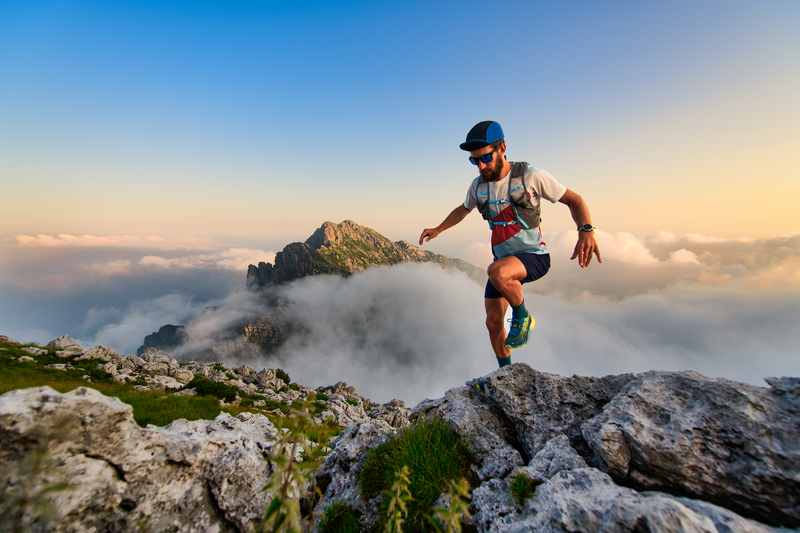
95% of researchers rate our articles as excellent or good
Learn more about the work of our research integrity team to safeguard the quality of each article we publish.
Find out more
PERSPECTIVE article
Front. Genet. , 22 June 2022
Sec. Evolutionary and Genomic Microbiology
Volume 13 - 2022 | https://doi.org/10.3389/fgene.2022.947572
This article is part of the Research Topic Chromosome and Genome Biology of Fungi View all 7 articles
Meiosis is an essential component of the sexual life cycle in eukaryotes. The independent assortment of chromosomes in meiosis increases genetic diversity at the level of whole chromosomes and meiotic recombination increases genetic diversity within chromosomes. The resulting variability fuels evolution. Interestingly, global mapping of recombination in diverse taxa revealed dramatic changes in its frequency distribution between closely related species, subspecies, and even isolated populations of the same species. New insight into mechanisms for these evolutionarily rapid changes has come from analyses of environmentally induced plasticity of recombination in fission yeast. Many different DNA sites, and where identified their binding/activator proteins, control the positioning of recombination at hotspots. Each different class of hotspots functions as an independently controlled rheostat that modulates rates of recombination over a broad dynamic range in response to changing conditions. Together, this independent modulation can rapidly and dramatically alter the global frequency distribution of recombination. This process likely contributes substantially to (i.e., can largely explain) evolutionarily rapid, Prdm9-independent changes in the recombination landscape. Moreover, the precise control mechanisms allow cells to dynamically favor or disfavor newly arising combinations of linked alleles in response to changing extracellular and intracellular conditions, which has striking implications for the impacts of meiotic recombination on evolution.
With few exceptions, eukaryotes have an obligate sexual life cycle with alternating haploid and diploid states; these are coupled by meiosis and fertilization. Homologous recombination is induced to high levels in meiosis and serves two key functions (Henderson and Bomblies, 2021). First, meiotic recombination is generally required for the proper segregation of chromosomes in meiosis and, correspondingly, for the faithful transmission of chromosomes between generations. Second, recombination shuffles the linkages of gene-alleles within chromosomes. The independent assortment of chromosomes in meiosis promotes genetic diversity at the level of whole chromosomes and meiotic recombination promotes genetic diversity within chromosomes; together, these processes provide genetic variability that is the fuel for evolution. Notably, meiotic recombination is the only way to uncouple newly arising, cis-linked, deleterious mutations and to generate new combinations of beneficial alleles within the same chromosome (Otto, 2021). To adapt a quote from Lewis Thomas (Thomas, 1979), if there were no meiotic recombination, we would still be anaerobic bacteria and there would be no music.
Meiotic recombination is controlled at multiple levels including where it initiates, how pathway intermediates are resolved, and the modulation of average rates along entire chromosomes in response to chromosome size (Zelkowski et al., 2019; Sanchez et al., 2021; Yadav and Bouuaert, 2021). As with many biochemical pathways, a crucial, rate-limiting step occurs early in the pathway; specifically, the induction of recombination-initiating dsDNA breaks (DSBs). These DSBs are created by the meiotically induced, basal recombination machinery via its catalytic subunit, Spo11/Rec12 (Lam and Keeney, 2014; Robert et al., 2016; Jing et al., 2019). The broken chromosome is repaired using its homolog (or sister chromatid) as a template. When the repair events encompass heterologies (such as single-nucleotide polymorphisms, or SNPs), they can produce scorable recombination events. These manifest primarily as gene conversion events, a subset of which are accompanied by reciprocal recombination events (crossovers) between the homologs (Figure 1A) (Lorenz and Mpaulo, 2022). Correspondingly, there is good agreement between mapped distributions of DSBs, gene conversions, and conversion-associated crossovers across the genome.
FIGURE 1. Highly dynamic, extensive changes in the meiotic recombination landscape (A) Meiotic recombination leads to gene conversion, with or without reciprocal exchanges (crossovers) (B) Meiotically induced, recombination-initiating dsDNA breaks (DSBs) cluster at hotspots that position recombination in the genome. This example depicts frequency distribution of DSBs along a portion of chromosome one in fission yeast (C) Example of evolutionarily rapid changes. Plot shows distribution of recombination rates along chromosome one in two different populations (red, blue) of stickleback fish, along with positions of identified hotspots (tic marks) (D) Example of environmentally induced changes. Plot shows effects of temperature on DSB hotspot positions (tic marks) on right arm of chromosome seven in budding yeast. We posit that the dramatic, evolutionarily rapid (panel C) and environmentally induced (panel D) changes in the recombination landscape share a common molecular mechanism.
Genetic approaches to map global frequency distributions of recombination each involve the genotyping (by deep sequencing or DNA microarray hybridization) of many genomes to detect, and measure or infer with precision, rates of recombination between markers (e.g., SNPs) along chromosomes (Penalba and Wolf, 2020). There are also molecular approaches to map the distribution of recombination-initiating DSBs (e.g., Figure 1B) (Gerton et al., 2000; Blitzblau et al., 2007; Pan et al., 2011). These DSB-mapping approaches, so far applied to a limited but growing number of species, have higher resolution and sensitivity than the genetics-based approaches, whose resolution is limited by the density and distribution of genetic markers, such as SNPs. Regardless of approach, mapping of recombination in diverse taxa has revealed that recombination is clustered preferentially at discrete locations, called hotspots, which control the frequency distribution of recombination across the genome (Wahls and Davidson, 2012; Choi and Henderson, 2015; Ergoren, 2018).
