosuppressive and immunostimulatory properties [(Jones and Pearce, 2017), (Weichhart et al., 2015)]. In addition, some MSC-mediated metabolic effects, including the inhibition of mTOR, reduction in glycolysis, and promotion of autophagy, are associated with T-cell-mediated memory formation and longevity [(Kesarwani et al., 2014), (Crompton et al., 2015)].
Studies have shown that the mTOR signaling pathway plays a crucial role in traditional T cells and T-RegS-mediated immune function (Liu et al., 2015). Nitric oxide produced by MSCs inhibits T cells by regulating the LKB1-AMPK-mTOR pathway, thereby inhibiting CD25 translation (Yoo et al., 2017). Moreover, the proinflammatory response mediated by T helper 17 (Th17) cells is increased, while the anti-inflammatory effect mediated by regulatory T (Treg) cells is decreased, exacerbating renal tubular epithelial cell injury (Luo et al., 2021). However, there is considerable evidence that MSCs can control Th17 and Treg cell imbalances (Savio-Silva et al., 2020; Song et al., 2020). By interfering with mTOR signaling, MSCs suppress the differentiation of CD4 (+) T cells into Th17 cells and facilitate Treg cell production (Ghannam et al., 2013; Varco-Merth et al., 2022). Although MSCs suppress normal B-cell proliferation, differentiation, and antibody secretion, CCL2 silencing blocks the suppressive effects on B cells in the MSCs of systemic lupus erythematosus (SLE) patients (Che et al., 2014; Yang et al., 2021a). In a recent study, CCL2 deficiency was shown to enhance synonyms B-cell receptor (BCR) signal transduction through the MST1-mTORC1-STAT1 axis, resulting in a decrease in marginal zone (MZ) B cells and an increase in germinal center (GC) B cells (Yang et al., 2021a). In addition, the suppression of mTORC1 can rescue the aberrant changes in MZ and GC B cells in vivo (Yang et al., 2021a).
Healthy ageing is a complex biological process characterized by the gradual accumulation of senescent cells and is characterized by stable cell cycle arrest, resulting in impaired homeostasis, impaired regenerative potential, and a gradual decline in the functions of multiple tissues and organs (Shi et al., 2021). Adult stem cells are pivotal for organ-specific regeneration and self-renewal with advancing age, MSCs have become a dependable cell source for stem cell transplantation and are currently being studies in extensive clinical trials (Zhang et al., 2015). The use of MSCs, particularly BMSCs, has therapeutic potential in the treatment of rheumatic diseases and regenerative medicine. In BMSCs, mTOR signaling plays a key role in skewed differentiation and ageing, but its role in inhibiting MSC differentiation remains controversial (Al-Azab et al., 2020). Furthermore, clinical inhibition of this pathway may treat ageing-related diseases, especially osteoporosis and arthritis (Ganguly et al., 2017). Thus, abnormal activation of mTOR signaling plays a crucial role in the treatment of ageing-related diseases by MSCs (Liu et al., 2011; Gharibi et al., 2014).
The aging of MSCs seriously affects their function in stem cell transplantation therapy. In recent years, inhibiting MSC ageing has become the focus of extensive research. Previous reports have suggested that ascorbic acid and coenzyme Q10 suppress MSC senescence, and high glucose induces MSC ageing through Akt/mTOR signaling (Zhang et al., 2015; Zhang et al., 2017; Yang et al., 2018). Moreover, Indian Hedgehog regulates MSC senescence by modulating the ROS/mTOR/4EBP1 and p70S6K1/2 pathways (Al-Azab et al., 2020). However, there are many ageing-related diseases related to MSCs that are also regulated by the mTOR signaling pathway, such as Alzheimer’s disease (AD), Parkinson’s disease (PD), osteoporosis, atherogenesis and so on.
AD is a complex, heterogeneous and severe neurodegenerative disease that represents a major form of dementia and is characterized by cognitive behavioral impairment, psychiatric symptoms, progressive cognitive decline, disorientation, behavioral changes and death (Nikolac Perkovic and Pivac, 2019). AD is the most common form of dementia and has huge socio‐economic impacts worldwide (Dong et al., 2019). AD has been historically considered as a disease of gray matter, but accumulating evidence has suggested that white matter alteration occurs in the disease course (Sachdev et al., 2013). MSCs have received much attention for their potential in regenerative medicine, and they offer new hope as a therapeutic strategy for neurodegenerative disease treatment, including AD (Divya et al., 2012; Farahzadi et al., 2020). New studies have shown that multiple signaling pathways are involved in the pathophysiology of AD, the most important of which include mTOR, AMPK, glycogen synthase kinase 3 (GSK3), and Wnt3/β-catenin (Godoy et al., 2014; Tramutola et al., 2015). Therefore, the effect of MSCs on nerve cells as an AD treatment may be largely related to the mTOR signal transduction network. In addition, autophagy has been shown to reduce the proliferation of BMSCs treated with amyloid-β1-42 (Aβ1-42) through the Akt/mTOR signaling pathway (Yang et al., 2019).
PD is an ageing-related neurodegenerative disorder characterized by the loss of dopaminergic neurons in the substantia nigra midbrain region and the presence of intracytoplasmic inclusions called Lewy bodies (Dexter and Jenner, 2013; Anderson and Maes, 2014; Safari et al., 2016). When nearly 60% of dopaminergic neurons in the aubstantia nigra dense region are eliminated, Parkinsonian symptoms begin to appear (Cooper et al., 2009). The occurrence of PD is related to many factors, such as ageing and oxidative stress (Ma et al., 2022). It is estimated that approximately 12% of people 65 and older have PD (Lan et al., 2017). In advanced PD patients, the glutathione level is lower than that in the age‐matched control group, which suggests that the disease course may be involved in the vulnerability of the substantia nigra pars compacta (SNc) to oxidative stress (Salaramoli et al., 2022). In previous studies, the combination of granulocyte colony-stimulating factor and BMSCs has beneficial effects on a PD model (Ghahari et al., 2020). There have been few reports of the direct involvement of MSCs and regulation of the mTOR signaling pathway in PD, but increasing evidence indicates that mTOR plays key roles in the pathogenesis of PD (Zhu et al., 2019). The mTOR protein, which is a key regulator of cell metabolism and survival, has emerged as a novel therapeutic target for PD.
Osteoporosis is a major risk cause of fracture or broken bones in later life (Otonari et al., 2021). In older individuals, the rate of bone resorption exceeds the rate of bone formation, resulting in bone loss (Owen-Woods and Kusumbe, 2022). Osteoporosis is a major public health problem and a heterogeneous disease with significant socioeconomic importance because it is associated with fracture and appropriate early intervention can greatly alleviate the problem before fracture occurs (Pietschmann and Peterlik, 1999; Jordan and Cooper, 2002). The decline in MSC proliferation and stem cell properties with age is also thought to account for the gradual decrease in bone mass and reduced risk of osteoporosis and fracture in older adults (Bellantuono et al., 2009).
BMSCs isolated from elderly individuals or aged animals exhibit decreased bone marrow frequencies and proliferation rates and higher levels of ageing and ageing-related changes than young cells (Gharibi et al., 2014). It has been reported that activation/inhibition of mTOR signaling positively/negatively regulates BMSC/osteoblast-mediated bone formation, adipogenic differentiation, osteoblast homeostasis, and osteoclast mediated bone resorption, leading to altered bone homeostasis, which can lead to or prevent osteoporosis (Shen et al., 2018). Other studies have shown that amyloid β induces osteoporotic defects in vivo and in vitro through mTOR and autophagy, and the regulation of amyloid β on BMSCs is dependent on mTOR, thus providing a possible mechanism for osteoporotic remodeling in AD patients (Yang et al., 2019; Lin et al., 2021).
