- 1Department of Biomedical Engineering, Marshall University, Huntington, WV, United States
- 2Department of Anesthesia, Indiana University Health Arnett Hospital, Lafayette, IN, United States
- 3Department of Cardiothoracic Surgery, Southcoast Health, Fall River, MA, United States
- 4Department of Pathology and Laboratory Medicine, Brown University, Providence, RI, United States
- 5National Institute of Mental Health, National Institute of Health, Bethesda, MD, United States
Symptoms of normal pressure hydrocephalus (NPH) and Alzheimer’s disease (AD) are somewhat similar, and it is common to misdiagnose these two conditions. Although there are fluid markers detectable in humans with NPH and AD, determining which biomarker is optimal in representing genetic characteristics consistent throughout species is poorly understood. Here, we hypothesize that NPH can be differentiated from AD with mRNA biomarkers of unvaried proximity to telomeres. We examined human caudate nucleus tissue samples for the expression of transient receptor potential cation channel subfamily V member 4 (TRPV4) and amyloid precursor protein (APP). Using the genome data viewer, we analyzed the mutability of TRPV4 and other genes in mice, rats, and humans through matching nucleotides of six genes of interest and one house keeping gene with two factors associated with high mutation rate: 1) proximity to telomeres or 2) high adenine and thymine (A + T) content. We found that TRPV4 and microtubule associated protein tau (MAPT) mRNA were elevated in NPH. In AD, mRNA expression of TRPV4 was unaltered unlike APP and other genes. In mice, rats, and humans, the nucleotide size of TRPV4 did not vary, while in other genes, the sizes were inconsistent. Proximity to telomeres in TRPV4 was <50 Mb across species. Our analyses reveal that TRPV4 gene size and mutability are conserved across three species, suggesting that TRPV4 can be a potential link in the pathophysiology of chronic hydrocephalus in aged humans (>65 years) and laboratory rodents at comparable ages.
Introduction
Normal pressure hydrocephalus (NPH), an abnormal accumulation of cerebrospinal fluid (CSF) in the cerebral ventricles with close to normal intracranial pressure (ICP) (Hill and Volpe, 1981), occurs as the flow of CSF is blocked in the brain and/or spinal cord (Zhang et al., 2019; Han et al., 2021; Tseng et al., 2022). NPH can be found in young adults but is most common in the elderly (Borzage et al., 2021; Buyukgok et al., 2021; Koo et al., 2021; Oike et al., 2021; Mechelli et al., 2022). There are two types of NPH, one (idiopathic NPH, iNPH) with an unknown cause, and another (secondary NPH, sNPH) resulting from a subarachnoid hemorrhage, head trauma, infection, tumor, or complications of surgery (Kiefer and Unterberg, 2012; Williams and Malm, 2016; Gavrilov et al., 2019; Tan et al., 2021; Thavarajasingam et al., 2021; Tseng et al., 2022). A previous survey described the prevalence of NPH as 11/100,000 in adults 19–64 years of age, and 175/100,000 in the elderly over 65 years of age (Isaacs et al., 2018). A more recent review on incidence reported overall crude rates of NPH from 0.07/100,000/year in people aged younger than 60 years to 1.2/1,000 per year in people aged older than 70 years (Zaccaria et al., 2020).
Alzheimer’s disease (AD) is the most common form of dementia and may contribute to roughly 70% of cases (GBD 2019 Dementia Forecasting Collaborators, 2022). In America, as many as 5.8 million individuals had AD in 2020 (Matthews et al., 2019). iNPH is estimated to constitute about 10% of the people diagnosed with a dementia-related disorder, which is expected to exceed 150 million by 2050 (Reeves et al., 2020). NPH and AD share multiple clinical and pathologic features such as amyloid beta (Aβ) deposition, cerebrovascular inflammation, impaired localization of aquaporin 4 (AQP-4), and sleep disturbances (Reeves et al., 2020).
Molecular biomarkers of NPH have been proposed and include amyloid precursor protein (APP) (Miyajima et al., 2013; Pyykko et al., 2014; Jingami et al., 2015; Kazui et al., 2016; Niermeyer et al., 2020; Torretta et al., 2021; Jeppsson et al., 2022) and angiogenic proteins such as vascular endothelial growth factor (VEGF) (Huang et al., 2016) and matrix metalloproteinases (Jeppsson et al., 2022) present in CSF. Markers involved in glymphatic clearance of CSF in the periventricular white matter of the brain have also been proposed (Weiss et al., 2019; Morales et al., 2021; Warsi and Ibrahim, 2021). However, there is no consensus as to whether these markers are specific enough to identify patients with NPH over other age-related conditions such as AD. At present, there is no specific clinically relevant biomarker differentiating NPH (Dewan et al., 2018) from normal aging (Zhang et al., 2021; de Souza et al., 2022; Palmer et al., 2022). Diagnosis remains primarily through neuroimaging with brain scans, which are used for screening, diagnostic, and monitoring purposes (Chatzidakis et al., 2008; Ringstad et al., 2017; Agerskov et al., 2020; Ugga et al., 2020; Virhammar et al., 2022).
The caudate nucleus, a pair of brain structures that reside on the striatal side lateral to the cerebral ventricles, forms part of the basal ganglia and control high-level functions such as planning, movement, and learning in humans (Vergara-Aragon et al., 2011; Karim et al., 2018; Sobue et al., 2018). Because glymphatic system dysfunction evidenced by reduced metabolic clearance appears in NPH and AD alike, the caudate nucleus received much attention as this is the site where the contrast agent is injected (Cserr and Ostrach, 1974) and removal of extracellular marker is monitored (Cserr et al., 1977). In a previous rabbit study by Del Bigio and Bruni, it was demonstrated that experimental hydrocephalus induced by silicone oil injection into the cisterna magna of juvenile rabbits caused a reduction in the number of capillaries in the periventricular region involving the caudate nucleus, while no changes were observed in large blood vessels (Del Bigio and Bruni, 1988). This finding on ‘capillaries’ suggests that vascular endothelia rather than pericytes and smooth muscle cells in the caudate nucleus, primarily contribute to the decreased cerebral blood flow observed in hydrocephalus of neonates and juveniles (Del Bigio and Bruni, 1988; da Silva et al., 1995) and in NPH of aged individuals (Bateman, 2009; Sakellaridis and Stavropoulos, 2010; Sedighi et al., 2014; Ziegelitz et al., 2016; Azuma et al., 2019). Furthermore, caudate structural abnormalities (DeVito et al., 2007), cognitive impairment (Peterson et al., 2019), and striatal dopamine deficit and motor impairment (Pozzi et al., 2021) highlighted the significance of the caudate nucleus in NPH of elderly individuals. The most common form of hydrocephalus in ‘adults’ is iNPH (Williams and Malm, 2016), which is often confused with AD due to similarities in symptoms (Graff-Radford, 2014; Yamada et al., 2016). Although the cognitive impairment can be confused between the NPH and AD, gait dysfunction is a primary differentiating feature, which is present in NPH but not in AD (He D. et al., 2021; He M. et al., 2021; Chunyan et al., 2021; Davis et al., 2021; Hnin et al., 2021; Jeong et al., 2021; Kuruvithadam et al., 2021; Morel et al., 2021; Nikaido et al., 2021; Tang et al., 2021; Yamada et al., 2021; Maier et al., 2022; Nikaido et al., 2022; Sun et al., 2022; Suzuki et al., 2022).
Abnormal tau proteins found in brains cells have been associated with AD and other neurodegenerative diseases (Iqbal et al., 2010). Mutations of genes encoding APP and the p. A152T variant of microtubule associated protein tau (MAPT) (Coppola et al., 2012) contribute to the pathogenesis of AD (Mullan et al., 1992) and amyloidogenesis (Di Fede et al., 2009). A673V APP mutations in human brains have shown that amyloid beta deposits are found in all areas of the cerebral cortex, including the caudate nucleus (Giaccone et al., 2010). Although fluid-derived beta-amyloid protein, total tau protein, and phosphorylated tau protein have all been proposed as biomarker proteins of AD (Mulder et al., 2010; Brickman et al., 2022). However, the presence of AD-specific APP but not MAPT (Trabzuni et al., 2012) mRNA, in the caudate nucleus remain elusive (De Meyer et al., 2010).
Transient receptor potential cation channel subfamily V member 4 (TRPV4) is an ion channel protein that is recently being studied as a potential target for congenital hydrocephalus (CH) of neonates. Pharmacological inhibition of TRPV4 in hydrocephalic rats (Shim et al., 2019) ameliorates ventriculomegaly (Hochstetler et al., 2020). In addition to having similar genomic features that are associated with high mutation rates (McKnight et al., 2021), adult mice lacking zinc finger CCHC domain-containing protein 8 (ZCCHC8) (Gable et al., 2019) develop hydrocephalus. Thromboxane A synthase 1 (TBXAS1) is a cytochrome P450 enzyme that catalyzes the conversion of prostaglandin H2 to thromboxane A2, and is a potent vasoconstrictor and inducer of platelet aggregation (Ramazi et al., 2019). TBXAS1 has been reported to improve blood flow and recovery from hindlimb ischemia in mice (Amano et al., 2016), and plays a role in cardiovascular disease, stroke, and dementia (Ramazi et al., 2019; Bhatia and Singh, 2021a; b). However, whether these molecules at the mRNA level can serve as biomarkers of NPH and/or AD remains unsubstantiated.
While a larger scale analysis of the whole transcriptome undoubtedly requires higher (at least six) replicates, we have embarked on an idea that downsizing into select genes would allow immediate screens of the unknown such as ZCCHC8 as compared to a widely investigated gene like APP, given the repetitive failures of clinical trials for therapies of AD (Anderson et al., 2017).
The goal of this study was to quantify relative mRNA levels of TRPV4, ZCCHC8, VEGF, TBXAS1, MAPT, and APP, in postmortem human caudate nucleus samples from elderly (>66 years of age) cadaveric donors previously diagnosed with NPH and AD as compared to unaffected controls. We also examined the mutable characteristics of the various genes across species, comparing human, mouse, and rat genomes. Finally, we investigated across species whether mRNA expressions of these genes correlate with their relative mutability through two factors associated with high mutation rates.
Results
We investigated the mRNA expression of six genes relative to the internal reference, GAPDH, in caudate nucleus samples harvested from postmortem brains of aged humans with NPH. These tissues had been preserved as frozen samples and were obtained from multiple repositories of the NIH NBB (Figure 1A). Expression patterns of the six genes from the set of samples that arrived from the biobank were assessed using RT-PCR (n = 7 per group). All six genes of interest appeared to demonstrate differential mRNA expressions in NPH compared to control samples (Figure 1B). In a subsequent image analysis on DNA agarose gels (Figure 1C), we found that mRNA expressions of TRPV4 and MAPT were significantly elevated in the caudate nucleus samples of NPH (n = 7) as compared to those of unaffected controls (n = 7) (Figures 1C,D).
