- 1Key Laboratory of Metabolism and Molecular Medicine, Ministry of Education, Department of Biochemistry and Molecular Biology, School of Basic Medical Sciences, Shanghai Medical College, Fudan University, Shanghai, China
- 2Huashan Hospital, Fudan University, Shanghai, China
- 3Children’s Hospital, Fudan University, Shanghai, China
Gaucher disease (GD) is an autosomal recessive lysosomal storage disorder caused by mutations in the GBA1 gene, which produces the glucocerebrosidase (GCase) protein. There are more than 500 mutations reported in GBA1, among which L444P (p.Leu444Pro) and F213I (p.Phe213Ile) are the most common in the Chinese population, while the function of F213I mutation remains elusive. This study aims to establish the GD mouse model of partially humanized Gba1 gene with F213I mutation. In vitro GCase activity assays showed that the product of partially humanized Gba1 gene, in which the mouse exons 5-7 were replace by the corresponding human exons, displayed similar activity with the wild-type mouse Gba1, while the F213I mutation in the humanized Gba1 led to significant decrease in enzyme activity. ES cell targeting was used to establish the mice expressing the partially humanized Gba1-F213I. Gba1F213I/+ mice did not show obviously abnormal phenotypes, but homozygous Gba1F213I/F213I mice died within 24 h after birth, whose epidermal stratum corneum were abnormal from the wild-type. The GCase activity in Gba1F213I/F213I mice greatly decreased. In conclusion, our results showed that the partially humanized GD mouse model with the F213I mutation was developed and homozygous F213I mutation is lethal for newborn mice.
Introduction
Gaucher disease (GD) is one of the most common lysosomal storage diseases. GD is an autosomal recessive hereditary disease caused by mutations in the gene encoding β- Glucocerebrosidase (GBA1). Due to the deficiency of glucocerebrosidase (GCase) activity, glucosylceramide (GlcCer) accumulates in the lysosomes and is metabolized to produce glucosylsphingosine, sphingosine and then sphingosine-1-phosphate (S1P) (Stirnemann et al., 2017). GD is characterized by enlargement of liver and spleen, lesions in the bones, and, in the most severe cases, neuropathology accompanied by neuroinflammation (Dasgupta et al., 2015; Do et al., 2019; Oguri et al., 2020). According to the impairment of central nervous system function, there are three GD types (Carubbi et al., 2020). Gaucher disease type 1 (GD1) was regarded as the mildest form without obvious neurologic involvement at an early stage, but some GD1 patients developed Parkinson disease phenotype at older age (Kartha et al., 2020). Type 2 is the most severe form and appears as an early onset of neurologic disease with an acute course. Type 3 disease is of intermediate severity with a later onset of neurologic symptoms and a more chronic course (Kartha et al., 2020).
GBA1 is located in human chromosome 1q21-22, 7.2 kb long, composed of 11 exons and 10 introns. More than 500 types of mutations linked to GD have been found in GBA1, including splice site mutation, point mutation, coding frameshift mutation, insertion or deletion mutation (Milenkovic et al., 2022). The clinical features of GD are dictated to a large extent by mutation patterns carried in the GBA1 gene. In China, the most common mutations in GBA1 include L444P (p.Leu444Pro, 33.00%), F213I (p.Phe213Ile, 5.33%) and N188S (p.Asn188Ser, 5.33%) (Zhang et al., 2009). F213I mutation, the A-to-T transversion at nt 754 in exon 6 (NM_000157.4: c.754T > A), is also named F252I (p.Phe252Ile) according to the new nomenclature and is the second common point mutant GBA1 allele in Chinese GD patients (He et al., 1992; Zhang et al., 2009; Oto et al., 2021). The F213I mutation was found in all three types of GD, and F213I-associated types 2 GD and type 3 GD were more prevalent in Asian populations (Koprivica et al., 2000; Tajima et al., 2009; Zhang et al., 2009; Vieira and Schapira, 2021).
Mouse models are widely used in GD research. Several mouse models with common Gba1 mutations in GD patients have been established, such as L444P mice, D409V and N370S mice (Liu et al., 1998; Xu et al., 2003; Jackson et al., 2019; Liou et al., 2019; Migdalska-Richards et al., 2020). However, up to date, there have been no investigation on the F213I mutation in mice. Furthermore, researches on conserved genes have shown that human-mouse chimeric gene can function normally and, based on this finding, exons of mouse genes could be replaced by human counterparts to generate partially humanized mouse model, which will be suitable to detect effectiveness of human genome-editing therapeutic methods in vivo in mice (Dong et al., 2012; Takeuchi et al., 2019; Guo et al., 2021). In this study, a GD mouse model with partially humanized F213I Gba1 was established.
Materials and Methods
Mice and Genotyping
The Institutional Animal Care and Use Committee of Fudan University, China approved all protocols. Mice with partially humanized Gba1 F213I allele (mhGba1-F213I) were generated by using the ES-cell-based gene targeting technology at Shanghai Model Organisms Center in Shanghai, China. Briefly, the ES cell targeting vector was constructed by fusions, containing 3.0 kb 5′ homologous arm, hGBA1 exon 5-7 with F213I mutation, PGK-Neo-poly A, 3.0 kb 3′ homologous arm and MC1-TK-polyA, a negative screening marker. The vector was linearized and transferred into JM8A3 ES cell by electroporation. After PCR identification, the positive ES cell clones were amplified and injected into the blastocysts of C57BL/6J mice to obtain chimeric mice. The Neo-removed Gba1+/F213I mice were obtained by mating chimeric mice with mice with Flp gene. In this way, the exon 5-7 site of the mouse Gba1 gene was replaced by the human GBA1 exon 5–7. The genotype of each mouse was determined by PCR analysis of genomic DNA prepared from tail biopsies. PCR was performed by using the forward primer mGba1-F and the reverse primer mGba1-T for wild type or the forward primer hGBA1-F and the reverse primer hGBA1-T for mutants. The 5′ homology arm and the 3′ homology arm were amplified for sequencing. Sequencing was performed by the Tsingke Biotechnology Co., Ltd. in China. Primer mGba1-5F and hGBA1-5T were designed for 5′ homology arm, while hGBA1-3F and mGba1-3T were designed for 3’ homology arm. The primers are listed in Supplementary Table S1.
mRNA Extraction and qRT-PCR
Total RNA was extracted from tissues using TRIzol reagent (Thermo Fisher) according to the manufacturer’s protocol. RNA degradation and contamination were assessed on 1% agarose gels, and the RNA concentration was measured by using a NanoDrop 1,000 spectrophotometer (Thermo Scientific). cDNA was synthesized by using the Hifair III 1st Strand cDNA Synthesis SuperMix for qPCR (gDNA digester plus; Yeasen), and the integrity of the synthesized cDNA was confirmed by using glyceraldehyde 3-phosphate dehydrogenase (Gapdh) as the endogenous control. Real-time PCR was carried out using SYBR Premix Ex Taq TM II (Perfect Real Time; TaKaRa) and measured by using an ABI 7500 instrument. qRT-PCR was performed by using the forward primer mGapdh-RF and the reverse primer mGapdh-RT for Gapdh or the forward primer mGba1-RF and the reverse primer mGba1-RT for Gba1. The primers are listed in Supplementary Table S1. PCR was performed as reported by Zhang et al. (Zhang et al., 2019).