Atherosclerosis is a common age-related disease, and increasing age is one of the major risk factors for developing atherosclerosis (Wang and Bennett, 2012). One of the phenotypic manifestations of the arteries is atherosclerosis, which is a chronic inflammatory disease (Poznyak et al., 2022). Primary atherosclerosis may be a phenotypic feature of mitochondrial disease. It has been suggested that atherosclerosis may be a major manifestation of metabolic defects (Finsterer, 2020). In many cases, atherosclerosis is the root cause of vascular disease, including heart disease and stroke (Kazemi-Bajestani and Ghayour-Mobarhan, 2013). Previous studies have shown that mesenchymal stem cells (MSCs) have therapeutic effects on a variety of diseases, including atherosclerosis (Yang et al., 2021b). Maldifferentiation of mesenchymal stem cells and maladjustment of cell fate programs associated with age and metabolic diseases may exacerbate arteriosclerosis due to excessive transformation into osteoblast-like calcified cells (Schaub et al., 2019). As a chronic vascular inflammatory disease, atherosclerosis has been demonstrated to exert immunomodulatory and immunosuppressive effects on MSCs by secreting humoral factors (Takafuji et al., 2019). It has been reported that Sestrin2 is a stress-inducing protein that inhibits mTOR by activating AMPK (Tian et al., 2021b). Furthermore, Sestrin2 is strongly associated with atherosclerosis, suggesting that inhibition of mTOR signaling can be used to treat atherosclerosis (Tian et al., 2021b). In other words, mTOR is a target for the treatment of atherosclerosis.
The mTOR signaling pathway is involved in the development of many human diseases, and its dysregulation has been reported in several pathological processes, especially in age-related human diseases and in mouse models of accelerated aging (Lee et al., 2020). In addition to the specific diseases mentioned above, mTOR signaling is also closely related to the occurrence and development of many major diseases related to aging, such as cardiovascular diseases and bone disease (He and Liu, 2014). In the cardiovascular system, the mTOR signaling can regulate angiogenesis (Samidurai et al., 2018; Liu et al., 2022). Angiogenesis depends on the full function of vascular smooth muscle cell progenitors such as pericytes and their circulating counterparts mesenchymal stromal cells (Schaub et al., 2019). MSCs are located around blood vessels, and hematopoietic stem cells (HSCs) and MSCs occupy special microenvironments (Owen-Woods and Kusumbe, 2022). Blood vessel wear is a precursor to aging, and loss of vascular density and pericytes is a sign of aging of organs and tissues (Chen et al., 2021a). Therefore, vascular vessels and microenvironments provide secretory signals to affect cell function in age-related diseases, except regulating organ development and stem cell behavior (Chen et al., 2021b; Owen-Woods and Kusumbe, 2022).
Moreover, the Notch pathway is one of several pro-proliferative pathways activated by mTOR (Ivanovska et al., 2017). Notch signaling which negatively controls angiogenesis, is an important component of crosstalk between vascular cells and bone lineage cells in the process of bone formation and remodeling (Chen et al., 2020). Activation of the VEGF-Notch signaling pathway may restore the proliferation of MSCs in patients with aplastic anemia (Deng et al., 2019). Anyhow, crosstalk between the Notch and mTOR pathways may play important roles in MSC-mediated senile diseases. In addition, bone mesenchymal cells also undergo age-dependent alterations (Chen et al., 2020). In osteoarthritis patients, TGF-β1 and mTOR are highly expressed, and blocking TGF-β signaling in the MSCs of subchondral bone deadens osteoarthritis (Zhen et al., 2013; Owen-Woods and Kusumbe, 2022). In mice model, osteoarthritis was induced by TGF-β1 overexpression, which resulted in increased mTOR expression (Davidson et al., 2007; Wen et al., 2021; Owen-Woods and Kusumbe, 2022). In addition, previous studies have revealed that PI3K/AKT/mTOR signaling is involved in the important regulation of ischemic brain injury and tumors (Xu et al., 2020).
We summarized relevant studies on the involvement of mTOR in the differentiation of adipocytes, bone and muscle. We found that the mTOR signaling pathway in MSCs is crucial. Inhibition or activation of this pathway can affect cell differentiation of MSCs. In addition, there are many mTOR related signaling pathways that have a certain impact on the immune response, and regulate angiogenesis, such as the Notch pathway. Therefore, in the future, the influences caused by mTOR may be regulated by other pathways. What are the difficulties in identifying these new approaches? What are the disadvantages of these new pathways compared to the mTOR pathway? Is the activation time of other regulatory pathways different from the time or stage of activation of the mTOR signaling pathway? Is it cost-effective to regulate MSC differentiation through these new pathways? These questions are all worth considering.
In recent years, there has been a sharp increase in the number of articles on the activation and inhibition of the mTOR signaling pathway, and many researchers have focused on the development of innovative inhibitors. Most of the reported inhibitors target mTORC1 or PI3K and mTOR dual inhibitors, including competitive inhibitors and allosteric inhibitors. The discovery of rapamycin-insensitive mTORC2 stimulated the development of mTOR inhibitors that target the kinase domain (el Hage and Dormond, 2021). However, we need to identify more effective activators that target positively regulated pathways. Therefore, we can attempt to develop new inhibitors that negatively regulate pathways or activators that positively regulate pathways by exploring the relevant mechanisms of mTORC2. Perhaps we can find more effective inhibitors or activators by examining other upstream or downstream factors in the mTOR signaling pathway. Moreover, although senile diseases are a difficult problem facing society, there have been few studies on MSCs or the regulation of the mTOR signaling pathway. In particular, research on PD is scarce, but it is a typical disease worthy of further study. Therefore, there is still considerable space and prospects in this field, which is worthy of further exploration. We believe that these problems will be solved in the future, so the mTOR signaling pathway is still worthy of investigation.
Conceptualization, HC and ZW; Writing—original draft preparation, HC, ZW, and WT; Writing—review and editing, EZ and XK All authors have read and agreed to the published version of the manuscript.
This work was supported by the Graduate Scientific Research Innovation Project of Chongqing (CYS22255), the Natural Science Foundation of Chongqing (cstc2022ycjh-bgzxm0113).
We thank Kui Zhang, Ruichen Liu and Longfei Deng for their help. Moreover, certain elements of Figure 2 were from BioRender.com.
The authors declare that the research was conducted in the absence of any commercial or financial relationships that could be construed as a potential conflict of interest.
All claims expressed in this article are solely those of the authors and do not necessarily represent those of their affiliated organizations, or those of the publisher, the editors and the reviewers. Any product that may be evaluated in this article, or claim that may be made by its manufacturer, is not guaranteed or endorsed by the publisher.