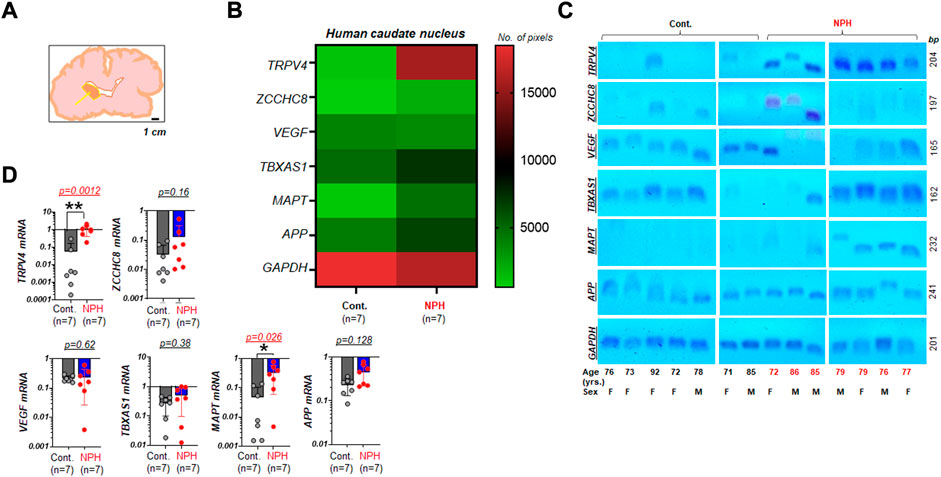
FIGURE 1. Elevated TRPV4 mRNA in the caudate nucleus with NPH (A) A simplified illustration indicating the neuroanatomical location where human postmortem tissue was harvested for assays (arrow: the caudate nucleus). The illustration was redrawn following the photograph taken at one of NeuroBioBank (NBB) tissue repositories. Scale bar, 1 cm (B) a color-coded map illustrating the summary of the assay result on expression levels of TRPV4 and other genes associated with NPH in the caudate nucleus of humans with unaffected control (Cont.) and NPH, respectively. Note that 6 genes of interest and 1 housekeeping gene (GAPDH) were marked on the left y axis. The number of pixels from ImageJ with coded color was shown on the right. n = 7 per group. (C) Agarose gels displaying mRNA expressions of TRPV4, ZCCHC8, VEGF, TBXAS1, MAPT, and APP relative to GAPDH in the caudate nucleus of aged human postmortem brains classified as Cont. (n = 7) and NPH (n = 7). yrs., years (D) bar graphs with scatter plots summarizing mRNA expressions of select genes relative to GAPDH in NPH (n = 7) as compared to those of unaffected controls (n = 7). **, p < 0.01; *, p < 0.05 by Mann-Whitney test.
We next tested whether those genes assayed for NPH (Figure 1) are differentially regulated in the caudate nucleus of aged humans with AD. In the mRNA assays by RT-PCR with the set of samples obtained from the biobank, several genes of interest appeared to demonstrate altered gene expressions in NPH and/or AD as compared to unaffected controls (Figure 2A). In the image analyses of DNA agarose gels (Supplementary Figure S1), we found that mRNA expressions of ZCCHC8, TBXAS1, MAPT, and APP in AD (n = 5) were significantly elevated as compared to those of controls (n = 7). Unlike these four genes, mRNA level of VEGF was not significantly altered as compared to that of controls. TRPV4 mRNA in the caudate nucleus was elevated in patients with NPH but not in the cases with AD as compared to unaffected controls (Figure 2B).
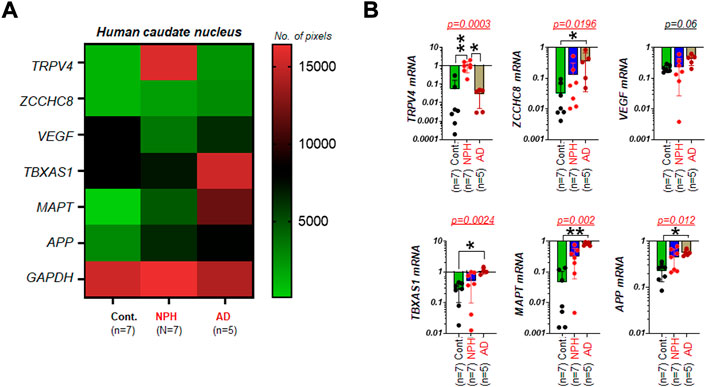
FIGURE 2. TRPV4 mRNA in the caudate nucleus is elevated in NPH but not in AD (A) A color-coded map illustrating the summary of the mRNA assay result on expression levels of TRPV4 and five other genes associated with either NPH or AD in the caudate nucleus of humans with unaffected control (Cont.), NPH, and AD, respectively. The number of pixels from ImageJ with coded color was shown on the right. Note that TRPV4 and VEGF mRNA were not altered in AD as compared controls. N = 5-7 per group. (B) bar graphs with scatter plots summarizing mRNA expressions of multiple genes relative to GAPDH in AD (n = 5) as compared to those of Cont. (n = 7). **, p < 0.01; *, p < 0.05 by Kruskal-Wallis test.
We have previously shown that there are two factors based on gene characteristics that are associated with high mutation rates (Nusbaum et al., 2006), and can be used to assess the relative mutability of genes associated with various human diseases (Lucas et al., 2021; McKnight et al., 2021) and those targeted by specific drugs (Raines et al., 2022). These two factors are proximity to telomeres and high A + T content and were proposed in studies of human chromosomes (Nusbaum et al., 2006). Using these two factors as a screening tool, we asked if the mutability of the six genes of interest with one housekeeping gene we assayed from the caudate nucleus of human samples are similar or different across mammalian species. Using NCBI genome resources (txid9606 [orgn]), we organized the chromosome count of humans and other mammalian species such as mouse, rat, dog, horse, goat, sheep, zebrafish, orangutan, and rabbit, which are frequently used in the laboratory investigation. Due to differences in chromosomal numbers, the organized data suggest that the chromosomal location of specific genes could vary widely across species. For instance, orangutans and rabbits have similar chromosome counts as humans at 46 (23 pairs) while dogs have roughly twice as many chromosomes (Figure 3A).
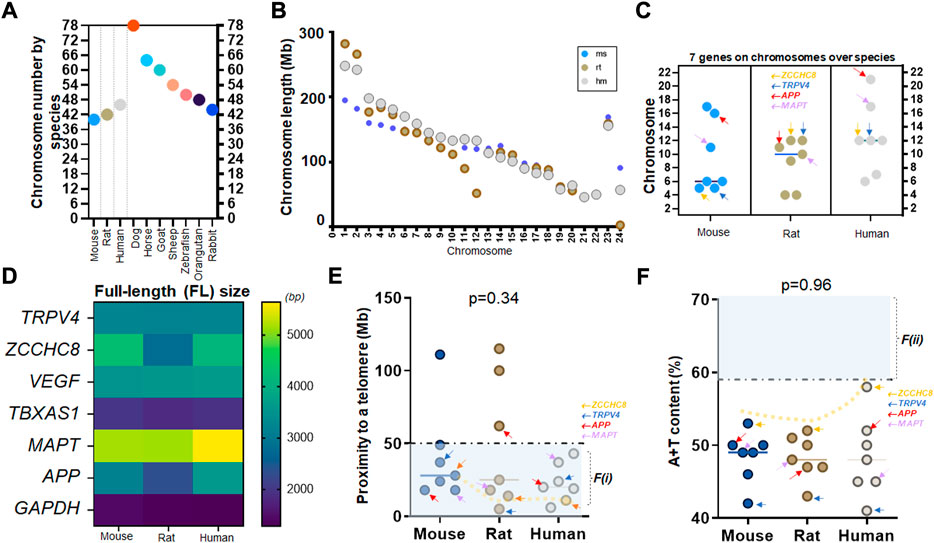
FIGURE 3. A consistent mutability of TRPV4 over mouse, rat, and human genomes (A) Chromosome numbers of frequently used laboratory animals (mice and rats) and humans as compared to other species such as dogs to rabbits. Note that orangutan (48) and rabbit (44) have similar numbers to humans at 46 chromosomes (23 pairs) while dogs are much higher at 78. (B) Scatter plots showing chromosome length of three species with respect to each chromosome (chr) of chr 1 to X or Y; mice (ms), rats (rt), and humans (hm). Note that larger animals do not always show longer chromosomes. In chr 1, rats, which are smaller in body size, have longer nucleotide sizes than humans. x-axis is pair-wise; humans showing 23 pairs from chr 1 to chr X or Y (Morton, 1991) (C) Locations of seven genes investigated in this study over three species of mouse, rat, and human. Note that APP and MAPT have evolved into shorter chromosomes (chr17-22) in humans as compared to rodents (D) The full-length (FL) size of seven genes over three species. Note that TRPV4, VEGF, and TBXAS1 show consistent molecular sizes over three species while ZCCHC8, APP, and MAPT differ in humans as compared to rodents. (E) Proximity to telomeres of seven genes over three species. Note that seven human genes investigated in this study have evolved in a way meeting proximity to telomeres or the first factor, F(i), associated with high mutation rate as all seven human genes are at less than 50 Mb as compared to those of mice and rats. (F) A+T content of seven genes over three species. Seven human genes demonstrate a similar characteristic of difficulty in meeting this second factor, F(ii), associated with high mutation rate while ZCCHC8 gene has evolved towards 59%. A dotted trend line follows ZCCHC8 gene in mice, rats, and humans (E,F). Arrows in orange, blue, red, and purple indicating ZCCHC8, TRPV4, APP, and MAPT, respectively (C,E,F).
To gain a better understanding of the association of telomere proximity with physical characteristics in various species, we first plotted the nucleotide or transcript length of chromosomes between mice, rats, and humans, and showed that body size is not necessarily linearly associated with chromosome size. Evidently, chromosome #1 (chr 1) is the longest among all chromosomes in these three species but the chromosome length by species does not conform to the rank order of the body size. In other words, even though humans are significantly bigger than rats, the length of chr 1 in rats (∼300 Mb) is longer than that of humans (∼250 Mb) (Figure 3B). We then compared the distributions of the six genes of interest and one housekeeping gene in three species: mice, rats, and humans (Supplementary Tables S1.1–S1.3). Given that the three species share similar counts of chromosome pairs at 20 (mouse), 21 (rat), and 23 (human), we found that genes were on different chromosomes depending on species. Taking the observed peak value (chr 21 for APP, marked in red arrows) in humans for example, the same gene is at chr 16 in mice, while APP gene in rats is at chr 11 (arrows in red; Figure 3C). Two known fluid biomarker genes of AD, APP and MAPT, are found on much shorter chromosomes in humans compared to mice and rats. (Figures 3B,C). Contrastingly, the location of ZCCHC8 and TRPV4 genes, did not jump to chr 20 or higher (relatively shorter chromosomes) in the human genome, suggesting no abrupt relocation from the longer sized chromosomes (chr 1–8) to the intermediate ones (chr 9–16) in mouse and rat genome (Figure 3C).
We next assessed the nucleotide (transcript) size of the seven genes in mice, rats, and humans. By color-coding, we found that TRPV4, VEGF, TBXAS1, and GAPDH nucleotide size are consistent over the three species (Figure 3D). Two known fluid markers of AD, MAPT and APP demonstrated a longer nucleotide size in the human genome as compared to mice and rats (Figure 3D). Assessments of proximity to telomeres suggest that while most genes across species satisfied proximity to telomeres at <50 Mb, it was only in humans where all seven genes met this criterion (Figure 3E). Although one nucleotide difference in mouse and human isoform such as VEGF-A164 in mice pertaining to VEGF-A165 in humans is well appreciated, elongated nucleotide sizes in APP and MAPT of humans compared to mice and rats were suggestive of higher GC content in APP and MAPT gene. Indeed, APP gene showed the highest A + T content in human genome as compared to mouse and rat genome (Figure 3F).