GCase Expression in vitro
Crispr/Cas9 system was used to reduce the interference of the endogenous GCase activity, as we performed previously (Abbasi et al., 2020). Briefly, HEK293 cells were infected with lentivirus expressing CAS9 protein and sgRNA of GBA1. Infected cells were pooled by using puromycin selection (1ug/ml), and after 7 days, the cells were conducted for other assays. Partially humanized cDNA was constructed by Tsingke Biotechnology Co., Ltd. Single mutagenesis was inserted by overlapping PCR. Wild type mouse cDNA was synthesized by taking mRNA from wild type mice as template. The sequences are listed in Supplementary Table S2. All types of cDNA were subcloned into the PCDH- immediate early enhancer and promoter (CMV)-HA plasmid. Plasmids were transferred into HEK293 cells whose GBA1 was knocked down by CRISPR-Cas9. Cells were harvested for following GCase activity assays and western blotting forty-eight hours after the transient transformation.
GCase Enzyme Activity Assay
The GCase enzyme activity assay on the homogenate samples including sample preparation was performed according to the manufacturer’s instructions of Glucosylceramidase Activity Assay Kit (Fluorometric; BioVision). Fluorescence intensity (Ex/Em = 360/445 nm) was detected in a Multimode Plate Reader (PerkinElmer, EnSpire). During the measurement, blank controls without GCase were set to remove background value. According to the manufacturer’s instructions, specific sample Gucosylceramidase activity = B/(30 × V × P) × D = pmol/min. mg ≡ µU/mg, where B is 4-MU amount from the standard curve (pmol), 30 is the reaction time (min), V is sample volume added into the reaction well (ml), P is initial sample concentration in mg-protein/ml (mg/ml), D is sample dilution factor. One unit of Glucosylceramidase activity is the amount of enzyme that generates 1.0 µmol of 4-Methylumbelliferone per min at pH 4.5 at 37°C. The weight of each mouse tissue has been carefully weighed, and the relative quantity of GCase per mg tissue was calculated, respectively.
Western Blot Analysis
Protein extracts from cells were prepared using a lysis buffer (200 mM Tris-HCl [pH 7.5], 1.5 M NaCl, 10 mM EDTA, 10 mM EGTA, 25 mM sodium pyrophosphate, 10 mM β-glycerophosphate, 1 mM Na3VO4, 50 mM NaF) supplied with a protease inhibitor cocktail (Roche Diagnostics). Protein samples of 20 ug each sample were separated on a 10% polyacrylamide gel and analyzed by Western blot using anti-HA (Proteintech, 51064-2-AP, 1:3,000) and anti-Gapdh (Proteintech, 10494-1-AP, 1:1,000) antibodies. Peroxidase-conjugated rabbit immunoglobulin G (IgG; Jackson ImmunoResearch, 1:2,500) was used as the secondary antibody. Western blots were developed using ImmobilonTM Western Chemiluminescent HRP Substrate (Merck Millipore), and analysis was performed with a Luminescent Image Analyzer (GE, ImageQuant LAS 4000 mini). The results were quantified by using the ImageJ software.
Histological Analysis
Tissues were immersion fixed with 4% neutral-buffered paraformaldehyde, embedded tissues in paraffin blocks and prepared 5 µm sections. Sections of the different tissues were stained with hematoxylin and eosin as reported previously (Xiao et al., 2017; Du et al., 2019). Sections of skin were also stained with period acid-Schiff as reported (Sun et al., 2020).
Statistical Analysis
Statistical significance was assessed using Student’s t-test, as reported in the figure legends. The results were significant at p values under 0.05. All statistical tests were performed using Prism software (GraphPad, version 8.0).
Results
F213I Point Mutation in Partially Humanized GBA1 Gene Led to Decreased GCase Activity
The GBA1 gene is highly conserved in mice and humans. Both of them have 11 exons and exons matched one-to-one (had the same boundaries in both genomes), and matching encoding exons are highly similar (84% sequence identity). F213I, the second popular point mutant GBA1 allele in Chinese GD patients, lies in the exon 6 of human and mouse GBA1 genes. In order to establish the GD mouse model with partially humanized Gba1 gene carrying F213I mutation, we planned to replace mouse Gba1 exons 5-7 with human exons 5-7 carrying the F213I (Figure 1A). First, we detected the effects of the partial humanization on the activity of GCase. In order to exclude the interference of the endogenous GCase activity, the GBA1 gene of human HEK293 cells was knocked down by using Crispr/Cas9 system (Figure 1B). The GBA1-knocked down HEK293 cells were transfected, respectively with the plasmids expressing mouse Gba1 (mGba1), partially humanized Gba1 (mhGba1) and mhGba1 with F213I (mhGba1-F213I). GCase activity assays showed that there was no significant difference in GCase activity between mGba1 and mhGba1 while the activity of mhGba1-F213I was greatly reduced (Figure 1C). Western botting was carried out to detect the expression of the different types of HA-tagged GCase (Figure 1D). Considering the relative values of GCase activity to protein amount, the activity of the mhGba-F213I protein production was about 19% of the mGBA, while the activity of. mhGBA was similar to the mGba.