Al-Azab, M., Wang, B., Elkhider, A., Walana, W., Li, W., Yuan, B., et al. (2020). Indian Hedgehog regulates senescence in bone marrow-derived mesenchymal stem cell through modulation of ROS/mTOR/4EBP1, p70S6K1/2 pathway. Aging 12 (7), 5693–5715. doi:10.18632/aging.102958
Anderson, G., and Maes, M. (2014). Neurodegeneration in Parkinson's disease: Interactions of oxidative stress, tryptophan catabolites and depression with mitochondria and sirtuins. Mol. Neurobiol. 49 (2), 771–783. doi:10.1007/s12035-013-8554-z
Andrzejewska, A., Lukomska, B., and Janowski, M. (2019). Concise review: Mesenchymal stem cells: From roots to boost. Stem Cells 37 (7), 855–864. doi:10.1002/stem.3016
Bartholomew, A., Sturgeon, C., Siatskas, M., Ferrer, K., McIntosh, K., Patil, S., et al. (2002). Mesenchymal stem cells suppress lymphocyte proliferation in vitro and prolong skin graft survival in vivo. Exp. Hematol. 30 (1), 42–48. doi:10.1016/s0301-472x(01)00769-x
Bellantuono, I., Aldahmash, A., and Kassem, M. (2009). Aging of marrow stromal (skeletal) stem cells and their contribution to age-related bone loss. Biochim. Biophys. Acta 1792 (4), 364–370. doi:10.1016/j.bbadis.2009.01.008
Blocki, A., Wang, Y., Koch, M., Peh, P., Beyer, S., Law, P., et al. (2013). Not all MSCs can act as pericytes: Functional in vitro assays to distinguish pericytes from other mesenchymal stem cells in angiogenesis. Stem Cells Dev. 22 (17), 2347–2355. doi:10.1089/scd.2012.0415
Bottcher, M., Hofmann, A. D., Bruns, H., Haibach, M., Loschinski, R., Saul, D., et al. (2016). Mesenchymal stromal cells disrupt mTOR-signaling and aerobic glycolysis during T-cell activation. Stem Cells 34 (2), 516–521. doi:10.1002/stem.2234
Brunn, G. J., Hudson, C. C., Sekulic, A., Williams, J. M., Hosoi, H., Houghton, P. J., et al. (1997). Phosphorylation of the translational repressor PHAS-I by the mammalian target of rapamycin. Science 277 (5322), 99–101. doi:10.1126/science.277.5322.99
Butt, G., Shahwar, D., Qureshi, M. Z., Attar, R., Akram, M., Birinci, Y., et al. (2019). Role of mTORC1 and mTORC2 in breast cancer: Therapeutic targeting of mTOR and its partners to overcome metastasis and drug resistance. Adv. Exp. Med. Biol. 1152, 283–292. doi:10.1007/978-3-030-20301-6_152nd Edition
Caffarelli, C., Montagnani, A., Nuti, R., and Gonnelli, S. (2017). Bisphosphonates, atherosclerosis and vascular calcification: Update and systematic review of clinical studies. Clin. Interv. Aging 12, 1819–1828. doi:10.2147/CIA.S138002
Cai, Z. Y., Yang, B., Shi, Y. X., Zhang, W. L., Liu, F., Zhao, W., et al. (2018). High glucose downregulates the effects of autophagy on osteoclastogenesis via the AMPK/mTOR/ULK1 pathway. Biochem. Biophys. Res. Commun. 503 (2), 428–435. doi:10.1016/j.bbrc.2018.04.052
Calejman, C. M., Trefely, S., Entwisle, S. W., LuciAno, A., Jung, S. M., Hsiao, W., et al. (2020). Author correction: mTORC2-AKT signaling to ATP-citrate lyase drives brown adipogenesis and de novo lipogenesis. Nat. Commun. 11 (1), 4585. doi:10.1038/s41467-020-18510-9
Caplan, A. I. (2008). All MSCs are pericytes? Cell Stem Cell 3 (3), 229–230. doi:10.1016/j.stem.2008.08.008
Caron, A., Richard, D., and Laplante, M. (2015). The roles of mTOR complexes in lipid metabolism. Annu. Rev. Nutr. 3535, 321–348. doi:10.1146/annurev-nutr-071714-034355
Cen, X., Pan, X., Zhang, B., Huang, W., Xiong, X., Huang, X., et al. (2021). Mechanosensitive non-coding RNAs in osteogenesis of mesenchymal stem cells. Cell Transplant. 30.
Chakrabarti, P., and Kandror, K. V. (2015). The role of mTOR in lipid homeostasis and diabetes progression. Curr. Opin. Endocrinol. Diabetes Obes. 22 (5), 340–346. doi:10.1097/MED.0000000000000187
Che, N., Li, X., Zhang, L., Liu, R., Chen, H., Gao, X., et al. (2014). Impaired B cell inhibition by lupus bone marrow mesenchymal stem cells is caused by reduced CCL2 expression. J. Immunol. 193 (10), 5306–5314. doi:10.4049/jimmunol.1400036
Chen, J. Q., and Long, F. X. (2015). mTORC1 signaling promotes osteoblast differentiation from preosteoblasts. Plos One 10 (6), e0130627. doi:10.1371/journal.pone.0130627
Chen, J. Y., Hendriks, M., Chatzis, A., Ramasamy, S. K., and Kusumbe, A. P. (2020). Bone vasculature and bone marrow vascular niches in health and disease. J. Bone Min. Res. 35 (11), 2103–2120. doi:10.1002/jbmr.4171
Chen, J. Y., Lippo, L., Labella, R., Tan, S. L., Marsden, B. D., Dustin, M. L., et al. (2021). Decreased blood vessel density and endothelial cell subset dynamics during ageing of the endocrine system. Embo J. 40 (1), e105242. doi:10.15252/embj.2020105242
Chen, J. Y., Sivan, U., Tan, S. L., Lippo, L., De Angelis, J., Labella, R., et al. (2021). High-resolution 3D imaging uncovers organ-specific vascular control of tissue aging. Sci. Adv. 7 (6), eabd7819. doi:10.1126/sciadv.abd7819
Chen, K., Chen, X., Xue, H., Zhang, P., Fang, W., Chen, X., et al. (2019). Coenzyme Q10 attenuates high- fat diet- induced non- alcoholic fatty liver disease through activation of the AMPK pathway. Food Funct. 10 (2), 814–823. doi:10.1039/c8fo01236a
Chen, R., Hao, Z., Wang, Y., Zhu, H., Hu, Y., Chen, T., et al. (2022). Mesenchymal stem cell-immune cell interaction and related modulations for bone tissue engineering. Stem Cells Int. 2022, 7153584. doi:10.1155/2022/7153584
Chiarini, F., Evangelisti, C., Lattanzi, G., McCubrey, J. A., and Martelli, A. M. (2019). Advances in understanding the mechanisms of evasive and innate resistance to mTOR inhibition in cancer cells. Biochim. Biophys. Acta. Mol. Cell Res. 1866 (8), 1322–1337. doi:10.1016/j.bbamcr.2019.03.013
Ciolczyk-Wierzbicka, D., Gil, D., Zarzycka, M., and Laidler, P. (2020). mTOR inhibitor everolimus reduces invasiveness of melanoma cells. Hum. Cell 33 (1), 88–97. doi:10.1007/s13577-019-00270-4
Cooper, O., Astradsson, A., Hallett, P., Robertson, H., Mendez, I., and Isacson, O. (2009). Lack of functional relevance of isolated cell damage in transplants of Parkinson's disease patients. J. Neurol. 256, S310–S316. doi:10.1007/s00415-009-5242-z
Crompton, J. G., Sukumar, M., Roychoudhuri, R., Clever, D., Gros, A., Eil, R. L., et al. (2015). Akt inhibition enhances expansion of potent tumor-specific lymphocytes with memory cell characteristics. Cancer Res. 75 (2), 296–305. doi:10.1158/0008-5472.CAN-14-2277
Dai, Q. G., Xu, Z., Ma, X., Niu, N., Zhou, S., Xie, F., et al. (2017). mTOR/Raptor signaling is critical for skeletogenesis in mice through the regulation of Runx2 expression. Cell Death Differ. 24 (11), 1886–1899. doi:10.1038/cdd.2017.110
Das, A., Das, D., Abdelmohsen, K., and Panda, A. C. (2020). Circular RNAs in myogenesis. Biochim. Biophys. Acta. Gene Regul. Mech. 1863 (4), 194372. doi:10.1016/j.bbagrm.2019.02.011
Davidson, E. N. B., van der Kraan, P. M., and van den Berg, W. B. (2007). TGF-beta and osteoarthritis. Osteoarthr. Cartil. 15 (6), 597–604. doi:10.1016/j.joca.2007.02.005