Next, we examined the alterations of two factors in seven genes we assayed for NPH and AD over three species. Using color-coded plots, we organized these genes relative to chromosomal location, proximity to telomeres, and A + T content in mouse, rat, and human genome. We found that chromosomal characteristics of VEGF, TBXAS1, and MAPT differ depending on species (Figures 4A–C). As to the genetic mutability identified by proximity to telomeres, mouse TBXAS1 gene was relatively less mutable as this gene failed to satisfy F(i) (Figure 4D). In the rat genome, as we assessed the mutability of seven genes by proximity to telomeres, TBXAS1, APP, and VEGF were relatively less mutable as these three genes did not meet F(i) (Figure 4E). In human chromosomes, seven genes were relatively much more mutable by proximity to telomeres as these genes satisfied F(i). The mutability of seven genes demonstrated a consistent trend by A + T content as all genes failed to satisfy F(ii) over species (Figures 4D–F). In humans, TBXAS1, APP, and VEGF were located in more proximity to their telomeres at <50 Mb as compared to rodent genomes, suggesting that proximity to telomeres of these genes are not consistent when it comes to human chromosomes as compared to those of mice and rats (Figure 4F).
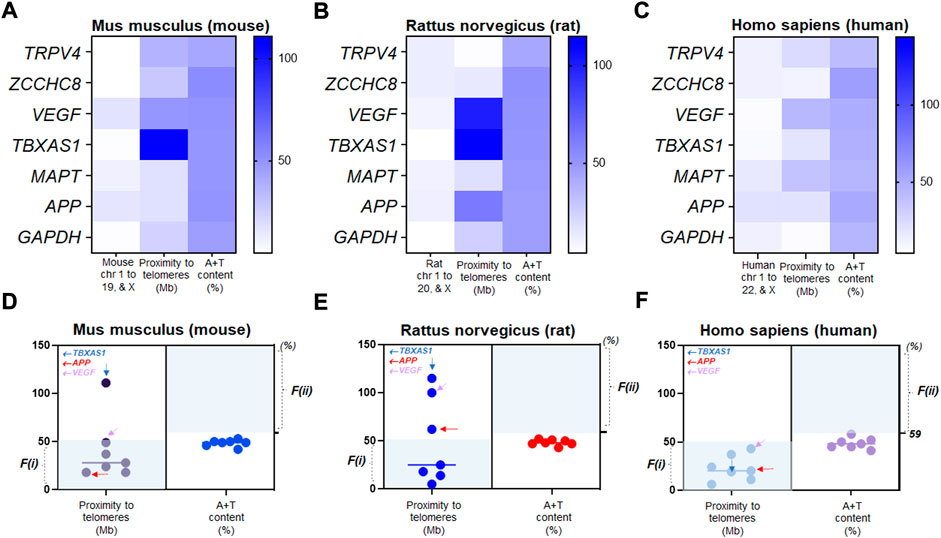
FIGURE 4. Relative mutability in human genes driven by proximity to telomeres (A) The color-coded map showing chromosomal location, distance to a telomere, and A+T content of Mus musculus (mouse) TRPV4 and other genes in chromosome numbers (chr 1 to 19 and X), proximity to telomeres (Mb), and A+T content (%). (B) the color-coded map showing chromosomal location, distance to a telomere, and A+T content of Rattus norvegicus (rat) TRPV4 and other genes in chromosome numbers (chr 1 to 20 and X), proximity to telomeres (Mb), and A+T content (%). (C) the color-coded map showing chromosomal location, distance to a telomere, and A+T content of Homo sapiens (human) TRPV4 and other genes in chromosome numbers (chr 1 to 22 and X), proximity to telomeres (Mb), and A+T content (%). (D) Scatter plots displaying relative mutability of seven mouse genes by proximity to telomeres (left) and A+T content (right). Shaded regions with light colors (blue) indicate genes satisfying either of the two factors. Genes outside the shaded regions at n = 2 by F(i); n = 7 by F(ii)) are relatively less mutable. (E) Scatter plots displaying relative mutability of seven rat genes by proximity to telomeres (left) and A+T content (right). Shaded regions with light colors (blue) indicate genes satisfying either of the two factors. Genes outside the shaded regions at n = 3 by F(i); n = 7 by F(ii)) are relatively less mutable. (F) Scatter plots displaying relative mutability of the same seven genes by proximity to telomeres (left) and A+T content (right) in human chromosomes. Shaded regions with light colors (blue) indicate human genes satisfying either of the two factors. Genes outside the shaded regions at n = 0 by F(i); n = 7 by F(ii)) are relatively less mutable. Note that all human genes satisfied proximity to telomeres, F(i), while none met F(ii).
To further investigate TRPV4 localization in human cells, we cultured vascular EC line and culture over 14 days (Supplementary Figure S2). When TRPV4 was co-labeled with α tubulin, a marker for microtubules, areas of the TRPV4 positive immunofluorescence were conversely associated with areas of the α tubulin positive immunoreactivity in these cells in vitro (Supplementary Figures S2A,B). This finding was consistent with an inverse relationship between the microtubule length in radially spanning elliptic formation of α tubulin in the cytoplasm and DAPI-positive cell numbers over 14 days of the culture (Supplementary Figures S2C,D). This finding on microtubule elongation/shortening in TRPV4-positive cellular phenotype was consistent with our observation of ependymal cilia in neonatal rat brains in vivo (Shim et al., 2019). In human vascular ECs, there were two distinct patterns of TRPV4 proteins near cytoplasm and cell nucleus. Specifically, the area of TRPV4-positive immunoreactivity was significantly decreased (p = 0.002) in human ECs with nuclear TRPV4 as compared to those with cytoplasmic TRPV4 (Supplementary Figures S2E–J).
Discussion
We demonstrated from mRNA assays of the caudate nucleus specimens obtained from aged cadavers that TRPV4 has the potential to be a novel tissue marker of NPH, although follow-up studies with a larger same size are warranted (Figure 1). Moreover, TRPV4 expression is specifically elevated in NPH but not in AD. Our finding of elevated expression of APP in AD caudate nucleus is consistent with the expected elevations of these markers in the plasma or CSF of individuals with AD (Brickman et al., 2022). The finding that MAPT mRNA is elevated in the caudate nucleus from NPH as well as AD patients suggests that NPH and AD are not differentiated with MAPT alone, if used as an mRNA biomarker. Additionally, the elevated TBXAS1 mRNA expression levels we found in these AD tissues bolsters the finding of the previous report (Grubman et al., 2019) (Figure 2).
While the plethora of recent articles can be retrieved as one uses the search keyword of ‘biomarker and hydrocephalus’ (Nakajima et al., 2010; Miyajima et al., 2013; Pyykko et al., 2014; Jingami et al., 2015; Kazui et al., 2016; Nagata et al., 2017; Abu-Rumeileh et al., 2019; Niermeyer et al., 2020; Nakajima et al., 2021; Torretta et al., 2021; Daly et al., 2022; Jeppsson et al., 2022; Mazzeo et al., 2022), we have not yet found literature focusing on ‘mRNA biomarker and hydrocephalus’. Instead, there were two recent articles related to ‘mRNA biomarker and Alzheimer’s (Fiala et al., 2011; Isik and Beydemir, 2022), suggesting that most biomarker studies on iNPH and/or sNPH might be designed through fluid or CSF assays (Nakajima et al., 2010; Miyajima et al., 2013; Pyykko et al., 2014; Jingami et al., 2015; Kazui et al., 2016; Nagata et al., 2017; Abu-Rumeileh et al., 2019; Niermeyer et al., 2020; Nakajima et al., 2021; Torretta et al., 2021; Daly et al., 2022; Jeppsson et al., 2022; Mazzeo et al., 2022). This highlights the novelty and utility of tissue assays in which mRNA markers were pursued with the caudate nucleus.
In our unbiased assortation of chromosome numbers in ten species, we found, as expected, that primates (orangutans) have chromosome numbers consistent with those of humans. However, dogs, a species commonly used for neurosurgical studies (Dombrowski et al., 2006; Park et al., 2010; Gomes et al., 2020b; a;Hasegawa, 2020; Olszewska et al., 2020; Ito et al., 2021; Lee et al., 2021), have significantly more chromosomes (78), suggesting reconsiderations regarding the appropriateness of the canine model for certain genetic studies.
We also report that one of the highly used housekeeping genes, GAPDH, maintains a consistent nucleotide size across mouse, rat, and human genomes, along with TRPV4, VEGF, and TBXAS1 genes (Figure 3). In evaluating the mutability factor F(i) (Lucas et al., 2021; McKnight et al., 2021; Raines et al., 2022) in the three species assessed, we did not expect biomarker signatures or mutability of TBXAS1, APP, and VEGF genes to be similar in mice and rats as compared to humans. However, contrarily, mutability of MAPT, ZCCHC8, and TRPV4 genes were found to be consistent across mice, rats, and humans (Figures 4E,F) except molecular sizes (Figure 4D).
The findings of clearly elevated MAPT and APP mRNA in AD samples compared to controls suggest the specificity of tau protein and amyloid beta as potential caudate nucleus markers of AD. Although the antibody-based detection method is still available, the diagnostic utility of PCR has risen since the COVID-19 pandemic. Our mRNA assay with the caudate nucleus suggests that MAPT, when combined with multiple genes such as APP and TBXAS1, is a more specific way of distinguishing AD compared to using only a single or dual markers. Our data, based on human caudate nucleus tissue samples from postmortem brains provide useful insight into novel potential tissue markers of AD, Firstly, TBXAS1 mRNA, a marker of platelet aggregation or atheroprone vasculature (Ramazi et al., 2019) is positively detected in all caudate nucleus samples of AD, while TBXAS1 mRNA is not always detectable in controls. Secondly, mRNA of VEGF-A165, which is indicative of dysfunctional vasculatures or insufficient angiogenesis (Shim and Madsen, 2018) required for the maintenance of adult brains (Echeverria et al., 2017), did not alter in the caudate nucleus from NPH and AD alike. When unaffected control tissue (the caudate nucleus) is not available but specimens from patients such as those with AD and NPH are available, MAPT mRNA in combination with APP, TBXAS1, and ZCCHC8 can distinguish AD with higher accuracy than with a single biomarker.
