- 1Department of Otolaryngology-Head and Neck Surgery, University of Michigan Health System, Ann Arbor, MI, United States
- 2Department of Internal Medicine, Division of Hematology/Oncology, University of Michigan Medical School, Ann Arbor, MI, United States
- 3Veterans Affairs Ann Arbor Healthcare System, Ann Arbor, MI, United States
- 4Department of Biomedical Engineering, University of Michigan, Ann Arbor, MI, United States
- 5Center for Computational Biology and Bioinformatics, University of Michigan, Ann Arbor, MI, United States
Cancer biomarkers are a promising tool for cancer detection, personalization of therapy, and monitoring of treatment response or recurrence. “Liquid biopsy” commonly refers to minimally invasive or non-invasive sampling of a bodily fluid (i.e., blood, urine, saliva) for detection of cancer biomarkers such as circulating tumor cells or cell-free tumor DNA (ctDNA). These methods offer a means to collect frequent tumor assessments without needing surgical biopsies. Despite much progress with blood-based liquid biopsy approaches, there are limitations—including the limited amount of blood that can be drawn from a person and challenges with collecting blood samples at frequent intervals to capture ctDNA biomarker kinetics. These limitations are important because ctDNA is present at extremely low levels in plasma and there is evidence that measuring ctDNA biomarker kinetics over time can be useful for clinical prediction. Additionally, blood-based assays require access to trained phlebotomists and often a trip to a healthcare facility. In contrast, urine is a body fluid that can be self-collected from a patient’s home, at frequent intervals, and mailed to a laboratory for analysis. Multiple reports indicate that fragments of ctDNA pass from the bloodstream through the kidney’s glomerular filtration system into the urine, where they are known as trans-renal ctDNA (TR-ctDNA). Accumulating studies indicate that the limitations of blood based ctDNA approaches for cancer can be overcome by measuring TR-ctDNA. Here, we review current knowledge about TR-ctDNA in urine as a cancer biomarker approach, and discuss its clinical potential and open questions in this research field.
Introduction
Cancer biomarkers have emerged as promising tools of precision medicine for designing effective treatment regimens, evaluating treatment response, and detecting primary or recurrent cancers. The sampling of body fluid to detect cancer cells or material derived from cancer cells is commonly referred to as “liquid biopsy” (Mader and Pantel 2017). Most commonly, liquid biopsy involves the detection and analysis of circulating tumor cells (CTC), or of fragments of cell-free circulating tumor DNA (ctDNA) that are shed by dying cancer cells into the bloodstream. This offers a minimally-invasive tool to detect and gather information on a patient’s cancer, including characterization of potential tumor genetic heterogeneity, assessment of treatment response, or even emergence of treatment resistance mechanisms (e.g., via detection of specific therapeutic resistance mutations) (Alix-Panabières and Pantel 2021).
Although blood-based liquid biopsy has been clinically successful across many cancer types, this approach still faces limitations, including the limited quantity of blood that can be obtained from a patient for analysis, challenges with obtaining blood samples at high frequency for serial monitoring, and difficulties with reaching patients who have limited access to clinical facilities for phlebotomy. This is relevant because ctDNA is present at extremely low levels in plasma and is undetectable using standard blood sampling volumes in many cancer patients. In addition, there is evidence that measuring ctDNA biomarker kinetics at high time resolution can be useful for clinical prediction, and there is a great need to enable equitable access to healthcare for all members of society.
An alternative approach that has been gaining momentum in the cancer research field is the use of urine as the biofluid specimen for liquid biopsy, in particular for cancers present in organs across the body, rather than just those originating in the urinary tract (e.g., bladder cancer). Whereas cancers present in the urinary tract can directly shed cancer cells or tumor DNA into the urine, there is a phenomenon known as “trans-renal” transit of DNA that enables urine-based access to ctDNA for cancers from distant organs. In this process, fragments of cell-free DNA (cfDNA) present in blood (including ctDNA fragments) are filtered through the kidney’s glomerular filtration system, into the urine (Wang et al. 2017; Moreira et al. 2009; Crisafulli et al. 2019; Lu and Li 2017) (Figure 1). The resulting ctDNA fragments present in the urine are known as trans-renal ctDNA (TR-ctDNA). A key feature of TR-ctDNA is that it allows, in principle, the detection of ctDNA released from cancer in virtually any organ in the body, as long as ctDNA is present in the plasma and able to transit into the urine.
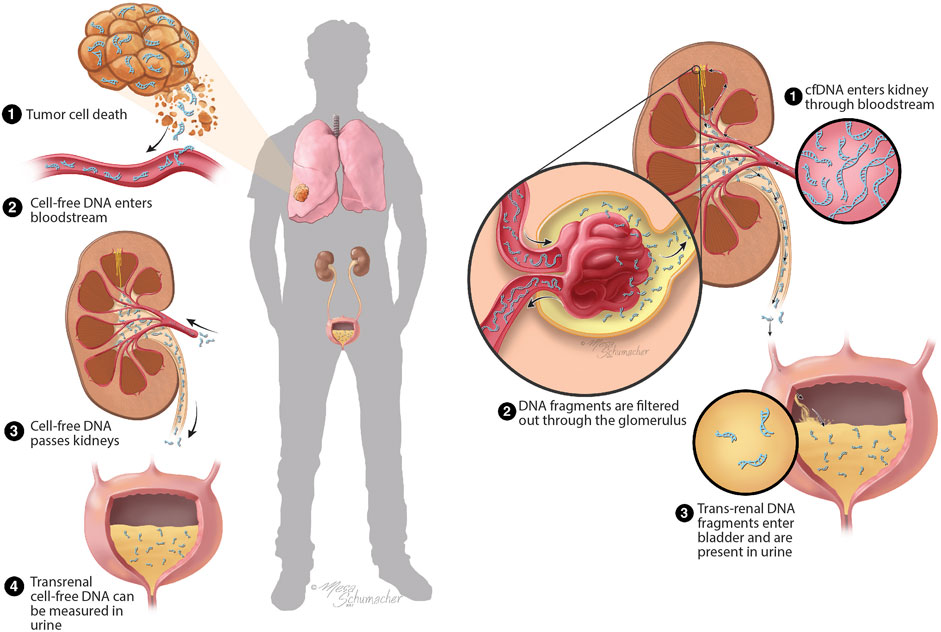
FIGURE 1. Current model of formation of trans-renal ctDNA. The drawing on the left provides a conceptualized overview of the process of ctDNA generation and its transit to the urine. The drawing on the right provides a more detailed conceptualization of the presumed transit of cell-free DNA through the glomerular barrier of the kidney. Abbreviation used in the drawing: cfDNA, cell-free DNA. The artwork in this figure was created by Mesa Schumacher, M.A.