Deng, S., Xiang, J. J., Shen, Y. Y., Lin, S. Y., Zeng, Y. Q., and Shen, J. P. (2019). Effects of VEGF-notch signaling pathway on proliferation and apoptosis of bone marrow MSC in patients with aplastic anemia. Zhongguo Shi Yan Xue Ye Xue Za Zhi 27 (6), 1925–1932. doi:10.19746/j.cnki.issn.1009-2137.2019.06.035
Dexter, D. T., and Jenner, P. (2013). Parkinson disease: From pathology to molecular disease mechanisms. Free Radic. Biol. Med. 62, 132–144. doi:10.1016/j.freeradbiomed.2013.01.018
Ding, J., Li, T., Wang, X., Zhao, E., Choi, J. H., Yang, L., et al. (2013). The histone H3 methyltransferase G9A epigenetically activates the serine-glycine synthesis pathway to sustain cancer cell survival and proliferation. Cell Metab. 18 (6), 896–907. doi:10.1016/j.cmet.2013.11.004
Divya, M. S., Roshin, G. E., Divya, T. S., Rasheed, V. A., Santhoshkumar, T. R., Elizabeth, K. E., et al. (2012). Umbilical cord blood-derived mesenchymal stem cells consist of a unique population of progenitors co-expressing mesenchymal stem cell and neuronal markers capable of instantaneous neuronal differentiation. Stem Cell Res. Ther. 3, 57. doi:10.1186/scrt148
Dong, R., Wang, H., Ye, J., Wang, M., and Bi, Y. (2019). Publication trends for Alzheimer's disease worldwide and in China: A 30-year bibliometric analysis. Front. Hum. Neurosci. 13, 259. doi:10.3389/fnhum.2019.00259
Duvel, K., Yecies, J. L., Menon, S., Raman, P., Lipovsky, A. I., Souza, A. L., et al. (2010). Activation of a metabolic gene regulatory network downstream of mTOR complex 1. Mol. Cell 39 (2), 171–183. doi:10.1016/j.molcel.2010.06.022
el Hage, A., and Dormond, O. (2021). Combining mTOR inhibitors and T cell-based immunotherapies in cancer treatment. Cancers 13 (6), 1359. doi:10.3390/cancers13061359
Fan, J. B., Liu, W., Zhu, X. H., Yuan, K., Xu, D. W., Chen, J. J., et al. (2015). EGFR-AKT-mTOR activation mediates epiregulin-induced pleiotropic functions in cultured osteoblasts. Mol. Cell. Biochem. 398 (1-2), 105–113. doi:10.1007/s11010-014-2210-4
Farahzadi, R., Fathi, E., and Vietor, I. (2020). Mesenchymal stem cells could Be considered as a candidate for further studies in cell-based therapy of Alzheimer's disease via targeting the signaling pathways. ACS Chem. Neurosci. 11 (10), 1424–1435. doi:10.1021/acschemneuro.0c00052
Feng, Z., Zhang, H., Levine, A. J., and Jin, S. (2005). The coordinate regulation of the p53 and mTOR pathways in cells. Proc. Natl. Acad. Sci. U. S. A. 102 (23), 8204–8209. doi:10.1073/pnas.0502857102
Fernandez-Veledo, S., Vazquez-Carballo, A., Vila-Bedmar, R., Ceperuelo-Mallafre, V., and Vendrell, J. (2013). Role of energy- and nutrient-sensing kinases AMP-activated protein kinase (AMPK) and mammalian target of rapamycin (mTOR) in adipocyte differentiation. Iubmb Life 65 (7), 572–583. doi:10.1002/iub.1170
Finsterer, J. (2020). Atherosclerosis can Be mitochondrial: A review. Cureus 12 (2), e6987. doi:10.7759/cureus.6987
Ganguly, P., El-Jawhari, J. J., Giannoudis, P. V., Burska, A. N., Ponchel, F., and Jones, E. A. (2017). Age-related changes in bone marrow mesenchymal stromal cells: A potential impact on osteoporosis and osteoarthritis development. Cell Transpl. 26 (9), 1520–1529. doi:10.1177/0963689717721201
Ghahari, L., Safari, M., Rahimi Jaberi, K., Jafari, B., Safari, K., and Madadian, M. (2020). Mesenchymal stem cells with granulocyte colony-stimulating factor reduce stress oxidative factors in Parkinson's disease. Iran. Biomed. J. 24 (2), 89–98. doi:10.29252/ibj.24.2.89
Ghannam, S., Pene, J., Moquet-Torcy, G., Jorgensen, C., and Yssel, H. (2013). Correction: Mesenchymal stem cells inhibit human Th17 cell differentiation and function and induce a T regulatory cell phenotype. J. I. 191 (11), 5777. doi:10.4049/jimmunol.1390061
Gharibi, B., Farzadi, S., Ghuman, M., and Hughes, F. J. (2014). Inhibition of akt/mTOR attenuates age-related changes in mesenchymal stem cells. Stem Cells 32 (8), 2256–2266. doi:10.1002/stem.1709
Godoy, J. A., Rios, J. A., Zolezzi, J. M., Braidy, N., and Inestrosa, N. C. (2014). Signaling pathway cross talk in Alzheimer's disease. Cell Commun. Signal. 12, 23. doi:10.1186/1478-811X-12-23
Habinowski, S. A., and Witters, L. A. (2001). The effects of AICAR on adipocyte differentiation of 3T3-L1 cells. Biochem. Biophys. Res. Commun. 286 (5), 852–856. doi:10.1006/bbrc.2001.5484
Harwood, F. C., Klein Geltink, R. I., O'Hara, B. P., Cardone, M., Janke, L., Finkelstein, D., et al. (2018). ETV7 is an essential component of a rapamycin-insensitive mTOR complex in cancer. Sci. Adv. 4 (9), eaar3938. doi:10.1126/sciadv.aar3938
He, J. Y., and Liu, F. (2014). mTOR signaling in aging and aging-associated diseases. Prog. Biochem. Biophysics 41 (3), 257–265.
Holz, M. K., Ballif, B. A., Gygi, S. P., and Blenis, J. (2005). mTOR and S6K1 mediate assembly of the translation preinitiation complex through dynamic protein interchange and ordered phosphorylation events. Cell 123 (4), 569–580. doi:10.1016/j.cell.2005.10.024
Holz, M. K., Ballif, B. A., Gygi, S. P., and Blenis, J. (2021). mTOR and S6K1 mediate assembly of the translation preinitiation complex through dynamic protein interchange and ordered phosphorylation events. Cell 184 (8), 2255. doi:10.1016/j.cell.2021.03.060
Huang, S. (2020). mTOR signaling in metabolism and cancer. Cells 9 (10), E2278. doi:10.3390/cells9102278
Isomoto, S., Hattori, K., Ohgushi, H., Nakajima, H., Tanaka, Y., and Takakura, Y. (2007). Rapamycin as an inhibitor of osteogenic differentiation in bone marrow-derived mesenchymal stem cells. J. Orthop. Sci. 12 (1), 83–88. doi:10.1007/s00776-006-1079-9
Ivanovska, J., Shah, S., Wong, M. J., Kantores, C., Jain, A., Post, M., et al. (2017). mTOR-Notch3 signaling mediates pulmonary hypertension in hypoxia-exposed neonatal rats independent of changes in autophagy. Pediatr. Pulmonol. 52 (11), 1443–1454. doi:10.1002/ppul.23777
Jones, R. G., and Pearce, E. J. (2017). MenTORing immunity: mTOR signaling in the development and function of tissue-resident immune cells. Immunity 46 (5), 730–742. doi:10.1016/j.immuni.2017.04.028
Jordan, K. M., and Cooper, C. (2002). Epidemiology of osteoporosis. Best. Pract. Res. Clin. Rheumatol. 16 (5), 795–806. doi:10.1053/berh.2002.0264
Julien, L. A., and Roux, P. P. (2010). mTOR, the mammalian target of rapamycin. Med. Sci. 26 (12), 1056–1060. doi:10.1051/medsci/201026121056
Kang, J. Y., Kang, N., Yang, Y. M., Hong, J. H., and Shin, D. M. (2020). The role of Ca2+-NFATc1 signaling and its modulation on osteoclastogenesis. Int. J. Mol. Sci. 21 (10), E3646. doi:10.3390/ijms21103646
Kang, S. S. (2008). Regulation of early steps of chondrogenesis in the developing limb. Animal Cells Syst. 12 (1), 1–9. doi:10.1080/19768354.2008.9647147
Karagianni, F., Pavlidis, A., Malakou, L. S., Piperi, C., and Papadavid, E. (2022). Predominant role of mTOR signaling in skin diseases with therapeutic potential. Int. J. Mol. Sci. 23 (3), 1693. doi:10.3390/ijms23031693
Kazemi-Bajestani, S. M., and Ghayour-Mobarhan, M. (2013). Concept of atherosclerosis velocity: Is it a better measure of cardiovascular risk? Iran. J. Med. Sci. 38 (3), 210–220.
