- 1Invermay Agricultural Centre, AgResearch Ltd., Mosgiel, New Zealand
- 2Ruakura Research Centre, AgResearch Ltd., Hamilton, New Zealand
- 3Grasslands Research Centre, AgResearch Ltd., Palmerston North, New Zealand
Global agreements in place to reduce methane emissions in livestock are a potential threat to food security. Successful but independent breeding strategies for improved production and lower methane are in place. The unanswered questions are whether these strategies can be combined and how they impact one another, physically and economically. The New Zealand economy is largely dependent on pastoral agriculture from grazing ruminants. The sheep industry produces ∼20 million lamb carcasses for export each year primarily from grass. Methane emitted from the fermentation of forage by grazing ruminants accounts for one-third of all New Zealand’s greenhouse gas emissions. Here, we use sheep selection lines bred for divergent methane production and large numbers of their relatives to determine the genetic and phenotypic correlations between enteric methane emissions, carcass yield, and meat quality. The primary objectives were to determine whether previously shown physiological differences between methane selection lines (differing by ∼12% in methane) result in a negative impact on meat production and quality by measuring close relatives. The results show no negative effects of breeding for lowered methane on meat and carcass quality. Gross methane emissions were highly correlated with liveweight and measures of carcass weight and negatively correlated with dressing-out percentage and fat yield (GR). Trends were similar but not significant for methane yield (g CH4/kg DMI). Preliminary evidence, to date, shows that breeding for low methane may result in animals with higher lean yields that are economically favorable even before carbon costs and environmental benefits are taken into account. These benefits were seen in animals measured for methane on fixed intakes and require validation on intakes that are allowed to vary.
Introduction
Greater than one-quarter of the Earth’s total land mass is used for grazing ruminants (FAO, 2006). The evolutionary adaptation of the ruminant to convert pasture to animal products such as meat, milk, and fibre may have been successful, but ruminant production has an unwanted by-product that is highly detrimental to the environment. During the breakdown and fermentation of plant material in the rumen by symbiotic microbes, hydrogen is produced (Figure 1). This hydrogen is largely utilized by rumen methanogens and eructed by the animal as methane, a potent greenhouse gas in terms of global warming potential. The sustainability of profitable livestock farming throughout the world is increasingly being threatened by methane emissions. Enteric methane from grazing ruminants is the source of 35% of New Zealand’s total greenhouse gas emissions and, globally, livestock contributes approximately 14–18% of the total greenhouse gas emissions (FAO, 2006; Ministry for the Environment, 2021). Methane is not only an environmentally detrimental waste product but also represents ∼2–10% energy loss to the animal (Goopy et al., 2013). Methane emissions per unit of dry matter intake (i.e., methane yield) have been shown to vary between individual sheep, and furthermore, these differences are heritable (Pinares-Patiño et al., 2013; Goopy et al., 2013; Jonker et al., 2018). One strategy to mitigate methane emissions is therefore to select breeding stock for lowered emissions (Clark, 2009). In New Zealand, low and high methane yield selection lines were established through screening research flocks for sheep that were extreme for methane yield. Then, the methane yield selection line flocks were closed to outside animals, and selection was within each line (Pinares-Patiño et al., 2013; Jonker et al., 2018; Rowe et al., 2019). After 10 years of selection, the high and low methane yield lines have an ∼12% difference in methane yield (Rowe et al., 2019).
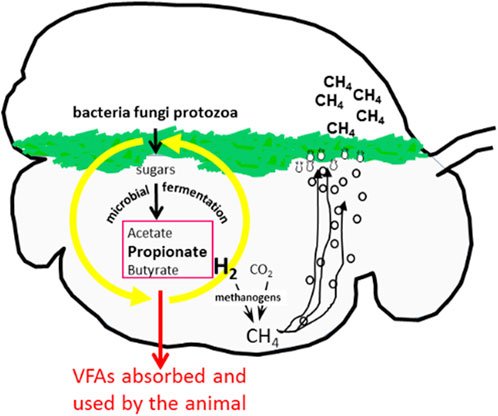
FIGURE 1. Complex polymers such as cellulose are partially digested in the ruminant foregut or rumen. During the fermentation process volatile fatty acids are released and methanogens metabolise hydrogen to form methane. Image courtesy of Graeme Attwood, AgResearch Ltd.
Before undertaking the use of breeding for adaptation strategies in ruminants such as low methane emissions, it will be crucial for us to understand the impact of this on the animal’s digestive physiology and any potential negative outcomes on animal production (Waghorn and Hegarty, 2011).
Evidence for differences in particle retention time, digesta passage rate, and rumen size in sheep bred for low methane (Goopy et al., 2013; Bain et al., 2014; Waite et al., 2019) suggests that there are associated physiological changes in the alimentary tract when breeding for methane. There is also evidence for different gut microbiota leading to different fermentation profiles, ultimately delivering a different source of energy to the animal (Hess et al., 2020; Bilton et al., 2021). These changes might affect the energy metabolism and partitioning in the animal (Bergman, 1990) with potential effects on carcass characteristics.