Thus, the phenomenon of trans-renal passage of ctDNA presents an exciting emerging approach for completely non-invasive liquid biopsy with broad potential applications. In this review, we will discuss what is known about the biology of trans-renal DNA, studies to date on TR-ctDNA as a cancer biomarker, its future clinical potential and current gaps in knowledge.
Non-Oncology Studies Establishing the Trans-renal Cell-Free DNA Concept
A seminal study examining the trans-renal cell-free DNA concept was conducted by Botezatu et al. and marks the first demonstration of trans-renal DNA in both animal and human models (Botezatu et al. 2000). The authors sought to assess if cfDNA from the bloodstream crosses the glomerular filtration barrier and can be analyzed via standard genetic techniques. This group examined both human and animal models and described the phenomenon of trans-renal passage of circulating DNA. For the animal experiments, mice were injected with either human Raji cells (a lymphoblast-like human cell line) or radiolabeled DNA. Analysis of urine from the mice injected with human Raji cells demonstrated that human genomic Alu sequences were detectable in the urine. The injected radiolabeled DNA was also detectable in urine in a polymeric form, albeit this represented only a small fraction (∼0.06%) of the total injected DNA (Botezatu et al. 2000). When examining urine of humans receiving blood transfusions, Botezatu et al. found male-specific DNA sequences in females who had received blood transfusion from a male donor, which were presumed to represent DNA that had been present in the transfused blood product and crossed the kidney’s glomerular barrier (Botezatu et al. 2000). Similarly, pregnant females with male fetuses showed detectable male-specific DNA sequences in the mother’s urine (Botezatu et al. 2000).
Although the study of Botezatu et al. provided early proof-of-concept that the renal barrier in both mice and humans is permeable to cfDNA in some form, some early subsequent studies cast controversy over this finding, as they reported an inability to detect cfDNA of male fetuses in the urine of pregnant women carrying male fetuses (Y. Li et al., 2003; Illanes et al., 2006), a result that in retrospect may have been due to differences in urine processing and preservation or other technical differences. This is presumed to be the case because multiple other follow-up studies have confirmed the presence of trans-renal DNA in diverse clinical settings, including in pregnancy (Tsui et al., 2012; Shekhtman et al., 2009; Koide et al., 2005; S. C. Y. Yu et al., 2013), hematopoietic stem cell transplantation (Cheng et al., 2017; Hung et al., 2009), infectious disease (Cannas et al., 2008), and systemic histiocytic disorders (Hyman et al., 2015).
Trans-Renal Cell-Free DNA in Oncology
Soon after the earliest studies of trans-renal cell-free DNA in pregnancy, multiple groups reported investigations using this approach in the setting of tumor-derived DNA in cancer patients. Some of the earliest studies demonstrated proof-of-concept for TR-ctDNA in the setting of colorectal cancer patients, where mutant K-ras DNA (Su et al., 2004, 2005, 2008) and subsequently hypermethylated vimentin gene sequences (B. P. Song et al., 2012) were detected in urine using PCR analysis. Concomitant gel fractionation experiments indicated that TR-ctDNA fragment length is in the 150–250 bp range (Su et al., 2004).
In parallel work, Chan et al. studied TR-ctDNA in EBV-associated nasopharyngeal carcinoma, by assaying the urinary excretion of circulating EBV DNA in relation to plasma EBV DNA levels (Chan et al., 2008). In this form of cancer, the EBV DNA is present in episomal form in the cancer cells, and when released from cells, it represents in effect a form of ctDNA. Using real-time PCR, this group quantified the amount of urine EBV ctDNA of seventy-four patients with nasopharyngeal carcinoma. They found detectable EBV TR-ctDNA in over half of the study cohort and that there was a positive correlation between plasma and urine concentrations of EBV ctDNA. In addition, the authors compared results obtained using 59-bp and 76-bp amplicon assays, which indicated that the EBV sequences found in urine are predominantly <76 bp because of greater signal obtained with the 59 bp assay.
Subsequently, a series of studies focused on detection of EGFR or KRAS mutations as TR-ctDNA in urine of patients with non-small cell lung cancer (Berz et al. 2015; Reckamp et al. 2016; Chen et al. 2017; Tchekmedyian et al. 2017; Husain et al. 2017; Franovic et al. 2017; F. Li et al. 2017; Wang et al. 2017; H. Zhang et al. 2018; Hu et al. 2018; Jin, Gou, and Qian 2019). These studies, some of which used commercial assays available at the time, demonstrated not only that urinary TR-ctDNA is detectable in lung cancer patients, but more specifically that the therapeutic resistance-associated EGFR T790M mutation (Berz et al. 2015; Reckamp et al. 2016; Chen et al. 2017; Franovic et al. 2017; F. Li et al. 2017) could be detected non-invasively through urine. Furthermore, multiple of these reports demonstrated the longitudinal tracking of EGFR mutant TR-ctDNA in non-small cell lung cancer patients over time, including one study that pursued high time-resolution urine collection (i.e., daily collection) and found associations between TR-ctDNA kinetics early during therapy and treatment outcomes (Husain et al. 2017).