It is standard practice in the field to annotate the positions of hotspots based on some type of frequency cutoff threshold (i.e., a given location in the genome is judged to be either “hot” or “not hot”). However, there are differences in the sensitivities and resolutions of the different mapping approaches that are used. Moreover, hotspot calling criteria differ substantially among studies. Such factors can complicate the interpretation of hotspot maps. More fundamentally, the process of annotating the positions of hotspots can be misleading because—as described subsequently in this perspective—individual hotspots actually function as rheostats that can variably modulate rates of recombination over a broad dynamic range (Brown et al., 2020; Protacio et al., 2022). From our perspective, these factors are germane to long-recognized, mechanistically enigmatic features of the meiotic recombination landscape.
One might expect that the global frequency distribution of meiotic recombination would differ substantially between highly diverged taxa. Conversely, one might expect recombination landscapes to be similar between closely related species. Intriguingly, and contrary to expectations, analyses conducted in diverse taxa have revealed striking differences in fine-scale recombination maps and annotated hotspot positions between closely related species, sub-species and isolated populations of the same species.
An example of this phenomenon is provided in Figure 1C. This plot shows the frequency of crossover recombination per unit distance, calculated using linkage disequilibrium (LD) values, in two different populations of stickleback fish that live in distinct environments (marine and freshwater) (Shanfelter et al., 2019). There are profound differences in the recombination landscapes between these two populations. Moreover, as is standard practice in the field, the authors used a peak calling algorithm to annotate the positions of recombination hotspots. This approach revealed that about 85% of identified hotspots are at different positions in the two populations—even though those populations only became isolated from each other within the past 15 thousand years.
Similar differences have been documented in other taxa. For example, in flycatcher birds 55%–61% of annotated hotspots are at different positions between pairs of closely related species, and 31%–49% of hotspot positions differ between isolated populations of the same species (Kawakami et al., 2017). Likewise, about 80% of inferred hotspots are at different locations in sub-species of rice (Marand et al., 2019). Similarly, the distribution of annotated hotspots differs substantially between species of the yeast genus Lachancea; as well as between Lachancea sp and Saccharomyces sp (Brion et al., 2017). There are also differences in hotspot positions between species of the genus Saccharomyces (Tsai et al., 2010; Lam and Keeney, 2015). Mechanisms for such evolutionarily rapid, Prdm9-independent changes in the global frequency distribution of meiotic recombination across different kingdoms of the eukaryotic domain were unknown. From our perspective, the mechanisms are related to those of another interesting phenomenon; namely, environmentally induced plasticity of recombination.
More than a century ago, it was discovered that extrinsic factors (e.g., temperature) and intrinsic factors (e.g., genetic differences) affect rates of meiotic recombination (Plough, 1917; Sturtevant, 1917). Subsequent studies revealed that such plasticity is common, if not ubiquitous, across taxa. Intrinsic factors such as genetic background, sex, mating type, auxotrophies, and DNA sequence polymorphisms can each affect rates and patterns of recombination (Szankasi et al., 1988; Parvanov et al., 2008; Kong et al., 2010; Hyppa et al., 2014; Brick et al., 2018). Extrinsic factors such as temperature, nutrients, osmolarity, stress and parasite infection can also affect recombination (Abdullah and Borts, 2001; Parvanov et al., 2008; Cotton et al., 2009; Stahl et al., 2016; Singh, 2019; Brown et al., 2020). Most of the work on the effects of environmental conditions (e.g., temperature) on recombination has been conducted at low resolution (e.g., by counting chiasmata, which are cytological manifestations of crossovers) or by analyzing frequencies of DSBs and/or rates of recombination at a limited number of locations in the genome. However, in a few cases, the impacts of environmental conditions on the global frequency distribution of recombination has been determined at high resolution under well-controlled laboratory conditions.
A striking example of genome-wide plasticity came from determining the effects of temperature on the distribution of DSB hotspots, as defined using a frequency cutoff threshold, in budding yeast (Figure 1D) (Zhang et al., 2017). Remarkably, only about 20% of annotated hotspots throughout the genome were at the same positions at all three temperatures; the positions of ∼80% of hotspots differed between two or all three temperatures. The conclusions are unambiguous: changing environmental conditions can trigger dramatic, genome-wide changes in the fine-scale recombination landscape.
As is the case for the evolutionarily rapid changes in the recombination landscape described above, mechanisms for environmentally induced plasticity of recombination have been elusive. One long-standing notion is that the synaptonemal complex (SC) might contribute to such plasticity [see reviews by (Morgan et al., 2017; Henderson and Bomblies, 2021)]. However, while changes in the SC could affect recombination rates at broad scale (e.g., at the level of whole chromosomes), it is difficult to envision how the SC could mediate dramatic changes in the fine-scale recombination landscape (e.g., Figures 1C,D). Moreover, organisms that lack an SC, such as fission yeast (Kohli and Bahler, 1994), still have fine-scale plasticity (Hyppa et al., 2014; Brown et al., 2020; Protacio et al., 2022). Such localized changes in recombination rates—which are increased at some sites and decreased at others—would require perforce a means to dynamically and differentially control rates of recombination locally; i.e., at the resolution of individual recombination hotspots.
To identify mechanisms that control the redistribution (plasticity) of meiotic recombination, one must first identify what controls its positioning in the genome. In other words, what makes a hotspot hot? The primary, cis-acting determinants are unknown in most species. However, by focusing on an allele-specific hotspot in fission yeast (Gutz, 1971), Jürg Kohli’s lab discovered the DNA site-dependent control of hotspots (Schuchert et al., 1991). Contemporaneously, Tom Petes’ group provided evidence for such regulation in budding yeast (White et al., 1991; White et al., 1993). Subsequently, using an insightful genetic screen in fission yeast, Walter Steiner’s lab identified about 200 additional, distinct, short (30 base pairs or less), hotspot-activating DNA sequences (Steiner et al., 2009). Five of the regulatory DNA sequence motifs (DNA sites) have been defined with precision by systematic, comprehensive, base pair substitutions in the genome (Schuchert et al., 1991; Steiner et al., 2009; Steiner et al., 2011); and the binding/activator protein complexes have been identified for three of these DNA sites (Figure 2A) (Wahls and Smith, 1994; Kon et al., 1997; Steiner et al., 2011). The different classes of DNA site-dependent hotspots each function by a common downstream effector mechanism. They each trigger the displacement of nucleosomes to increase access for the basal recombination machinery to its DNA substrates in chromatin (Storey et al., 2018; Mukiza et al., 2019), thereby stimulating the frequency of recombination-initiating DSBs (e.g., Figure 2B) (Steiner et al., 2002; Wahls and Davidson, 2010; Fowler et al., 2014).