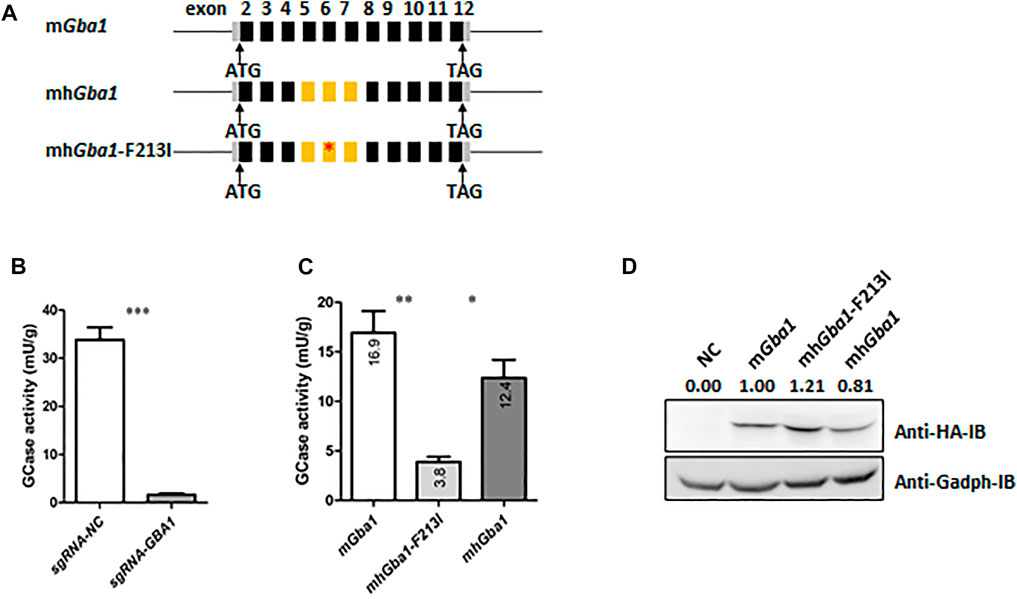
FIGURE 1. F213I point mutation in partially humanized Gba1 gene led to decreased GCase activity in vitro. (A) Structures of the mhGba1-WT and mhGba1-F213I expression plasmids. Exons 5-7 of mouse Gba1 cDNA are replaced by exons 5-7 of human GBA1. F213I mutation was introduced into the partially humanized Gba1 cDNA. Black bars represent mouse Gba1 coding exons. Grey bars represent Gba1 uncoding exons. Lines between bars represent introns. Yellow bars represent human GBA1 coding exons, and red asterisk represents F213I mutation site. (B) GCase activity was detected in the Crispr/Cas9-mediated GBA1-knockdown human HEK293 cells, using normal human HEK293 cells as control. To reduce interference of the endogenous GCase activity, the human HEK293 cells were infected with lentivirus expressing CAS9 protein and sgRNA of GBA1, pooled by using puromycin selection (1 ug/ml) for 7 days and were collected for the GCase activity assays. The results were expressed as the mean—SEM and difference between groups was analyzed by Student’s t-test, ***p < 0.001. (C) The endogenous GBA1-knocked down human HEK293 cells were transfected respectively with the mhGba1-WT, mhGba1-F213I and mGba1-WT expression plasmids, and collected for GCase activity assays 48 h after transfection. The results were expressed as the mean—SEM and differences between every two group were analyzed by Student’s t-test, *p < 0.05, **p < 0.01. (D) Western blot was used to detect the expression of the different types of HA-tagged GCase, with Gapdh as an internal reference. Results were quantified with the ImageJ software, and the relative expression values were labelled, taking the mGba1 as 100%.
Establishment of GD Mouse Model With Partially Humanized Gba1 Gene and F213I Point Mutation
By using gene targeting technology, the mouse Gba1 genomic DNA fragment containing exons 5 to 7 were substituted by the human counterparts carrying the F213I mutation. The upstream and downstream recombination boundaries were validated by DNA sequencing, revealing that the mouse Gba1 DNA fragment from exon 5 to 7 was correctly replaced with the humanized fragment (Figure 2A). Genomic PCR was used for genotyping (Figure 2B). Gba1+/F213I mice were obtained by crossing chimeric mice with wild-type mice and displayed no obvious abnormality. Up to now, we have not observed the symptoms of Parkinson disease in 6-month-old F213I heterozygotes. A lifespan observation of the F213I mice may be needed in future research.
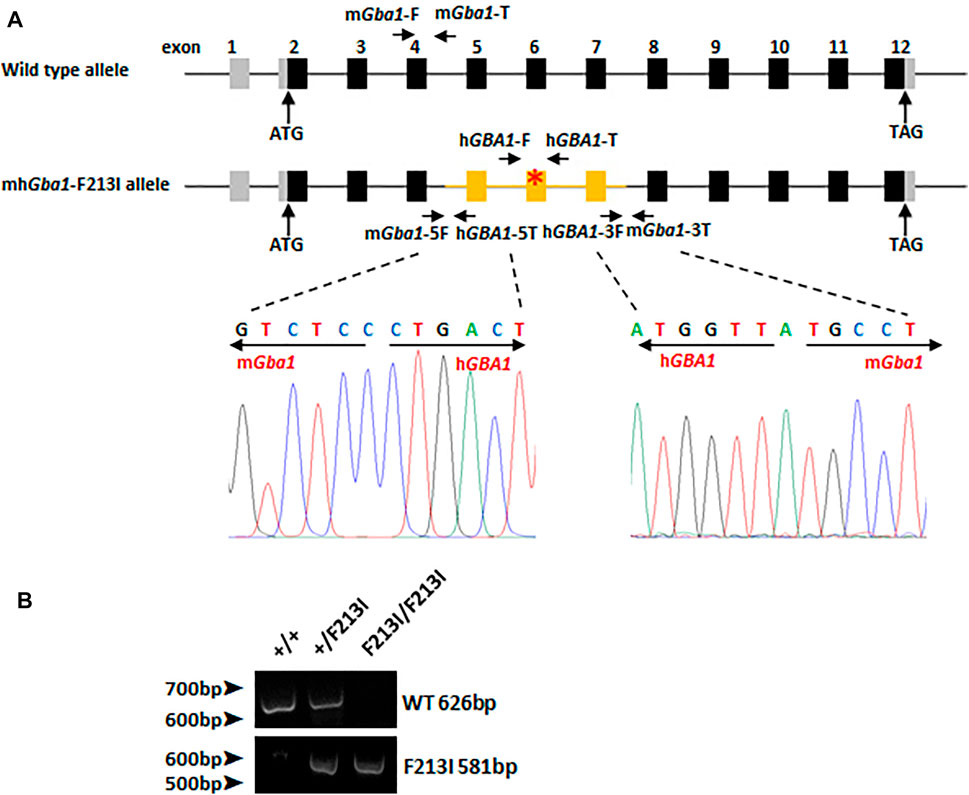
FIGURE 2. The construction of the mhGba1-F213I mice. (A) A general scheme of the genome of wild type mice and mhGba1-F213I mice. Black bars represent mouse Gba1 coding exons. Grey bars represent Gba1 uncoding exons. Yellow bars represent human GBA1 coding exons, and red asterisk represents mutation site. The WT allele of Gba1 was measured by using the mGba1-F primer with the reverse mGba1-T primer. The mhGba-F213I allele was measured by using the hGBA1-F primer with the reverse hGBA1-T primer. Recombination sites were amplified by PCR and sequenced to confirm the homologous substitution. Primer mGba1-5F and hGBA1-5T were used for upstream recombination boundary sequencing. Primer hGBA1-3F and mGba1-3T were used for downstream recombination boundary sequencing. (B) PCR analysis of DNA extracted from tails of Gba1 (+/+) mice, Gba1 (F213I/+) mice and Gba1 (F213I/F213I) mice for genotype identification.