More recently, there has been renewed attention to TR-ctDNA in the context of its use as a biomarker for colorectal cancer, with PCR-based assays of targeted mutations (e.g., KRAS, BRAF) (T. Song et al. 2018; H. Yu et al. 2020; Ohta et al. 2021) or methylated DNA loci (Bach et al. 2021) confirming that mutant TR-ctDNA is detectable in the urine of a majority of colorectal cancer patients whose tissue biopsies showed the same mutations. In the Bach et al. study, a combined methylated DNA marker panel was able to detect up to 70% of colorectal cancer patients with 86% specificity (Bach et al. 2021). Furthermore, two recent studies used next-generation sequencing (NGS) to characterize TR-ctDNA in colorectal cancer patients. One study, utilizing whole exome sequencing, found that cancer-specific mutations and copy number changes could be identified in TR-ctDNA and estimated a median length of 112 bp for TR-ctDNA fragments (Crisafulli et al. 2019). The other study utilized targeted NGS to characterize ctDNA in urine, in order to detect minimal residual disease in colorectal cancer patients after neoadjuvant chemotherapy (Pellini et al. 2021). This study also confirmed the detection of TR-ctDNA in a majority, though not all, patients with minimal residual disease after treatment and estimated the average TR-ctDNA length to be 150 bp (Pellini et al. 2021).
One additional next-generation sequencing (NGS) study recently published examined TR-ctDNA in the context of patients with glioma, an aggressive type of brain tumor that is commonly lethal (Mouliere et al. 2021). Using a targeted hybridization-capture NGS technique to look for specific tumor mutations, the investigators found that TR-ctDNA could be detected in six out of eight patients at a pre-surgery urine collection and estimated the median length of the TR-ctDNA fragments to be 101 bp. It is worth noting that some additional NGS- and non-NGS-based studies have detected ctDNA in urine of kidney cancer patients (Smith et al. 2020; Nuzzo et al. 2020; Eisenberger et al. 1999), but will not be discussed further here because it is difficult to know the extent to which such ctDNA represents TR-ctDNA vs. “post-renal” ctDNA that is introduced directly into the urothelial tract through tumor invasion into the renal collecting system.
The TR-ctDNA research field has continued to expand to encompass other types of cancer, including the demonstration of TR-ctDNA detection in patients with breast cancer (Liu and Liu 2018; Guan et al. 2020; J. Zhang, Zhang, and Shen 2020; Zuo et al. 2020), liver cancer (Hann et al. 2017), pancreatic cancer (Terasawa et al. 2019) and gastric cancer (Shi et al. 2017).
Discussion
The expanding base of published literature investigating TR-ctDNA as a biomarker for diverse cancer types highlights the strong potential of this approach for enabling completely non-invasive cancer diagnostics, using a self-collected biospecimen that does not have the collection frequency or sample volume limitations of blood-based liquid biopsies. However, this is still an emerging area and there are multiple gaps in knowledge that need to be addressed as part of successful development of this approach toward effective clinical application.
One of the important questions pertains to the physical nature of TR-ctDNA, in particular the length of fragments that comprise TR-ctDNA, because this impacts the design of TR-ctDNA assays. Literature to date in cancer studies is conflicting, ranging from 150–250 bp size in early studies, to 100–150 bp from sequencing studies, to <78 bp from a quantitative PCR-based study. Although it is tempting to give more credence to the NGS-based studies, they are also subject to technical biases related to the library preparation methods used, including for example, targeted capture-seq methods, which have inherent fragment length biases. Further analysis using sequencing methods that have minimal bias will be important for determining most accurately the size profile of TR-ctDNA in diverse cancer types. A recent NGS study took a step in this direction by characterizing fragmentation patterns of total cell-free DNA in urine from cancer patients and healthy controls (Markus et al. 2021), which were found to differ and were able to distinguish between these two groups. However, because that study focused on total cfDNA and not TR-ctDNA in particular, it is difficult to know to what extent the fragment sizes observed can be extrapolated to describe TR-ctDNA.
Another class of open questions pertains to pre-analytic variables impacting TR-ctDNA analysis, which are important to characterize and where possible, to control, in order to enable reliable diagnostic tests. One of these pre-analytic variables is ensuring stability of TR-ctDNA at the point of collection. At least two studies of the kinetics of urine cfDNA degradation indicate that cfDNA in urine specimens is not stable, decaying under first-order kinetics with a half-life of 2.6–5.1 h in one study (Cheng et al. 2017), and with a half-life that was too short to accurately measure in the other study (Yao et al. 2016). The addition of EDTA at the point of collection has been reported to stabilize cell-free DNA in urine (Lee et al. 2020; Murugesan et al. 2019; Bosschieter et al. 2018), suggesting that endogenous deoxyribonucleases are responsible for the degradation. EDTA has been used as an additive to urine in some, but not all of the cancer studies of TR-ctDNA to date. Furthermore, the final concentrations of EDTA used have been variable, and could contribute to variation across studies. Additional approaches for urine DNA stabilization are also being developed (P. Li et al. 2019). Additional rigorous studies of methods and protocols for urine collection, cell-free DNA stabilization and specimen processing for TR-ctDNA analysis are an essential next step for clinical development of TR-ctDNA-based biomarkers.
Other pre-analytical variables include ones that are more biological in nature, such as the optimal time of day of urine collection, impact of comorbidities such as kidney disease, the impact of medications including certain chemotherapy agents that may be nephrotoxic, as well as variation in hydration status and use of diuretics. Such variables have begun to be examined in the context of TR-ctDNA (Augustus et al. 2020) and assessing their potential impact in specific cancer types may be important to study to increase reliability and consistency in TR-ctDNA assays. It is also not known yet whether approaches that correct for biologically-based, pre-analytic variations such as sample-to-sample variations in glomerular filtration will be of any utility. It may also be possible that much of the confounding effect of biological variations could be overcome by collecting pooled urine samples from a patient over a few days, to average out the impact of factors such as day-to-day variation in glomerular filtration, hydration, etc.
An additional area of research and development is defining the optimal methods and assays for TR-ctDNA biomarker analysis. This includes methods for the initial processing to obtain cell-free urine, extraction of cell-free DNA, and technologies for quantifying specific biomarkers such as mutant or methylated TR-ctDNA fragments. It appears to be important that optimized urine centrifugation protocols are used to prevent contamination with genomic DNA found in cells in the urine (Augustus et al. 2020). Extraction of cell-free DNA from urine can be challenging, in particular from larger volumes (e.g., >10 ml) of urine. Protocols for efficient cfDNA from urine samples of greater volumes are beginning to be developed (Zainabadi et al. 2019), and will benefit from more widespread validation and standardization across studies. Methods for analysis of TR-ctDNA biomarkers are varied, but two very common approaches are droplet digital PCR (ddPCR)-based assays and NGS. The ddPCR approach has the advantage of high sensitivity and reproducibility, high sample throughput and relatively quick turnaround time, and lower cost compared to NGS. However, ddPCR has limited multiplexing capability compared to NGS-based methods and therefore NGS may be preferable in settings where assay of a large number of DNA targets is desirable. It has been reported that the adoption of NGS-based technology reduces the need for laboratory personnel time dedicated to testing activities, which can reduce overall cost of testing per sample (Pisapia et al. 2022). Thus, in appropriate settings, NGS technologies can provide a practical and robust, cost-saving solution for routine analysis (Pisapia et al. 2022; Malapelle et al. 2021).