FIGURE 2. Mechanisms for plasticity in the frequency distribution of meiotic recombination (A) Examples of DNA site-specific protein-DNA complexes (cis-acting regulatory modules) that activate recombination hotspots (B) The cis-acting regulatory modules promote catalysis of DSBs by the basal recombination machinery. In this example, Oligo-C DNA sites in the genome were identified, oriented and aligned; plot shows average distribution of DSBs (blue) and nucleosomes (grey) around the DNA site (C) Hotspots function as rheostats that variably modulate recombination rates in response to extracellular and intracellular cues. Example shows rates of recombination for the Oligo-C hotspot and for a basal recombination control at different temperatures (D) Model for plasticity in the recombination landscape. Each hotspot DNA site promotes recombination in its vicinity. Each different class of hotspots is independently controlled in response to changing conditions. Their net contributions under different conditions dramatically alter the recombination landscape (E) It is standard practice in the field to apply frequency thresholds (illustrated by dashed red lines) to annotate hotspot positions (numbered tic marks). This can give the false impression that the positions of hotspots “move” from condition to condition or population to population (compare E to D).
Hotspot-activating DNA sites and binding/activator proteins discovered in fission yeast are conserved functionally in its distant cousin, budding yeast (Steiner and Steiner, 2012; Wahls and Davidson, 2012). This is notable because fission yeast and budding yeast are about as evolutionarily distant from each other as humans are from nematodes (Sipiczki, 2000; Heckman et al., 2001). Moreover, hotspot-associated DNA sites identified computationally from recombination maps in other taxa, such as honeybees (Mougel et al., 2014), are identical or similar to hotspot-activating DNA sites of fission yeast. Thus, DNA site-dependent mechanisms for the positioning of meiotic recombination are likely broadly conserved. In support of this idea, downstream effector mechanisms discovered in fission yeast [e.g. (Mizuno et al., 1997; Yamada et al., 2004; Hirota et al., 2007),] are also conserved. For example, diverse species exhibit the displacement of nucleosomes at or near hotspot centers [reviewed by (Tock and Henderson, 2018), which is a fundamental characteristic of, and known effector mechanism for, DNA site-dependent hotspots [see Figure 2B and (Mizuno et al., 1997; Mukiza et al., 2019)]. As a more discrete example of conserved effector mechanisms, orthologs of an ATP-dependent chromatin remodeling enzyme of fission yeast (Snf22) and mice (Hells) are each recruited to, and help to activate via nucleosome displacement, DNA site-dependent hotspots (Yamada et al., 2004; Storey et al., 2018; Mukiza et al., 2019; Spruce et al., 2020).
Nearly two decades after the DNA-site dependent activation of hotspots was discovered in fission yeast (Schuchert et al., 1991), it was implicated by association in mammals (Myers et al., 2008). In a subset of metazoans (e.g., mice, cattle and humans), Prdm9 binding sites can activate hotspots [see reviews by (Grey et al., 2018; Paigen and Petkov, 2018)]. Because this mechanism operates in humans and the DNA binding domain of Prdm9 evolves rapidly, it has garnered much attention. However, a far greater diversity of species (e.g., fungi, birds, amphibians, many fishes, canids, marsupials and plants) lack Prdm9 or one of its functionally important domains, but still have hotspots that tend to distribute in a more yeast-like fashion in the genome [e.g. (Munoz-Fuentes et al., 2011; Axelsson et al., 2012; Kawakami et al., 2017),]. Interestingly, Prdm9-expressing species can still have hotspots that are located remote from Prdm9 binding sites (Schield et al., 2020). Moreover, upon the ablation of Prdm9 in mice and rats hotspots are not eliminated; instead, they adopt a more yeast-like distribution in the genome (Brick et al., 2012; Mihola et al., 2021). Such findings suggest that there is an evolutionarily ancient, Prdm9-independent mechanism for distributing recombination to hotspots. The functionally conserved, cis-acting regulatory elements discovered in fission yeast provide such a mechanism (Wahls and Davidson, 2012).
In summary, paradigms from fission yeast (e.g., about 200 different, short DNA sequences are known to directly activate hotspots) and data from other species each support the original, evidence-based hypothesis that much, if not most meiotic recombination is positioned in the genome by hotspot-activating DNA sites and their binding proteins (Wahls and Smith, 1994).
If most meiotic recombination is positioned in the genome by hotspot-activating DNA sites, then those DNA sites might directly control plasticity of the recombination landscape. A recent study took advantage of the powerful fission yeast model system to test this hypothesis (Protacio et al., 2022). That study determined the effects of changes in three different environmental conditions (temperature, carbon source and osmolarity) on rates of recombination at a test locus that contained one of three hotspot-activating DNA sites (M26, CCAAT or Oligo-C) or a basal recombination control that lacked those DNA sites. This approach revealed that the impacts of changing environmental conditions on local rates of recombination are mediated directly and primarily by DNA site-specific hotspots and their binding/activator proteins. Key findings include the following.
First, changing environmental conditions has little or no impact on rates of basal (hotspot-independent) recombination. Second, DNA site-dependent hotspots function as rheostats that variably modulate rates of recombination over a broad dynamic range in response to changing conditions (e.g., Figure 2C). A given hotspot can range from being quiescent (i.e., not promote recombination beyond basal levels) to being highly recombinogenic (i.e., greatly stimulate the initiation of recombination by the basal recombination machinery). Third, each different class of DNA site-dependent hotspots functions as an independently controlled rheostat. For example, under a given set of conditions one type of hotspot can promote recombination substantially while another type of hotspot is quiescent. As another example, a discrete change in the environment that increase the activity of one class of hotspots can decrease the activity of another class.
The new discoveries support a model for environmentally induced plasticity of the meiotic recombination landscape (Figure 2D) (Protacio et al., 2022). Each class of hotspot-activating DNA sites functions as an independently controlled rheostat that modulates rates of recombination at its own locations in the genome in response to its own constellation of signals. This independent modulation of rates by many different classes of DNA sites provides a molecular mechanism for precisely controlled, highly dynamic, large-scale changes in the global frequency distribution of meiotic recombination.