We hypothesize that the physiological differences associated with low-methane-yield sheep would have the potential to affect the growth and performance of the sheep and the subsequent quality and conformation of the carcass. The objectives of the current study were to determine the relationship between methane emissions and carcass, and meat quality characteristics.
Materials and methods
All animal experiments were conducted to meet the guidelines of the 1999 New Zealand Animal Welfare Act and AgResearch Code of Ethical Conduct and were approved by the AgResearch Grasslands (Palmerston North, NZ) and AgResearch Invermay (Mosgiel, NZ) animal ethics committees.
Animals
Trait data from selected New Zealand sheep flocks, born between 2002 and 2013, were obtained from animals recorded on the Sheep Improvement Limited (SIL) database (Newman et al., 2009). Methane data were obtained from the methane yield selection lines (SIL Flock ID 3633, flock 1). In brief, the development of the methane yield selection lines was based on the screening of progeny from a research flock (SIL Flock ID 2638, flock 2; Jonker et al., 2018) and three New Zealand central progeny test (CPT) flocks (SIL Flock IDs 4640, 4757, and 9153, flocks 3, 4, and 5, respectively; McLean et al., 2006) for methane yield (g CH4/kg DMI) as detailed by Pinares-Patino et al. (2013) and Jonker et al. (2018). The lines were created from the progeny of the top and bottom 10 sires. The lines were closed in 2012, and all sires used from 2012 onward were born in the methane yield selection flock. The lines are currently maintained at 100 ewes per line. In this study, 1,825 animals born in 2007 and 2009–13 provide methane yields for correlation with carcass data collected from over 25,000 animals in the 5 flocks mentioned earlier. All animals were born and managed in a ryegrass-based pastoral grazing system.
Measurements
A summary of the traits and their abbreviations are provided in Table 1. The number of records for each methane, ultrasound, live animal, and carcass trait is provided in Table 2. For the meat and carcass traits, a record refers to a single animal, whereas for methane traits, the number of records reflects multiple measures on each animal. Supplementary Appendix Table SA1 lists the traits and birth flocks, and the birth years from which the data were sourced.
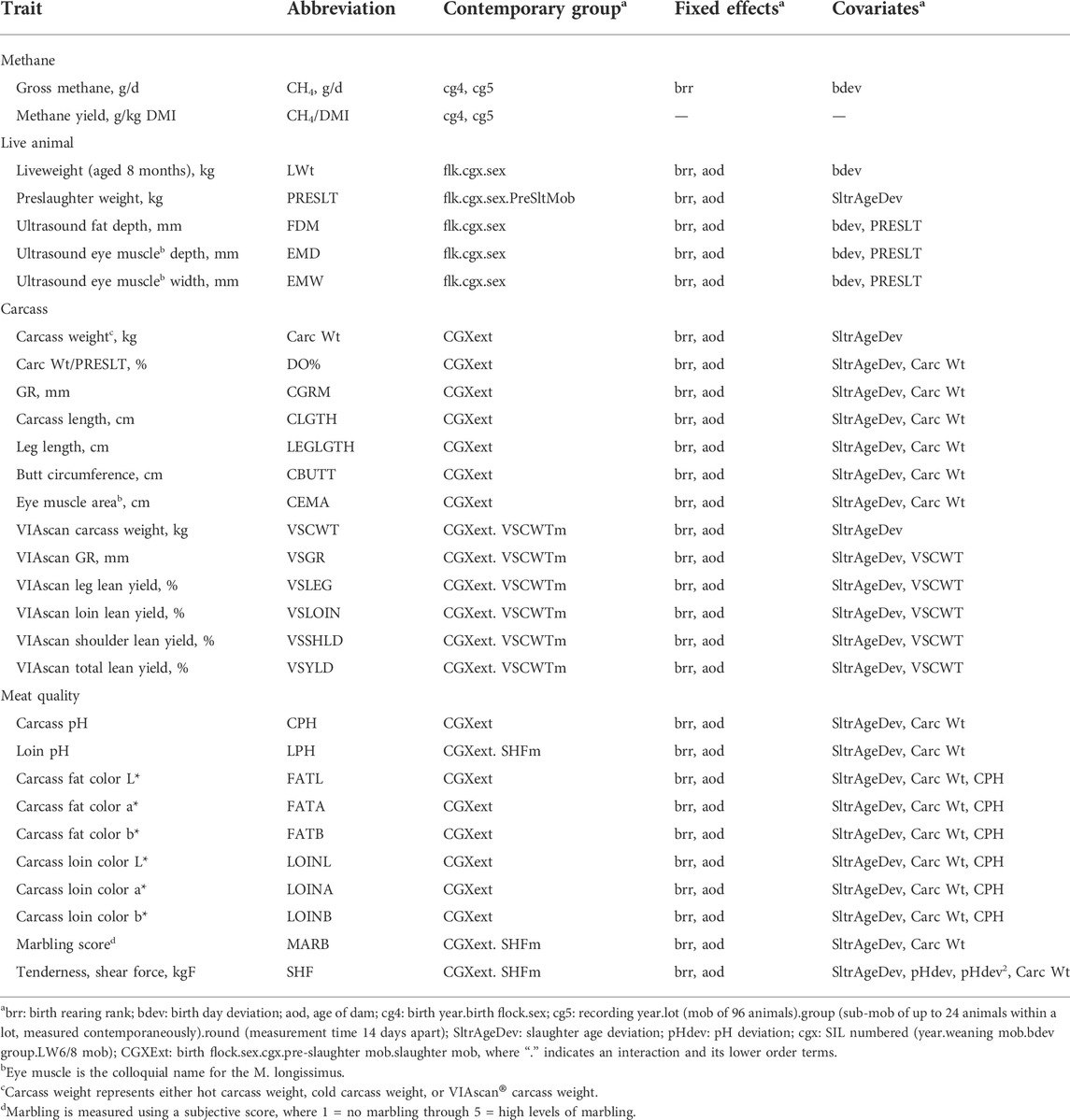
TABLE 1. Methane, live animal, ultrasound, carcass, and meat quality trait description with details of final mixed model effects and covariates for genetic parameter analysis.