Here, the utility of the caudate nucleus as a target specimen for mRNA marker detection is demonstrated but the assay should be extended to other regions of the brain to overcome the inherent limitation. In AD, there are prior studies measuring mRNA or microRNA in the blood (Kester et al., 2011; Lu et al., 2018) or CSF (Gui et al., 2015). We confirmed the previous finding that MAPT and APP mRNA are elevated in the caudate nucleus (tissue), consistent with fluid assays of AD (Musiek and Holtzman, 2012; Lafirdeen et al., 2019; McLimans et al., 2019; Barthelemy et al., 2020; Shen et al., 2020; Shen et al., 2021). With the sample size applied in the current findings, it is not yet reasonable to predict or utilize other mRNA markers as a diagnostic tool for AD (compared to control) given the number of other studies that have examined far larger cohort sizes (Georganopoulou et al., 2005; Holtta et al., 2013). Although assaying mRNA in the blood (Ekici et al., 2010) or CSF with NPH has been rarely reported, we found that an attempt has been made to detect microRNA in the CSF of patients with congenital hydrocephalus (Chen et al., 2022). Nevertheless, we envision that the data presented in this report on a more specific marker gene like TRPV4 and MAPT in NPH can significantly improve the prior cases reporting misdiagnosis of either AD or NPH (Joseph et al., 2006; Joseph et al., 2008; Lee et al., 2010; Shimada et al., 2011). Biomechanical aspects of intracranial compliance (Gholampour et al., 2022) should also be considered in the future as well for NPH and AD biomarker studies.
There are immunological cells such as T lymphocytes in the CSF (Evans et al., 2019) that can be analyzed by single cell techniques (Esaulova et al., 2020; Guan et al., 2022; Piper et al., 2022; You et al., 2022). To study CSF and compare that with the brain tissue finding is thus highly relevant. This is important since one cannot yet expect deep brain biopsy (Yu et al., 1998; Yamada et al., 2004; Moriuchi et al., 2011; Ritz et al., 2011; Ooba et al., 2013; Iijima et al., 2015; Wang et al., 2016; Arakawa et al., 2020; Qin et al., 2021; Tang and Wang, 2021; Zhu et al., 2021) to be a realistic diagnostic tool to distinct either iNPH or sNPH from AD. Furthermore, there are already less invasive biomarkers, from brain imaging and CSF, available for that (Nakajima et al., 2010; Torretta et al., 2021; Jeppsson et al., 2022; Mazzeo et al., 2022). To suggest TRPV4 as a biomarker for differential diagnostics, it is thought that there should be a larger replication cohort.
In conclusion, TRPV4 mRNA in the caudate nucleus is elevated in NPH but not in AD. The result suggests that TRPV4 can be a potential link in the pathophysiology of chronic hydrocephalus in aged humans (>65 years) and laboratory rodents at comparable ages.
Methods
Human postmortem tissues
Postmortem tissues were requested from the National Institute of Health (NIH) NeuroBioBank (NBB), USA over a period of 1 year. We collected the postmortem tissues of aged individuals through multiple repositories of the NIH NBB, which provided the caudate nucleus (DeVito et al., 2007; Deshpande et al., 2009; Jang et al., 2017; Peterson et al., 2019) (Figure 1A) in a frozen state. Caudate nucleus specimens in frozen state were transported to our lab. Per the record provided by the NBB, the specimens were collected at postmortem intervals of 16 ± 8 h (mean ± std; range 4–25 h after death, n = 7 in unaffected controls; n = 7 in NPH; n = 5 in AD). Inclusion criteria and diagnosis are provided in Supplementary Tables S2, S3. Seven male and twelve female brain specimens are used, where sex is noted in Supplementary Figure S1 and Supplementary Table S3.
Primer design
We designed the primers for six genes of interest with one housekeeping gene based on the prior reports (Giaccone et al., 2010; Trabzuni et al., 2012; Mesitskaya et al., 2018; Shim and Madsen, 2018; Gable et al., 2019; Cacabelos, 2020; Hochstetler et al., 2020). Human gene transcripts were searched using Ensembl database (http://useast.ensembl.org/index.html). Using Primer3 online, we determined the sequences of a specific exon per gene transcript (https://bioinfo.ut.ee/primer3-0.4.0/). Then, lyophilized forms were manufactured and provided by the vendor (Thermofisher scientific, Waltham, MA). Seven human gene primers were designed (Supplementary Table S4).
Total RNA isolation and cDNA generation
Total RNA was isolated from the caudate nucleus of the unaffected control, NPH, and AD specimens using QIA-ZOL based RNA isolation kit (RNeasy Lipid Tissue Mini Kit, QIAGEN). Concentration and quality of samples were analyzed with a NanoDrop spectrophotometer (Thermofisher). Total RNA (500 ng/reaction) was reverse-transcribed using the High-Capacity RNA-to-cDNA Kit (Thermofisher; Catalog number: 4368814) at ABI SimpliAmp Thermal Cycler System (Thermofisher).
Reverse transcription polymerase chain reaction
RT-PCR was carried out in 25 μL containing 250 ng cDNA following the manufacturer’s instructions (GoTaq®Green Master Mix). Cycling conditions were composed of three steps: Denaturation at 95 °C for 2 min, followed by 32 cycles of denaturation at 95°C for 30 s, annealing at 60 °C for 30 s, and extension at 72 °C for 30 s (Promega, Madison, WI). The PCR products were then separated by electrophoresis on horizontal 1.25% agarose gels in 1x Tris/boric acid/EDTA (TBE) buffer and visualized by staining with Maestro dye (MaestroSafe, Maestrogen). The fluorescent signal was photographed with the built-in camera of an iPhone 12 (Apple).
ImageJ analysis
The image analysis of the DNA agarose gels was conducted using NIH ImageJ. The process is composed of six steps: 1) Open gel image at ImageJ, 2) Select with rectangle, 3) Analyze-gels-1st lane and the next until the end, 4) Analyze-gels-plot lanes, 5) Connect with straight lines, and 6) Select with points. The area calculated per each band was collected in the result file and saved in the spreadsheet. The relative fold change for each gene of interest was quantified with respect to the housekeeping gene (Schneider et al., 2012; Shim et al., 2013; Shim et al., 2016).
Statistical analysis
Statistical analyses were performed using Prism (version 9.3.0, GraphPad Software Inc.), enabling the generation of a heatmap plot and bar graphs of the data obtained during analysis with ImageJ. The non-parametric test was employed as this approach can reach a more conservative conclusion than a parametric test. As a result, a Mann-Whitney test and Kruskal-Wallis test were used for two group and three group comparisons, respectively. The difference between data sets was considered significant at p < 0.05; p values are identified in the figures and legends as *p < 0.05, **p < 0.01, and ***p < 0.005.
Data availability statement
The original contributions presented in the study are included in the article/Supplementary Material, further inquiries can be directed to the corresponding author/s.
Author contributions
All authors listed have made a substantial, direct, and intellectual contribution to the work and approved it for publication.
Funding
This work was supported by the state of West Virginia startup fund to faculty members at Marshall University (to JS). This research was also made possible by the NASA Established Program to Stimulate Competitive Research, Grant # 80NSSC22M0027 (to JS). The Genomics Core is supported by funding from the WV-INBRE grant (NIH P20GM103434), the COBRE ACCORD grant (P20GM121299) and the West Virginia Clinical and Translational Science Institute (WV-CTSI) grant (2U54GM104942).
Acknowledgments
We thank Regan Raines and Joshua Conrad for valuable comments on the manuscript. We wish to acknowledge the Marshall University Genomics Core for providing access to shared instrumentation. We thank for the Faculty Research and Travel award by the West Virginia Space Grant Consortium (WVSGC) sponsored by NASA. We also acknowledge the receipt of postmortem tissues from four repositories of the NIH NBB: 1) The University of Maryland, 2) Sepulveda VA Medical Center (Los Angeles, CA), 3) The Mount Sinai Hospital (Bronx, NY), and 4) McLean Hospital and Harvard Medial School (Belmont, MA).
Conflict of interest
The authors declare that the research was conducted in the absence of any commercial or financial relationships that could be construed as a potential conflict of interest.
Publisher’s note
All claims expressed in this article are solely those of the authors and do not necessarily represent those of their affiliated organizations, or those of the publisher, the editors and the reviewers. Any product that may be evaluated in this article, or claim that may be made by its manufacturer, is not guaranteed or endorsed by the publisher.
Supplementary material
The Supplementary Material for this article can be found online at: https://www.frontiersin.org/articles/10.3389/fgene.2022.936151/full#supplementary-material
References
Abu-Rumeileh, S., Giannini, G., Polischi, B., Albini-Riccioli, L., Milletti, D., Oppi, F., et al. (2019). Revisiting the cerebrospinal fluid biomarker profile in idiopathic normal pressure hydrocephalus: The bologna pro-hydro study. J. Alzheimers Dis. 68, 723–733. doi:10.3233/JAD-181012
Agerskov, S., Arvidsson, J., Ziegelitz, D., Lagerstrand, K., Starck, G., Bjorkman-Burtscher, I. M., et al. (2020). MRI diffusion and perfusion alterations in the mesencephalon and pons as markers of disease and symptom reversibility in idiopathic normal pressure hydrocephalus. PLoS One 15, e0240327. doi:10.1371/journal.pone.0240327
Amano, H., Nakamura, M., Ito, Y., Kakutani, H., Eshima, K., Kitasato, H., et al. (2016). Thromboxane A synthase enhances blood flow recovery from hindlimb ischemia. J. Surg. Res. 204, 153–163. doi:10.1016/j.jss.2016.04.011
Anderson, R. M., Hadjichrysanthou, C., Evans, S., and Wong, M. M. (2017). Why do so many clinical trials of therapies for Alzheimer's disease fail? Lancet 390, 2327–2329. doi:10.1016/S0140-6736(17)32399-1
Arakawa, A., Saito, Y., Seki, T., Mitsutake, A., Sato, T., Katsumata, J., et al. (2020). Corticobasal degeneration with deep white matter lesion diagnosed by brain biopsy. Neuropathology 40, 287–294. doi:10.1111/neup.12638
Azuma, S., Kazui, H., Kanemoto, H., Suzuki, Y., Sato, S., Suehiro, T., et al. (2019). Cerebral blood flow and Alzheimer's disease-related biomarkers in cerebrospinal fluid in idiopathic normal pressure hydrocephalus. Psychogeriatrics 19, 527–538. doi:10.1111/psyg.12435
Barthelemy, N. R., Bateman, R. J., Hirtz, C., Marin, P., Becher, F., Sato, C., et al. (2020). Cerebrospinal fluid phospho-tau T217 outperforms T181 as a biomarker for the differential diagnosis of Alzheimer's disease and PET amyloid-positive patient identification. Alzheimers Res. Ther. 12, 26. doi:10.1186/s13195-020-00596-4
Bateman, G. A. (2009). Cerebral blood flow and hydrocephalus. J. Neurosurg. Pediatr. 3, 244. author reply 245. doi:10.3171/2008.11.17594
Bhatia, P., and Singh, N. (2021a). Ameliorative effect of ozagrel, a thromboxane A2 synthase inhibitor, in hyperhomocysteinemia-induced experimental vascular cognitive impairment and dementia. Fundam. Clin. Pharmacol. 35, 650–666. doi:10.1111/fcp.12610
Bhatia, P., and Singh, N. (2021b). Thromboxane A2 synthase inhibition ameliorates endothelial dysfunction, memory deficits, oxidative stress and neuroinflammation in rat model of streptozotocin diabetes induced dementia. Physiol. Behav. 241, 113592. doi:10.1016/j.physbeh.2021.113592
Borzage, M., Saunders, A., Hughes, J., Mccomb, J. G., Bluml, S., and King, K. S. (2021). The first examination of diagnostic performance of automated measurement of the callosal angle in 1856 elderly patients and volunteers indicates that 12.4% of exams met the criteria for possible normal pressure hydrocephalus. AJNR. Am. J. Neuroradiol. 42, 1942–1948. doi:10.3174/ajnr.A7294
Brickman, A. M., Manly, J. J., Honig, L. S., Sanchez, D., Reyes-Dumeyer, D., Lantigua, R. A., et al. (2022). Correlation of plasma and neuroimaging biomarkers in Alzheimer's disease. Ann. Clin. Transl. Neurol. 9, 756–761. doi:10.1002/acn3.51529
Buyukgok, D., Ozdemir, O., Unal, T. C., and Barlas, O. (2021). When to assess: Cognitive impact of ventriculoperitoneal shunt operation in elderly adults with normal pressure hydrocephalus. World Neurosurg. 154, e302–e312. doi:10.1016/j.wneu.2021.07.015
Cacabelos, R. (2020). Pharmacogenomics of Alzheimer's and Parkinson's diseases. Neurosci. Lett. 726, 133807. doi:10.1016/j.neulet.2018.09.018
Chatzidakis, E. M., Barlas, G., Condilis, N., Bouramas, D., Anagnostopoulos, D., Volikas, Z., et al. (2008). Brain CT scan indexes in the normal pressure hydrocephalus: Predictive value in the outcome of patients and correlation to the clinical symptoms. Ann. Ital. Chir. 79, 353–362.