Early Postnatal Lethality in Mice With the Homozygous F213I Mutation
Genotype and survival statistics on offspring of Gba1+/F213I mice were collected (Figure 3A). Homozygous mhGba1-F213I mutant mice were severely affected with small body size and turgor, red, and wrinkled appearance (Figure 3B). Sprinkling water to increase the humidity of the cage can prolong their survival time, and H&E staining of brain, liver and skin showed that no obvious Gaucher cells were found in available living Gba1F213I/F213I mice at P0, which was similar to the L444P mice (Liu et al., 1998). In the skin of Gba1F213I/F213I mice and inbred controls, all the four layers—basal (stratum basal), spinous (stratum spinosum), granular (stratum granulosum), and cornified (stratum corneum)—were identified in the epidermis. The Gba1F213I/F213I cornified layer appeared abnormal organization. Compared with wildtype littermate controls, the stratum corneum of newborn Gba1F213I/F213I mice was more compact between layers and more basophilic (Figure 3C). The Periodic Acid–Schiff (PAS) carbohydrate stain is a method that can detect glycolipids accumulation including glucosylceramide (Bogoeva and Petrusevska, 2001; Farfel-Becker et al., 2014), and the result showed that PAS-positive granules in the granular and spinous layers were more prominent in Gba1F213I/F213I mice (Figure 3D).
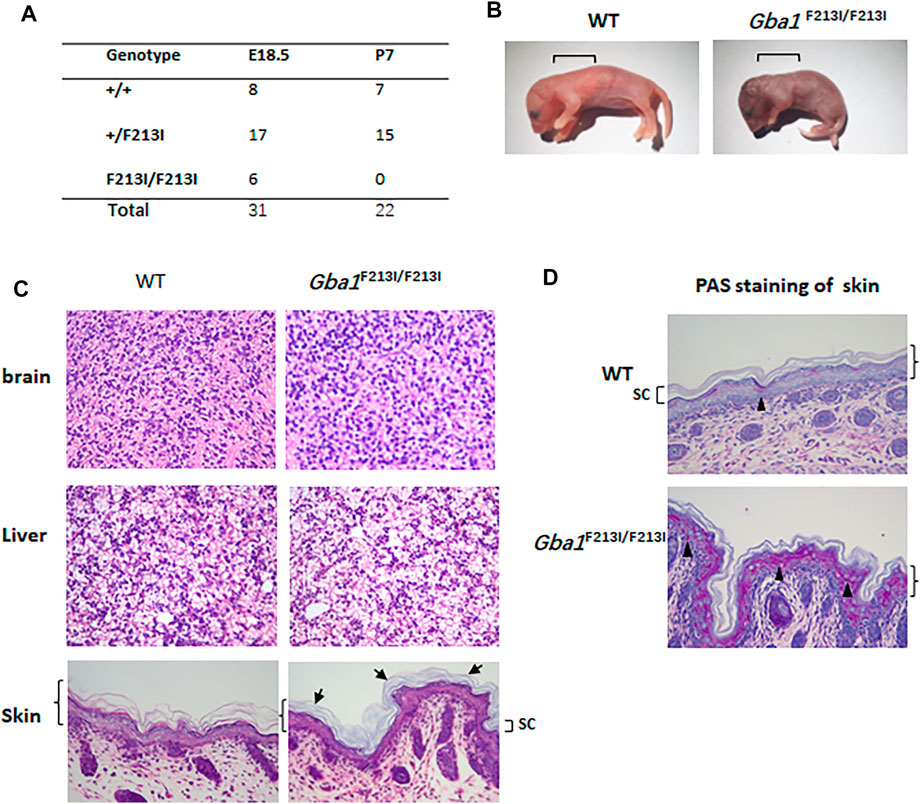
FIGURE 3. Mice with homozygous F213I mutation died postnatally within 24 h and displayed abnormal epidermis structure. (A) The number of embryos at E18.5 and the number of neonates at P14. Homozygotes for the F213I mutation died within 24 h after birth. (B) Photographs of the mice within 12 h after birth. Note the smaller size and wrinkled skin of the F213I mouse. The square brackets indicate the sites where skin samples were taken for the photomicrographs in C. (C) H&E images of brains, livers and epidermis. Tissues were collected from F213I homozygotes and wildtype littermate controls within 12 h after birth and processed for staining. The angle bracket indicates the epidermal layer. No significant differences are noted in the three other layers of the epidermis (stratum basal, stratum spinosum, and stratum granulosum) or the dermis. Stratum corneum (SC) was indicated by square basket. SC of newborn Gba1F213I/F213I mice was more basophilic, and more compact between layers, which was indicated by arrowheads. (D) PAS images of epidermis (×150). The angle bracket indicates the epidermal layer. Stratum corneum (SC) was indicated by square basket. Black triangles are used to highlight PAS-positive granules. PAS-positive granules in the granular and spinous layers were more prominent in the F213I mice than in control mice.
GCase Activity Decreased in F213I Mutation Mice
cDNA from Gba1F213I/F213I mice was sequenced to confirm the correct splicing of the partially humanized Gba1 mRNA, as designed (Figure 4A). Gba1 mRNA expression in E17 whole embryos was measured by using quantitative reverse-transcription polymerase chain reaction (qRT-PCR), and the results showed that the expression of Gba1 transcripts had no significant difference between Gba1F213I/F213I mice and wild type mice (Figure 4B). A large reduction in GCase activity was found in extracts from skin, liver and brain from Gba1F213I/F213I mice. Activity in the F213I mouse tissues was about 20% of normal controls, consistent with the values of clinic GD patients carrying the F213I mutation (Figure 4C).