The findings also have important implications for hotspot annotation and interpretations therefrom. It is standard practice in the field to define the positions of hotspots by applying frequency cutoff thresholds to recombination maps. However, individual DNA site-specific hotspots actually control recombination rates over a broad dynamic range in response to changing conditions (Protacio et al., 2022). Consequently, the process of annotation can give the false impression that the positions of hotspots “move” from sample to sample, even when the positions of the hotspot regulating DNA sites have not changed (illustrated in Figure 2E). From our perspective, such factors force a shift in thinking about—and reveal fundamental mechanisms for—meiotic recombination-mediated dynamics of genomes, as well as the interplay between meiotic recombination and evolution.
The substantial differences between recombination landscapes of closely related species, sub-species and isolated populations of species that lack Prdm9 have been baffling. Given the low amounts of overall DNA sequence divergence (e.g., between populations), it is implausible that the differences in recombination stem from large-scale changes in the sequences of hotspot-regulating DNA sites throughout the genome or in the DNA binding site specificities of the various binding/activator proteins. The discovery of mechanisms for environmentally induced plasticity (Protacio et al., 2022) provides solutions to this quandary.
First, many of the observed differences (e.g., between populations) might stem from environmentally induced, hotspot DNA site-dependent changes in the recombination landscape. For example, the differences in LD-based recombination maps between freshwater and marine populations of stickleback fish (e.g., Figure 1C) might be due largely to the differences in their environments (e.g., Figure 1D). A testable prediction of this hypothesis is that changing the environmental conditions would trigger substantial changes in distributions of recombination within each population; and that those changes would affect rates of recombination at most of the locations where substantial, population-dependent differences in the recombination landscape have been observed. Such a test would require a more direct approach to map recombination than the widely employed, LD-based approach, which infers average historical rates of recombination over many generations (Penalba and Wolf, 2020).
Second, the molecular mechanisms for plasticity also provide a way to fix (via genetic drift) some of the changes in recombination landscapes during isolation, divergence and speciation. Initial clues for this came from the M26 class of DNA site-specific hotspots. As one might expect based on their response to changing environmental conditions (Brown et al., 2020; Protacio et al., 2022), M26-class hotspots are controlled by components of several different signal transduction pathways that respond to environmental and metabolic cues (Kon et al., 1998; Mizuno et al., 2001; Hirota et al., 2003; Hirota et al., 2004; Yamada et al., 2004; Hirota et al., 2007; Hirota et al., 2008; Gao et al., 2009; Storey et al., 2018). Extending such analyses to multiple different classes of DNA site-specific hotspots was particularly informative. As is the case for the different environmental cues, individual mutations in different components of the signaling pathways affect differentially rates of recombination among distinct classes of hotspots (Mukiza et al., 2019; Protacio et al., 2022). One point in particular—that as little as a single heterology in the genome (e.g., one mutation in a signal transduction pathway) can be sufficient to strongly and differentially adjust the distinct hotspot rheostats—has profound implications. Even minor genetic differences between species, subspecies and isolated populations can, by affecting signal transduction networks that differentially control distinct classes DNA site-specific hotspots, trigger substantial changes in the distribution of recombination across the genome (Protacio et al., 2022). Correspondingly, factors such as genetic drift, bottlenecks, loss and fixation of polymorphisms that affect signal transduction networks would each contribute to changes in the recombination landscape during isolation and speciation—mediated ultimately and directly through the hotspot-regulating DNA sites and their respective binding/activator proteins. Notably, such evolution in the recombination landscape would not require any changes in the distribution of the regulatory DNA sites or in their binding/activator proteins.
A third DNA site-dependent mechanism for changing the recombination landscape over evolutionary time scales involves the loss and gain of hotspot-activating DNA sites themselves (Wahls and Davidson, 2011). Because recombination hotspots serve preferentially as recipients of genetic information during gene conversion (Gutz, 1971), they are inherently suicidal. For example, DNA site-dependent hotspots promote the formation of recombination-initiating DSBs in their vicinity (e.g., Figure 2B) (Steiner et al., 2002; Wahls and Davidson, 2010; Fowler et al., 2014). When the hotspot DNA site is heterozygous, resection of the DSB and repair of that break from the homologous chromosome template will tend to remove the regulatory DNA site. The finding that all well-defined, hotspot-activating DNA sites are substantially under-represented in the genome provides evidence for such meiotic drive towards loss over evolutionary time scales (Wahls and Davidson, 2011). But despite their suicidal tendencies, hotspots have persisted in genomes (albeit not necessarily at their ancestral locations); this has been called the “hotspot paradox” (Boulton et al., 1997). Notably, the generation of hotspot DNA sites by mutations provides a solution to this paradox. For any given hotspot-activating DNA site, there is a large number of inactive, closely related, “cryptic” permutations of that DNA site which can be rendered active by as little as a single base pair substitution. Such cryptic DNA sites are abundant in the genome; e.g., using just five of the hotspot-activating motifs discovered by Steiner et al. (Steiner et al., 2009), we identified 64,622 cryptic motifs with average spacing of one every 194 base pairs (Wahls and Davidson, 2011). Similar considerations apply for the hundreds of other hotspot-activating DNA sequence elements (by extension, cryptic motifs would be ubiquitous and densely packed in the genome). Consequently, each spontaneous mutation has a remarkably high probability of generating a hotspot-activating DNA site. Thus, an equilibrium between rates of hotspot loss (via gene conversion) and hotspot gain (via mutations) would contribute to the retention and repositioning of DNA site-dependent hotspots over evolutionary time scales (Wahls and Davidson, 2011). Presumably, selective pressures that impinge upon the cis-acting regulatory modules also influence the retention, loss and gain of hotspots at various locations in the genome over time.
Lastly, meiotic recombination fuels evolution by uncoupling newly arising, cis-linked, deleterious mutations and by generating new combinations of beneficial alleles within the same chromosome (Otto, 2021). The ability to rapidly, precisely and extensively remodel the recombination landscape provides a way to favor or disfavor newly arising combinations of alleles in response to changing intracellular and extracellular conditions (Protacio et al., 2022). Hypothetically, this form of precisely controlled adaptation confers selective advantages to cells in meiosis, or at subsequent vegetative stages of the life cycle, or both. In support of this idea, meiotic recombination hotspots contribute to combinatorial diversity within the major histocompatibility complex (MHC) and, moreover, hotspot usage varies among populations (Fulton et al., 2016; Beeson et al., 2019; Sun et al., 2022). Thus, the presence of recombination hotspots within the MHC promotes, and can variably control, the diversity of haplotypes that confer self/non-self recognition and adaptive functions of the immune system. We posit that such changes in meiotic recombination, and their impacts on adaptation over evolutionary time scales, are exerted largely through hotspot-regulating DNA sites and their binding/activator proteins.