Kesarwani, P., Al-Khami, A. A., Scurti, G., Thyagarajan, K., Kaur, N., Husain, S., et al. (2014). Promoting thiol expression increases the durability of antitumor T-cell functions. Cancer Res. 74 (21), 6036–6047. doi:10.1158/0008-5472.CAN-14-1084
Kim, H., Kim, D. H., Jeong, B., Kim, J. H., Lee, S. R., and Sonn, J. K. (2017). Blebbistatin induces chondrogenesis of single mesenchymal cells via PI3K/PDK1/mTOR/p70S6K pathway. Biologia 72 (6), 694–701. doi:10.1515/biolog-2017-0078
Kim, J. E., and Chen, J. (2004). Regulation of peroxisome proliferator-activated receptor-gamma activity by mammalian target of rapamycin and amino acids in adipogenesis. Diabetes 53 (11), 2748–2756. doi:10.2337/diabetes.53.11.2748
Kim, J. O., Kim, K. H., Song, I. S., Cheon, K. S., Kim, O. H., Lee, S. C., et al. (2017). Potentiation of the anticancer effects of everolimus using a dual mTORC1/2 inhibitor in hepatocellular carcinoma cells. Oncotarget 8 (2), 2936–2948. doi:10.18632/oncotarget.13808
Kloos, B., Chakraborty, S., Lindner, S. G., Noack, K., Harre, U., Schett, G., et al. (2015). Pasteurella multocida toxin-induced osteoclastogenesis requires mTOR activation. Cell Commun. Signal. 13, 40. doi:10.1186/s12964-015-0117-7
Knight, J. D. R., and Kothary, R. (2011). The myogenic kinome: Protein kinases critical to mammalian skeletal myogenesis. Skelet. Muscle 1.
Lan, A. P., Chen, J., Zhao, Y., Chai, Z., and Hu, Y. (2017). mTOR signaling in Parkinson's disease. Neuromolecular Med. 19 (1), 1–10. doi:10.1007/s12017-016-8417-7
Laplante, M., and Sabatini, D. M. (2012). mTOR signaling in growth control and disease. Cell 149 (2), 274–293. doi:10.1016/j.cell.2012.03.017
Lee, J. H., Lee, S. H., Lee, H. S., Ji, S. T., Jung, S. Y., Kim, J. H., et al. (2016). Lnk is an important modulator of insulin-like growth factor-1/Akt/peroxisome proliferator-activated receptor-gamma axis during adipogenesis of mesenchymal stem cells. Korean J. Physiol. Pharmacol. 20 (5), 459–466. doi:10.4196/kjpp.2016.20.5.459
Lee, J. Y., Kennedy, B. K., and Liao, C. Y. (2020). Mechanistic target of rapamycin signaling in mouse models of accelerated aging. J. Gerontol. A Biol. Sci. Med. Sci. 75 (1), 64–72. doi:10.1093/gerona/glz059
Lee, K., Kim, H., and Jeong, D. (2014). Microtubule stabilization attenuates vascular calcification through the inhibition of osteogenic signaling and matrix vesicle release. Biochem. Biophys. Res. Commun. 451 (3), 436–441. doi:10.1016/j.bbrc.2014.08.007
Li, H. F., Tang, J. j., Chen, J., Zhang, P., Wang, T., Chen, T. y., et al. (2015). Regulation of bone formation by baicalein via the mTORC1 pathway. Drug Des. devel. Ther. 9, 5169–5183. doi:10.2147/DDDT.S81578
Li, X. L., Guan, Y., Li, C., Zhang, T., Meng, F., Zhang, J., et al. (2022). Immunomodulatory effects of mesenchymal stem cells in peripheral nerve injury. Stem Cell Res. Ther. 13 (1), 18. doi:10.1186/s13287-021-02690-2
Lin, Y., Chen, T., Chen, J., Fang, Y., and Zeng, C. (2021). Endogenous Aβ induces osteoporosis through an mTOR-dependent inhibition of autophagy in bone marrow mesenchymal stem cells (BMSCs). Ann. Transl. Med. 9 (24), 1794. doi:10.21037/atm-21-6427
Liu, C. H., Chapman, N. M., Karmaus, P. W. F., Zeng, H., and Chi, H. (2015). mTOR and metabolic regulation of conventional and regulatory T cells. J. Leukoc. Biol. 97 (5), 837–847. doi:10.1189/jlb.2RI0814-408R
Liu, G. Y., and Sabatini, D. M. (2020). Author correction: mTOR at the nexus of nutrition, growth, ageing and disease. Nat. Rev. Mol. Cell Biol. 21 (4), 246. doi:10.1038/s41580-020-0219-y
Liu, N., Williams, A. H., Kim, Y., McAnally, J., Bezprozvannaya, S., Sutherland, L. B., et al. (2007). An intragenic MEF2-dependent enhancer directs muscle-specific expression of microRNAs 1 and 133. Proc. Natl. Acad. Sci. U. S. A. 104 (52), 20844–20849. doi:10.1073/pnas.0710558105
Liu, R. S., Li, B., Li, W. D., Du, X. L., and Li, X. Q. (2022). miRNA-130 promotes migration and angiogenesis of endothelial progenitor cells through PI3K/AKT/mTOR pathways. J. Biomater. tissue Eng. 12 (1), 206–214. doi:10.1166/jbt.2022.2658
Liu, S., Liu, S., Wang, X. Y., Zhou, J. X., Cao, Y. J., Wang, F., et al. (2011). The PI3K-Akt pathway inhibits senescence and promotes self-renewal of human skin-derived precursors in vitro. Aging Cell 10 (4), 661–674. doi:10.1111/j.1474-9726.2011.00704.x
Liu, Y., Kou, X., Chen, C., Yu, W., Su, Y., Kim, Y., et al. (2016). Chronic high dose alcohol induces osteopenia via activation of mTOR signaling in bone marrow mesenchymal stem cells. Stem Cells 34 (8), 2157–2168. doi:10.1002/stem.2392
Loewith, R., Jacinto, E., Wullschleger, S., Lorberg, A., Crespo, J. L., Bonenfant, D., et al. (2002). Two TOR complexes, only one of which is rapamycin sensitive, have distinct roles in cell growth control. Mol. Cell 10 (3), 457–468. doi:10.1016/s1097-2765(02)00636-6
Lund-Ricard, Y., Cormier, P., Morales, J., and Boutet, A. (2020). mTOR signaling at the crossroad between metazoan regeneration and human diseases. Int. J. Mol. Sci. 21 (8), E2718. doi:10.3390/ijms21082718
Luo, Y., Liu, L., Wu, Y., Singh, K., Su, B., Zhang, N., et al. (2015). Rapamycin inhibits mSin1 phosphorylation independently of mTORC1 and mTORC2. Oncotarget 6 (6), 4286–4298. doi:10.18632/oncotarget.3006
Luo, Y. S., Guo, J., Zhang, P., Cheuk, Y. C., Jiang, Y., Wang, J., et al. (2021). Mesenchymal stem cell protects injured renal tubular epithelial cells by regulating mTOR-mediated Th17/treg Axis. Front. Immunol. 12, 684197. doi:10.3389/fimmu.2021.684197
Luzzani, C. D., and Miriuka, S. G. (2017). Pluripotent stem cells as a robust source of mesenchymal stem cells. Stem Cell Rev. Rep. 13 (1), 68–78. doi:10.1007/s12015-016-9695-z
Ma, J., Du, D., Liu, J., Guo, L., Li, Y., Chen, A., et al. (2020). Hydrogen sulphide promotes osteoclastogenesis by inhibiting autophagy through the PI3K/AKT/mTOR pathway. J. Drug Target. 28 (2), 176–185. doi:10.1080/1061186X.2019.1624969
Ma, J. J., Shi, X., Li, M., Chen, S., Gu, Q., Zheng, J., et al. (2022). MicroRNA-181a-2-3p shuttled by mesenchymal stem cell-secreted extracellular vesicles inhibits oxidative stress in Parkinson's disease by inhibiting EGR1 and NOX4. Cell Death Discov. 8 (1), 33. doi:10.1038/s41420-022-00823-x
Ma, L., Zhang, R., Li, D., Qiao, T., and Guo, X. (2021). Fluoride regulates chondrocyte proliferation and autophagy via PI3K/AKT/mTOR signaling pathway. Chem. Biol. Interact., 109659. doi:10.1016/j.cbi.2021.109659