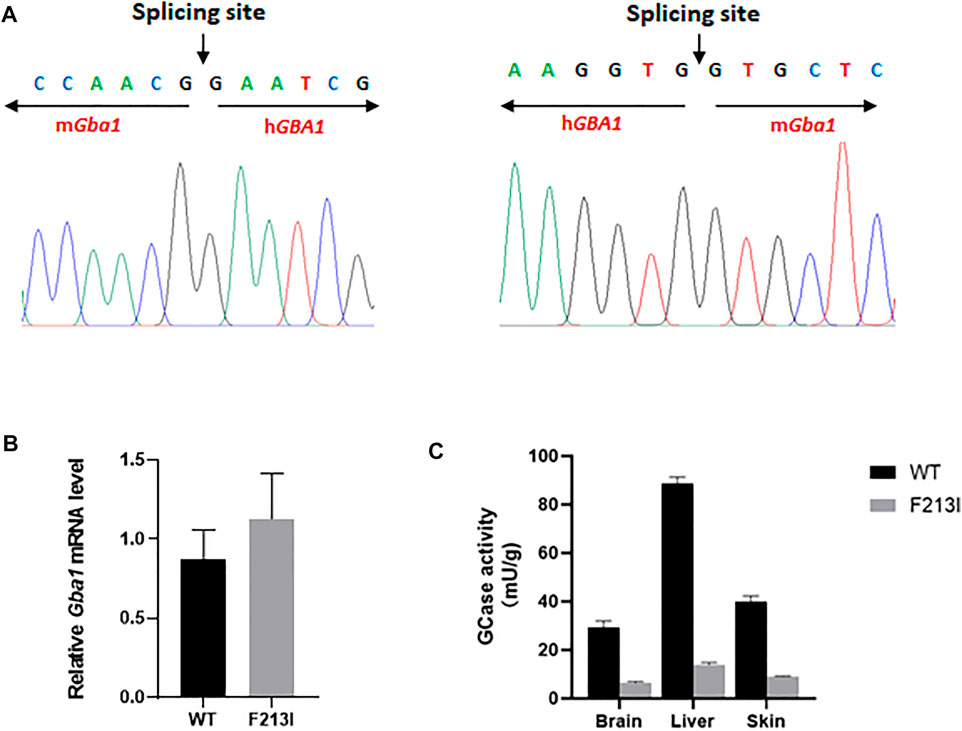
FIGURE 4. GCase activity decreased in F213I mutation mice. (A) Sequencing results of the splice sites of mhGba1-F213I mRNA. The RNA was isolated from the brain of Gba1F213I/F213I mice on P0 day and reverse-transcripted. The splice sites were amplified by PCR and sequenced. The result showed that the partially humanized mhGba1-F213I gene was correctly spliced. (B) qRT-PCR analysis of mRNA extracted from Gba1F213I/F213I mice (n = 3) and wild type mice (n = 3) at embryonic day 17, revealing no significant mRNA expression difference between Gba1F213I/F213I mice and wild type mice. Results were expressed as the mean—standard error of the mean (SEM) and the difference between the groups was analyzed by Student’s t-test. (C) GCase activity was detected in the skin, brain and liver of Gba1F213I/F213I mice (n = 3) on P0 day, using wild type mice (n = 3) as controls. The results were expressed as the mean—SEM and difference between groups was analyzed by Student’s t-test, ***p < 0.001. ****p < 0.0001.
Discussion
GD is a common lysosomal storage disease in humans. It is caused by mutations in the gene (GBA1) coding for GCase, which lead to the accumulation of glucosylceramide in lysosomes (Stirnemann et al., 2017). There are nearly 500 types of allele variants reported in GBA1. Due to the distribution and diversity of human genetic variation, the frequency of each mutant allele varies. Several genetically modified GD mouse models have been established. The first GCase-deficient mouse was created by insertion of a neomycin resistance gene into Gba1 gene, and died shortly after birth (Tybulewicz et al., 1992). Inducible Gba1 deletion mouse models, in which the exons 9–11 are flanked by LoxP sites, was generated in 2006 (Enquist et al., 2006; Du et al., 2019). To simulate the gene mutation of GD patients, some Gba1 point mutation GD mouse models were developed, such as L444P mice, D409V mice, and RecNciI (L444p and A456P double mutation) mice (Strasberg et al., 1994; Liu et al., 1998; Weber et al., 2021). F213I allele is the second high-frequency point mutation of GBA1 gene in Chinese GD patients. So far, there have been few studies and no animal model on this allele. Therefore, to investigate the function of F213I allele and develop a model for future therapy by gene editing, we constructed a GD mouse model with partially humanized Gba1 F213I allele.
Previous studies showed that it was feasible for conserved genes to exchange corresponding conserved exons to generate human-mouse chimeric gene without affecting the gene function. GBA1 gene is highly conserved in human and mouse. In this research, the mouse Gba1 exon 5-7 was replaced by the human counterparts to generate the human-mouse chimeric Gba1 gene (mhGba1) and in vitro GCase assays showed that the partial humanization had little effect on the activity of GCase, while the F213I mutation in mhGba1 (mhGba1-F213I) greatly reduced the activity. To generate the mhGba1-F213I mice, the mouse genomic fragment containing exons 5-7 were replaced by human corresponding region carrying F213I mutation, and the correctly spliced mature mRNA of mhGba1-F213I was generated. GCase activity assay revealed that the GCase activity decreased in both central and peripheral tissues of Gba1F213I/F213I mice. However, like the previously reported Gba1 point mutation mice, homozygous Gba1F213I/F213I mice died within 24 h after birth (Liu et al., 1998). So far, GD patients homozygous for N370S (p.Asn370Ser) or L444P mutations have been reported, and F213I was only found in compound heterozygote forms with N370S or L444P, but skin abnormalities were not diagnosed in these GD patients (Koprivica et al., 2000; Choy et al., 2007). Our results showed that the F213I homozygotes died within 24 h of birth and had red, wrinkled, dry skin that was indicative of disruption of the skin permeability barrier, resembling the phenotypes of mice homozygous for Gba1 knockout, L444P or N370S mutation (Tybulewicz et al., 1992; Liu et al., 1998; Xu et al., 2003). Saposin C enhances GCase activity and protects GCase from intracellular proteolysis, Gba1D409V/D409V:Saposin Cnull/null mice also displayed similar skin phenotypes (Liou et al., 2019). As explained by Liu, Y., epidermal abnormalities were not observed in Gaucher patients, which may arise from the differences in skin barrier formation during fetal development (Liu et al., 1998). In rodents a competent skin permeability barrier forms very late in gestation 1–2 days before birth, while the permeability barrier normally forms well before birth at between 30 and 34 weeks of gestation in human, which provides enough time for residual GCase mediated conversion of glucosylceramide to ceramide during this period to produce a competent barrier (Holleran et al., 1994; Kalia et al., 1998; Doering et al., 2002). However, the residual level of GCase activity in F213I mice may be insufficient to completely process the epidermal glucosylceramide in this short time period during gestation. Infants with less residual level of GCase activity have been described to have a severe skin phenotype (Sidransky et al., 1996). Another factor contributing to the glucosylceramide storage in epidermis but not in brain and liver in the F213I mouse could be related to biochemical differences of the glucosylceramides found in different tissues (Liu et al., 1998), for example skin contains glucosylceramides with additional hydroxyl groups and with very long chain fatty acids, in addition to common types of glucosylceramides like those found in brain and liver (Wertz, 1992). The F213I mutation may render the GCase enzyme less active against the hydroxylated glucosylceramides with long chain fatty acids than against the common types of glucosylceramides, resulting in storage restricted to epidermis.
Enzyme replacement therapy (ERT) and substrate reducing therapy (SRT) are used as clinical therapeutic methods, and AAV-mediated gene addition has been investigated by other researchers and our team (Du et al., 2019; Hurvitz et al., 2019; Jackson et al., 2019; Peng et al., 2021). Gene editing or repairing could be an alternative treatment method for GD disease, but no researches have been conducted. Because this mhGba1-F213I mice model has the human genomic DNA around F231I mutation site in mouse Gba1 allele, it will be suitable to detect Crispr/CAS9-mediated repairing of human GBA1-F213I mutation in this model. And as we described previously, Ubc-CreERT2-induced global Gba1 knockout (Gba1Flox/Flox:Ubc-CreERT2 mice) can solve the problem of early postnatal death (Du et al., 2019). It will be interesting to detect whether Gba1F213I/Flox:Ubc-CreERT2 mice have extended survival time and can be used for future gene edition therapy. Several researches have reported that the carbohydrate mimic N-octyl-β-valienamine (NOV) up-regulated cellular enzyme activity of some GCase mutants in cultured GD fibroblasts, including F213I, N188S, G202R and N370S (Lin et al., 2004; Lei et al., 2007; Luan et al., 2010), so it will be interesting to detect the therapeutic effects of NOV in Gba1F213/F213I mice. Because F213I homozygotes die early after birth, it will be feasible to treat pregnant mice and detect its effects on the pups and enzyme activity.