From our perspective, the punctate distribution of meiotic recombination across genomes and the striking plasticity of recombination landscapes are each readily explained by the fact that many, distinct DNA sites each position recombination at hotspots. Each class of hotspots functions as an independently and precisely controlled rheostat; together, they can dramatically remodel the recombination landscape in response to changing conditions.
There are many interesting questions for future study. For example, are the fundamental mechanisms of plasticity discovered in fission yeast conserved throughout eukaryotes? To what extent do hotspot DNA site-mediated, evolutionarily instantaneous changes in the recombination landscape contribute to the observed differences between related species, subspecies and isolated populations? Similarly, what fraction of the differences in recombination landscapes become fixed during isolation or speciation (e.g., by drift or divergence in DNA sites, binding/activator proteins, and regulatory signal transduction networks)? Lastly, are the molecular mechanisms for rapid remodeling (adaptation) in the frequency distribution of recombination also adaptive (beneficial) over evolutionary time scales?
No new data were generated for this perspective article. Published data were replotted in Figure 1D (Zhang et al., 2017) and Figure 2C (Protacio et al., 2022). Figure 1B and Figure 2B were reproduced with permission under CC BY-NC 4.0 from (Fowler et al., 2014); Figure 1C with permission under CC BY 4.0 from (Shanfelter et al., 2019); Figures 2D,E with permission under CC BY from (Protacio et al., 2022). Images were reproduced with minor modifications (cropping, scaling and labeling) for clarity and parallel construction.
All authors listed have made a substantial, direct, and intellectual contribution to the work and approved it for publication.
Our work was supported grants from the National Institute of General Medical Sciences at the National Institutes of Health (grant numbers GM081766 and GM145834 to WPW).
The authors declare that the research was conducted in the absence of any commercial or financial relationships that could be construed as a potential conflict of interest.
All claims expressed in this article are solely those of the authors and do not necessarily represent those of their affiliated organizations, or those of the publisher, the editors and the reviewers. Any product that may be evaluated in this article, or claim that may be made by its manufacturer, is not guaranteed or endorsed by the publisher.
Abdullah, M. F. F., and Borts, R. H. (2001). Meiotic Recombination Frequencies are Affected by Nutritional States in Saccharomyces Cerevisiae. Proc. Natl. Acad. Sci. U. S. A. 98, 14524–14529. doi:10.1073/pnas.201529598
Axelsson, E., Webster, M. T., Ratnakumar, A., Ponting, C. P., and Lindblad-Toh, K. (2012). Death of PRDM9 Coincides with Stabilization of the Recombination Landscape in the Dog Genome. Genome Res. 22, 51–63. doi:10.1101/gr.124123.111
Beeson, S. K., Mickelson, J. R., and McCue, M. E. (2019). Exploration of Fine-Scale Recombination Rate Variation in the Domestic Horse. Genome Res. 29, 1744–1752. doi:10.1101/gr.243311.118
Blitzblau, H. G., Bell, G. W., Rodriguez, J., Bell, S. P., and Hochwagen, A. (2007). Mapping of Meiotic Single-Stranded DNA Reveals Double-Strand-Break Hotspots Near Centromeres and Telomeres. Curr. Biol. 17, 2003–2012. doi:10.1016/j.cub.2007.10.066
Boulton, A., Myers, R. S., and Redfield, R. J. (1997). The Hotspot Conversion Paradox and the Evolution of Meiotic Recombination. Proc. Natl. Acad. Sci. U. S. A. 94, 8058–8063. doi:10.1073/pnas.94.15.8058
Brick, K., Smagulova, F., Khil, P., Camerini-Otero, R. D., and Petukhova, G. V. (2012). Genetic Recombination Is Directed Away from Functional Genomic Elements in Mice. Nature 485, 642–645. doi:10.1038/nature11089
Brick, K., Thibault-Sennett, S., Smagulova, F., Lam, K.-W. G., Pu, Y., Pratto, F., et al. (2018). Extensive Sex Differences at the Initiation of Genetic Recombination. Nature 561, 338–342. doi:10.1038/s41586-018-0492-5
Brion, C., Legrand, S., Peter, J., Caradec, C., Pflieger, D., Hou, J., et al. (2017). Variation of the Meiotic Recombination Landscape and Properties over a Broad Evolutionary Distance in Yeasts. PLoS Genet. 13, e1006917. doi:10.1371/journal.pgen.1006917
Brown, S. D., Audoynaud, C., and Lorenz, A. (2020). Intragenic Meiotic Recombination in Schizosaccharomyces Pombe is Sensitive to Environmental Temperature Changes. Chromosome Res. 28, 195–207. doi:10.1007/s10577-020-09632-3
Choi, K., and Henderson, I. R. (2015). Meiotic Recombination Hotspots - a Comparative View. Plant J. 83, 52–61. doi:10.1111/tpj.12870
Cotton, V. E., Hoffmann, E. R., Abdullah, M. F. F., and Borts, R. H. (2009). Interaction of Genetic and Environmental Factors in Saccharomyces Cerevisiae Meiosis: the Devil is in the Details. Methods Mol. Biol. 557, 3–20. doi:10.1007/978-1-59745-527-5_1
Ergoren, M. C. (2018). The Control of Meiotic Recombination in the Human Genome. Crit. Rev. Eukaryot. Gene Expr. 28, 187–204. doi:10.1615/critreveukaryotgeneexpr.2018024601
Fowler, K. R., Sasaki, M., Milman, N., Keeney, S., and Smith, G. R. (2014). Evolutionarily Diverse Determinants of Meiotic DNA Break and Recombination Landscapes across the Genome. Genome Res. 24, 1650–1664. doi:10.1101/gr.172122.114