Mezzaroba, L., Alfieri, D. F., Colado Simao, A. N., and Vissoci Reiche, E. M. (2019). The role of zinc, copper, manganese and iron in neurodegenerative diseases. Neurotoxicology 74, 230–241. doi:10.1016/j.neuro.2019.07.007
Montero, J. A., and Hurle, J. M. (2007). Deconstructing digit chondrogenesis. Bioessays 29 (8), 725–737. doi:10.1002/bies.20607
Murugan, A. K. (2019). mTOR: Role in cancer, metastasis and drug resistance. Semin. Cancer Biol. 59, 92–111. doi:10.1016/j.semcancer.2019.07.003
Nakasa, T., Ishikawa, M., Shi, M., Shibuya, H., Adachi, N., and Ochi, M. (2010). Acceleration of muscle regeneration by local injection of muscle-specific microRNAs in rat skeletal muscle injury model. J. Cell. Mol. Med. 14 (10), 2495–2505. doi:10.1111/j.1582-4934.2009.00898.x
Nikolac Perkovic, M., and Pivac, N. (2019). Genetic markers of Alzheimer's disease. Adv. Exp. Med. Biol. 1192, 27–52. doi:10.1007/978-981-32-9721-0_3
Otonari, J., Ikezaki, H., Furusyo, N., and Sudo, N. (2021). Association of lifestyle factors with osteoporosis and fracture in postmenopausal women: A Japanese cohort study. Menopause 28 (11), 1254–1263. doi:10.1097/GME.0000000000001840
Owen-Woods, C., and Kusumbe, A. (2022). Fundamentals of bone vasculature: Specialization, interactions and functions. Semin. Cell Dev. Biol. 123, 36–47. doi:10.1016/j.semcdb.2021.06.025
Peter, C., Waldmann, H., and Cobbold, S. P. (2010). mTOR signalling and metabolic regulation of T cell differentiation. Curr. Opin. Immunol. 22 (5), 655–661. doi:10.1016/j.coi.2010.08.010
Pietschmann, P., and Peterlik, M. (1999). [Pathophysiology and therapy of osteoporosis]. Radiologe 39 (3), 228–234. doi:10.1007/s001170050500
Pollizzi, K. N., and Powell, J. D. (2014). Integrating canonical and metabolic signalling programmes in the regulation of T cell responses. Nat. Rev. Immunol. 14 (7), 435–446. doi:10.1038/nri3701
Popova, N. V., and Jucker, M. (2021). The role of mTOR signaling as a therapeutic target in cancer. Int. J. Mol. Sci. 22 (4), 1743. doi:10.3390/ijms22041743
Porstmann, T., Santos, C. R., Griffiths, B., Cully, M., Wu, M., Leevers, S., et al. (2008). SREBP activity is regulated by mTORC1 and contributes to Akt-dependent cell growth. Cell Metab. 8 (3), 224–236. doi:10.1016/j.cmet.2008.07.007
Poznyak, A. V., Sukhorukov, V. N., Zhuravlev, A., Orekhov, N. A., Kalmykov, V., and Orekhov, A. N. (2022). Modulating mTOR signaling as a promising therapeutic strategy for atherosclerosis. Int. J. Mol. Sci. 23 (3), 1153. doi:10.3390/ijms23031153
Pumberger, M., Qazi, T. H., Ehrentraut, M. C., Textor, M., Kueper, J., Stoltenburg-Didinger, G., et al. (2016). Synthetic niche to modulate regenerative potential of MSCs and enhance skeletal muscle regeneration. Biomaterials 99, 95–108. doi:10.1016/j.biomaterials.2016.05.009
Refaie, A. F., Elbassiouny, B. L., Kloc, M., Sabek, O. M., Khater, S. M., Ismail, A. M., et al. (2021). From mesenchymal stromal/stem cells to insulin-producing cells: Immunological considerations. Front. Immunol. 12, 690623. doi:10.3389/fimmu.2021.690623
Rion, N., Castets, P., Lin, S., Enderle, L., Reinhard, J. R., Eickhorst, C., et al. (2019). mTOR controls embryonic and adult myogenesis via mTORC1. Development 146 (7), dev172460. doi:10.1242/dev.172460
Risson, V., Mazelin, L., Roceri, M., Sanchez, H., Moncollin, V., Corneloup, C., et al. (2009). Muscle inactivation of mTOR causes metabolic and dystrophin defects leading to severe myopathy. J. Cell Biol. 187 (6), 859–874. doi:10.1083/jcb.200903131
Sachdev, P. S., Zhuang, L., Braidy, N., and Wen, W. (2013). Is Alzheimer's a disease of the white matter? Curr. Opin. Psychiatry 26 (3), 244–251. doi:10.1097/YCO.0b013e32835ed6e8
Safari, M., Jafari, B., Zarbakhsh, S., Sameni, H., Vafaei, A. A., Mohammadi, N. K., et al. (2016). G-CSF for mobilizing transplanted bone marrow stem cells in rat model of Parkinson's disease. Iran. J. Basic Med. Sci. 19 (12), 1318–1324. doi:10.22038/ijbms.2016.7918
Salaramoli, S., Joshaghani, H., and Hashemy, S. I. (2022). Selenium effects on oxidative stress-induced calcium signaling pathways in Parkinson's disease. Indian J. Clin. biochem. 37, 257–266. doi:10.1007/s12291-022-01031-1
Samidurai, A., Kukreja, R. C., and Das, A. (2018). Emerging role of mTOR signaling-related miRNAs in cardiovascular diseases. Oxidative Med. Cell. Longev. 2018.
Savio-Silva, C., Soinski-Sousa, P. E., Balby-Rocha, M. T. A., Lira, A. d. O., and Rangel, E. B. (2020). Mesenchymal stem cell therapy in acute kidney injury (AKI): Review and perspectives. Rev. Assoc. Med. Bras. 66, S45–S54. doi:10.1590/1806-9282.66.S1.45
Schaub, T., Gurgen, D., Maus, D., Lange, C., Tarabykin, V., Dragun, D., et al. (2019). mTORC1 and mTORC2 differentially regulate cell fate programs to coordinate osteoblastic differentiation in mesenchymal stromal cells. Sci. Rep. 9, 20071. doi:10.1038/s41598-019-56237-w
Shams, R., Ito, Y., and Miyatake, H. (2021). Mapping of mTOR drug targets: Featured platforms for anti-cancer drug discovery. Pharmacol. Ther. 232, 108012. doi:10.1016/j.pharmthera.2021.108012
Shan, H. J., Gao, X., Zhang, M., Huang, M., Fang, X., Chen, H., et al. (2021). Injectable ROS-scavenging hydrogel with MSCs promoted the regeneration of damaged skeletal muscle. J. Tissue Eng. 12, 20417314211031378. doi:10.1177/20417314211031378
Shanahan, C. M. (2013). Autophagy and matrix vesicles: New partners in vascular calcification. Kidney Int. 83 (6), 984–986. doi:10.1038/ki.2013.75
Shen, G. Y., Ren, H., Qiu, T., Liang, D., Xie, B., Zhang, Z., et al. (2016). Implications of the interaction between miRNAs and autophagy in osteoporosis. Calcif. Tissue Int. 99 (1), 1–12. doi:10.1007/s00223-016-0122-x
Shen, G. Y., Ren, H., Qiu, T., Zhang, Z., Zhao, W., Yu, X., et al. (2018). Mammalian target of rapamycin as a therapeutic target in osteoporosis. J. Cell. Physiol. 233 (5), 3929–3944. doi:10.1002/jcp.26161
Shi, H. Z., Zeng, J. C., Shi, S. H., Giannakopoulos, H., Zhang, Q. Z., and Le, A. D. (2021). Extracellular vesicles of GMSCs alleviate aging-related cell senescence. J. Dent. Res. 100 (3), 283–292. doi:10.1177/0022034520962463
Shimizu, H., Yokoyama, S., and Asahara, H. (2007). Growth and differentiation of the developing limb bud from the perspective of chondrogenesis. Dev. Growth Differ. 49 (6), 449–454. doi:10.1111/j.1440-169X.2007.00945.x