While there are important gaps in knowledge to be addressed as part of the development process to translate TR-ctDNA tests into the clinic, the recent advances in this field are exciting because they open up opportunities for completely non-invasive ctDNA testing using urine for a broad range of cancer types. Table 1 highlights key TR-ctDNA studies in oncology published thus far, as well as non-oncologic studies that have helped develop the concept of TR-ctDNA. This presents exciting opportunities to advance patient care and clinical research, with myriad potential clinical applications. In addition to applications demonstrated for blood-based ctDNA assays (e.g., tumor genotyping, detecting and monitoring of treatment responses, minimal residual disease detection, and early detection of primary cancer or recurrence), the ability to monitor ctDNA kinetics at high time-resolution (Husain et al. 2017) is uniquely enabled by TR-ctDNA. Furthermore, given that urine can be collected in much larger volumes than blood, it may be possible to increase clinical sensitivity, especially for detection of smaller, early-stage cancers, simply through analysis of large urine volumes. This hypothesis will be important to test in future studies. As understanding and control of pre-analytical variables increases and methods become standardized, this will pave the way for clinical biomarker validation studies in large patient populations to better characterize biomarker performance, which is an essential part of the development process toward clinical translation.
The fact that TR-ctDNA testing involves a completely non-invasive, self-collected sample that can be mailed to a central laboratory is also relevant to development toward the clinic. Multiple studies have shown that patients prefer non-invasive testing options, such as saliva and urine, when possible, as compared to blood testing (Dhima et al. 2013; Koka et al. 2008; Osborne et al. 2018). In light of the COVID-19 pandemic, recent work has also illuminated the importance of self-collected samples for increasing adherence to cancer screening (Gorin et al. 2021). These preferences for bodily fluid collection may influence willingness to participate in research trials and adherence to longitudinal cancer surveillance programs. As such, urine-based assays may improve access for underserved populations and those who cannot readily travel to hospitals and testing facilities. The ability to provide disease surveillance remotely, thus reducing the need for visits to clinical facilities, might also prove to reduce healthcare costs, even in settings where healthcare facility access is not a limiting factor. A recent cost-effectiveness analysis of urine TR-ctDNA for therapy resistance mutation detection in non-small cell lung cancer found a reduced overall cost-of-care using this approach (Sands, Li, and Hornberger 2017).
In conclusion, while much work remains to be done to bring TR-ctDNA testing into widespread clinical use, trans-renal cell-free DNA research has gained momentum over the past 2 decades. It represents an emerging liquid biopsy approach that has potential for broad clinical impact because of the completely non-invasive, self-collected sampling approach and the accumulating base of evidence demonstrating TR-ctDNA detection across diverse cancer types.
Author Contributions
SD, CB and MT drafted the manuscript. PS and JB provided intellectual input and assisted in revising the manuscript. All authors approved the final manuscript.
Funding
This mini-review benefited from institutional support of the authors by the University of Michigan. SD received funding support from a National Institutes of Health Training Grant (NIH/NIDCD T32 DC005356) and from an AHNS Alando J. Ballantyne Resident Research Pilot Grant of the CORE Grants Program of the American Academy of Otolaryngology. CB is an awardee of an American Cancer Society Mission Boost grant (MBGI-22-056-01-MBG).
Conflict of Interest
The authors declare that the research was conducted in the absence of any commercial or financial relationships that could be construed as a potential conflict of interest.
Publisher’s Note
All claims expressed in this article are solely those of the authors and do not necessarily represent those of their affiliated organizations, or those of the publisher, the editors and the reviewers. Any product that may be evaluated in this article, or claim that may be made by its manufacturer, is not guaranteed or endorsed by the publisher.
References
Alix-Panabières, C, and Pantel, K (2021). Liquid Biopsy: From Discovery to Clinical Application. Cancer Discov 11 (4), 858–873. doi:10.1158/2159-8290.CD-20-1311
Augustus, E., Van Casteren, K., Sorber, L., van Dam, P., Roeyen, G., Peeters, M., Vorsters, A., Wouters, A., Raskin, J., Rolfo, C., Zwaenepoel, K., and Pauwels, P. (2020). The Art of Obtaining a High Yield of Cell-Free DNA from Urine. PloS One 15 (4), e0231058. doi:10.1371/journal.pone.0231058
Bach, S., Paulis, I., Sluiter, N. R., Tibbesma, M., Martin, I., van de Wiel, M. A., Tuynman, J. B., Bahce, I., Kazemier, G., and Steenbergen, R. D. M. (2021). Detection of Colorectal Cancer in Urine Using DNA Methylation Analysis. Sci Rep 11 (1), 2363. doi:10.1038/s41598-021-81900-6
Berz, D., Raymond, V. M., Garst, J. H., and Erlander, M. G. (2015). Non-Invasive Urine Testing of EGFR Activating Mutation and T790M Resistance Mutation in Non-Small Cell Lung Cancer. Exp Hematol Oncol 5, 24. doi:10.1186/s40164-016-0052-3
Bosschieter, J., Bach, S., Bijnsdorp, I. V., Segerink, L. I., Rurup, W. F., van Splunter, A. P., Bahce, I., Novianti, P. W., Kazemier, G., van Moorselaar, R. J. A., Steenbergen, R. D. M., and Nieuwenhuijzen, J. A. (2018). A Protocol for Urine Collection and Storage prior to DNA Methylation Analysis. PloS One 13 (8), e0200906. doi:10.1371/journal.pone.0200906
Botezatu, I, Serdyuk, O, Potapova, G, Shelepov, V, Alechina, R, Molyaka, Y, Ananév, V, Bazin, I, Garin, A, Narimanov, M, Knysh, V, Melkonyan, H, Umansky, S, and Lichtenstein, A (2000). Genetic Analysis of DNA Excreted in Urine: A New Approach for Detecting Specific Genomic DNA Sequences from Cells Dying in an Organism. Clin Chem 46 (8 Pt 1), 1078–84. doi:10.1093/clinchem/46.8.1078
1Cannas, A, Goletti, D, Girardi, E, Chiacchio, T, Calvo, L, Cuzzi, G, Piacentini, M, Melkonyan, H, Umansky, SR, Lauria, FN, Ippolito, G, and Tomei, LD (2008). Mycobacterium Tuberculosis DNA Detection in Soluble Fraction of Urine from Pulmonary Tuberculosis Patients. Int J Tuberc Lung Dis 12 (2), 146–51.