Chen, S., Li, H., Zheng, J., Hao, L., Jing, T., Wu, P., et al. (2022). Expression profiles of exosomal MicroRNAs derived from cerebrospinal fluid in patients with congenital hydrocephalus determined by MicroRNA sequencing. Dis. Markers 2022, 5344508. doi:10.1155/2022/5344508
Chunyan, L., Rongrong, H., Youping, W., Hongliang, L., Qiong, Y., Xing, L., et al. (2021). Gait characteristics and effects of the cerebrospinal fluid tap test in probable idiopathic normal pressure hydrocephalus. Clin. Neurol. Neurosurg. 210, 106952. doi:10.1016/j.clineuro.2021.106952
Coppola, G., Chinnathambi, S., Lee, J. J., Dombroski, B. A., Baker, M. C., Soto-Ortolaza, A. I., et al. (2012). Evidence for a role of the rare p.A152T variant in MAPT in increasing the risk for FTD-spectrum and Alzheimer's diseases. Hum. Mol. Genet. 21, 3500–3512. doi:10.1093/hmg/dds161
Cserr, H. F., Cooper, D. N., and Milhorat, T. H. (1977). Flow of cerebral interstitial fluid as indicated by the removal of extracellular markers from rat caudate nucleus. Exp. Eye Res. 25 (Suppl. l), 461–473. doi:10.1016/s0014-4835(77)80041-9
Cserr, H. F., and Ostrach, L. H. (1974). Bulk flow of interstitial fluid after intracranial injection of blue dextran 2000. Exp. Neurol. 45, 50–60. doi:10.1016/0014-4886(74)90099-5
Da Silva, M. C., Michowicz, S., Drake, J. M., Chumas, P. D., and Tuor, U. I. (1995). Reduced local cerebral blood flow in periventricular white matter in experimental neonatal hydrocephalus-restoration with CSF shunting. J. Cereb. Blood Flow. Metab. 15, 1057–1065. doi:10.1038/jcbfm.1995.132
Daly, S., Hanson, J. T., Mavanji, V., Gravely, A., Jean, J., Jonason, A., et al. (2022). Using kinematics to re-define the pull test as a quantitative biomarker of the postural response in normal pressure hydrocephalus patients. Exp. Brain Res. 240, 791–802. doi:10.1007/s00221-021-06292-5
Davis, A., Luciano, M., Moghekar, A., and Yasar, S. (2021). Assessing the predictive value of common gait measure for predicting falls in patients presenting with suspected normal pressure hydrocephalus. BMC Neurol. 21, 60. doi:10.1186/s12883-021-02068-0
De Meyer, G., Shapiro, F., Vanderstichele, H., Vanmechelen, E., Engelborghs, S., De Deyn, P. P., et al. (2010). Diagnosis-independent Alzheimer disease biomarker signature in cognitively normal elderly people. Arch. Neurol. 67, 949–956. doi:10.1001/archneurol.2010.179
De Souza, G. S., Andrade, M. A., Borelli, W. V., Schilling, L. P., Matushita, C. S., Portuguez, M. W., et al. (2022). Amyloid-beta PET classification on cognitive aging stages using the centiloid scale. Mol. Imaging Biol. 24, 394–403. doi:10.1007/s11307-021-01660-7
Del Bigio, M. R., and Bruni, J. E. (1988). Changes in periventricular vasculature of rabbit brain following induction of hydrocephalus and after shunting. J. Neurosurg. 69, 115–120. doi:10.3171/jns.1988.69.1.0115
Deshpande, A., Dombrowski, S. M., Leichliter, A., Krajcir, N., Zingales, N., Inoue, M., et al. (2009). Dissociation between vascular endothelial growth factor receptor-2 and blood vessel density in the caudate nucleus after chronic hydrocephalus. J. Cereb. Blood Flow. Metab. 29, 1806–1815. doi:10.1038/jcbfm.2009.98
Devito, E. E., Salmond, C. H., Owler, B. K., Sahakian, B. J., and Pickard, J. D. (2007). Caudate structural abnormalities in idiopathic normal pressure hydrocephalus. Acta Neurol. Scand. 116, 328–332. doi:10.1111/j.1600-0404.2007.00906.x
Dewan, M. C., Rattani, A., Mekary, R., Glancz, L. J., Yunusa, I., Baticulon, R. E., et al. (2018). Global hydrocephalus epidemiology and incidence: Systematic review and meta-analysis. J. Neurosurg. 130, 1065–1079. doi:10.3171/2017.10.JNS17439
Di Fede, G., Catania, M., Morbin, M., Rossi, G., Suardi, S., Mazzoleni, G., et al. (2009). A recessive mutation in the APP gene with dominant-negative effect on amyloidogenesis. Science 323, 1473–1477. doi:10.1126/science.1168979
Dombrowski, S. M., Schenk, S., Leichliter, A., Leibson, Z., Fukamachi, K., and Luciano, M. G. (2006). Chronic hydrocephalus-induced changes in cerebral blood flow: Mediation through cardiac effects. J. Cereb. Blood Flow. Metab. 26, 1298–1310. doi:10.1038/sj.jcbfm.9600282
Echeverria, V., Barreto, G. E., Avila-Rodriguezc, M., Tarasov, V. V., and Aliev, G. (2017). Is VEGF a key target of cotinine and other potential therapies against alzheimer disease? Curr. Alzheimer Res. 14, 1155–1163. doi:10.2174/1567205014666170329113007
Ekici, A. B., Hilfinger, D., Jatzwauk, M., Thiel, C. T., Wenzel, D., Lorenz, I., et al. (2010). Disturbed wnt signalling due to a mutation in CCDC88C causes an autosomal recessive non-syndromic hydrocephalus with medial diverticulum. Mol. Syndromol. 1, 99–112. doi:10.1159/000319859
Esaulova, E., Cantoni, C., Shchukina, I., Zaitsev, K., Bucelli, R. C., Wu, G. F., et al. (2020). Single-cell RNA-seq analysis of human CSF microglia and myeloid cells in neuroinflammation. Neurol. Neuroimmunol. Neuroinflamm. 7, e732. doi:10.1212/NXI.0000000000000732
Evans, F. L., Dittmer, M., De La Fuente, A. G., and Fitzgerald, D. C. (2019). Protective and regenerative roles of T cells in central nervous system disorders. Front. Immunol. 10, 2171. doi:10.3389/fimmu.2019.02171
Fiala, M., Mahanian, M., Rosenthal, M., Mizwicki, M. T., Tse, E., Cho, T., et al. (2011). MGAT3 mRNA: A biomarker for prognosis and therapy of alzheimer's disease by vitamin D and curcuminoids. J. Alzheimers Dis. 25, 135–144. doi:10.3233/JAD-2011-101950
Gable, D. L., Gaysinskaya, V., Atik, C. C., Talbot, C. C., Kang, B., Stanley, S. E., et al. (2019). ZCCHC8, the nuclear exosome targeting component, is mutated in familial pulmonary fibrosis and is required for telomerase RNA maturation. Genes. Dev. 33, 1381–1396. doi:10.1101/gad.326785.119
Gavrilov, G. V., Gaydar, B. V., Svistov, D. V., Korovin, A. E., Samarcev, I. N., Churilov, L. P., et al. (2019). Idiopathic normal pressure hydrocephalus (Hakim-Adams syndrome): Clinical symptoms, diagnosis and treatment. Psychiatr. Danub. 31, 737–744.