Now, it is clear that the presence of GBA1 mutation in homozygous or heterozygous form is associated with an approximately 20-fold increase in the risk for Parkinson disease (PD) (Schapira, 2015; Gegg and Schapira, 2018; Do et al., 2019). F213I was the second most common mutation in patients with Gaucher disease (14%), but its mutation frequency was relatively low (only 2% of the pathogenic variants in patients with PD) (Mitsui et al., 2009; Sun et al., 2010). Up to now, we have not observed the symptoms of Parkinson disease in 6-month-old F213I heterozygotes. A lifespan observation of the F213I mice may be needed in future research.
Moreover, GBA1 has a pseudogene GBAP1, acting as competing-endogenous RNA (ceRNA) to regulate GBA1 expression (Straniero et al., 2017). It is possible that GBAP1 is involved in the pathogenesis of PD and GD, and manipulation of GBAP1 may have potential therapeutic effects on the diseases. A research also designed specific easy-to-use CRISPR-Cas9 gene editing strategy to correct the common GBA1 N370S mutation and to ensure the integrity of this pseudogene (Hanss et al., 2019). Mice lack the pseudogene that is present in humans and apes, so we should consider this deficiency when using GD mouse models, for example it cannot be excluded that the absence of the pseudogene in mice may affect the manifestation of PD or GD symptoms.
In summary, our research revealed that F213I mutation caused early postnatal lethality and this partially humanized mouse GD model has the potential for future gene repairing researches in vivo.
Data Availability Statement
The raw data supporting the conclusions of this article will be made available by the authors, without undue reservation.
Ethics Statement
The animal study was reviewed and approved by The Institutional Animal Care and Use Committee of Fudan University, China.
Author Contributions
DM and JZ conceived the idea. NJ conducted the analyses. J-NG provided the data. All authors contributed to the writing and revisions.
Funding
This work was supported by the National Key Research and Development Program of China (No. 2021YFC2700803), Commission of Science and Technology of Shanghai (No. 18411953300), the National Natural Science Foundation of China (No. 81971197) and Fudan Undergraduate Research Opportunities Program (FDUROP, No. 20070).
Conflict of Interest
The authors declare that the research was conducted in the absence of any commercial or financial relationships that could be construed as a potential conflict of interest.
Publisher’s Note
All claims expressed in this article are solely those of the authors and do not necessarily represent those of their affiliated organizations, or those of the publisher, the editors and the reviewers. Any product that may be evaluated in this article, or claim that may be made by its manufacturer, is not guaranteed or endorsed by the publisher.
Supplementary Material
The Supplementary Material for this article can be found online at: https://www.frontiersin.org/articles/10.3389/fgene.2022.892457/full#supplementary-material
References
Abbasi, F., Kodani, M., Emori, C., Kiyozumi, D., Mori, M., Fujihara, Y., et al. (2020). CRISPR/Cas9-Mediated Genome Editing Reveals Oosp Family Genes Are Dispensable for Female Fertility in Mice. Cells 9 (4). doi:10.3390/cells9040821
Bogoeva, B., and Petrusevska, G. (2001). Immunohistochemical and Ultrastructural Features of Gaucher's Cells-Ffive Case Reports. Acta Med. Croat. 55 (3), 131
Carubbi, F., Cappellini, M. D., Fargion, S., Fracanzani, A. L., and Nascimbeni, F. (2020). Liver Involvement in Gaucher Disease: A Practical Review for the Hepatologist and the Gastroenterologist. Dig. Liver Dis. 52 (4), 368–373. doi:10.1016/j.dld.2020.01.004
Choy, F. Y. M., Zhang, W., Shi, H.-P., Zay, A., Campbell, T., Tang, N., et al. (2007). Gaucher Disease Among Chinese Patients: Review on Genotype/phenotype Correlation from 29 Patients and Identification of Novel and Rare Alleles. Blood Cells, Mol. Dis. 38 (3), 287–293. doi:10.1016/j.bcmd.2006.11.003
Dasgupta, N., Xu, Y. H., Li, R., Peng, Y., Pandey, M. K., Tinch, S. L., et al. (2015). Neuronopathic Gaucher Disease: Dysregulated mRNAs and miRNAs in Brain Pathogenesis and Effects of Pharmacologic Chaperone Treatment in a Mouse Model. Hum. Mol. Genet. 24 (24), 7031–7048. doi:10.1093/hmg/ddv404
Do, J., McKinney, C., Sharma, P., and Sidransky, E. (2019). Glucocerebrosidase and its Relevance to Parkinson Disease. Mol. Neurodegener. 14 (1), 36. doi:10.1186/s13024-019-0336-2
Doering, T., Brade, H., and Sandhoff, K. (2002). Sphingolipid Metabolism during Epidermal Barrier Development in Mice. J. Lipid Res. 43 (10), 1727–1733. doi:10.1194/jlr.m200208-jlr200
Dong, Q., Ostedgaard, L. S., Rogers, C., Vermeer, D. W., Zhang, Y., and Welsh, M. J. (2012). Human-mouse Cystic Fibrosis Transmembrane Conductance Regulator (CFTR) Chimeras Identify Regions that Partially Rescue CFTR-Δf508 Processing and Alter its Gating Defect. Proc. Natl. Acad. Sci. U.S.A. 109 (3), 917–922. doi:10.1073/pnas.1120065109
Du, S., Ou, H., Cui, R., Jiang, N., Zhang, M., Li, X., et al. (2019). Delivery of Glucosylceramidase Beta Gene Using AAV9 Vector Therapy as a Treatment Strategy in Mouse Models of Gaucher Disease. Hum. Gene Ther. 30 (2), 155–167. doi:10.1089/hum.2018.072