Chan, K. C. A., Leung, S. F., Yeung, S. W., Chan, A. T. C., and Lo, Y. M. D. (2008). Quantitative Analysis of the Transrenal Excretion of Circulating EBV DNA in Nasopharyngeal Carcinoma Patients. Clin Cancer Res 14 (15), 4809–4813. doi:10.1158/1078-0432.ccr-08-1112
Cheng, T. H. T., Jiang, P., Tam, J. C. W., Sun, X., Lee, W.-S., Yu, S. C. Y., Teoh, J. Y. C., Chiu, P. K. F., Ng, C.-F., Chow, K.-M., Szeto, C.-C., Chan, K. C. A., Chiu, R. W. K., and Lo, Y. M. D. (2017). Genomewide Bisulfite Sequencing Reveals the Origin and Time-Dependent Fragmentation of Urinary cfDNA. Clinical Biochemistry 50 (9), 496–501. doi:10.1016/j.clinbiochem.2017.02.017
Chen, S., Zhao, J., Cui, L., and Liu, Y. (2017). Urinary Circulating DNA Detection for Dynamic Tracking of EGFR Mutations for NSCLC Patients Treated with EGFR-TKIs. Clin Transl Oncol: Official Publication of the Federation of Spanish Oncology Societies and of the National Cancer Institute of Mexico 19 (3), 332–340. doi:10.1007/s12094-016-1534-9
Crisafulli, G., Mussolin, B., Cassingena, A., Montone, M., Bartolini, A., Barault, L., Martinetti, A., Morano, F., Pietrantonio, F., Sartore-Bianchi, A., Siena, S., Di Nicolantonio, F., Marsoni, S., Bardelli, A., and Siravegna, G. (2019). Whole Exome Sequencing Analysis of Urine Trans-Renal Tumour DNA in Metastatic Colorectal Cancer Patients. ESMO Open 4 (6), e000572. doi:10.1136/esmoopen-2019-000572
Dhima, M., Salinas, T. J., Wermers, R. A., Weaver, A. L., and Koka, S. (2013). Preference Changes of Adult Outpatients for Giving Saliva, Urine and Blood for Clinical Testing after Actual Sample Collection. Journal of Prosthodontic Research 57 (1), 51–56. doi:10.1016/j.jpor.2012.09.004
Eisenberger, C. F., Schoenberg, M., Enger, C., Hortopan, S., Shah, S., Chow, N.-H., Marshall, F. F., and Sidransky, D. (1999). Diagnosis of Renal Cancer by Molecular Urinalysis. JNCI Journal of the National Cancer Institute 91 (23), 2028–2032. doi:10.1093/jnci/91.23.2028
Franovic, A, Raymond, VM, Erlander, MG, and Reckamp, KL (2017). Urine Test for EGFR Analysis in Patients with Non-Small Cell Lung Cancer. J Thorac Dis 9 (Suppl 13), S1323–31. doi:10.21037/jtd.2017.06.144
García Moreira, V, Prieto GarcíaGarcía, B, de la Cera Martínez, T, Alvarez Menéndez, FV, and Alvarez, M (2009). Elevated Transrenal DNA (cell-Free Urine DNA) in Patients with Urinary Tract Infection Compared to Healthy Controls. Clin Biochem 42 (7-8), 729–31. doi:10.1016/j.clinbiochem.2008.12.021
Gorin, S. N. S., Jimbo, M., Heizelman, R., Harmes, K. M., and Harper, D. M. (2021). The future of cancer screening after COVID‐19 may be at home. Cancer 127 (4), 498–503. doi:10.1002/cncr.33274
Guan, G., Wang, Y., Sun, Q., Wang, L., Xie, F., Yan, J., Huang, H., and Liu, H. (2020). Utility of Urinary ctDNA to Monitoring Minimal Residual Disease in Early Breast Cancer Patients. Cbm 28 (1), 111–119. doi:10.3233/cbm-190523
Hann, HW, Jain, S, Park, G, Steffen, JD, Song, W, and Su, YH (2017). Detection of Urine DNA Markers for Monitoring Recurrent Hepatocellular Carcinoma. Hepatoma Res 3 (June), 105–111. doi:10.20517/2394-5079.2017.15
Hung, E. C., Shing, T. K., Chim, S. S., Yeung, P. C., Chan, R. W. Y., Chik, K. W., Lee, V., Tsui, N. B. Y., Li, C.-K., Wong, C. S. C., Chiu, R. W. K., and Lo, Y. M. D. (2009). Presence of Donor-Derived DNA and Cells in the Urine of Sex-Mismatched Hematopoietic Stem Cell Transplant Recipients: Implication for the Transrenal Hypothesis. Clinical Chemistry 55 (4), 715–722. doi:10.1373/clinchem.2008.113530
Husain, H., Melnikova, V. O., Kosco, K., Woodward, B., More, S., Pingle, S. C., Weihe, E., Park, B. H., Tewari, M., Erlander, M. G., Cohen, E., Lippman, S. M., and Kurzrock, R. (2017). Monitoring Daily Dynamics of Early Tumor Response to Targeted Therapy by Detecting Circulating Tumor DNA in Urine. Clin Cancer Res 23 (16), 4716–4723. doi:10.1158/1078-0432.ccr-17-0454
Hu, T., Shen, H., Huang, H., Song, M., Yang, Z., Zhou, Y., and Zhao, G. (2018). Urinary Circulating DNA Profiling in Non-Small Cell Lung Cancer Patients Following Treatment Shows Prognostic Potential. J. Thorac. Dis. 10 (7), 4137–4146. doi:10.21037/jtd.2018.06.50
Hyman, D. M., Diamond, E. L., Vibat, C. R. T., Hassaine, L., Poole, J. C., Patel, M., Holley, V. R., Cabrilo, G., Lu, T. T., Arcila, M. E., Chung, Y. R., Rampal, R., Lacouture, M. E., Rosen, N., Meric-Bernstam, F., Baselga, J., Kurzrock, R., Erlander, M. G., Janku, F., and Abdel-Wahab, O. (2015). Prospective Blinded Study of BRAFV600E Mutation Detection in Cell-Free DNA of Patients with Systemic Histiocytic Disorders. Cancer Discovery 5 (1), 64–71. doi:10.1158/2159-8290.cd-14-0742