GBD 2019 Dementia Forecasting Collaborators (2022). Estimation of the global prevalence of dementia in 2019 and forecasted prevalence in 2050: An analysis for the global burden of disease study 2019. Lancet. Public Health 7, e105–e125. doi:10.1016/S2468-2667(21)00249-8
Georganopoulou, D. G., Chang, L., Nam, J. M., Thaxton, C. S., Mufson, E. J., Klein, W. L., et al. (2005). Nanoparticle-based detection in cerebral spinal fluid of a soluble pathogenic biomarker for Alzheimer's disease. Proc. Natl. Acad. Sci. U. S. A. 102, 2273–2276. doi:10.1073/pnas.0409336102
Gholampour, S., Yamini, B., Droessler, J., and Frim, D. (2022). A new definition for intracranial compliance to evaluate adult hydrocephalus after shunting. Front. Bioeng. Biotechnol. 10, 900644. doi:10.3389/fbioe.2022.900644
Giaccone, G., Morbin, M., Moda, F., Botta, M., Mazzoleni, G., Uggetti, A., et al. (2010). Neuropathology of the recessive A673V APP mutation: Alzheimer disease with distinctive features. Acta Neuropathol. 120, 803–812. doi:10.1007/s00401-010-0747-1
Gomes, S. A., Targett, M., and Lowrie, M. (2020a). Congenital external hydrocephalus in a dog. J. Small Anim. Pract. 61, 710–713. doi:10.1111/jsap.13016
Gomes, S. A., Targett, M., and Lowrie, M. (2020b). Response to: An objection to "Congenital external hydrocephalus in a dog. J. Small Anim. Pract. 61, 715. doi:10.1111/jsap.13229
Graff-Radford, N. R. (2014). Alzheimer CSF biomarkers may be misleading in normal-pressure hydrocephalus. Neurology 83, 1573–1575. doi:10.1212/WNL.0000000000000916
Grubman, A., Chew, G., Ouyang, J. F., Sun, G., Choo, X. Y., Mclean, C., et al. (2019). A single-cell atlas of entorhinal cortex from individuals with Alzheimer's disease reveals cell-type-specific gene expression regulation. Nat. Neurosci. 22, 2087–2097. doi:10.1038/s41593-019-0539-4
Guan, Q., Liu, W., Mu, K., Hu, Q., Xie, J., Cheng, L., et al. (2022). Single-cell RNA sequencing of CSF reveals neuroprotective RAC1(+) NK cells in Parkinson's disease. Front. Immunol. 13, 992505. doi:10.3389/fimmu.2022.992505
Gui, Y., Liu, H., Zhang, L., Lv, W., and Hu, X. (2015). Altered microRNA profiles in cerebrospinal fluid exosome in Parkinson disease and Alzheimer disease. Oncotarget 6, 37043–37053. doi:10.18632/oncotarget.6158
Han, S. W., Park, Y. H., Ryoo, N., Kim, K., Pyun, J. M., and Kim, S. (2021). Pearls & oy-sters: Idiopathic normal pressure hydrocephalus with synucleinopathy: Diagnosis and treatment. Neurology 97, 196–199. doi:10.1212/WNL.0000000000012099
Hasegawa, D. (2020). An objection to: "Congenital external hydrocephalus in a dog. J. Small Anim. Pract. 61, 714. doi:10.1111/jsap.13227
He, D., Tan, S. Q., Lan, L. F., and Fan, Y. H. (2021). [Cognitive and gait dysfunction in the elderly caused by age-related cerebral small vessel disease and idiopathic normal pressure hydrocephalus: A case series of three patients]. Zhonghua Yi Xue Za Zhi 101, 1093–1096. doi:10.3760/cma.j.cn112137-20200726-02213
He, M., Qi, Z., Shao, Y., Yao, H., Zhang, X., Zhang, Y., et al. (2021). Quantitative evaluation of gait changes using APDM inertial sensors after the external lumbar drain in patients with idiopathic normal pressure hydrocephalus. Front. Neurol. 12, 635044. doi:10.3389/fneur.2021.635044
Hill, A., and Volpe, J. J. (1981). Normal pressure hydrocephalus in the newborn. Pediatrics 68, 623–629. doi:10.1542/peds.68.5.623
Hnin, H. H., Bovonsunthonchai, S., Witthiwej, T., Vachalathiti, R., and Ariyaudomkit, R. (2021). Feasibility of action observation effect on gait and mobility in idiopathic normal pressure hydrocephalus patients. Dement. Neuropsychol. 15, 79–87. doi:10.1590/1980-57642021dn15-010008
Hochstetler, A. E., Smith, H. M., Preston, D. C., Reed, M. M., Territo, P. R., Shim, J. W., et al. (2020). TRPV4 antagonists ameliorate ventriculomegaly in a rat model of hydrocephalus. JCI Insight 5, 137646. doi:10.1172/jci.insight.137646
Holtta, M., Hansson, O., Andreasson, U., Hertze, J., Minthon, L., Nagga, K., et al. (2013). Evaluating amyloid-beta oligomers in cerebrospinal fluid as a biomarker for Alzheimer's disease. PLoS One 8, e66381. doi:10.1371/journal.pone.0066381
Huang, H., Yang, J., Luciano, M., and Shriver, L. P. (2016). Longitudinal metabolite profiling of cerebrospinal fluid in normal pressure hydrocephalus links brain metabolism with exercise-induced VEGF production and clinical outcome. Neurochem. Res. 41, 1713–1722. doi:10.1007/s11064-016-1887-z
Iijima, K., Hirato, M., Miyagishima, T., Horiguchi, K., Sugawara, K., Hirato, J., et al. (2015). Microrecording and image-guided stereotactic biopsy of deep-seated brain tumors. J. Neurosurg. 123, 978–988. doi:10.3171/2014.10.JNS14963
Iqbal, K., Liu, F., Gong, C. X., and Grundke-Iqbal, I. (2010). Tau in Alzheimer disease and related tauopathies. Curr. Alzheimer Res. 7, 656–664. doi:10.2174/156720510793611592
Isaacs, A. M., Riva-Cambrin, J., Yavin, D., Hockley, A., Pringsheim, T. M., Jette, N., et al. (2018). Age-specific global epidemiology of hydrocephalus: Systematic review, metanalysis and global birth surveillance. PLoS One 13, e0204926. doi:10.1371/journal.pone.0204926
Isik, M., and Beydemir, S. (2022). AChE mRNA expression as a possible novel biomarker for the diagnosis of coronary artery disease and Alzheimer's disease, and its association with oxidative stress. Arch. Physiol. Biochem. 128, 352–359. doi:10.1080/13813455.2019.1683584
Ito, D., Ishikawa, C., Jeffery, N. D., and Kitagawa, M. (2021). Cerebrospinal fluid flow on time-spatial labeling inversion pulse images before and after treatment of congenital hydrocephalus in a dog. J. Vet. Intern. Med. 35, 490–496. doi:10.1111/jvim.16020
Jang, S. H., Chang, C. H., Jung, Y. J., and Lee, H. D. (2017). Recovery of akinetic mutism and injured prefronto-caudate tract following shunt operation for hydrocephalus and rehabilitation: A case report. Med. Baltim. 96, e9117. doi:10.1097/MD.0000000000009117
Jeong, S., Yu, H., Park, J., and Kang, K. (2021). Quantitative gait analysis of idiopathic normal pressure hydrocephalus using deep learning algorithms on monocular videos. Sci. Rep. 11, 12368. doi:10.1038/s41598-021-90524-9
Jeppsson, A., Bjerke, M., Hellstrom, P., Blennow, K., Zetterberg, H., Kettunen, P., et al. (2022). Shared CSF biomarker profile in idiopathic normal pressure hydrocephalus and subcortical small vessel disease. Front. Neurol. 13, 839307. doi:10.3389/fneur.2022.839307
Jingami, N., Asada-Utsugi, M., Uemura, K., Noto, R., Takahashi, M., Ozaki, A., et al. (2015). Idiopathic normal pressure hydrocephalus has a different cerebrospinal fluid biomarker profile from Alzheimer's disease. J. Alzheimers Dis. 45, 109–115. doi:10.3233/JAD-142622
Joseph, S., Robbins, K. R., and Rekaya, R. (2006). A statistical and biological approach for identifying misdiagnosis of incipient Alzheimer patients using gene expression data. Conf. Proc. IEEE Eng. Med. Biol. Soc. 2006, 5854–5857. doi:10.1109/IEMBS.2006.259371
Joseph, S., Robbins, K., Zhang, W., and Rekaya, R. (2008). Effects of misdiagnosis in input data on the identification of differential expression genes in incipient Alzheimer patients. Silico Biol. 8, 545–554.
Karim, T. J., Aksel, C., Kharas, N., Reyes-Vasquez, C., and Dafny, N. (2018). Caudate nucleus neurons participate in methylphenidate function: Behavioral and neuronal recordings from freely behaving adolescent rats. Brain Res. Bull. 142, 241–252. doi:10.1016/j.brainresbull.2018.07.008
Kazui, H., Kanemoto, H., Yoshiyama, K., Kishima, H., Suzuki, Y., Sato, S., et al. (2016). Association between high biomarker probability of Alzheimer's disease and improvement of clinical outcomes after shunt surgery in patients with idiopathic normal pressure hydrocephalus. J. Neurol. Sci. 369, 236–241. doi:10.1016/j.jns.2016.08.040
Kester, M. I., Van Der Flier, W. M., Visser, A., Blankenstein, M. A., Scheltens, P., and Oudejans, C. B. (2011). Decreased mRNA expression of CCL5 [RANTES] in Alzheimer's disease blood samples. Clin. Chem. Lab. Med. 50, 61–65. doi:10.1515/CCLM.2011.731
Kiefer, M., and Unterberg, A. (2012). The differential diagnosis and treatment of normal-pressure hydrocephalus. Dtsch. Arztebl. Int. 109, 15–25. quiz 26. doi:10.3238/arztebl.2012.0015
Koo, A. B., Elsamadicy, A. A., Lin, I. H., David, W. B., Reeves, B. C., Santarosa, C., et al. (2021). Patient risk factors associated with 30- and 90-day readmission after ventriculoperitoneal shunt placement for idiopathic normal pressure hydrocephalus in elderly patients: A nationwide readmission study. World Neurosurg. 152, e23–e31. doi:10.1016/j.wneu.2021.04.010
Kuruvithadam, K., Menner, M., Taylor, W. R., Zeilinger, M. N., Stieglitz, L., and Schmid Daners, M. (2021). Data-driven investigation of gait patterns in individuals affected by normal pressure hydrocephalus. Sensors (Basel) 21, 6451. doi:10.3390/s21196451
Lafirdeen, A. S. M., Cognat, E., Sabia, S., Hourregue, C., Lilamand, M., Dugravot, A., et al. (2019). Biomarker profiles of Alzheimer's disease and dynamic of the association between cerebrospinal fluid levels of beta-amyloid peptide and tau. PLoS One 14, e0217026. doi:10.1371/journal.pone.0217026
Lee, C. H., Jung, Y. S., and Lee, S. H. (2010). Hydrocephalus as a presenting manifestation of neurosarcoidosis : Easy to misdiagnose as tuberculosis. J. Korean Neurosurg. Soc. 48, 79–81. doi:10.3340/jkns.2010.48.1.79
Lee, D. N., Ha, M., Kwak, I., Amhed, S., Kang, K., Woo, S. H., et al. (2021). Severe hydrocephalus in a raccoon dog (Nyctereutes procyonoides). J. Vet. Med. Sci. 83, 1086–1089. doi:10.1292/jvms.20-0607
Lu, R., Wang, J., Tao, R., Wang, J., Zhu, T., Guo, W., et al. (2018). Reduced TRPC6 mRNA levels in the blood cells of patients with Alzheimer's disease and mild cognitive impairment. Mol. Psychiatry 23, 767–776. doi:10.1038/mp.2017.136
Lucas, H. B., Mcknight, I., Raines, R., Hijazi, A., Hart, C., Lee, C., et al. (2021). Factors associated with mutations: Their matching rates to cardiovascular and neurological diseases. Int. J. Mol. Sci. 22, 5057. doi:10.3390/ijms22105057