Fulton, J. E., McCarron, A. M., Lund, A. R., Pinegar, K. N., Wolc, A., Chazara, O., et al. (2016). A High-Density SNP Panel Reveals Extensive Diversity, Frequent Recombination and Multiple Recombination Hotspots within the Chicken Major Histocompatibility Complex B Region between BG2 and CD1A1. Genet. Sel. Evol. 48, 1. doi:10.1186/s12711-015-0181-x
Gao, J., Davidson, M. K., and Wahls, W. P. (2009). Phosphorylation-Independent Regulation of Atf1-Promoted Meiotic Recombination by Stress-Activated, p38 Kinase Spc1 of Fission Yeast. PLoS One 4, e5533. doi:10.1371/journal.pone.0005533
Gerton, J. L., DeRisi, J., Shroff, R., Lichten, M., Brown, P. O., and Petes, T. D. (2000). Global Mapping of Meiotic Recombination Hotspots and Coldspots in the Yeast Saccharomyces Cerevisiae. Proc. Natl. Acad. Sci. U. S. A. 97, 11383–11390. doi:10.1073/pnas.97.21.11383
Grey, C., Baudat, F., and de Massy, B. (2018). PRDM9, a Driver of the Genetic Map. PLoS Genet. 14, e1007479. doi:10.1371/journal.pgen.1007479
Gutz, H. (1971). Site Specific Induction of Gene Conversion in Schizosaccharomyces Pombe. Genetics 69, 331–337. doi:10.1093/genetics/69.3.317
Heckman, D. S., Geiser, D. M., Eidell, B. R., Stauffer, R. L., Kardos, N. L., and Hedges, S. B. (2001). Molecular Evidence for the Early Colonization of Land by Fungi and Plants. Science 293, 1129–1133. doi:10.1126/science.1061457
Henderson, I. R., and Bomblies, K. (2021). Evolution and Plasticity of Genome-Wide Meiotic Recombination Rates. Annu. Rev. Genet. 55, 23–43. doi:10.1146/annurev-genet-021721-033821
Hirota, K., Hasemi, T., Yamada, T., Mizuno, K. I., Hoffman, C. S., Shibata, T., et al. (2004). Fission Yeast Global Repressors Regulate the Specificity of Chromatin Alteration in Response to Distinct Environmental Stresses. Nucleic Acids Res. 32, 855–862. doi:10.1093/nar/gkh251
Hirota, K., Hoffman, C. S., Shibata, T., and Ohta§, K. (2003). Fission Yeast Tup1-Like Repressors Repress Chromatin Remodeling at the fbp1+ Promoter and the ade6-M26 Recombination Hotspot. Genetics 165, 505–515. doi:10.1093/genetics/165.2.505
Hirota, K., Mizuno, K.-I., Shibata, T., and Ohta, K. (2008). Distinct Chromatin Modulators Regulate the Formation of Accessible and Repressive Chromatin at the Fission Yeast Recombination Hotspot ade6-M26. Mol. Biol. Cell 19, 1162–1173. doi:10.1091/mbc.e07-04-0377
Hirota, K., Steiner, W. W., Shibata, T., and Ohta, K. (2007). Multiple Modes of Chromatin Configuration at Natural Meiotic Recombination Hot Spots in Fission Yeast. Eukaryot. Cell 6, 2072–2080. doi:10.1128/ec.00246-07
Hyppa, R. W., Fowler, K. R., Cipak, L., Gregan, J., and Smith, G. R. (2014). DNA Intermediates of Meiotic Recombination in Synchronous S. Pombe at Optimal Temperature. Nucleic Acids Res. 42, 359–369. doi:10.1093/nar/gkt861
Jing, J.-L., Zhang, T., Wang, Y.-Z., and He, Y. (2019). Advances towards How Meiotic Recombination Is Initiated: A Comparative View and Perspectives for Plant Meiosis Research. Int. J. Mol. Sci. 20, 4718. doi:10.3390/ijms20194718
Kawakami, T., Mugal, C. F., Suh, A., Nater, A., Burri, R., Smeds, L., et al. (2017). Whole-Genome Patterns of Linkage Disequilibrium across Flycatcher Populations Clarify the Causes and Consequences of Fine-Scale Recombination Rate Variation in Birds. Mol. Ecol. 26, 4158–4172. doi:10.1111/mec.14197
Kohli, J., and Bähler, J. (1994). Homologous Recombination in Fission Yeast: Absence of Crossover Interference and Synaptonemal Complex. Experientia 50, 295–306. doi:10.1007/bf01924013
Kon, N., Krawchuk, M. D., Warren, B. G., Smith, G. R., and Wahls, W. P. (1997). Transcription Factor Mts1/Mts2 (Atf1/Pcr1, Gad7/Pcr1) Activates the M26 Meiotic Recombination Hotspot in Schizosaccharomyces Pombe. Proc. Natl. Acad. Sci. U. S. A. 94, 13765–13770. doi:10.1073/pnas.94.25.13765
Kon, N., Schroeder, S. C., Krawchuk, M. D., and Wahls, W. P. (1998). Regulation of the Mts1-Mts2-Dependent ade6-M26 Meiotic Recombination Hotspot and Developmental Decisions by the Spc1 Mitogen-Activated Protein Kinase of Fission Yeast. Mol. Cell. Biol. 18, 7575–7583. doi:10.1128/mcb.18.12.7575
Kong, A., Thorleifsson, G., Gudbjartsson, D. F., Masson, G., Sigurdsson, A., Jonasdottir, A., et al. (2010). Fine-Scale Recombination Rate Differences between Sexes, Populations and Individuals. Nature 467, 1099–1103. doi:10.1038/nature09525
Lam, I., and Keeney, S. (2014). Mechanism and Regulation of Meiotic Recombination Initiation. Cold Spring Harb. Perspect. Biol. 7, a016634. doi:10.1101/cshperspect.a016634
Lam, I., and Keeney, S. (2015). Nonparadoxical Evolutionary Stability of the Recombination Initiation Landscape in Yeast. Science 350, 932–937. doi:10.1126/science.aad0814
Lorenz, A., and Mpaulo, S. J. (2022). Gene Conversion: A Non-Mendelian Process Integral to Meiotic Recombination. Heredity. doi:10.1038/s41437-022-00523-3
Marand, A. P., Zhao, H., Zhang, W., Zeng, Z., Fang, C., and Jiang, J. (2019). Historical Meiotic Crossover Hotspots Fueled Patterns of Evolutionary Divergence in Rice. Plant Cell 31, 645–662. doi:10.1105/tpc.18.00750
Mihola, O., Landa, V., Pratto, F., Brick, K., Kobets, T., Kusari, F., et al. (2021). Rat PRDM9 Shapes Recombination Landscapes, Duration of Meiosis, Gametogenesis, and Age of Fertility. BMC Biol. 19, 86. doi:10.1186/s12915-021-01017-0