Illanes, S., Denbow, M. L., Smith, R. P., Overton, T. G., Soothill, P. W., and Finning, K. (2006). Detection of Cell-Free Fetal DNA in Maternal Urine. Prenat. Diagn. 26 (13), 1216–1218. doi:10.1002/pd.1591
Jin, P. C., Gou, B., and Qian, W. (2019). Urinary Markers in Treatment Monitoring of Lung Cancer Patients with Bone Metastasis. Int J Biol Markers 34 (3), 243–250. doi:10.1177/1724600819848762
Koide, K., Sekizawa, A., Iwasaki, M., Matsuoka, R., Honma, S., Farina, A., Saito, H., and Okai, T. (2005). Fragmentation of Cell-Free Fetal DNA in Plasma and Urine of Pregnant Women. Prenat. Diagn. 25 (7), 604–607. doi:10.1002/pd.1213
Koka, S., Beebe, T. J., Merry, S. P., DeJesus, R. S., Berlanga, L. D., Weaver, A. L., Montori, V. M., and Wong, D. T. (2008). The Preferences of Adult Outpatients in Medical or Dental Care Settings for Giving Saliva, Urine or Blood for Clinical Testing. The Journal of the American Dental Association 139 (6), 735–740. doi:10.14219/jada.archive.2008.0255
Lee, E. Y., Lee, E.-J., Yoon, H., LeeLee, D. H., and Kim, K. H. (2020). Comparison of Four Commercial Kits for Isolation of Urinary Cell-Free DNA and Sample Storage Conditions. Diagnostics 10 (4), 234. doi:10.3390/diagnostics10040234
Li, F., Huang, J., Ji, D., Meng, Q., Wang, C., Chen, S., Wang, X., Zhu, Z., Jiang, C., Shi, Y., Liu, S., and Li, C. (2017). Utility of Urinary Circulating Tumor DNA for EGFR Mutation Detection in Different Stages of Non-Small Cell Lung Cancer Patients. Clin Transl Oncol: Official Publication of the Federation of Spanish Oncology Societies and of the National Cancer Institute of Mexico 19 (10), 1283–1291. doi:10.1007/s12094-017-1669-3
Li, P., Ning, J., Luo, X., Du, H., Zhang, Q., Zhou, G., Du, Q., Ou, Z., Wang, L., and Wang, Y. (2019). New Method to Preserve the Original Proportion and Integrity of Urinary Cell-Free DNA. J Clin Lab Anal 33 (2), e22668. doi:10.1002/jcla.22668
Liu, Z., and Liu, W. (2018). Association of Urinary and Plasma DNA in Early Breast Cancer Patients and Its Links to Disease Relapse. Clin Transl Oncol: Official Publication of the Federation of Spanish Oncology Societies and of the National Cancer Institute of Mexico 20 (8), 1053–1060. doi:10.1007/s12094-017-1825-9
Li, Y., Zhong, X. Y., Kang, A., Troeger, C., Holzgreve, W., and Hahn, S. (2003). Inability to Detect Cell Free Fetal DNA in the Urine of Normal Pregnant Women nor in Those Affected by Preeclampsia Associated HELLP Syndrome. Journal of the Society for Gynecologic Investigation 10 (8), 503–508. doi:10.1016/s1071-55760300155-2
Lu, T, and Li, J (2017). Clinical Applications of Urinary Cell-Free DNA in Cancer: Current Insights and Promising Future. Am J Cancer Res 7 (11), 2318–2332.
Mader, S, and Pantel, K (2017). Liquid Biopsy: Current Status and Future Perspectives. Oncol Res Treat 40 (7-8), 404–408. doi:10.1159/000478018
Malapelle, U., Pepe, F., Pisapia, P., Sgariglia, R., Nacchio, M., Barberis, M., Bilh, M., et al. (2021). TargetPlex FFPE-Direct DNA Library Preparation Kit for SiRe NGS Panel: An International Performance Evaluation Study. Journal of Clinical Pathology 1, 1. doi:10.1136/jclinpath-2021-207450
Markus, H., Zhao, J., Contente-Cuomo, T., Stephens, M. D., Raupach, E., Odenheimer-Bergman, A., Connor, S., McDonald, B. R., Moore, B., Hutchins, E., McGilvrey, M., de la Maza, M. C., Van Keuren-Jensen, K., Pirrotte, P., Goel, A., Becerra, C., Von Hoff, D. D., Celinski, S. A., Hingorani, P., and Murtaza, M. (2021). Analysis of Recurrently Protected Genomic Regions in Cell-Free DNA Found in Urine. Sci. Transl. Med. 13 (581), eaaz3088. doi:10.1126/scitranslmed.aaz3088
Mouliere, F, Smith, CG, Heider, K, Su, J, van der Pol, Y, Thompson, M, Morris, J, Wan, JCM, Chandrananda, D, Hadfield, J, Grzelak, M, Hudecova, I, Couturier, DL, Cooper, W, Zhao, H, Gale, D, Eldridge, M, Watts, C, Brindle, K, Rosenfeld, N, and Mair, R (2021). Fragmentation Patterns and Personalized Sequencing of Cell-Free DNA in Urine and Plasma of Glioma Patients. EMBO Mol Med 13 (8), e12881. doi:10.15252/emmm.202012881
Murugesan, K., Hogan, C. A., Palmer, Z., Reeve, B., Theron, G., Andama, A., Somoskovi, A., Steadman, A., Madan, D., Andrews, J., Croda, J., Sahoo, M. K., Cattamanchi, A., Pinsky, B. A., and Banaei, N. (2019). Investigation of Preanalytical Variables Impacting Pathogen Cell-Free DNA in Blood and Urine. J Clin Microbiol 57 (11), e00782–19. doi:10.1128/JCM.00782-19