Maier, I. L., Heide, M., Hofer, S., Dechent, P., Fiss, I., Von Der Brelie, C., et al. (2022). High periventricular T1 relaxation times predict gait improvement after spinal tap in patients with idiopathic normal pressure hydrocephalus. Clin. Neuroradiol. [Epub ahead of print]. doi:10.1007/s00062-022-01155-0
Matthews, K. A., Xu, W., Gaglioti, A. H., Holt, J. B., Croft, J. B., Mack, D., et al. (2019). Racial and ethnic estimates of Alzheimer's disease and related dementias in the United States (2015-2060) in adults aged ≥65 years. Alzheimers Dement. 15, 17–24. doi:10.1016/j.jalz.2018.06.3063
Mazzeo, S., Emiliani, F., Bagnoli, S., Padiglioni, S., Del Re, L. M., Giacomucci, G., et al. (2022). Influence of ApoE genotype and clock T3111C interaction with cardiovascular risk factors on the progression to alzheimer's disease in subjective cognitive decline and mild cognitive impairment patients. J. Pers. Med. 12, E45. doi:10.3390/jpm10020045
Mcknight, I., Hart, C., Park, I. H., and Shim, J. W. (2021). Genes causing congenital hydrocephalus: Their chromosomal characteristics of telomere proximity and DNA compositions. Exp. Neurol. 335, 113523. doi:10.1016/j.expneurol.2020.113523
Mclimans, K. E., Clark, B. E., Plagman, A., Pappas, C., Klinedinst, B., Anatharam, V., et al. (2019). Is cerebrospinal fluid superoxide dismutase 1 a biomarker of tau but not amyloid-induced neurodegeneration in alzheimer's disease? Antioxid. Redox Signal. 31, 572–578. doi:10.1089/ars.2019.7762
Mechelli, A., Quattrone, A., Nistico, R., Crasa, M., La Torre, D., Vescio, B., et al. (2022). Blink reflex recovery cycle distinguishes patients with idiopathic normal pressure hydrocephalus from elderly subjects. J. Neurol. 269, 1007–1012. doi:10.1007/s00415-021-10687-3
Mesitskaya, D. F., Syrkin, A. L., Aksenova, M. G., Zhang, Y., Zamyatnin, A. A., and Kopylov, P. Y. (2018). Thromboxane A synthase: A new target for the treatment of cardiovascular diseases. Cardiovasc. Hematol. Agents Med. Chem. 16, 81–87. doi:10.2174/1871525716666180724115132
Miyajima, M., Nakajima, M., Ogino, I., Miyata, H., Motoi, Y., and Arai, H. (2013). Soluble amyloid precursor protein alpha in the cerebrospinal fluid as a diagnostic and prognostic biomarker for idiopathic normal pressure hydrocephalus. Eur. J. Neurol. 20, 236–242. doi:10.1111/j.1468-1331.2012.03781.x
Morales, D. M., Smyser, C. D., Han, R. H., Kenley, J. K., Shimony, J. S., Smyser, T. A., et al. (2021). Tract-specific relationships between cerebrospinal fluid biomarkers and periventricular white matter in posthemorrhagic hydrocephalus of prematurity. Neurosurgery 88, 698–706. doi:10.1093/neuros/nyaa466
Morel, E., Armand, S., Assal, F., and Allali, G. (2021). Normal pressure hydrocephalus and CSF tap test response: The gait phenotype matters. J. Neural Transm. 128, 121–125. doi:10.1007/s00702-020-02270-3
Moriuchi, S., Yamada, K., Dehara, M., Teramoto, Y., Soda, T., Imakita, M., et al. (2011). Use of 5-aminolevulinic acid for the confirmation of deep-seated brain tumors during stereotactic biopsy. Report of 2 cases. J. Neurosurg. 115, 278–280. doi:10.3171/2011.4.JNS102137
Morton, N. E. (1991). Parameters of the human genome. Proc. Natl. Acad. Sci. U. S. A. 88, 7474–7476. doi:10.1073/pnas.88.17.7474
Mulder, C., Verwey, N. A., Van Der Flier, W. M., Bouwman, F. H., Kok, A., Van Elk, E. J., et al. (2010). Amyloid-beta(1-42), total tau, and phosphorylated tau as cerebrospinal fluid biomarkers for the diagnosis of Alzheimer disease. Clin. Chem. 56, 248–253. doi:10.1373/clinchem.2009.130518
Mullan, M., Crawford, F., Axelman, K., Houlden, H., Lilius, L., Winblad, B., et al. (1992). A pathogenic mutation for probable Alzheimer's disease in the APP gene at the N-terminus of beta-amyloid. Nat. Genet. 1, 345–347. doi:10.1038/ng0892-345
Musiek, E. S., and Holtzman, D. M. (2012). Origins of alzheimer's disease: Reconciling cerebrospinal fluid biomarker and neuropathology data regarding the temporal sequence of amyloid-beta and tau involvement. Curr. Opin. Neurol. 25, 715–720. doi:10.1097/WCO.0b013e32835a30f4
Nagata, Y., Bundo, M., Sugiura, S., Kamita, M., Ono, M., Hattori, K., et al. (2017). PTPRQ as a potential biomarker for idiopathic normal pressure hydrocephalus. Mol. Med. Rep. 16, 3034–3040. doi:10.3892/mmr.2017.7015
Nakajima, M., Arai, H., and Miyajima, M. (2010). [Diagnostic value of CSF biomarker profile in idiopathic normal pressure hydrocephalus; leucine-rich alpha-2-glycoprotein is a potential biological marker]. Rinsho Shinkeigaku 50, 973–976. doi:10.5692/clinicalneurol.50.973
Nakajima, M., Rauramaa, T., Makinen, P. M., Hiltunen, M., Herukka, S. K., Kokki, M., et al. (2021). Protein tyrosine phosphatase receptor type Q in cerebrospinal fluid reflects ependymal cell dysfunction and is a potential biomarker for adult chronic hydrocephalus. Eur. J. Neurol. 28, 389–400. doi:10.1111/ene.14575
Niermeyer, M., Gaudet, C., Malloy, P., Piryatinsky, I., Salloway, S., Klinge, P., et al. (2020). Frontal behavior syndromes in idiopathic normal pressure hydrocephalus as a function of alzheimer's disease biomarker status. J. Int. Neuropsychol. Soc. 26, 883–893. doi:10.1017/S1355617720000387
Nikaido, Y., Okada, Y., Urakami, H., Ishida, N., Akisue, T., Kawami, Y., et al. (2022). Dynamic stability during gait in idiopathic normal pressure hydrocephalus and Parkinson's disease. Acta Neurol. Scand. 145, 215–222. doi:10.1111/ane.13537
Nikaido, Y., Urakami, H., Akisue, T., Okada, Y., Kawami, Y., Ishida, N., et al. (2021). Perceived and actual changes in gait balance after CSF shunting in idiopathic normal pressure hydrocephalus. Acta Neurol. Scand. 144, 21–28. doi:10.1111/ane.13421
Nusbaum, C., Mikkelsen, T. S., Zody, M. C., Asakawa, S., Taudien, S., Garber, M., et al. (2006). DNA sequence and analysis of human chromosome 8. Nature 439, 331–335. doi:10.1038/nature04406
Oike, R., Inoue, Y., Matsuzawa, K., and Sorimachi, T. (2021). Screening for idiopathic normal pressure hydrocephalus in the elderly after falls. Clin. Neurol. Neurosurg. 205, 106635. doi:10.1016/j.clineuro.2021.106635
Olszewska, A., Farke, D., and Schmidt, M. J. (2020). Spontaneous hemispheric ventricular collapse and subarachnoid haemorrhages in a dog with congenital hydrocephalus internus. Ir. Vet. J. 73, 5. doi:10.1186/s13620-020-00159-x
Ooba, H., Abe, T., Momii, Y., and Fujiki, M. (2013). Stereotactic biopsy with electrical monitoring for deep-seated brain tumors. World Neurosurg. 79, 207.e1–e5. doi:10.1016/j.wneu.2010.05.028
Palmer, J. A., Kaufman, C. S., Vidoni, E. D., Honea, R. A., Burns, J. M., and Billinger, S. A. (2022). Cerebrovascular response to exercise interacts with individual genotype and amyloid-beta deposition to influence response inhibition with aging. Neurobiol. Aging 114, 15–26. doi:10.1016/j.neurobiolaging.2022.02.014
Park, E. H., Dombrowski, S., Luciano, M., Zurakowski, D., and Madsen, J. R. (2010). Alterations of pulsation absorber characteristics in experimental hydrocephalus. J. Neurosurg. Pediatr. 6, 159–170. doi:10.3171/2010.5.PEDS09142
Peterson, K. A., Mole, T. B., Keong, N. C. H., Devito, E. E., Savulich, G., Pickard, J. D., et al. (2019). Structural correlates of cognitive impairment in normal pressure hydrocephalus. Acta Neurol. Scand. 139, 305–312. doi:10.1111/ane.13052
Piper, C., Hainstock, E., Yin-Yuan, C., Chen, Y., Khatun, A., Kasmani, M. Y., et al. (2022). Single-cell immune profiling reveals a developmentally distinct CD4+ GM-CSF+ T-cell lineage that induces GI tract GVHD. Blood Adv. 6, 2791–2804. doi:10.1182/bloodadvances.2021006084
Pozzi, N. G., Brumberg, J., Todisco, M., Minafra, B., Zangaglia, R., Bossert, I., et al. (2021). Striatal dopamine deficit and motor impairment in idiopathic normal pressure hydrocephalus. Mov. Disord. 36, 124–132. doi:10.1002/mds.28366
Pyykko, O. T., Lumela, M., Rummukainen, J., Nerg, O., Seppala, T. T., Herukka, S. K., et al. (2014). Cerebrospinal fluid biomarker and brain biopsy findings in idiopathic normal pressure hydrocephalus. PLoS One 9, e91974. doi:10.1371/journal.pone.0091974
Qin, F., Huang, Z., Dong, Q., Xu, X., Lu, T., Chen, J., et al. (2021). Stereotactic biopsy for lesions in brainstem and deep brain: A single-center experience of 72 cases. Braz J. Med. Biol. Res. 54, e11335. doi:10.1590/1414-431X2021e11335
Raines, R., Mcknight, I., White, H., Legg, K., Lee, C., Li, W., et al. (2022). Drug-targeted genomes: Mutability of ion channels and GPCRs. Biomedicines 10, 594. doi:10.3390/biomedicines10030594
Ramazi, S., Heydari-Zarnagh, H., Goudarzian, M., Khalaj-Kondori, M., and Bonyadi, M. (2019). Thromboxane A synthase 1 gene expression and promotor haplotypes are associated with risk of large artery-atherosclerosis stroke in Iranian population. J. Cell. Biochem. 120, 15222–15232. doi:10.1002/jcb.28787
Reeves, B. C., Karimy, J. K., Kundishora, A. J., Mestre, H., Cerci, H. M., Matouk, C., et al. (2020). Glymphatic system impairment in alzheimer's disease and idiopathic normal pressure hydrocephalus. Trends Mol. Med. 26, 285–295. doi:10.1016/j.molmed.2019.11.008
Ringstad, G., Vatnehol, S. a. S., and Eide, P. K. (2017). Glymphatic MRI in idiopathic normal pressure hydrocephalus. Brain 140, 2691–2705. doi:10.1093/brain/awx191
Ritz, R., Feigl, G. C., Schuhmann, M. U., Ehrhardt, A., Danz, S., Noell, S., et al. (2011). Use of 5-ALA fluorescence guided endoscopic biopsy of a deep-seated primary malignant brain tumor. J. Neurosurg. 114, 1410–1413. doi:10.3171/2010.11.JNS10250
Sakellaridis, N., and Stavropoulos, S. (2010). Normal-pressure hydrocephalus and cerebral blood flow. J. Neurosurg. 112, 1351. author reply 1351. doi:10.3171/2009.10.JNS091489
Schneider, C. A., Rasband, W. S., and Eliceiri, K. W. (2012). NIH image to ImageJ: 25 years of image analysis. Nat. Methods 9, 671–675. doi:10.1038/nmeth.2089
Sedighi, B., Shafiee, K., Seifaldini, R., and Abdi, A. (2014). Changing cerebral blood flow in normal pressure hydrocephalus after the tap test can predict clinical improvement. Iran. J. Neurol. 13, 245–249.