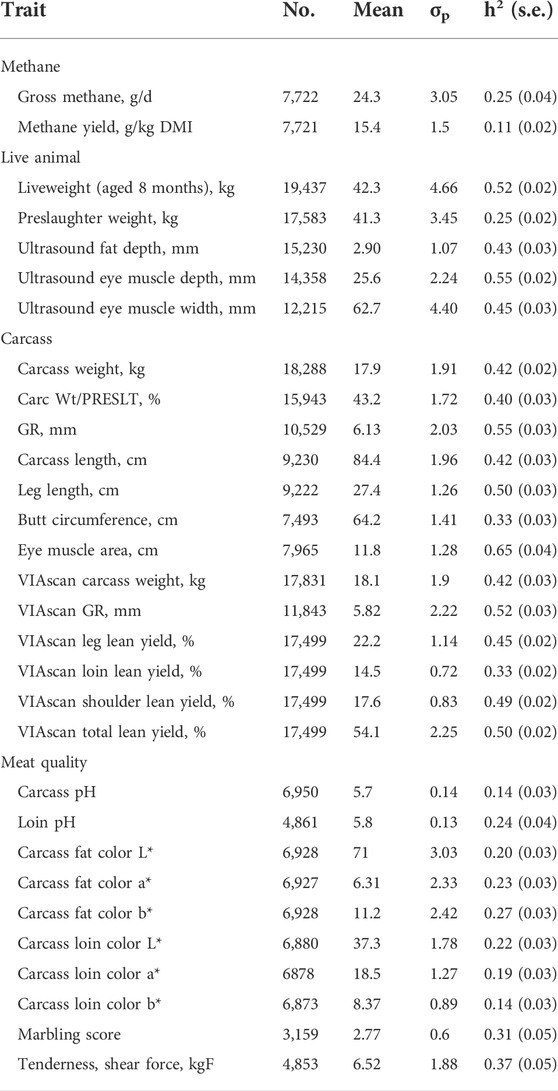
TABLE 2. Number of records/animals, mean, phenotypic standard deviation, and heritability estimate for methane, live animal, ultrasound, carcass, and meat quality traits.
Methane
Traits recorded were gross emissions (CH4, g/d) and methane yield (g CH4/kg DMI). Enteric methane emissions were measured on male and female lambs between 5 and 10 months of age (30–40 kg liveweight) in a facility with 24 respiration chambers as described by Pinares-Patino et al. (2013) and Jonker et al. (2018). The animals were acclimatized in pens for 19–21 days to a lucerne pellet diet. This was followed by two measurement rounds (R1 and R2) of 48 h in the respiration chambers, with the rounds separated by a 10- to 15-day interval (Figure 2). In each round, individual feed dry matter intake (DMI) was measured in metabolic crates (2 days) and then in respiration chambers (2 days). The feeding level was based on the liveweight and was 2.0 times the estimated maintenance metabolizable energy requirements (CSIRO, 1990). Animals were measured in batches of 96. Both in R1 and R2, individuals were randomly allocated to measurement groups (4 groups) and 1 of 24 respiration chambers, and typically, 10 progeny per sire were randomly selected to be measured for gross emissions and methane yield. Each methane record represents 24-h continuous monitoring in the respiration chambers. There were 7,722 methane records collected from 1,825 animals (344 males and 1,481 females). The methane measurement trials with CH4 yield selection line progeny in three birth years have confirmed that the methane phenotype on lucerne pellets is repeatable when the same animals received ryegrass-based pasture (Jonker et al., 2017, 2020).