Enquist, I. B., Nilsson, E., Ooka, A., Månsson, J.-E., Olsson, K., Ehinger, M., et al. (2006). Effective Cell and Gene Therapy in a Murine Model of Gaucher Disease. Proc. Natl. Acad. Sci. U.S.A. 103 (37), 13819–13824. doi:10.1073/pnas.0606016103
Farfel-Becker, T., Vitner, E. B., Kelly, S. L., Bame, J. R., Duan, J., Shinder, V., et al. (2014). Neuronal Accumulation of Glucosylceramide in a Mouse Model of Neuronopathic Gaucher Disease Leads to Neurodegeneration. Hum. Mol. Genet. 23 (4), 843–854. doi:10.1093/hmg/ddt468
Gegg, M. E., and Schapira, A. H. V. (2018). The Role of Glucocerebrosidase in Parkinson Disease Pathogenesis. FEBS J. 285 (19), 3591–3603. doi:10.1111/febs.14393
Guo, Y., Kawaguchi, A., Takeshita, M., Sekiya, T., Hirohama, M., Yamashita, A., et al. (2021). Potent Mouse Monoclonal Antibodies that Block SARS-CoV-2 Infection. J. Biol. Chem. 296, 100346. doi:10.1016/j.jbc.2021.100346
Hanss, Z., Boussaad, I., Jarazo, J., Schwamborn, J. C., and Krüger, R. (2019). Quality Control Strategy for CRISPR-Cas9-Based Gene Editing Complicated by a Pseudogene. Front. Genet. 10, 1297. doi:10.3389/fgene.2019.01297
He, G.-S., Grace, M. E., and Grabowski, G. A. (1992). Gaucher Disease: Four Rare Alleles Encoding F213I, P289L, T323I, and R463C in Type 1 Variants. Hum. Mutat. 1 (5), 423–427. doi:10.1002/humu.1380010513
Holleran, W. M., Ginns, E. I., Menon, G. K., Grundmann, J. U., Fartasch, M., McKinney, C. E., et al. (1994). Consequences of Beta-Glucocerebrosidase Deficiency in Epidermis. Ultrastructure and Permeability Barrier Alterations in Gaucher Disease. J. Clin. Invest. 93 (4), 1756–1764. doi:10.1172/jci117160
Hurvitz, N., Dinur, T., Becker-Cohen, M., Cozma, C., Hovakimyan, M., Oppermann, S., et al. (2019). Glucosylsphingosine (Lyso-Gb1) as a Biomarker for Monitoring Treated and Untreated Children with Gaucher Disease. Int. J. Mol. Sci. 20 (12), 3033. doi:10.3390/ijms20123033
Jackson, K. L., Viel, C., Clarke, J., Bu, J., Chan, M., Wang, B., et al. (2019). Viral Delivery of a microRNA to Gba to the Mouse Central Nervous System Models Neuronopathic Gaucher Disease. Neurobiol. Dis. 130, 104513. doi:10.1016/j.nbd.2019.104513
Kalia, Y. N., Nonato, L. B., Lund, C. H., and Guy, R. H. (1998). Development of Skin Barrier Function in Premature Infants. J. Investigative Dermatology 111 (2), 320–326. doi:10.1046/j.1523-1747.1998.00289.x
Kartha, R. V., Terluk, M. R., Brown, R., Travis, A., Mishra, U. R., Rudser, K., et al. (2020). Patients with Gaucher Disease Display Systemic Oxidative Stress Dependent on Therapy Status. Mol. Genet. Metabolism Rep. 25, 100667. doi:10.1016/j.ymgmr.2020.100667
Koprivica, V., Stone, D. L., Park, J. K., Callahan, M., Frisch, A., Cohen, I. J., et al. (2000). Analysis and Classification of 304 Mutant Alleles in Patients with Type 1 and Type 3 Gaucher Disease. Am. J. Hum. Genet. 66 (6), 1777–1786. doi:10.1086/302925
Lei, K., Ninomiya, H., Suzuki, M., Inoue, T., Sawa, M., Iida, M., et al. (2007). Enzyme Enhancement Activity of N-Octyl-β-Valienamine on β-glucosidase Mutants Associated with Gaucher Disease. Biochimica Biophysica Acta (BBA) - Mol. Basis Dis. 1772 (5), 587–596. doi:10.1016/j.bbadis.2007.02.003
Lin, H., Sugimoto, Y., Ohsaki, Y., Ninomiya, H., Oka, A., Taniguchi, M., et al. (2004). N-Octyl-β-valienamine Up-Regulates Activity of F213I Mutant β-glucosidase in Cultured Cells: a Potential Chemical Chaperone Therapy for Gaucher Disease. Biochimica Biophysica Acta (BBA) - Mol. Basis Dis. 1689 (3), 219–228. doi:10.1016/j.bbadis.2004.03.007
Liou, B., Zhang, W., Fannin, V., Quinn, B., Ran, H., Xu, K., et al. (2019). Combination of Acid β-glucosidase Mutation and Saposin C Deficiency in Mice Reveals Gba1 Mutation Dependent and Tissue-specific Disease Phenotype. Sci. Rep. 9 (1), 5571. doi:10.1038/s41598-019-41914-7
Liu, Y., Suzuki, K., Reed, J. D., Grinberg, A., Westphal, H., Hoffmann, A., et al. (1998). Mice with Type 2 and 3 Gaucher Disease Point Mutations Generated by a Single Insertion Mutagenesis Procedure (SIMP). Proc. Natl. Acad. Sci. U.S.A. 95 (5), 2503–2508. doi:10.1073/pnas.95.5.2503
Luan, Z., Ninomiya, H., Ohno, K., Ogawa, S., Kubo, T., Iida, M., et al. (2010). The Effect of N-Octyl-β-Valienamine on β-glucosidase Activity in Tissues of Normal Mice. Brain Dev. 32 (10), 805–809. doi:10.1016/j.braindev.2009.12.005
Migdalska-Richards, A., Wegrzynowicz, M., Harrison, I. F., Verona, G., Bellotti, V., Spillantini, M. G., et al. (2020). L444P Gba1 Mutation Increases Formation and Spread of α-synuclein Deposits in Mice Injected with Mouse α-synuclein Pre-formed Fibrils. PLoS One 15 (8), e0238075. doi:10.1371/journal.pone.0238075
Milenkovic, I., Blumenreich, S., and Futerman, A. H. (2022). GBA Mutations, Glucosylceramide and Parkinson's Disease. Curr. Opin. Neurobiol. 72, 148–154. doi:10.1016/j.conb.2021.11.004
Mitsui, J., Mizuta, I., Toyoda, A., Ashida, R., Takahashi, Y., Goto, J., et al. (2009). Mutations for Gaucher Disease Confer High Susceptibility to Parkinson Disease. Arch. Neurol. 66 (5), 571–576. doi:10.1001/archneurol.2009.72
Oguri, M., Saito, Y., Okanishi, T., Matuura, Y., Akiyama, S., Ikeguchi, T., et al. (2020). High-frequency Component in Flash Visual Evoked Potentials in Type 3 Gaucher Disease. Brain Dev. 42 (1), 19–27. doi:10.1016/j.braindev.2019.08.005