Singha, U. K., Jiang, Y., Yu, S., Luo, M., Lu, Y., Zhang, J., et al. (2008). Rapamycin inhibits osteoblast proliferation and differentiation in MC3T3-E1 cells and primary mouse bone marrow stromal cells. J. Cell. Biochem. 103 (2), 434–446. doi:10.1002/jcb.21411
Smink, J. J., Begay, V., Schoenmaker, T., Sterneck, E., de Vries, T. J., and Leutz, A. (2009). Transcription factor C/EBPbeta isoform ratio regulates osteoclastogenesis through MafB. Embo J. 28 (12), 1769–1781. doi:10.1038/emboj.2009.127
Song, B. Q., Chi, Y., Li, X., Du, W. j., Han, Z. B., Tian, J. j., et al. (2015). Inhibition of Notch signaling promotes the adipogenic differentiation of mesenchymal stem cells through autophagy activation and PTEN-PI3K/AKT/mTOR pathway. Cell. Physiol. biochem. 36 (5), 1991–2002. doi:10.1159/000430167
Song, T. R., Eirin, A., Zhu, X., Zhao, Y., Krier, J. D., Tang, H., et al. (2020). Mesenchymal stem cell-derived extracellular vesicles induce regulatory T cells to ameliorate chronic kidney injury. Hypertension 75 (5), 1223–1232. doi:10.1161/HYPERTENSIONAHA.119.14546
Su, J. A., You, P., Li, W. L., Tao, X. R., Zhu, H. Y., Yao, Y. C., et al. (2010). The existence of multipotent stem cells with epithelial-mesenchymal transition features in the human liver bud. Int. J. Biochem. Cell Biol. 42 (12), 2047–2055. doi:10.1016/j.biocel.2010.09.009
Sun, S. Y. (2021). mTOR-targeted cancer therapy: great target but disappointing clinical outcomes, why? Front. Med. 15 (2), 221–231. doi:10.1007/s11684-020-0812-7
Sun, Y. T., Ge, Y., Drnevich, J., Zhao, Y., Band, M., and Chen, J. (2010). Mammalian target of rapamycin regulates miRNA-1 and follistatin in skeletal myogenesis. J. Cell Biol. 189 (7), 1157–1169. doi:10.1083/jcb.200912093
Sun, Z., and Liu, J. L. (2019). mTOR-S6K1 pathway mediates cytoophidium assembly. J. Genet. Genomics 46 (2), 65–74. doi:10.1016/j.jgg.2018.11.006
Takafuji, Y., Hori, M., Mizuno, T., and Harada-Shiba, M. (2019). Humoral factors secreted from adipose tissue-derived mesenchymal stem cells ameliorate atherosclerosis in Ldlr(-/-) mice. Cardiovasc. Res. 115 (6), 1041–1051. doi:10.1093/cvr/cvy271
Takahara, T., Amemiya, Y., Sugiyama, R., Maki, M., and Shibata, H. (2020). Amino acid-dependent control of mTORC1 signaling: A variety of regulatory modes. J. Biomed. Sci. 27 (1), 87. doi:10.1186/s12929-020-00679-2
Tanaka, Y., Sonoda, S., Yamaza, H., Murata, S., Nishida, K., Hama, S., et al. (2018). Suppression of AKT-mTOR signal pathway enhances osteogenic/dentinogenic capacity of stem cells from apical papilla. Stem Cell Res. Ther. 9, 334. doi:10.1186/s13287-018-1077-9
Thoreen, C. C., Kang, S. A., Chang, J. W., Liu, Q., Zhang, J., Gao, Y., et al. (2009). An ATP-competitive mammalian target of rapamycin inhibitor reveals rapamycin-resistant functions of mTORC1. J. Biol. Chem. 284 (12), 8023–8032. doi:10.1074/jbc.M900301200
Tian, Y. H., Chen, J., Yan, X., Ren, D., Liu, M., Zhang, Q., et al. (2021). Overloaded orthopedic force induces condylar subchondral bone absorption by stimulating rat mesenchymal stem cells differentiating into osteoclasts via mTOR-regulated RANKL/OPG secretion in osteoblasts. Stem Cells Dev. 30 (1), 29–38. doi:10.1089/scd.2020.0163
Tian, Z., Yan, B. J., Luo, W., Gui, D. D., Zhou, K., Tian, K. J., et al. (2021). Sestrin2 in atherosclerosis. Clin. Chim. Acta. 523, 325–329. doi:10.1016/j.cca.2021.10.019
Tong, X. S., Gu, J., Chen, M., Wang, T., Zou, H., Song, R., et al. (2020). p53 positively regulates osteoprotegerin-mediated inhibition of osteoclastogenesis by downregulating TSC2-induced autophagy in vitro. Differentiation. 114, 58–66. doi:10.1016/j.diff.2020.06.002
Tramutola, A., Triplett, J. C., Di Domenico, F., Niedowicz, D. M., Murphy, M. P., Coccia, R., et al. (2015). Alteration of mTOR signaling occurs early in the progression of alzheimer disease (AD): Analysis of brain from subjects with pre-clinical AD, amnestic mild cognitive impairment and late-stage AD. J. Neurochem. 133 (5), 739–749. doi:10.1111/jnc.13037
Umezawa, A., and Akutsu, H. (2008). Osteogenesis and chondrogenesis from a stem cell source. Clin. Calcium 18 (12), 1721–1727.
Varco-Merth, B. D., Brantley, W., Marenco, A., Duell, D. D., Fachko, D. N., Richardson, B., et al. (2022). Rapamycin limits CD4(+) T cell proliferation in simian immunodeficiency virus-infected rhesus macaques on antiretroviral therapy. J. Clin. Invest. 132 (10), e156063. doi:10.1172/JCI156063
Vila-Bedmar, R., Lorenzo, M., and Fernandez-Veledo, S. (2010). Adenosine 5'-monophosphate-activated protein kinase-mammalian target of rapamycin cross talk regulates brown adipocyte differentiation. Endocrinology 151 (3), 980–992. doi:10.1210/en.2009-0810
Vizoso, F. J., Eiro, N., Cid, S., Schneider, J., and Perez-Fernandez, R. (2017). Mesenchymal stem cell secretome: Toward cell-free therapeutic strategies in regenerative medicine. Int. J. Mol. Sci. 18 (9), E1852. doi:10.3390/ijms18091852
Wang, J. C., and Bennett, M. (2012). Aging and atherosclerosis mechanisms, functional consequences, and potential therapeutics for cellular senescence. Circ. Res. 111 (2), 245–259. doi:10.1161/CIRCRESAHA.111.261388
Wang, Z., Cai, H., Zhao, E., and Cui, H. (2021). The diverse roles of histone demethylase KDM4B in normal and cancer development and progression. Front. Cell Dev. Biol. 9, 790129. doi:10.3389/fcell.2021.790129
Weichhart, T., Hengstschlager, M., and Linke, M. (2015). Regulation of innate immune cell function by mTOR. Nat. Rev. Immunol. 15 (10), 599–614. doi:10.1038/nri3901
Wen, L. R., Gao, M., He, Z., Guo, P., Liu, Z., Zhang, P., et al. (2021). Noggin, an inhibitor of bone morphogenetic protein signaling, antagonizes TGF-beta 1 in a mouse model of osteoarthritis. Biochem. Biophysical Res. Commun. 570, 199–205. doi:10.1016/j.bbrc.2021.07.044
Williams, A. H., Valdez, G., Moresi, V., Qi, X., McAnally, J., Elliott, J. L., et al. (2009). MicroRNA-206 delays ALS progression and promotes regeneration of neuromuscular synapses in mice. Science 326 (5959), 1549–1554. doi:10.1126/science.1181046
Wu, J. W., Ye, T., Hongwei, W., Dachuan, L., Fei, Z., Jianyuan, J., et al. (2022). The role of TAK1 in RANKL-induced osteoclastogenesis. Calcif. Tissue Int. 111 (1), 1–12. doi:10.1007/s00223-022-00967-z