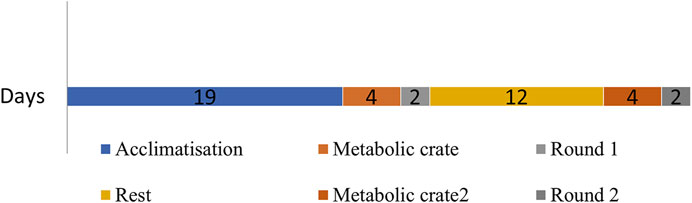
FIGURE 2. A typical timeline for two rounds of methane measurements. Numbers indicate days. Acclimatisation involved housing animals on a Lucerne pellet diet. Round 1 and Round 2 are methane measurements carried out in respiration chambers.
A range of measures associated with meat yield and quality were measured. The traits are briefly described below, with full descriptions provided by Brito et al. (2017) and Payne et al. (2009).
Live animal
The liveweight (LWT, kg) at 8 months of age (or if missing, at 6 months of age) was extracted from the SIL database, and to ensure that animals were comparable, data were scaled to a mean liveweight of 42 kg. The preslaughter liveweight (PRESLT, kg) was recorded prior to slaughter at approximately 8 months of age. The dimensions of the M. longissimus (colloquially known as the eye muscle) were made over the 12th rib by a commercial ultrasound operator, specifically eye muscle depth (EMD, mm), width (EMW, mm), and fat depth over the muscle (FDM, mm).
Carcass
The carcass yield and quality traits, measured post-slaughter, varied depending on the source of the animals but included hot carcass weight (kg), butt circumference (CBUTT, cm), GR fat depth over the 12th rib at a distance of 110 mm from the midline (CGRM, mm), carcass length (CLGTH, cm), and leg length (LEGLGTH, cm). For a proportion of the animals that were processed through Alliance Group Ltd. processing plants, the traits estimated using the two-dimensional imaging system VIAscan® (Mclean et al., 2006) were available. VIAscan® (VS) traits included carcass weight (VSCWT, kg), GR as an estimate of carcass fat depth (VSGR, mm), and the percentage of lean meat in the carcass (VSYLD, %), leg (VSLEG, %), loin (VSLOIN, %), and shoulder (VSSHLD, %). The dressing percentage was calculated as the ratio of carcass weight divided by PRESLT (DO%).
The carcass weight (Carc Wt) is a consolidated trait, and for the majority of animals, it represents hot carcass weight; if the hot carcass weight was not measured on an animal, then the cold carcass weight or VSCWT was used, which is directly derived from the hot carcass weight.
Meat Quality
For a proportion of the animals, as indicated in Table 1, at 24 h post-slaughter, the carcass fat color (L*, a*, and b*, using a Minolta Chromometer) was measured on the external surface of the carcass (FATL, FATA, and FATB) as described by Payne et al. (2009), and the pH measurements were made on the M. longissimus (carcass pH; CPH). In the boning room, the area of the M. longissimus (colloquially referred to as the carcass eye muscle area, CEMA) and muscle color (L*, a*, and b*) were measured using a Minolta Chromometer, after allowing a fresh-cut surface of the muscle (colloquially referred to as the loin) to bloom for 30 min (LOINL, LOINA, and LOINB). The M. longissimus was then collected post the boning room, for frozen and tenderness measurements (shear force, SHF, kgF) that were subsequently undertaken as described by Brito et al. (2017). For the other animals, as indicated in Table 1, the M. longissimus was collected, and the measurements were undertaken at the Invermay Agricultural Centre as described by Brito et al. (2017). Specifically, the loin pH (LPH), marbling score, and muscle color and tenderness, as described earlier, were measured. The marbling score (MARB) was assessed on a 1–5 scale and used as a predictor of intramuscular fat content in the M. longissimus in lambs (Brito et al., 2017; Guy et al., 2019).
The carcass data included records from the research and CPT flocks, and data from surplus ram lambs from the high and low methane selection lines (60 born in 2012 and 60 born in 2013). The meat quality data were only available on the ones from the research and CPT flocks.
Statistical analyses
With the exception of the methane trait data, which were stored in an independent database, all other traits and animal data were extracted from the SIL database, together with pedigree information.
Measures of CH4 emissions were expressed as gross emissions (CH4, g/d) and methane yield (g CH4/kg DMI). Intake was based on the measured DMI on the day of the methane measurement. A randomized block design was used, and significant systematic effects and covariates for gross CH4 and CH4/DMI were determined using a general linear model procedure (SAS 2015).
The details of the final models used for the different traits are given in Table 1. For all traits, fixed effects fitted in initial models included birth/rearing rank (brr; born and reared as a combination of triplets, twins, and singletons: 33, 32, 31, 22, 21, and 11) and age of dam at the time of the animal’s birth (aod: 2–5 years). Birthday deviation within a year (bdev) or age at slaughter deviation within a year (SltrAgeDev) were fitted as covariates. The final contemporary groups fitted depended on the trait, but all included birth flock (flk: 2638, 3633, 4640, 4757, or 9153), birth year (byr: 2002–2013), and sex (male or female). Additionally, for the methane traits, recording year of methane measurements (ryr: 2011–2014), lot within a year (lot: mob of 96 animals, 1–5), group within a lot (group: sub-mob of up to 24 animals measured contemporaneously), and round of measurement, as each animal was measured twice with at least 2 weeks between measurements (round: 1 or 2), were also utilized to establish contemporary groups. Specifically, the contemporary groups for methane traits were byr.flk.sex (cg4) and ryr.lot.group.round (cg5). For the non-methane traits, the mob at the time of the measurements was also used to construct contemporary groups.