Oto, Y., Inoue, T., Nagai, S., Tanaka, S., Itabashi, H., Shiraisihi, M., et al. (2021). Successful Treatment of Gaucher Disease Type 1 by Enzyme Replacement Therapy over a 10-year Duration in a Japanese Pediatric Patient: A Case Report. Exp. Ther. Med. 21 (3), 246. doi:10.3892/etm.2021.9677
Peng, Y., Liu, B., and Lin, H. (2021). Substrate Reduction Therapy Reverses Mitochondrial, mTOR, and Autophagy Alterations in a Cell Model of Gaucher Disease. Cells 10 (9), 2286. doi:10.3390/cells10092286
Schapira, A. H. V. (2015). Glucocerebrosidase and Parkinson Disease: Recent Advances. Mol. Cell. Neurosci. 66 (Pt), 37–42. doi:10.1016/j.mcn.2015.03.013
Sidransky, E., Tayebi, N., Stubblefield, B. K., Eliason, W., Klineburgess, A., Pizzolato, G. P., et al. (1996). The Clinical, Molecular, and Pathological Characterisation of a Family with Two Cases of Lethal Perinatal Type 2 Gaucher Disease. J. Med. Genet. 33 (2), 132–136. doi:10.1136/jmg.33.2.132
Stirnemann, J., Belmatoug, N., Camou, F., Serratrice, C., Froissart, R., Caillaud, C., et al. (2017). A Review of Gaucher Disease Pathophysiology, Clinical Presentation and Treatments. Int. J. Mol. Sci. 18 (2), 441. doi:10.3390/ijms18020441
Straniero, L., Rimoldi, V., Samarani, M., Goldwurm, S., Di Fonzo, A., Krüger, R., et al. (2017). The GBAP1 Pseudogene Acts as a ceRNA for the Glucocerebrosidase Gene GBA by Sponging miR-22-3p. Sci. Rep. 7 (1), 12702. doi:10.1038/s41598-017-12973-5
Strasberg, P. M., Skomorowski, M. A., Warren, I. B., Hilson, W. L., Callahan, J. W., and Clarke, J. T. R. (1994). Homozygous Presence of the Crossover (Fusion Gene) Mutation Identified in a Type II Gaucher Disease Fetus: Is This Analogous to the Gaucher Knock-Out Mouse Model? Biochem. Med. Metabolic Biol. 53 (1), 16–21. doi:10.1006/bmmb.1994.1052
Sun, Q.-Y., Guo, J.-F., Wang, L., Yu, R.-H., Zuo, X., Yao, L.-Y., et al. (2010). Glucocerebrosidase Gene L444P Mutation Is a Risk Factor for Parkinson's Disease in Chinese Population. Mov. Disord. 25 (8), 1005–1011. doi:10.1002/mds.23009
Sun, W., Li, A., Wang, Z., Sun, X., Dong, M., Qi, F., et al. (2020). Tetramethylpyrazine Alleviates Acute Kidney Injury by Inhibiting NLRP3/HIF-1α and A-poptosis. Mol. Med. Rep. 22 (4), 2655–2664. doi:10.3892/mmr.2020.11378
Tajima, A., Yokoi, T., Ariga, M., Ito, T., Kaneshiro, E., Eto, Y., et al. (2009). Clinical and Genetic Study of Japanese Patients with Type 3 Gaucher Disease. Mol. Genet. Metabolism 97 (4), 272–277. doi:10.1016/j.ymgme.2009.05.001
Takeuchi, A., Mohri, S., Kai, H., Tamaoka, A., Kobayashi, A., Mizusawa, H., et al. (2019). Two Distinct Prions in Fatal Familial Insomnia and its Sporadic Form. Brain Commun. 1 (1), fcz045. doi:10.1093/braincomms/fcz045
Tybulewicz, V. L. J., Tremblay, M. L., LaMarca, R., Willemsen, R., Stubblefield, B. K., Winfield, S., et al. (1992). Animal Model of Gaucher's Disease from Targeted Disruption of the Mouse Glucocerebrosidase Gene. Nature 357 (6377), 407–410. doi:10.1038/357407a0
Vieira, S. R. L., and Schapira, A. H. V. (2021). Glucocerebrosidase Mutations: A Paradigm for Neurodegeneration Pathways. Free Radic. Biol. Med. 175, 42–55. doi:10.1016/j.freeradbiomed.2021.08.230
Weber, M., Min, S.-W., Truong, T., Hung, J., Dale, S., Reichelt, M., et al. (2021). Ocular Phenotypes in a Mouse Model of Impaired Glucocerebrosidase Activity. Sci. Rep. 11 (1), 6079. doi:10.1038/s41598-021-85528-4
Xiao, J., Jin, K., Wang, J., Ma, J., Zhang, J., Jiang, N., et al. (2017). Conditional Knockout of TFPI-1 in VSMCs of Mice Accelerates Atherosclerosis by Enhancing AMOT/YAP Pathway. Int. J. Cardiol. 228, 605–614. doi:10.1016/j.ijcard.2016.11.195
Xu, Y.-H., Quinn, B., Witte, D., and Grabowski, G. A. (2003). Viable Mouse Models of Acid β-Glucosidase Deficiency. Am. J. Pathology 163 (5), 2093–2101. doi:10.1016/s0002-9440(10)63566-3
Zhang, M., Jiang, N., Cui, R., Du, S., Ou, H., Chen, T., et al. (2019). Deregulated lncRNA Expression Profile in the Mouse Lung Adenocarcinomas with KRAS‐G12D Mutation and P53 Knockout. J. Cell. Mol. Med. 23 (10), 6978–6988. doi:10.1111/jcmm.14584
Keywords: Gaucher disease, GBA1 gene, F213I mutation, GD mouse model, partially humanized
Citation: Guo J-n, Guan M, Jiang N, Li N, Li Y-j, Zhang J and Ma D (2022) Establishment and Phenotypic Analysis of the Novel Gaucher Disease Mouse Model With the Partially Humanized Gba1 Gene and F213I Mutation. Front. Genet. 13:892457. doi: 10.3389/fgene.2022.892457
Received: 14 March 2022; Accepted: 06 May 2022;
Published: 27 May 2022.
Edited by:
Long Guo, RIKEN Center for Integrative Medical Sciences, JapanReviewed by:
Ying Sun, Cincinnati Children’s Hospital Medical Center, United StatesNeal Weinreb, University of Miami, United States
Copyright © 2022 Guo, Guan, Jiang, Li, Li, Zhang and Ma. This is an open-access article distributed under the terms of the Creative Commons Attribution License (CC BY). The use, distribution or reproduction in other forums is permitted, provided the original author(s) and the copyright owner(s) are credited and that the original publication in this journal is cited, in accordance with accepted academic practice. No use, distribution or reproduction is permitted which does not comply with these terms.
*Correspondence: Jin Zhang, SmluWmhhbmdAZnVkYW4uZWR1LmNu; Duan Ma, RHVhbk1hQGZ1ZGFuLmVkdS5jbg==
†These authors have contributed equally to this work