Mizuno, K.-i., Hasemi, T., Ubukata, T., Yamada, T., Lehmann, E., Kohli, J., et al. (2001). Counteracting Regulation of Chromatin Remodeling at a Fission Yeast cAMP Responsive Element-Related Recombination Hotspot by Stress-Activated Protein Kinase, cAMP-Dependent Kinase and Meiosis Regulators. Genetics 159, 1467–1478. doi:10.1093/genetics/159.4.1467
Mizuno, K., Emura, Y., Baur, M., Kohli, J., Ohta, K., and Shibata, T. (1997). The Meiotic Recombination Hot Spot Created by the Single-Base Substitution ade6-M26 Results in Remodeling of Chromatin Structure in Fission Yeast. Genes Dev. 11, 876–886. doi:10.1101/gad.11.7.876
Morgan, C. H., Zhang, H., and Bomblies, K. (2017). Are the Effects of Elevated Temperature on Meiotic Recombination and Thermotolerance Linked via the axis and Synaptonemal Complex? Phil. Trans. R. Soc. B 372, 20160470. doi:10.1098/rstb.2016.0470
Mougel, F., Poursat, M.-A., Beaume, N., Vautrin, D., and Solignac, M. (2014). High-Resolution Linkage Map for Two Honeybee Chromosomes: The Hotspot Quest. Mol. Genet. Genomics 289, 11–24. doi:10.1007/s00438-013-0784-2
Mukiza, T. O., Protacio, R. U., Davidson, M. K., Steiner, W. W., and Wahls, W. P. (2019). Diverse DNA Sequence Motifs Activate Meiotic Recombination Hotspots through a Common Chromatin Remodeling Pathway. Genetics 213, 789–803. doi:10.1534/genetics.119.302679
Muñoz-Fuentes, V., Di Rienzo, A., and Vilà, C. (2011). Prdm9, a Major Determinant of Meiotic Recombination Hotspots, Is Not Functional in Dogs and Their Wild Relatives, Wolves and Coyotes. PLoS One 6, e25498. doi:10.1371/journal.pone.0025498
Myers, S., Freeman, C., Auton, A., Donnelly, P., and McVean, G. (2008). A Common Sequence Motif Associated with Recombination Hot Spots and Genome Instability in Humans. Nat. Genet. 40, 1124–1129. doi:10.1038/ng.213
Otto, S. P. (2021). Selective Interference and the Evolution of Sex. J. Hered. 112, 9–18. doi:10.1093/jhered/esaa026
Paigen, K., and Petkov, P. M. (2018). PRDM9 and its Role in Genetic Recombination. Trends Genet. 34, 291–300. doi:10.1016/j.tig.2017.12.017
Pan, J., Sasaki, M., Kniewel, R., Murakami, H., Blitzblau, H. G., Tischfield, S. E., et al. (2011). A Hierarchical Combination of Factors Shapes the Genome-Wide Topography of Yeast Meiotic Recombination Initiation. Cell 144, 719–731. doi:10.1016/j.cell.2011.02.009
Parvanov, E., Kohli, J., and Ludin, K. (2008). The Mating-Type-Related Bias of Gene Conversion in Schizosaccharomyces Pombe. Genetics 180, 1859–1868. doi:10.1534/genetics.108.093005
Peñalba, J. V., and Wolf, J. B. W. (2020). From Molecules to Populations: Appreciating and Estimating Recombination Rate Variation. Nat. Rev. Genet. 21, 476–492. doi:10.1038/s41576-020-0240-1
Plough, H. H. (1917). The Effect of Temperature on Linkage in the Second Chromosome of Drosophila. Proc. Natl. Acad. Sci. U. S. A. 3, 553–555. doi:10.1073/pnas.3.9.553
Protacio, R. U., Mukiza, T. O., Davidson, M. K., and Wahls, W. P. (2022). Molecular Mechanisms for Environmentally Induced and Evolutionarily Rapid Redistribution (Plasticity) of Meiotic Recombination. Genetics 220 (2), iyab212. doi:10.1093/genetics/iyab212
Robert, T., Vrielynck, N., Mézard, C., de Massy, B., and Grelon, M. (2016). A New Light on the Meiotic DSB Catalytic Complex. Semin. Cell Dev. Biol. 54, 165–176. doi:10.1016/j.semcdb.2016.02.025
Sanchez, A., Reginato, G., and Cejka, P. (2021). Crossover or Non-Crossover Outcomes: Tailored Processing of Homologous Recombination Intermediates. Curr. Opin. Genet. Dev. 71, 39–47. doi:10.1016/j.gde.2021.06.012
Schield, D. R., Pasquesi, G. I. M., Perry, B. W., Adams, R. H., Nikolakis, Z. L., Westfall, A. K., et al. (2020). Snake Recombination Landscapes are Concentrated in Functional Regions Despite PRDM9. Mol. Biol. Evol. 37, 1272–1294. doi:10.1093/molbev/msaa003
Schuchert, P., Langsford, M., Käslin, E., and Kohli, J. (1991). A Specific DNA Sequence is Required for High Frequency of Recombination in the ade6 Gene of Fission Yeast. EMBO J. 10, 2157–2163. doi:10.1002/j.1460-2075.1991.tb07750.x
Shanfelter, A. F., Archambeault, S. L., and White, M. A. (2019). Divergent Fine-Scale Recombination Landscapes between a Freshwater and Marine Population of Threespine Stickleback Fish. Genome Biol. Evol. 11, 1573–1585. doi:10.1093/gbe/evz090
Singh, N. D. (2019). Wolbachia Infection Associated with Increased Recombination in Drosophila. G3 (Bethesda) 9, 229–237. doi:10.1534/g3.118.200827
Sipiczki, M. (2000). Where Does Fission Yeast Sit on the Tree of Life? Genome Biol. 1, REVIEWS1011. doi:10.1186/gb-2000-1-2-reviews1011
Spruce, C., Dlamini, S., Ananda, G., Bronkema, N., Tian, H., Paigen, K., et al. (2020). HELLS and PRDM9 Form a Pioneer Complex to Open Chromatin at Meiotic Recombination Hot Spots. Genes Dev. 34, 398–412. doi:10.1101/gad.333542.119
Stahl, F. W., Rehan, M. B. M., Foss, H. M., and Borts, R. H. (2016). Apparent Epigenetic Meiotic Double-Strand-Break Disparity in Saccharomyces cerevisiae: A Meta-Analysis. Genetics 204, 129–137. doi:10.1534/genetics.116.191635
Steiner, W. W., Davidow, P. A., and Bagshaw, A. T. M. (2011). Important Characteristics of Sequence-specific Recombination Hotspots in Schizosaccharomyces Pombe. Genetics 187, 385–396. doi:10.1534/genetics.110.124636