The final models for each carcass trait are described in Table 1. Interactions between the fixed effects were tested together with weight traits as covariates and, by a process of backward elimination, the parsimonious models were selected.
The phenotypic, genetic, and environmental variances and covariances between methane emissions and carcass or meat quality traits were estimated using the univariate and bivariate (two-trait) analyses undertaken using ASReml 3.0 (Gilmour et al., 2009).
For ultrasound and early liveweight measures from live animals (i.e., preslaughter), all 1,825 animals that had been measured for methane also had records for these traits, therefore, a direct estimation of the genetic and phenotypic correlations was possible. For post-slaughter data, however, the numbers varied per trait. The analysis, therefore, primarily used pedigree relationships to estimate methane. This enabled the use of detailed carcass data collected from all flocks to provide estimates of genetic and phenotypic correlations with methane traits. There were some traits where there were no animals with both methane and the slaughter trait recorded. These were specialist measures of fat color, meat color, pH, and eye muscle area traits recorded on CPT animals. For these traits, the genetic and phenotypic correlations were completely extrapolated based on correlations scaled by relatedness using a numerator relationship matrix within a linear mixed model, as described in detail by Gilmour et al. (2009). Heritabilities were classified as low, if less than 0.15; as moderate, if 0.15—0.30; and as high, if greater than 0.30 (Brito et al., 2017).
Results
The heritabilities for total methane production and methane yield were 0.25 and 0.11, respectively, while the heritabilities for carcass traits were moderate to high (0.14—0.65) (Table 2). The standard errors were low, indicating that estimates were accurate and significantly different from zero.
The gross methane production was positively genetically correlated with liveweight, carcass weight traits, and eye muscle depth and width, while negatively correlated with CGRM-a predictor of carcass fat (Table 3). These significant genetic correlations were also reflected in the phenotypic correlations except for CGRM; however, VSGR had a significant negative phenotypic correlation. The additional significant phenotypic correlations were FDM, dressing-out percentage, butt circumference, and carcass length.
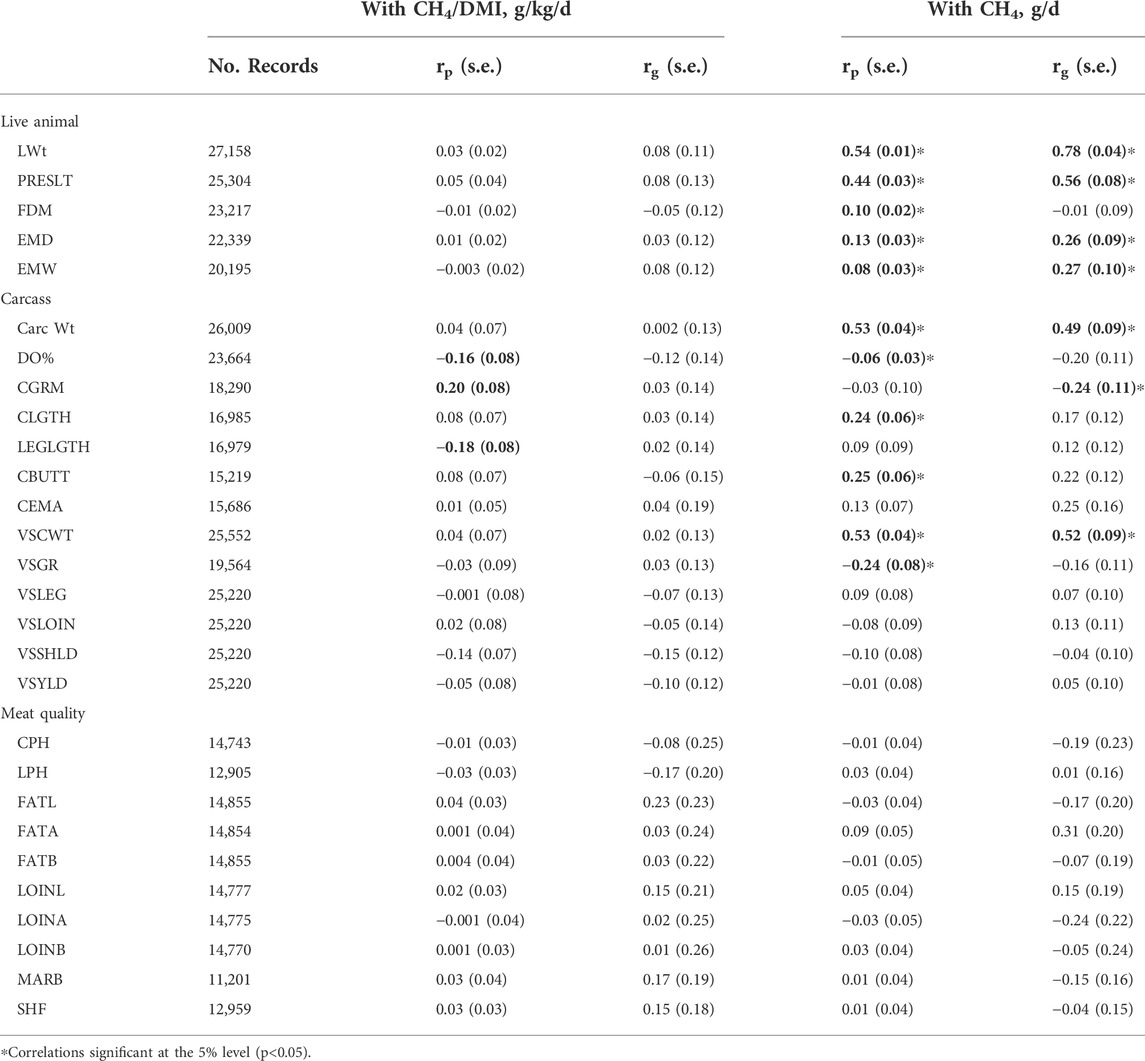
TABLE 3. Phenotypic (rp) and genetic (rg) correlations between methane emissions (g CH4/d) and methane yield (g CH4/kg DMI/d) with live animal, carcass, and meat quality traits. Significant correlations (estimate is greater than twice the standard error) are given in bold. See Table 1 for trait descriptions.
There were no significant genetic correlations between methane yield and carcass and quality traits. There was a significant positive phenotypic correlation with CGRM and negative correlations with dressing-out percentage and leg length.
Although not significant, the VIAscan® lean yield traits tended to have a negative (i.e., favorable) genetic correlation with methane yield.
Discussion
As the initial progenitors of the divergent methane lines were measured in the NZ central progeny test (CPT) flocks, and methane is heritable, we can predict methane yields in CPT animals. This meant that the associated detailed carcass data collected on CPT animals could inform likely trends and effects of selecting for methane in the NZ commercial sheep population.