Xia, P., Wang, X., Qu, Y., Lin, Q., Cheng, K., Gao, M., et al. (2017). TGF-beta 1-induced chondrogenesis of bone marrow mesenchymal stem cells is promoted by low-intensity pulsed ultrasound through the integrin-mTOR signaling pathway. Stem Cell Res. Ther. 8, 281. doi:10.1186/s13287-017-0733-9
Xiang, X. X., Zhao, J., Xu, G., Li, Y., and Zhang, W. (2011). mTOR and the differentiation of mesenchymal stem cells. Acta Biochim. Biophys. Sin. 43 (7), 501–510. doi:10.1093/abbs/gmr041
Xu, F., Na, L., Li, Y., and Chen, L. (2020). Roles of the PI3K/AKT/mTOR signalling pathways in neurodegenerative diseases and tumours. Cell Biosci. 10 (1), 54. doi:10.1186/s13578-020-00416-0
Yang, B., Cai, Z., Zhang, W., Yin, D., Zhao, W., and Yang, M. (2019). Autophagy alleviates the decrease in proliferation of amyloid (1-42)-treated bone marrow mesenchymal stem cells via the AKT/mTOR signaling pathway. Mol. Med. Rep. 19 (5), 4091–4100. doi:10.3892/mmr.2019.10069
Yang, L., Li, N., Yang, D., Chen, A., Tang, J., Jing, Y., et al. (2021). CCL2 regulation of MST1-mTOR-STAT1 signaling axis controls BCR signaling and B-cell differentiation. Cell Death Differ. 28 (9), 2616–2633. doi:10.1038/s41418-021-00775-2
Yang, M. K., Teng, S., Ma, C., Yu, Y., Wang, P., and Yi, C. (2018). Ascorbic acid inhibits senescence in mesenchymal stem cells through ROS and AKT/mTOR signaling. Cytotechnology 70 (5), 1301–1313. doi:10.1007/s10616-018-0220-x
Yang, W. Z., Yin, R., Zhu, X., Yang, S., Wang, J., Zhou, Z., et al. (2021). Mesenchymal stem-cell-derived exosomal miR-145 inhibits atherosclerosis by targeting JAM-A. Mol. Ther. Nucleic Acids 23, 119–131. doi:10.1016/j.omtn.2020.10.037
Yoo, H. S., Lee, K., Na, K., Zhang, Y. X., Lim, H-J., Yiet, T., et al. (2017). Mesenchymal stromal cells inhibit CD25 expression via the mTOR pathway to potentiate T-cell suppression. Cell Death Dis. 8.
Yu, W. H., Chen, Z., Zhang, J., Zhang, L., Ke, H., Huang, L., et al. (2008). Critical role of phosphoinositide 3-kinase cascade in adipogenesis of human mesenchymal stem cells. Mol. Cell. Biochem. 310 (1-2), 11–18. doi:10.1007/s11010-007-9661-9
Zhang, D. Y., Yan, B. S., Yu, S. S., Zhang, C., Wang, B. M., Wang, Y. Y., et al. (2015). Coenzyme Q10 inhibits the aging of mesenchymal stem cells induced by D-galactose through akt/mTOR signaling. Oxidative Med. Cell. Longev. 2015, 867293. doi:10.1155/2015/867293
Zhang, D. Y., Lu, H., Chen, Z., Wang, Y., Lin, J., Xu, S., et al. (2017). High glucose induces the aging of mesenchymal stem cells via Akt/mTOR signaling. Mol. Med. Rep. 16 (2), 1685–1690. doi:10.3892/mmr.2017.6832
Zhang, H. H., Huang, J., Duvel, K., Boback, B., Wu, S., Squillace, R. M., et al. (2009). Insulin stimulates adipogenesis through the akt-TSC2-mTORC1 pathway. Plos One 4 (7), e6189. doi:10.1371/journal.pone.0006189
Zhang, L., McKenna, M. A., Said-Al-Naief, N., Wu, X., Feng, X., and McDonald, J. M. (2005). Osteoclastogenesis: The role of calcium and calmodulin. Crit. Rev. Eukaryot. Gene Expr. 15 (1), 1–13. doi:10.1615/critreveukaryotgeneexpr.v15.i1.10
Zhang, Y., Yu, B., He, J., and Chen, D. (2016). From nutrient to MicroRNA: A novel insight into cell signaling involved in skeletal muscle development and disease. Int. J. Biol. Sci. 12 (10), 1247–1261. doi:10.7150/ijbs.16463
Zhao, E., Ding, J., Xia, Y., Liu, M., Ye, B., Choi, J. H., et al. (2016). KDM4C and ATF4 cooperate in transcriptional control of amino acid metabolism. Cell Rep. 14 (3), 506–519. doi:10.1016/j.celrep.2015.12.053
Zhao, E., Feng, L., Bai, L., and Cui, H. (2020). NUCKS promotes cell proliferation and suppresses autophagy through the mTOR-Beclin1 pathway in gastric cancer. J. Exp. Clin. Cancer Res. 39 (1), 194. doi:10.1186/s13046-020-01696-7
Zhao, E., Hou, J., Ke, X., Abbas, M. N., Kausar, S., Zhang, L., et al. (2019). The roles of sirtuin family proteins in cancer progression. Cancers (Basel) 11 (12), E1949. doi:10.3390/cancers11121949
Zhao, X. G., Zhao, Y., Sun, X., Xing, Y., Wang, X., and Yang, Q. (2020). Immunomodulation of MSCs and MSC-derived extracellular vesicles in osteoarthritis. Front. Bioeng. Biotechnol. 8, 575057. doi:10.3389/fbioe.2020.575057
Zhen, G. H., Wen, C., Jia, X., Li, Y., Crane, J. L., Mears, S. C., et al. (2013). Inhibition of TGF-beta signaling in mesenchymal stem cells of subchondral bone attenuates osteoarthritis. Nat. Med. 19 (6), 704–712. doi:10.1038/nm.3143
Zhou, X., Xu, S. N., Yuan, S. T., Lei, X., Sun, X., Xing, L., et al. (2021). Multiple functions of autophagy in vascular calcification. Cell Biosci. 11 (1), 159. doi:10.1186/s13578-021-00639-9
Zhu, Z., Yang, C., Iyaswamy, A., Krishnamoorthi, S., Sreenivasmurthy, S. G., Liu, J., et al. (2019). Balancing mTOR signaling and autophagy in the treatment of Parkinson's disease. Int. J. Mol. Sci. 20 (3), E728. doi:10.3390/ijms20030728
Keywords: mTOR, mesenchymal stem cells, differentiation, immune response, ageing-related diseases, therapeutic target
Citation: Cai H, Wang Z, Tang W, Ke X and Zhao E (2022) Recent advances of the mammalian target of rapamycin signaling in mesenchymal stem cells. Front. Genet. 13:970699. doi: 10.3389/fgene.2022.970699
Received: 16 June 2022; Accepted: 11 August 2022;
Published: 30 August 2022.
Edited by:
Fan He, Soochow University, ChinaReviewed by:
Anjali P. Kusumbe, University of Oxford, United KingdomCopyright © 2022 Cai, Wang, Tang, Ke and Zhao. This is an open-access article distributed under the terms of the Creative Commons Attribution License (CC BY). The use, distribution or reproduction in other forums is permitted, provided the original author(s) and the copyright owner(s) are credited and that the original publication in this journal is cited, in accordance with accepted academic practice. No use, distribution or reproduction is permitted which does not comply with these terms.
*Correspondence: Xiaoxue Ke, a2V4aWFveHVlQDEyNi5jb20=; Erhu Zhao, ZXJodXpoYW9AMTI2LmNvbQ==
†These authors have contributed equally to this work
Disclaimer: All claims expressed in this article are solely those of the authors and do not necessarily represent those of their affiliated organizations, or those of the publisher, the editors and the reviewers. Any product that may be evaluated in this article or claim that may be made by its manufacturer is not guaranteed or endorsed by the publisher.
Research integrity at Frontiers
Learn more about the work of our research integrity team to safeguard the quality of each article we publish.