Nuzzo, P. V., Berchuck, J. E., Korthauer, K., Spisak, S., Nassar, A. H., Abou Alaiwi, S., Chakravarthy, A., Shen, S. Y., Bakouny, Z., Boccardo, F., Steinharter, J., Bouchard, G., Curran, C. R., Pan, W., Baca, S. C., Seo, J.-H., Lee, G.-S. M., Michaelson, M. D., Chang, S. L., Waikar, S. S., Sonpavde, G., Irizarry, R. A., Pomerantz, M., De Carvalho, D. D., Choueiri, T. K., and Freedman, M. L. (2020). Detection of Renal Cell Carcinoma Using Plasma and Urine Cell-Free DNA Methylomes. Nat Med 26 (7), 1041–1043. doi:10.1038/s41591-020-0933-1
Ohta, R., Yamada, T., Sonoda, H., Matsuda, A., Shinji, S., Takahashi, G., Iwai, T., Takeda, K., Ueda, K., Kuriyama, S., Miyasaka, T., Yokoyama, Y., Hara, K., and Yoshida, H. (2021). Detection of KRAS Mutations in Circulating Tumour DNA from Plasma and Urine of Patients with Colorectal Cancer. European Journal of Surgical Oncology 47, 3151–3156. doi:10.1016/j.ejso.2021.07.017
Osborne, J., Flight, I., Wilson, C. J., Chen, G., Ratcliffe, J., and Young, G. (2018). The Impact of Sample Type and Procedural Attributes on Relative Acceptability of Different Colorectal Cancer Screening Regimens. Ppa 12 (September), 1825–1836. doi:10.2147/ppa.s172143
Pellini, B., Pejovic, N., Feng, W., Earland, N., Harris, P. K., Usmani, A., Szymanski, J. J., Qaium, F., Mudd, J., Petty, M., Jiang, Y., Singh, A., Maher, C. A., Henke, L. E., Park, H., Ciorba, M. A., Kim, H., Mutch, M. G., Pedersen, K. S., Tan, B. R., Hawkins, W. G., Fields, R. C., and Chaudhuri, A. A. (2021). ctDNA MRD Detection and Personalized Oncogenomic Analysis in Oligometastatic Colorectal Cancer From Plasma and Urine. JCO Precision Oncology 5 (February), 378–388. doi:10.1200/PO.20.00276
Pisapia, P., Pepe, F., Baggi, A., Barberis, M., Galvano, A., Gristina, V., Mastrilli, F., Novello, S., Pagni, F., Pasini, S., Perrone, G., Righi, D., Russo, A., Troncone, G., and Malapelle, U. (2022). Next Generation Diagnostic Algorithm in Non-Small Cell Lung Cancer Predictive Molecular Pathology: The KWAY Italian Multicenter Cost Evaluation Study. Critical Reviews in Oncology/hematology 169 (January), 103525. doi:10.1016/j.critrevonc.2021.103525
Reckamp, K. L., Melnikova, V. O., Karlovich, C., Sequist, L. V., Camidge, D. R., Wakelee, H., Perol, M., Oxnard, G. R., Kosco, K., Croucher, P., Samuelsz, E., Vibat, C. R., Guerrero, S., Geis, J., Berz, D., Mann, E., Matheny, S., Rolfe, L., Raponi, M., Erlander, M. G., and Gadgeel, S. (2016). A Highly Sensitive and Quantitative Test Platform for Detection of NSCLC EGFR Mutations in Urine and Plasma. Journal of Thoracic Oncology 11 (10), 1690–1700. doi:10.1016/j.jtho.2016.05.035
Sands, J., Li, Q., and Hornberger, J. (2017). Urine Circulating-Tumor DNA (ctDNA) Detection of Acquired EGFR T790M Mutation in Non-Small-Cell Lung Cancer: An Outcomes and Total Cost-of-Care Analysis. Lung Cancer 110 (August), 19–25. doi:10.1016/j.lungcan.2017.05.014
Shekhtman, E. M., Anne, K., Melkonyan, H. S., Robbins, D. J., Warsof, S. L., and Umansky, S. R. (2009). Optimization of Transrenal DNA Analysis: Detection of Fetal DNA in Maternal Urine. Clinical Chemistry 55 (4), 723–729. doi:10.1373/clinchem.2008.113050
Shi, X.-Q., Xue, W.-H., Zhao, S.-F., Zhang, X.-J., and Sun, W. (2017). Dynamic Tracing for Epidermal Growth Factor Receptor Mutations in Urinary Circulating DNA in Gastric Cancer Patients. Tumour Biology: The Journal of the International Society for Oncodevelopmental Biology and Medicine 39 (2), 1010428317691681. doi:10.1177/1010428317691681
Smith, C. G., Moser, T., Mouliere, F., Field-Rayner, J., Eldridge, M., Riediger, A. L., Chandrananda, D., Heider, K., Wan, J. C. M., Warren, A. Y., Morris, J., Hudecova, I., Cooper, W. N., Mitchell, T. J., Gale, D., Ruiz-Valdepenas, A., Klatte, T., Ursprung, S., Sala, E., Riddick, A. C. P., Aho, T. F., Armitage, J. N., Perakis, S., Pichler, M., Seles, M., Wcislo, G., Welsh, S. J., Matakidou, A., Eisen, T., Massie, C. E., Rosenfeld, N., Heitzer, E., and Stewart, G. D. (2020). Comprehensive Characterization of Cell-Free Tumor DNA in Plasma and Urine of Patients with Renal Tumors. Genome Med 12 (1), 23. doi:10.1186/s13073-020-00723-8
Song, B. P., Jain, S., Lin, S. Y., Chen, Q., Block, T. M., Song, W., Brenner, D. E., and Su, Y.-H. (2012). Detection of Hypermethylated Vimentin in Urine of Patients with Colorectal Cancer. The Journal of Molecular Diagnostics 14 (2), 112–119. doi:10.1016/j.jmoldx.2011.12.003
Song, T., Mao, F., Shi, L., Xu, X., Wu, Z., Zhou, J., and Xiao, M. (2018). Urinary Measurement of Circulating Tumor DNA for Treatment Monitoring and Prognosis of Metastatic Colorectal Cancer Patients. Clinical Chemistry and Laboratory Medicine: CCLM/FESCC 57 (2), 268–275. doi:10.1515/cclm-2017-0675