Heritability estimates for all carcass traits were moderate to high and close to previous estimates in New Zealand sheep (Jopson et al., 2009; Brito et al., 2017). Absolute methane emissions (g/d) were significantly and positively associated with liveweight and associated traits. This is unsurprising as the sheep were fed at a fixed feeding level 2.0 × maintenance metabolizable energy requirements in which liveweight is the main driver of the absolute feed offer (CSIRO, 1990) and with increasing body weights, the animal receives more feed which in turn leads to higher emissions (Swainson et al., 2018; Van Lingen et al., 2021). However, methane yield had no significant correlation with liveweight.
As expected, gross methane emissions were positively genetically correlated with liveweight but less so with carcass weight. There was a low negative genetic correlation between gross methane and dressing-out percentage and also a significant low negative genetic correlation with carcass fat. For methane yield, no genetic correlations with any of the meat and carcass traits were significant. But there were some significant phenotypic correlations that were similar to those previously published, such as higher fat content associated with higher methane yield and lower dressing-out percentage (Pinares-Patino et al., 2013; Elmes et al., 2014). These results together with those previously published suggest a growing body of evidence that selecting for lowered methane yield could lead to animals that are slightly leaner with a higher carcass yield per kilogram of body fat. This is in keeping with the evidence that breeding for lowered methane emissions selects for a reduced acetate to propionate ratio (Jonker et al., 2020). Propionate promotes gluconeogenesis and energy metabolized by the liver, whereas acetate is the primary source of energy metabolism in adipose tissues (Bergman, 1990). Given that the carcass meat yield and dressing-out percentage are traits of considerable economic importance in many sheep industries, it would be useful in future studies investigating selection for altered carcass composition to also monitor rumen volatile fatty acids and methane emissions in order to better estimate this genetic relationship.
Conclusion
These results show that the use of breeding as a mitigation strategy for lower methane yields and the resulting physiological changes do not negatively affect meat quality or carcass traits. Low-emitting animals may even have greater economic value through slightly higher dressing-out rates, decreased fat, and increased meat yields. These results are of importance for all ruminant livestock production systems. They are of particular relevance for meeting the globally agreed targets through the development of robust selection indices that aim to reduce methane emissions while also increasing productivity. Meat production and meat quality are increasingly important goals in sheep production and therefore accurate estimates of genetic and phenotypic parameters of these traits with methane production are essential for any industry implementation. The current work will underpin methane reduction via genetics in the New Zealand industry.
Data availability statement
The original contributions presented in this study are included in the article/Supplementary Material; further inquiries can be directed to the corresponding author.