Steiner, W. W., Schreckhise, R. W., and Smith, G. R. (2002). Meiotic DNA Breaks at the S. Pombe Recombination Hot Spot M26. Mol. Cell 9, 847–855. doi:10.1016/s1097-2765(02)00489-6
Steiner, W. W., and Steiner, E. M. (2012). Fission Yeast Hotspot Sequence Motifs are Also Active in Budding Yeast. PLoS One 7, e53090. doi:10.1371/journal.pone.0053090
Steiner, W. W., Steiner, E. M., Girvin, A. R., and Plewik, L. E. (2009). Novel Nucleotide Sequence Motifs that Produce Hotspots of Meiotic Recombination in Schizosaccharomyces Pombe. Genetics 182, 459–469. doi:10.1534/genetics.109.101253
Storey, A. J., Wang, H.-P., Protacio, R. U., Davidson, M. K., Tackett, A. J., and Wahls, W. P. (2018). Chromatin-Mediated Regulators of Meiotic Recombination Revealed by Proteomics of a Recombination Hotspot. Epigenetics Chromatin 11, 64. doi:10.1186/s13072-018-0233-x
Sturtevant, A. H. (1917). Genetic Factors Affecting the Strength of Linkage in Drosophila. Proc. Natl. Acad. Sci. U. S. A. 3, 555–558. doi:10.1073/pnas.3.9.555
Sun, Y., Yuan, F., Wang, L., Dai, D., Zhang, Z., Liang, F., et al. (2022). Recombination and Mutation Shape Variations in the Major Histocompatibility Complex. J. Genet. Genomics. doi:10.1016/j.jgg.2022.03.006
Szankasi, P., Heyer, W.-D., Schuchert, P., and Kohli, J. (1988). DNA Sequence Analysis of the ade6 Gene of Schizosaccharomyces Pombe. Wild-Type and Mutant Alleles Including the Recombination Hot Spot Allele ade6-M26. J. Mol. Biol. 204, 917–925. doi:10.1016/0022-2836(88)90051-4
Thomas, L. (1979). The Medusa and the Snail : More Notes of a Biology Watcher. New York: Viking Press.
Tock, A. J., and Henderson, I. R. (2018). Hotspots for Initiation of Meiotic Recombination. Front. Genet. 9, 521. doi:10.3389/fgene.2018.00521
Tsai, I. J., Burt, A., and Koufopanou, V. (2010). Conservation of Recombination Hotspots in Yeast. Proc. Natl. Acad. Sci. U. S. A. 107, 7847–7852. doi:10.1073/pnas.0908774107
Wahls, W. P., and Davidson, M. K. (2010). Discrete DNA Sites Regulate Global Distribution of Meiotic Recombination. Trends Genet. 26, 202–208. doi:10.1016/j.tig.2010.02.003
Wahls, W. P., and Davidson, M. K. (2011). DNA Sequence-Mediated, Evolutionarily Rapid Redistribution of Meiotic Recombination Hotspots. Genetics 189, 685–694. doi:10.1534/genetics.111.134130
Wahls, W. P., and Davidson, M. K. (2012). New Paradigms for Conserved, Multifactorial, cis-Acting Regulation of Meiotic Recombination. Nucleic Acids Res. 40, 9983–9989. doi:10.1093/nar/gks761
Wahls, W. P., and Smith, G. R. (1994). A Heteromeric Protein that Binds to a Meiotic Homologous Recombination Hot Spot: Correlation of Binding and Hot Spot Activity. Genes Dev. 8, 1693–1702. doi:10.1101/gad.8.14.1693
White, M. A., Dominska, M., and Petes, T. D. (1993). Transcription Factors Are Required for the Meiotic Recombination Hotspot at the HIS4 Locus in Saccharomyces Cerevisiae. Proc. Natl. Acad. Sci. U. S. A. 90, 6621–6625. doi:10.1073/pnas.90.14.6621
White, M. A., Wierdl, M., Detloff, P., and Petes, T. D. (1991). DNA-Binding Protein RAP1 Stimulates Meiotic Recombination at the HIS4 Locus in Yeast. Proc. Natl. Acad. Sci. U. S. A. 88, 9755–9759. doi:10.1073/pnas.88.21.9755
Yadav, V. K., and Bouuaert, C. C. (2021). Mechanism and Control of Meiotic DNA Double-Strand Break Formation in S. Cerevisiae. Front. Cell Dev. Biol. 9, 642737. doi:10.3389/fcell.2021.642737
Yamada, T., Mizuno, K.-i., Hirota, K., Kon, N., Wahls, W. P., Hartsuiker, E., et al. (2004). Roles of Histone Acetylation and Chromatin Remodeling Factor in a Meiotic Recombination Hotspot. EMBO J. 23, 1792–1803. doi:10.1038/sj.emboj.7600138
Zelkowski, M., Olson, M. A., Wang, M., and Pawlowski, W. (2019). Diversity and Determinants of Meiotic Recombination Landscapes. Trends Genet. 35, 359–370. doi:10.1016/j.tig.2019.02.002
Keywords: meiosis, recombination evolution, recombination hotspot, linkage disequiblibrium, genetic mapping, evolution, Schizosaccharomyces pombe
Citation: Protacio RU, Davidson MK and Wahls WP (2022) Adaptive Control of the Meiotic Recombination Landscape by DNA Site-dependent Hotspots With Implications for Evolution. Front. Genet. 13:947572. doi: 10.3389/fgene.2022.947572
Received: 18 May 2022; Accepted: 01 June 2022;
Published: 22 June 2022.
Edited by:
Simon Whitehall, Newcastle University, United KingdomReviewed by:
Yasushi Hiraoka, Osaka University, JapanCopyright © 2022 Protacio, Davidson and Wahls. This is an open-access article distributed under the terms of the Creative Commons Attribution License (CC BY). The use, distribution or reproduction in other forums is permitted, provided the original author(s) and the copyright owner(s) are credited and that the original publication in this journal is cited, in accordance with accepted academic practice. No use, distribution or reproduction is permitted which does not comply with these terms.
*Correspondence: Wayne P. Wahls, d2FobHN3YXluZXBAdWFtcy5lZHU=
Disclaimer: All claims expressed in this article are solely those of the authors and do not necessarily represent those of their affiliated organizations, or those of the publisher, the editors and the reviewers. Any product that may be evaluated in this article or claim that may be made by its manufacturer is not guaranteed or endorsed by the publisher.
Research integrity at Frontiers
Learn more about the work of our research integrity team to safeguard the quality of each article we publish.