Shen, X. N., Huang, Y. Y., Chen, S. D., Guo, Y., Tan, L., Dong, Q., et al. (2021). Plasma phosphorylated-tau181 as a predictive biomarker for Alzheimer's amyloid, tau and FDG PET status. Transl. Psychiatry 11, 585. doi:10.1038/s41398-021-01709-9
Shen, X. N., Li, J. Q., Wang, H. F., Li, H. Q., Huang, Y. Y., Yang, Y. X., et al. (2020). Plasma amyloid, tau, and neurodegeneration biomarker profiles predict Alzheimer's disease pathology and clinical progression in older adults without dementia. Alzheimers Dement. 12, e12104. doi:10.1002/dad2.12104
Shim, J. W., and Madsen, J. R. (2018). VEGF signaling in neurological disorders. Int. J. Mol. Sci. 19, E275. doi:10.3390/ijms19010275
Shim, J. W., Sandlund, J., Hameed, M. Q., Blazer-Yost, B., Zhou, F. C., Klagsbrun, M., et al. (2016). Excess HB-EGF, which promotes VEGF signaling, leads to hydrocephalus. Sci. Rep. 6, 26794. doi:10.1038/srep26794
Shim, J. W., Sandlund, J., Han, C. H., Hameed, M. Q., Connors, S., Klagsbrun, M., et al. (2013). VEGF, which is elevated in the CSF of patients with hydrocephalus, causes ventriculomegaly and ependymal changes in rats. Exp. Neurol. 247, 703–709. doi:10.1016/j.expneurol.2013.03.011
Shim, J. W., Territo, P. R., Simpson, S., Watson, J. C., Jiang, L., Riley, A. A., et al. (2019). Hydrocephalus in a rat model of Meckel Gruber syndrome with a TMEM67 mutation. Sci. Rep. 9, 1069. doi:10.1038/s41598-018-37620-5
Shimada, H., Ataka, S., Takeuchi, J., Mori, H., Wada, Y., Watanabe, Y., et al. (2011). Pittsburgh compound B-negative dementia: A possibility of misdiagnosis of patients with non-alzheimer disease-type dementia as having AD. J. Geriatr. Psychiatry Neurol. 24, 123–126. doi:10.1177/0891988711409410
Sobue, G., Ishigaki, S., and Watanabe, H. (2018). Pathogenesis of frontotemporal lobar degeneration: Insights from loss of function theory and early involvement of the caudate nucleus. Front. Neurosci. 12, 473. doi:10.3389/fnins.2018.00473
Sun, Y., Liang, S., Yu, Y., Yang, Y., Lu, J., Wu, J., et al. (2022). Plantar pressure-based temporal analysis of gait disturbance in idiopathic normal pressure hydrocephalus: Indications from a pilot longitudinal study. Comput. Methods Programs Biomed. 217, 106691. doi:10.1016/j.cmpb.2022.106691
Suzuki, Y., Iseki, C., Igari, R., Sato, H., Koyama, S., Kawahara, H., et al. (2022). Reduced cerebral blood flow of lingual gyrus associated with both cognitive impairment and gait disturbance in patients with idiopathic normal pressure hydrocephalus. J. Neurol. Sci. 437, 120266. doi:10.1016/j.jns.2022.120266
Tan, C., Wang, X., Wang, Y., Wang, C., Tang, Z., Zhang, Z., et al. (2021). The pathogenesis based on the glymphatic system, diagnosis, and treatment of idiopathic normal pressure hydrocephalus. Clin. Interv. Aging 16, 139–153. doi:10.2147/CIA.S290709
Tang, P., and Wang, R. K. (2021). 1700 nm broadband laser source enables deep brain optical biopsy. Light Sci. Appl. 10, 205. doi:10.1038/s41377-021-00652-0
Tang, Y. M., Yao, Y., Xu, S., Li, X., Hu, F., Wang, H., et al. (2021). White matter microstructural damage associated with gait abnormalities in idiopathic normal pressure hydrocephalus. Front. Aging Neurosci. 13, 660621. doi:10.3389/fnagi.2021.660621
Thavarajasingam, S. G., El-Khatib, M., Rea, M., Russo, S., Lemcke, J., Al-Nusair, L., et al. (2021). Clinical predictors of shunt response in the diagnosis and treatment of idiopathic normal pressure hydrocephalus: A systematic review and meta-analysis. Acta Neurochir. 163, 2641–2672. doi:10.1007/s00701-021-04922-z
Torretta, E., Arosio, B., Barbacini, P., Capitanio, D., Rossi, P. D., Moriggi, M., et al. (2021). Novel insight in idiopathic normal pressure hydrocephalus (iNPH) biomarker discovery in CSF. Int. J. Mol. Sci. 22, 8034. doi:10.3390/ijms22158034
Trabzuni, D., Wray, S., Vandrovcova, J., Ramasamy, A., Walker, R., Smith, C., et al. (2012). MAPT expression and splicing is differentially regulated by brain region: Relation to genotype and implication for tauopathies. Hum. Mol. Genet. 21, 4094–4103. doi:10.1093/hmg/dds238
Tseng, P. H., Wu, L. K., Wang, Y. C., Ho, T. J., Lin, S. Z., and Tsai, S. T. (2022). Diagnosis and treatment for normal pressure hydrocephalus: From biomarkers identification to outcome improvement with combination therapy. Tzu Chi Med. J. 34, 35–43. doi:10.4103/tcmj.tcmj_275_20
Ugga, L., Pontillo, G., Cuocolo, R., Cocozza, S., and Brunetti, A. (2020). MRI linear measurements in normal pressure hydrocephalus versus progressive supranuclear palsy. Mov. Disord. 35, 2121. doi:10.1002/mds.28330
Vergara-Aragon, P., Dominguez-Marrufo, L. E., Ibarra-Guerrero, P., Hernandez-Ramirez, H., Hernandez-Tellez, B., Lopez-Martinez, I. E., et al. (2011). Tio2-dopamine complex implanted unilaterally in the caudate nucleus improves motor activity and behavior function of rats with induced hemiparkinsonism. Proc. West. Pharmacol. Soc. 54, 15–20.
Virhammar, J., Blohme, H., Nyholm, D., Georgiopoulos, C., and Fallmar, D. (2022). Midbrain area and the hummingbird sign from brain MRI in progressive supranuclear palsy and idiopathic normal pressure hydrocephalus. J. Neuroimaging 32, 90–96. doi:10.1111/jon.12932
Wang, X., Li, L., Luo, P., Li, L., Cui, Q., Wang, J., et al. (2016). Neuronavigation-assisted trajectory planning for deep brain biopsy with susceptibility-weighted imaging. Acta Neurochir. 158, 1355–1362. doi:10.1007/s00701-016-2823-3
Warsi, N. M., and Ibrahim, G. M. (2021). Commentary: Tract-Specific relationships between cerebrospinal fluid biomarkers and periventricular white matter in posthemorrhagic hydrocephalus of prematurity. Neurosurgery 88, E267–E268. doi:10.1093/neuros/nyaa483
Weiss, A., Krause, M., Stockert, A., Richter, C., Puchta, J., Bhogal, P., et al. (2019). Rheologically essential surfactant proteins of the CSF interacting with periventricular white matter changes in hydrocephalus patients - implications for CSF dynamics and the glymphatic system. Mol. Neurobiol. 56, 7863–7871. doi:10.1007/s12035-019-01648-z
Williams, M. A., and Malm, J. (2016). Diagnosis and treatment of idiopathic normal pressure hydrocephalus. Contin. (Minneap Minn) 22, 579–599. doi:10.1212/CON.0000000000000305
Yamada, K., Goto, S., Kochi, M., and Ushio, Y. (2004). Stereotactic biopsy for multifocal, diffuse, and deep-seated brain tumors using Leksell's system. J. Clin. Neurosci. 11, 263–267. doi:10.1016/j.jocn.2003.03.004
Yamada, S., Aoyagi, Y., Ishikawa, M., Yamaguchi, M., Yamamoto, K., and Nozaki, K. (2021). Gait assessment using three-dimensional acceleration of the trunk in idiopathic normal pressure hydrocephalus. Front. Aging Neurosci. 13, 653964. doi:10.3389/fnagi.2021.653964
Yamada, S., Ishikawa, M., and Yamamoto, K. (2016). Comparison of CSF distribution between idiopathic normal pressure hydrocephalus and alzheimer disease. AJNR. Am. J. Neuroradiol. 37, 1249–1255. doi:10.3174/ajnr.A4695
You, G., Zhang, M., Bian, Z., Guo, H., Xu, Z., Ni, Y., et al. (2022). Decoding lymphomyeloid divergence and immune hyporesponsiveness in G-CSF-primed human bone marrow by single-cell RNA-seq. Cell. Discov. 8, 59. doi:10.1038/s41421-022-00417-y
Yu, X., Liu, Z., Tian, Z., Li, S., Huang, H., Zhao, Q., et al. (1998). CT-Guided stereotactic biopsy of deep brain lesions: Report of 310 cases. Chin. Med. J. 111, 361–363.
Zaccaria, V., Bacigalupo, I., Gervasi, G., Canevelli, M., Corbo, M., Vanacore, N., et al. (2020). A systematic review on the epidemiology of normal pressure hydrocephalus. Acta Neurol. Scand. 141, 101–114. doi:10.1111/ane.13182
Zhang, K., Mizuma, H., Zhang, X., Takahashi, K., Jin, C., Song, F., et al. (2021). PET imaging of neural activity, beta-amyloid, and tau in normal brain aging. Eur. J. Nucl. Med. Mol. Imaging 48, 3859–3871. doi:10.1007/s00259-021-05230-5
Zhang, L., Hussain, Z., and Ren, Z. (2019). Recent advances in rational diagnosis and treatment of normal pressure hydrocephalus: A critical appraisal on novel diagnostic, therapy monitoring and treatment modalities. Curr. Drug Targets 20, 1041–1057. doi:10.2174/1389450120666190214121342
Zhu, J., Freitas, H. R., Maezawa, I., Jin, L. W., and Srinivasan, V. J. (2021). 1700 nm optical coherence microscopy enables minimally invasive, label-free, in vivo optical biopsy deep in the mouse brain. Light Sci. Appl. 10, 145. doi:10.1038/s41377-021-00586-7
Ziegelitz, D., Arvidsson, J., Hellstrom, P., Tullberg, M., Wikkelso, C., and Starck, G. (2016). Pre-and postoperative cerebral blood flow changes in patients with idiopathic normal pressure hydrocephalus measured by computed tomography (CT)-perfusion. J. Cereb. Blood Flow. Metab. 36, 1755–1766. doi:10.1177/0271678X15608521
Keywords: MRNA marker, TRPV4, MAPT, normal pressure hydrocephalus, Alzheimer’s disease
Citation: White H, Webb R, McKnight I, Legg K, Lee C, Lee PHU, Spicer OS and Shim JW (2022) TRPV4 mRNA is elevated in the caudate nucleus with NPH but not in Alzheimer’s disease. Front. Genet. 13:936151. doi: 10.3389/fgene.2022.936151
Received: 05 May 2022; Accepted: 17 October 2022;
Published: 02 November 2022.
Edited by:
Ville Leinonen, Kuopio University Hospital, FinlandReviewed by:
David France Tang-Wai, University of Toronto, CanadaSeifollah Gholampour, The University of Chicago, United States
Copyright © 2022 White, Webb, McKnight, Legg, Lee, Lee, Spicer and Shim. This is an open-access article distributed under the terms of the Creative Commons Attribution License (CC BY). The use, distribution or reproduction in other forums is permitted, provided the original author(s) and the copyright owner(s) are credited and that the original publication in this journal is cited, in accordance with accepted academic practice. No use, distribution or reproduction is permitted which does not comply with these terms.
*Correspondence: Joon W. Shim, c2hpbUBtYXJzaGFsbC5lZHU=