Ethics statement
The animal study was reviewed and approved by Ruakura Ethics Committee, AgResearch, New Zealand.
Author contributions
JM, CP-P, SH, and SR conceived and designed this study. WB, GG, KK, SE, and EY carried out data collection and refined protocols. SH, JM, and SR wrote the manuscript. SH, AJ, KD, JM, NP, PJ, and SR interpreted and discussed the results. All the authors reviewed and approved the final manuscript.
Funding
This work was financially supported by the Pastoral Greenhouse Gas Research Consortium (PGgRc), the New Zealand Agricultural Greenhouse Gas Research Centre (NZAGRC), and the Sustainable Land Management and Climate Change Program (SLMACC) administered by the Ministry for Primary Industries (Wellington, New Zealand). The animals were part of the Ovita partnership and related central progeny test, both funded in part or whole by Beef + Lamb New Zealand.
Acknowledgments
The authors also thank the central progeny test collaborating organizations: AgResearch Woodlands, Paul Muir of On Farm Research, Chris Logan of Lincoln University, and Neville Jopson of AbacusBio Ltd.
Conflict of interest
The authors declare that the research was conducted in the absence of any commercial or financial relationships that could be construed as a potential conflict of interest.
Publisher’s note
All claims expressed in this article are solely those of the authors and do not necessarily represent those of their affiliated organizations, or those of the publisher, the editors, and the reviewers. Any product that may be evaluated in this article, or claim that may be made by its manufacturer, is not guaranteed or endorsed by the publisher.
Supplementary material
The Supplementary Material for this article can be found online at: https://www.frontiersin.org/articles/10.3389/fgene.2022.911355/full#supplementary-material
References
Bain, W. E., Bezuidenhout, L., Jopson, N. B., Pinares-Patiño, C. S., and McEwan, J. C. (2014). Rumen differences between sheep identified as being low or high methane emitters. Proc. Assoc. Advmt. Anim. Breed. Genet. 20, 376–378. doi:10.13140/2.1.3702.7206
Bergman, E. (1990). Energy contributions of volatile fatty acids from the gastrointestinal tract in various species. Physiol. Rev. 70, 567–590. doi:10.1152/physrev.1990.70.2.567
Bilton, T. P., Hickey, S. M., Janssen, P. H., Jonker, A. J., Hess, M. K., Bryson, B., et al. (2021). Impact of breeding for divergent methane yield on milk composition in breeding ewes. Proc. Assoc. Advmt. Anim. Breed. Genet. 24 (15), 18.
Brito, L. F., McEwan, J. C., Miller, S. P., Bain, W. E., Lee, M. A., Dodds, K. G., et al. (2017). Genetic parameters for various growth, carcass and meat quality traits in a New Zealand sheep population. Small Rumin. Res. 154, 81–91. doi:10.1016/j.smallrumres.2017.07.011
Clark, H. (2009). Methane emissions from ruminant livestock; are they important and can we reduce them? ProNZG. 71, 73–76. doi:10.33584/jnzg.2009.71.2774
Elmes, S. N., Bain, W. E., Greer, G. J., Hickey, S. M., Young, E. A., Pickering, N. K., et al. (2014). An exploratory investigation of the effects of selection for altered methane emissions on rumen physiology and carcass traits in sheep. Proc. N. Z. Soc. Ani. Prod. 74, 142.
FAO (2006). Livestock’s long shadow, environmental issues and options. Rome: Food and agriculture organisation of the United Nations. Accessed January 2021.
Gilmour, A. R., Gogel, B. J., Cullis, B. R., and Thompson, R. (2009). ASReml UserGuide release 3.0. UK: VSN International Ltd, Hemel Hempstead, HP1 1ES.
Goopy, J. P., Donaldson, A., Hegarty, R., Vercoe, P. E., Haynes, F., Barnett, M., et al. (2013). Low methane yield sheep have smaller rumens and shorter rumen retention time. Br. J. Nutr. 8, 578–585. doi:10.1017/s0007114513002936
Guy, S. Z. Y., McGilchrist, P., and Brown, D. J. (2019). Visual marble score as a predictor of intramuscular fat for the genetic improvement of eating quality in lamb. Proc. Assoc. Advmt. Anim. Breed. Genet. 23, 310–313.
Hess, M. K., Donaldson, A., Henry, H. M., Robinson, D. L., Hess, A. S., McEwan, J. C., et al. (2020). Across-country prediction of methane emissions using microbial profiles. Proc. Assoc. Advmt. Anim. Breed. Genet. 24, 163–166.