Su, YH, Wang, M, Aiamkitsumrit, B, Brenner, DE, and Block, TM (2005). Detection of a K-Ras Mutation in Urine of Patients with Colorectal Cancer. Cancer Biomark 1 (2-3), 177–82. doi:10.3233/cbm-2005-12-305
Su, Y.-H., Wang, M., Brenner, D. E., Ng, A., Melkonyan, H., Umansky, S., Syngal, S., Block, T. M., and Block, (2004). Human Urine Contains Small, 150 to 250 Nucleotide-Sized, Soluble DNA Derived from the Circulation and May Be Useful in the Detection of Colorectal Cancer. The Journal of Molecular Diagnostics 6 (2), 101–107. doi:10.1016/s1525-1578(10)60497-7
Su, YH, Wang, M, Brenner, DE, Norton, PA, and Block, TM (2008). Detection of Mutated K-Ras DNA in Urine, Plasma, and Serum of Patients with Colorectal Carcinoma or Adenomatous Polyps. Ann N Y Acad Sci 1137 (August), 197–206. doi:10.1196/annals.1448.027
Tchekmedyian, N., Mudad, R., BlancoRaymond, F. F.Victoria M., Raymond, V. M., Garst, J., Erlander, M. G., Haura, E., and Berz, D. (2017). Longitudinal Monitoring of ctDNA EGFR Mutation Burden from Urine Correlates with Patient Response to EGFR TKIs: A Case Series. Lung Cancer 108 (June), 22–28. doi:10.1016/j.lungcan.2017.02.010
Terasawa, H., Kinugasa, H., Ako, S., Hirai, M., Matsushita, H., Uchida, D., Tomoda, T., Matsumoto, K., Horiguchi, S., Kato, H., Nouso, K., and Okada, H. (2019). Utility of Liquid Biopsy Using Urine in Patients with Pancreatic Ductal Adenocarcinoma. Cancer Biology & Therapy 20 (10), 1348–1353. doi:10.1080/15384047.2019.1638685
Tsui, N. B. Y., Jiang, P., Chow, K. C. K., Su, X., Leung, T. Y., Sun, H., Chan, K. C. A., Chiu, R. W. K., and Lo, Y. M. D. (2012). High Resolution Size Analysis of Fetal DNA in the Urine of Pregnant Women by Paired-End Massively Parallel Sequencing. PloS One 7 (10), e48319. doi:10.1371/journal.pone.0048319
Wang, X, Meng, Q, Wang, C, Li, F, Zhu, Z, Liu, S, Shi, Y, Huang, J, Chen, S, and Li, C (2017). Investigation of Transrenal KRAS Mutation in Late Stage NSCLC Patients Correlates to Disease Progression. Biomarkers 22 (7), 654–660. doi:10.1080/1354750X.2016.1269202
Yao, W., Mei, C., Nan, X., and Hui, L. (2016). Evaluation and Comparison of in Vitro Degradation Kinetics of DNA in Serum, Urine and Saliva: A Qualitative Study. Gene 590 (1), 142–148. doi:10.1016/j.gene.2016.06.033
Yu, H, Han, L, Yuan, J, and Sun, Y (2020). Circulating Tumor Cell Free DNA from Plasma and Urine in the Clinical Management of Colorectal Cancer. Cancer Biomark 27 (1), 29–37. doi:10.3233/CBM-182344
Yu, S. C., Lee, S. W., Jiang, P., Leung, T. Y., Chan, K. A., Chiu, R. W., and Lo, Y. D. (2013). High-Resolution Profiling of Fetal DNA Clearance from Maternal Plasma by Massively Parallel Sequencing. Clinical Chemistry 59 (8), 1228–1237. doi:10.1373/clinchem.2013.203679
Zainabadi, K., Dhayabaran, V., Moideen, K., and Krishnaswamy, P. (2019). An Efficient and Cost-Effective Method for Purification of Small Sized DNAs and RNAs from Human Urine. PloS One 14 (2), e0210813. doi:10.1371/journal.pone.0210813
Zhang, H., He, B., Cui, J., Zhao, M., and Zhang, Z. (2018). Comparison of Circulating DNA from Plasma and Urine for EGFR Mutations in NSCLC Patients. Cbm 23 (3), 427–436. doi:10.3233/cbm-181511
Zhang, J., Zhang, X., and Shen, S. (2020). Treatment and Relapse in Breast Cancer Show Significant Correlations to Noninvasive Testing Using Urinary and Plasma DNA. Future Oncology 16 (13), 849–858. doi:10.2217/fon-2020-0074
Keywords: trans-renal, liquid biopsy, cell-free DNA, circulating tumor DNA, ctDNA, urine, biomarker, cancer
Citation: Dermody SM, Bhambhani C, Swiecicki PL, Brenner JC and Tewari M (2022) Trans-Renal Cell-Free Tumor DNA for Urine-Based Liquid Biopsy of Cancer. Front. Genet. 13:879108. doi: 10.3389/fgene.2022.879108
Received: 18 February 2022; Accepted: 07 April 2022;
Published: 27 April 2022.
Edited by:
Yu Zhao, Sun Yat-sen University, ChinaReviewed by:
Valerio Gristina, University of Palermo, ItalyCopyright © 2022 Dermody, Bhambhani, Swiecicki, Brenner and Tewari. This is an open-access article distributed under the terms of the Creative Commons Attribution License (CC BY). The use, distribution or reproduction in other forums is permitted, provided the original author(s) and the copyright owner(s) are credited and that the original publication in this journal is cited, in accordance with accepted academic practice. No use, distribution or reproduction is permitted which does not comply with these terms.
*Correspondence: Muneesh Tewari, bXRld2FyaUBtZWQudW1pY2guZWR1