Jonker, A., Hickey, S., Boma, P., Woyimo Woju, C., Sandoval, E., MacLean, S., et al. (2020). Individual-level correlations of rumen volatile fatty acids with enteric methane emissions for ranking methane yield in sheep fed fresh pasture. Anim. Prod. Sci. 61, 300–305. doi:10.1071/an20128
Jonker, A., Hickey, S., Pinares-Patiño, C., McEwan, J., Olinga, S., Díaz, A., et al. (2017). Sheep from low-methane-yield selection lines created on alfalfa pellets also have lower methane yield under pastoral farming conditions. J. Anim. Sci. 95, 3905–3913. doi:10.2527/jas2017.1709
Jonker, A., Hickey, S. M., Rowe, S. J., Janssen, P. H., Shackell, G. H., Elmes, S., et al. (2018). Genetic parameters of methane emissions determined using portable accumulation chambers in lambs and ewes grazing pasture and genetic correlations with emissions determined in respiration chambers. J. Anim. Sci. 96, 3031–3042. doi:10.1093/jas/sky187
Jopson, N. B., McEwan, J. C., Logan, C. M., and Muir, P. D. (2009). Genetic parameters for primal cut meat yield traits in sheep. Proc. N. Z. Soc. Ani. Prod. 69, 215.
McLean, N. J., Jopson, N. B., Campbell, A. W., Knowler, K., Behrent, M., Cruickshank, G., et al. (2006). An evaluation of sheep meat genetics in New Zealand: The central progeny test CPT). Proc. N. Z. Soc. Ani. Prod. 66, 368–372. doi:10.13140/2.1.2733.6961
Ministry for the Environment (MfE), (2021). New Zealand’s greenhouse gas inventory 1990-2019. An overview. Available at: www.mfe.govt.nz (Accessed 7th, May 2021).
Newman, S. A., McEwan, J. C., and Young, M. J. (2009). A decade of sheep improvement limited (SIL). Proc. Assoc. Advmt. Anim. Breed. Genet. 18, 624–627.
Payne, G. M., Campbell, A. W., Jopson, N. B., McEwan, J. C., Logan, C. M., and Muir, P. D. (2009). Genetic and phenotypic parameter estimates for growth, yield and meat quality traits in lamb. Proc. N. Z. Soc. Ani. Prod. 69, 210–214. doi:10.13140/2.1.1736.6400
Pinares-Patiño, C. S., Hickey, S. M., Young, E. A., Dodds, K. G., MacLean, S., Molano, G., et al. (2013). Heritability estimates of methane emissions from sheep. Animal 7, 316–321. doi:10.1017/S1751731113000864
Rowe, S. J., Hickey, S. M., Jonker, A., Hess, M. K., Janssen, P., Johnson, T., et al. (2019). Selection for divergent methane yield in New Zealand sheep – A ten-year perspective. Proc. Ass. Adv. Ani. Breed. Gene. 23, 306.
Swainson, N., Muetzel, S., and Clark, H. (2018). Updated predictions of enteric methane emissions from sheep suitable for use in the New Zealand national greenhouse gas inventory. Anim. Prod. Sci. 58, 973–979. doi:10.1071/an15766
van Lingen, H. J., Jonker, A., Kebreab, E., and Pacheco, D. (2021). Quantitative joint evaluation of sheep enteric methane emissions and faecal dry matter and nitrogen excretion. Agric. Ecosyst. Environ. 305, 107116. doi:10.1016/j.agee.2020.107116
Waghorn, G. C., and Hegarty, R. S. (2011). Lowering ruminant methane emissions through improved feed conversion efficiency. Animal Feed Sci. Technol. 166, 291–301. doi:10.1016/j.anifeedsci.2011.04.019
Keywords: sheep, respiration chamber, breeding, genetic correlation, methane emissions, meat quality
Citation: Rowe SJ, Hickey SM, Bain WE, Greer GJ, Johnson PL, Elmes S, Pinares-Patiño CS, Young EA, Dodds KG, Knowler K, Pickering NK, Jonker A and McEwan JC (2022) Can we have our steak and eat it: The impact of breeding for lowered environmental impact on yield and meat quality in sheep. Front. Genet. 13:911355. doi: 10.3389/fgene.2022.911355
Received: 02 April 2022; Accepted: 01 August 2022;
Published: 16 September 2022.
Edited by:
Saleh Shahinfar, Agriculture Victoria Research, AustraliaReviewed by:
Mohammad Ferdosi, University of New England, AustraliaAli Sadeghi-Sefidmazgi, University of Tehran, Iran
Copyright © 2022 Rowe, Hickey, Bain, Greer, Johnson, Elmes, Pinares-Patiño, Young, Dodds, Knowler, Pickering, Jonker and McEwan. This is an open-access article distributed under the terms of the Creative Commons Attribution License (CC BY). The use, distribution or reproduction in other forums is permitted, provided the original author(s) and the copyright owner(s) are credited and that the original publication in this journal is cited, in accordance with accepted academic practice. No use, distribution or reproduction is permitted which does not comply with these terms.
*Correspondence: S. J. Rowe, c3V6YW5uZS5yb3dlQGFncmVzZWFyY2guY28ubno=
†Present address: C. S. Pinares-Patiño, Mazingira Centre, International Livestock Research Institute (ILRI), Nairobi, KenyaN. K. Pickering, Focus Genetics, Napier, New Zealand